DOI:
10.1039/D1SC05391D
(Perspective)
Chem. Sci., 2022,
13, 3315-3334
Distinctive features and challenges in catenane chemistry
Received
30th September 2021
, Accepted 4th February 2022
First published on 7th February 2022
Abstract
From being an aesthetic molecular object to a building block for the construction of molecular machines, catenanes and related mechanically interlocked molecules (MIMs) continue to attract immense interest in many research areas. Catenane chemistry is closely tied to that of rotaxanes and knots, and involves concepts like mechanical bonds, chemical topology and co-conformation that are unique to these molecules. Yet, because of their different topological structures and mechanical bond properties, there are some fundamental differences between the chemistry of catenanes and that of rotaxanes and knots although the boundary is sometimes blurred. Clearly distinguishing these differences, in aspects of bonding, structure, synthesis and properties, between catenanes and other MIMs is therefore of fundamental importance to understand their chemistry and explore the new opportunities from mechanical bonds.
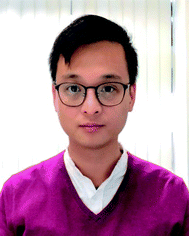 Ho Yu Au-Yeung | Ho Yu Au-Yeung is currently an Associate Professor at the Department of Chemistry, The University of Hong Kong. He obtained his PhD from the University of Cambridge (2010) and was a Croucher Postdoctoral Fellow at UC Berkeley (2011–2013). His group is interested in supramolecular and mechanical bond chemistry. He is the recipient of the Croucher Innovation Award (2016), the Graeme Hanson Early Career Researcher Award (2016), Asian Core Program Lectureship Award (Taiwan, 2018) and HKU Outstanding Young Researcher Award (2020), and a member of the Early Career Advisory Board of Chemical Reviews (2020–2021). |
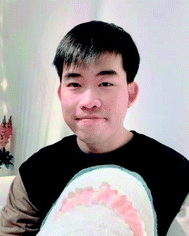 Yulin Deng | Yulin Deng received his BEng degree from South China University of Technology and finished his dissertation under the supervision of Anjun Qin. He joined the group of Ho Yu Au-Yeung in 2019 to pursue his PhD at The University of Hong Kong, and works on complex catenane synthesis and responsive catenanes. |
1. Introduction
Catenanes are a representative class of mechanically interlocked molecules (MIMs) that consist of interlocked macrocycles.1,2 It is the well-defined physical entanglement, or the mechanical bond, between the interlocked rings that characterises the many unique features of catenanes. In addition to catenanes, molecular entanglements also exist in rotaxanes, knots, and other related interlocked molecules, and the efficient formation and characterisation of the well-defined, entangled molecular structures are therefore a major focus in the early development of the chemistry of MIMs.1a,3 As such, catenane chemistry is highly related to and often discussed together with that of rotaxanes and knots. Owing to the different topologies, nature and properties of the entanglement in catenanes and other MIMs, there are however fundamental differences in the chemistry of these molecules (Fig. 1). Clearly distinguishing the features and properties, especially those related to the entanglement, in each type of MIM is therefore highly important for a deeper discussion of their chemistry, particularly when properties and applications are concerned.
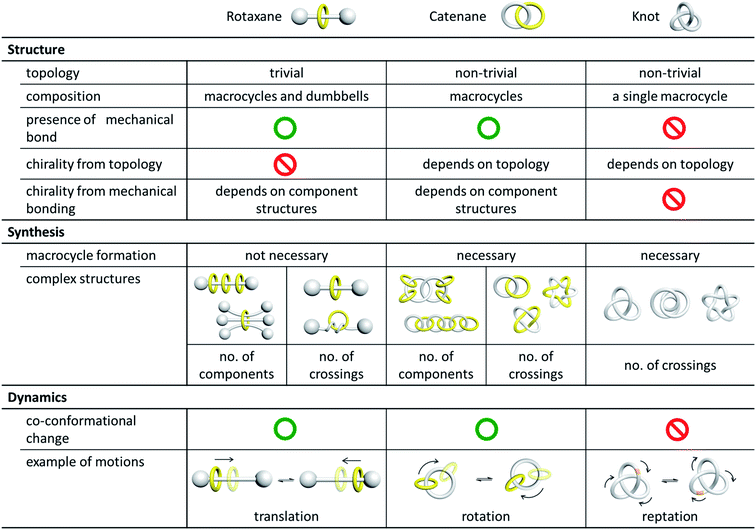 |
| Fig. 1 Comparison of catenanes, rotaxanes and knots. | |
In this perspective, we aim at discussing the distinctive features in the bonding, synthesis, structures and properties of catenanes. Similarities and differences between catenanes, rotaxanes and knots in these aspects will be highlighted when appropriate, to give a clearer picture on the uniqueness and future challenges in further advancing catenane chemistry in both fundamental and application perspectives. Instead of discussing specific examples in detail, we hope to give a more general discussion on the underlying considerations on the chemistry of catenanes. In particular, when applications are concerned, we highlight the importance of entanglement tightness and some other basic properties of the mechanical bond in catenanes that are fundamental to that specific catenane application. Some research challenges to further advance the chemistry of catenanes and MIMs are also discussed.
2. Bonding, topology and structure
2.1 Mechanical interlocking and mechanical bond
Catenanes, rotaxanes and knots are often discussed together in the literature as these are the three most representative classes of interlocked molecules that feature physical entanglement. Structurally, a catenane consists of only interlocked rings, while a rotaxane contains both macrocyclic and linear components that are interlocked. As such, there are at least two or more “molecular components” in both a catenane and a rotaxane, but a knot is one single entwined macrocycle. The interlocked macrocycles in a catenane, or the interlocked macrocycles and dumbbells in a rotaxane, are described as being held together by mechanical bonds,2a and the total number of interlocked components is indicated by the prefix n in [n]catenane and [n]rotaxane. Since the entanglement in a knot is formed within itself in the single macrocyclic component, no mechanical bond is present in a molecular knot.
In addition to van der Waals forces, it is common that metal–ligand interactions, hydrogen bonds, and other interactions of various strength and exchange rate are present between (or even within) the interlocked components in a catenane or rotaxane, and whether or not these interactions are to be considered in the classification and nomenclature of the corresponding MIM may be different in different cases. For example, while a MIM composed of two interlocked rings is now usually referred to as a [2]catenane irrespective of the type of the inter-component interactions, the rotaxane reported by Pöthig is named as [2]- and [3]rotaxane when the two pyrazole–imidazole macrocycles are connected (by Ag+ coordination) and disconnected respectively (Fig. 2).4 Some multinuclear Au(I) catenanes may also be considered as gold(I) nanoclusters with the presence of the multiple Au(I)⋯Au(I) interactions.5 If a dynamic covalent bond exists between the interlocked components, the number of interlocked components will also be dependent on the kinetics and thermodynamics of the dynamic covalent bonds under specific conditions.6 Although the nature of the inter-component interactions may present a grey area in the naming of some MIMs, more importantly, the presence of these interactions will directly influence the structure, dynamics and tightness of the entanglement, and hence the properties and applications of the MIMs. In fact, entanglement tightness is one important parameter that describes the interlocking in MIMs, which is in turn dependent on the physical size, number of crossings and rigidity of the MIM. More details will be discussed in the sections below.
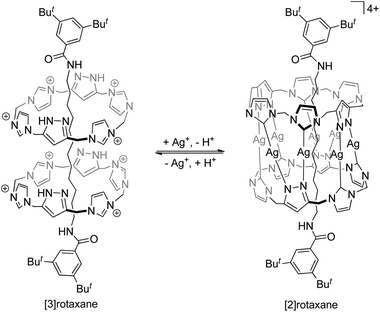 |
| Fig. 2 The rotaxanes reported by Pöthig and co-workers referred to as [3]- and [2]rotaxane when the interlocked macrocycles are separated and connected by Ag+ ions respectively.4 | |
2.2 Topology and structure
Catenanes and knots are topologically non-trivial and the entanglement cannot be resolved without breaking a covalent bond, or in other words, the MIMs and non-interlocked counterparts cannot be interconverted by continuous deformation.7 A rotaxane, on the other hand, is topologically trivial because the interlocked macrocycle and dumbbell can be separated if the macrocycle is stretched and slips through the dumbbell. In mathematical terms, a catenane is a link, and the knot theory encompasses the study of knots and links .8 A link and a knot can be classified as being prime or composite, in which a prime link and a prime knot cannot be described by a sum of other links and knots, similar to the idea of prime and composite numbers. The Alexander–Briggs notation (Xyz) is used to describe the topology of prime links and knots, in which x, y and z represent respectively the number of crossings, the number of discrete components and the arbitrary order of the link and knot in the knot table.9 For example, the Hopf link (221) is a simple [2]catenane with two crossings, and two non-interlocked macrocycles is a link with two components and zero crossings (021).
High-order [n]catenanes with multiple interlocked macrocycles are usually composite links and the Alexander–Briggs notation is not applicable in describing their topology and structure. The way how the interlocked macrocycles are arranged is often used to describe the structure of [n]catenanes (Fig. 3).1a,2a The most commonly known [n]catenanes are radial catenanes (also known as molecular necklaces), which consist of n − 1 peripheral macrocycles interlocked on one same central macrocycle. Linear and branched [n]catenanes are more difficult to synthesise and therefore rarer. When the number of interlocked rings and crossings in an [n]catenane increases, identification and description of the structure and topology would become more difficult, and a clear presentation of the 3D spatial relationship of the interlocked components will be essential. On the other hand, a rotaxane in which multiple macrocycles are interlocked on one dumbbell is the most prevalent among all possible [n]rotaxane architectures,10 but an [n]rotaxane with one macrocycle and multiple dumbbells, known as molecular sheaf, is relatively rare.11
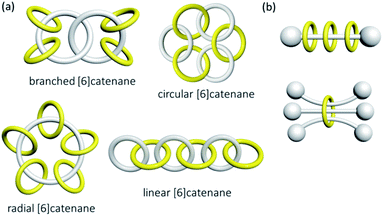 |
| Fig. 3 Possible structures of (a) [6]catenanes and (b) [4]rotaxanes. | |
2.3 Isomerism
In the presence of a mechanical bond, the spatial relationship of the constituting atoms in catenanes extends beyond that of covalent connection and new types of isomerism become possible when the interlocked components are differently arranged. The resulting isomers may or may not be interconvertible, depending on the topology, physical size and dynamics of the MIM and constituting interlocked components.
A common and perhaps the most well-known type of isomerism in catenanes and rotaxanes is co-conformational isomerism (Fig. 4). Taking a simple [2]catenane (i.e. Hopf link) as an example, different co-conformations will be obtained when the two interlocked macrocycles adopt different relative orientations. Interconversion between catenane co-conformers by motions such as circumrotation is possible depending on the thermodynamic and kinetic properties of the interlocked rings. Co-conformational switching in catenanes and rotaxanes has in fact been widely recognised as an efficient mechanism for developing molecular switches and machines.12,13 When two or more recognition sites are incorporated in a catenane or rotaxane, switching between several stable co-conformers can be achieved by applying a specific stimulus. Both the population and switching rate of the co-conformers will be critical to the performance of the molecular switches and machines. As molecular knots are composed of one entangled macrocycle, there will be no co-conformational isomers for knots.
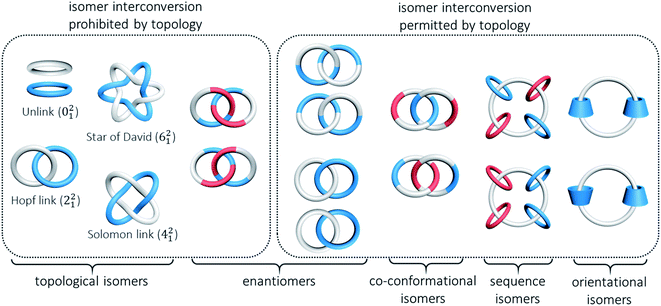 |
| Fig. 4 Possible types of catenane isomerism. | |
Another type of isomerism in catenanes is due to the topology of the interlocked structure. For example, a Hopf link (221), a Solomon link (421), a Star of David catenane (621), and an 8-crossing Torus link (821) are topological isomers of a [2]catenane with different number of crossings between the two interlocked macrocycles.14,15 Similarly, molecular knots that have the same covalent structure but different topologies (different number of crossings) are also topological isomers. Due to their different topologies, topological isomers are not interconvertible. On the other hand, a rotaxane is topologically trivial and hence rotaxanes that differ in the number of crossings between a pair of interlocked macrocycle and dumbbell are not topological isomers.
Orientational isomerism arises when macrocycles that lack a symmetry plane with respect to their cavity (e.g. cyclodextrins) are interlocked in a catenane or a rotaxane. On the other hand, if constitutionally different macrocycles are interlocked in different orders, sequence isomers are obtained. These two types of isomerism are not applicable in knots because at least three interlocked components are required to display orientational or sequence isomerism. Nevertheless, properties of isomeric catenanes that differ by the orientation and/or sequence of the mechanically bonded macrocycles have been rarely reported when compared with similar rotaxanes.16 One example of a catenane orientational isomer is the [3]catenanes with two interlocked cyclodextrins. The different symmetry imposed by the head-to-head and head-to-tail orientations of the two interlocked cyclodextrins has resulted in slightly different NMR spectral features and HPLC retention time.17 Our laboratory has recently reported two isomeric radial [5]catenanes in which the dynamics of the macrocycles are related to the sequence of the interlocked cucurbit[6]uril (CB[6]) and β-cyclodextrin (β-CD), although these isomers should be classified as constitutional isomers due to the different atom connectivity in the central macrocycle.18
2.4 Chirality
Many molecular links and knots are chiral, and the chirality that arises from the topology of the link and knot is known as topological chirality.19 For example, a Solomon link, Star of David catenane and trefoil knot are inherently chiral, and their chirality is due to their topology and independent of their chemical structures. Some catenanes are also topologically chiral if the interlocked macrocycles fulfill certain structural requirements. A Hopf link, for instance, composed of two directional interlocked macrocycles, is topologically chiral.20 Topological chirality is not applicable to rotaxanes because of their trivial topology.
Chirality can also be expressed by mechanical bonding in catenanes and rotaxanes when the interlocked components possess specific symmetry features.19a For example, there will be a chiral axis in a Hopf link if there is a prochiral centre in each of the two interlocked macrocycles.21 A Hopf link can also possess a chiral axis if it adopts a “twisted”, helical co-conformation,22 which has been described as mechanically helically chiral22b or mechanically axially chiral.19a
3. Catenane synthesis
The efficient generation of interlocked molecules with a well-defined entanglement is one major focus in the early development of the chemistry of catenanes and other MIMs. For the different structures and topologies of catenanes, rotaxanes and knots, considerations such as building block design and interlocking strategies would be quite different. For example, macrocycle formation is not necessary in rotaxane synthesis, and a rotaxane can be formed by either stoppering a pseudorotaxane complex or slipping a macrocycle through the bulky stopper of a dumbbell.23 In contrast, macrocycle formation from linear precursors will be necessary in catenane and knot synthesis because of their non-trivial topology.
Method development to control the topology and number of interlocked components could also be very different for catenanes, rotaxanes and knots. Currently, a majority of reported complex rotaxanes are those featuring different number of interlocked macrocyclic and linear components,10 and rotaxanes in which there are more than two crossings in a pair of an interlocked macrocycle and linear axle are very rare (if any). On the other hand, building blocks of highly elaborate structures such that a specifically oriented precursor can be generated before macrocycle formation are often required in the synthesis of complex knots and links.3a,24 If multiple covalent bonds are to be formed, the connectivity of the precursors will also be critical because links that contain different numbers of macrocyclic components and knots of different topologies could be obtained.25 For catenane synthesis, complexity in structures and the associated synthetic challenges can therefore come from the topology, the number of interlocked macrocycles or both.26
3.1 General considerations
Generally, entwining and interlocking weakly associated precursors into an MIM would reduce their conformational freedom which is entropically unfavourable. In fact, statistical synthesis of catenanes and rotaxanes is not efficient because of the low probability of the precursors to entangle at the time when a new covalent bond that interlocks the resulting components is formed.27,28 Templated synthesis, in which the low probability of interlocking is compensated by the favourable energetics of the templating interactions between the precursors, has therefore become a mainstream in MIM synthesis.1,2,3b,29 In a templated catenane synthesis, building blocks will first be preorganised via non-covalent interactions in a specific orientation that favours macrocycle interlocking, followed by a covalent ring-closing that simultaneously forms the mechanical bond (Fig. 5). Since mechanical interlocking is resulted only when the covalent bond (the macrocycle) is formed, efficiency of both the preorganisation and covalent ring-closing reaction will affect the yield of the target MIM. Efficiency of the preorganisation will be dependent on both the strength of the templating interactions and structural characteristics (e.g. backbone curvature, flexibility and length of linkers… etc.) of the building blocks, while efficient covalent bond formations such as click reactions,30 alkene metathesis31 and dynamic covalent bond forming reactions (e.g. imine32 and disulfide33) can give MIMs in high yields.
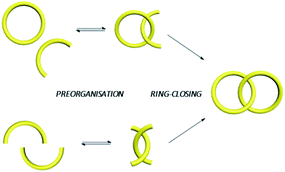 |
| Fig. 5 General templated synthesis of a catenane. | |
If the preorganisation and ring-closing steps are coupled such that macrocycles are formed only when the building blocks are preorganised, the efficiency of ring interlocking will be significantly enhanced. “Active template” is a strategy developed by Leigh, in which a metal ion serves as both a template for preorganisation and a catalyst for covalent bond formation.30,34 The use of cucurbit[6]uril (CB[6]), which can be assisted by cyclodextrins or pillararenes, to facilitate azide–alkyne cycloaddition inside the CB[6] cavity has also been used similarly for efficient rotaxane and catenane syntheses (Fig. 6).35
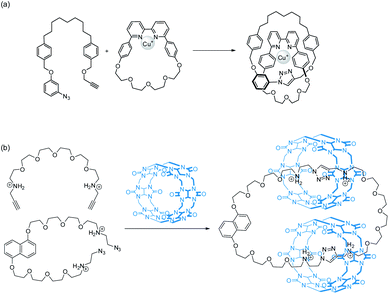 |
| Fig. 6 Example of catenane synthesis using (a) an active copper template34d and (b) CB[6]-mediated azide–alkyne cycloaddition.35g | |
MIM synthesis via a series of chemical transformations, in which a covalent scaffold with specifically oriented functional groups to favour entanglement formation is generated, is known as directed synthesis.36 There are relatively less examples of catenanes synthesised by directed synthesis although the first few examples have been described in the 1960's.36a Recently, Itami and co-workers have reported the strategic use of a tetrahedral organosilicon to direct the formation and interlocking of hydrocarbon macrocycles into [2]catenanes and trefoil knots, and the topology of these nano-sized carbon materials is expected to have interesting effects on their physical, dynamic and optoelectrical properties (Fig. 7).36g
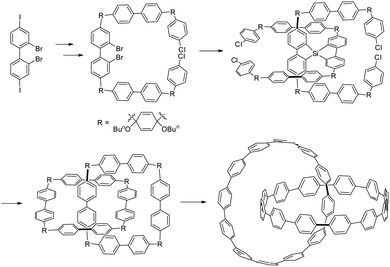 |
| Fig. 7 A hydrocarbon [2]catenane obtained through directed synthesis.36g | |
3.2 Creating specific entanglements
Generation of a specific number of crossings is key in the successful templated synthesis of molecular links and knots of a specific topology. In contrast, rotaxanes with a high level of entanglement are relatively less explored. Metal–ligand coordination featuring structurally sophisticated ligands is very successful in this regard for the diverse symmetry and coordination geometry of metal ions possessing different electronic structures.3b–d,37 For example, the use of the tetrahedral geometry of Cu(I) developed by Sauvage is one classical and highly efficient strategy to template [2]catenane (Hopf link) and trefoil knot formation (Fig. 8).38,39 Various metal ions of different coordination numbers and geometries have also been employed in the templated synthesis of other links and knots for creating the required molecular crossings.40 Purely organic molecular links beyond the Hopf link have also been reported, but generation of the required symmetry of the targeted topology using organic building blocks is not trivial, although thermodynamic synthesis seems to be particularly effective.41
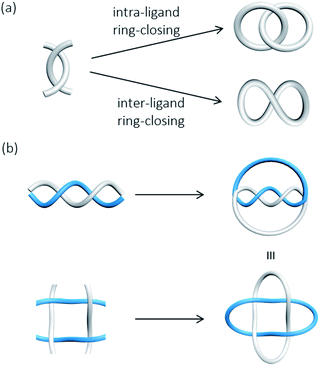 |
| Fig. 8 Templated synthesis of (a) a Hopf link using a tetrahedral template can lead to a molecular figure-8 and (b) Solomon link from either a helicate or 2 × 2 grid template. | |
To obtain links and knots with a high level of entanglement using metal templates, building blocks that contain multiple sets of coordination ligands, and/or well-defined structural characteristics are usually needed to create the required molecular crossings. For example, a Solomon link can be rationally synthesised from either a trinuclear double helicate or a 2 × 2 interdigitated grid wherein four crossings will be generated upon ring-closing of the templated precursors.42,43 The use of more complicated helicates and grid structures is currently one efficient and reliable strategy to expand the topological diversity of molecular links and knots.25a,b,44 Controlling the way of how the building blocks are connected upon ring-closing of the preorganised precursor is also important for obtaining a specific topology, although relevant strategies are relatively less discussed and developed. For example, two orthogonally preorganised ligands in a tetrahedral geometry can give either a Hopf link or a topologically trivial figure-8 depending on the specific way of macrocycle formation.45 In the synthesis of complex links and knots from a structurally elaborate preorganised precursor, the different possible ring-closing combinations and precursor connectivity will likely lead to topological isomers of similar properties, which could render the isolation of a particular link highly challenging. A careful characterisation of the link topology is also necessary.
On the other hand, self-assembly based on metal–ligand coordination can be equally successful in the formation of topologically complex links and knots.46 Unlike a rationally designed templated synthesis, the outcome of a self-assembly may be difficult to be predicted from the structure of the ligands because a slight structural modification in the ligand could have a large effect on the overall sum of the weak, non-covalent interactions.
3.3 Increasing the number of interlocked components
Except molecular knots which are one single entwined macrocycle, increasing the number of interlocked components is another key challenge in complex MIM synthesis. Many of the reported high-order [n]catenanes feature only two crossings between a pair of interlocked macrocycles, because achieving both a high number of crossings and interlocked rings in a single catenane will be extremely difficult and different strategies will have to be incorporated. On the other hand, high-order [n]rotaxanes that are composed of n − 1 macrocycles interlocked on one dumbbell are much more common,10 although counterparts featuring one macrocycle interlocked on n − 1 dumbbells, or other combinations of dumbbell and macrocycle numbers are relatively rare.11 In 2016, Leigh and co-workers have reported a triply threaded [4]rotaxane using active metal template synthesis.11c The relative size of the macrocycle and the stoppers, such that multiple axles can be accommodated in but do not dethread from the macrocycle, is critical.
Generally, a large number of mechanical and covalent bonds will have to be formed in an [n]catenane synthesis, and strategies to maintain high efficiency for both the preorganisation and ring-closing steps will be necessary.26 In particular, preorganisation involving multiple building blocks can be difficult because non-covalent interactions are usually weak, and the co-existence of many equilibrating species will result in interlocked products of different topologies and number of interlocked rings. If macrocycles are interlocked in multiple steps, the yield of the final [n]catenane will significantly drop with the overall length of the synthesis, even if both preorganisation and ring-closing are of high efficiency. On the other hand, [n]rotaxane synthesis can be achieved by strategies that involve no macrocycle formation (e.g. threading/slipping preformed macrocycles onto polymer or polymerising short pseudorotaxanes), which makes these [n]rotaxanes synthetically more accessible.10,47 For a similar reason, radial [n]catenane is the most common among all [n]catenane structures as it can be synthesised with the formation of only one new macrocycle (i.e. the central macrocycle) (Fig. 9).48
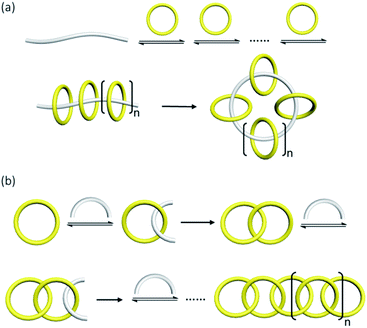 |
| Fig. 9 Synthesis of (a) a radial [n]catenane with one ring-closing reaction and (b) a linear [n]catenane with sequential interlocking of the macrocycles. | |
Linear and branched [n]catenanes are other catenane architectures that are much less common and their synthesis involves the formation of more than one new macrocycle. Linear [n]catenane is of particular interest in polymer and materials science because a polymer consisting of only mechanically interlocked rings will allow a maximum manifestation of the effects of the mechanical bond on the polymer mechanical and rheological properties.49 As one early hallmark in catenane chemistry, Stoddart's olympiadane is an example of a linear [5]catenane (Fig. 10a).50,51 A few other linear [4]- and [5]catenanes from either stepwise or one-pot synthesis have also been recently reported.52–55 In 2017, Rowan and co-workers have explored the use of a metallopolymer to obtain [n]catenanes.56 The metallopolymer consists of ditopic macrocycles and linear components coordinated via Zn2+ ions in an alternate fashion, from which a mixture of branched, linear and cyclic [n]catenanes were obtained upon ring-closing metathesis (Fig. 10b). Absolute molar mass measurement showed that there are 6 to 27 interlocked rings in the linear [n]catenane fraction.
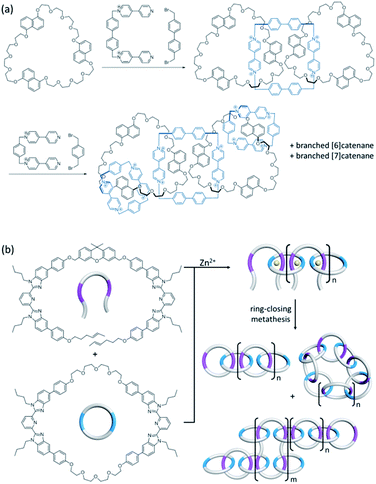 |
| Fig. 10 Synthesis of (a) linear [5]catenane (olympiadane) using an aromatic donor–acceptor template;50 and (b) [n]catenanes from a metallosupramolecular polymer.56 | |
Branched [n]catenanes have also been rarely reported, and the [6]- and [7]catenanes obtained from the same reaction in the synthesis of Stoddart's olympiadane are one early example of branched [n]catenanes.57 Our group has also recently reported the synthesis of a branched [6]- and [8]catenane featuring the use of orthogonal templates.58 Polymer- and DNA-based [n]catenanes with branched arrangements of interlocked rings have also been prepared.59 On the other hand, dendritic structures featuring catenane-based branches are yet to be reported, although related rotaxane dendrimers have been realised.60,61
Self-assembly can also give [n]catenanes although it may be difficult to rationally design a building block and predict the self-assembly process to target a specific [n]catenane structure.46 For example, Fujita and co-workers have shown that a 12-crossing [4]catenane and a 24-crossing [6]catenane can be assembled using Ag+ and peptide-based pyridine ligands.62,63 Assembly of only organic building blocks to nano-sized [n]catenane has recently been demonstrated by Yagai and co-workers, in which “supramolecular macrocycles” were assembled from the circular stacking of hydrogen-bonded, hexameric rosettes that interlock with each other to form catenanes.64 The number of interlocked nano-rings can be controlled by the assembly conditions (e.g. number of material injections).
4. Properties and applications of catenanes
Central to the unique properties of MIMs is the specific molecular entanglement present in the interlocked molecules. With the combined structural and topological diversity and various levels of molecular entanglement, catenanes are a versatile type of MIMs with a broad range of potential applications. With their related bonding and topology, applications of catenanes actually share a lot of similarities with those of rotaxanes and knots. Yet, with the differences in their synthesis, structures and dynamic properties, these different MIMs will have their own advantages when a specific application is concerned. On one hand, catenanes with few crossings and a low level of entanglement are co-conformationally more flexible and are suitable for applications that require dynamic properties such as developing molecular switches and machines. On the other hand, a highly entangled catenane will have a highly specific topological structure, and the associated stereochemical properties and structural rigidity could be suitable for binding and recognition. In fact, properties and applications of rotaxanes and knots that have been demonstrated to date are quite different due to their different topologies and dynamics. Rotaxanes are also common structures used in constructing molecular switches and machines due to their dynamic properties and stimuli-responsiveness,13 whereas the topological chirality and specific structural characteristics from the mechanically constrained knots are promising for guest binding and related applications.15c,41b,65
Recently, the concept of “supramolecular elements” has been introduced by Schmidt and Würthner, in which macrocycles in a catenane are considered analogous to atoms in a molecule.66 Features and characteristics of the mechanical bond between the interlocked macrocycles are therefore of fundamental importance to the properties and applications of a catenane, as if molecular properties of a molecule are governed by the covalent bonds between the constituting atoms. In fact, covalent bonds between different elements are of diverse nature, geometry and chemical reactivity. Features of the mechanical bond and entanglement between different interlocked components, in different types of MIMs, would also be very different. In this section, distinctive properties of catenanes will first be highlighted, followed by a discussion on catenane applications that are related to these properties with representative and recent examples.
4.1 Strength of the mechanical bond in catenanes
Bond strength is one important property that characterises a covalent bond, and a weaker bond is generally more reactive than a stronger bond. Information related to the strength of a mechanical bond may therefore be important to the properties of catenanes as well. For the non-trivial topology, a catenane structure cannot be maintained only when a covalent bond in the interlocked macrocycle is broken, and hence the strength of a mechanical bond in a catenane is generally considered to be similar to that of the weakest covalent bond in the macrocycles.16a,67 For example, a Hopf link that contains bulky groups can be converted to a (pseudo)rotaxane which also contains a mechanical bond of a different nature and strength when the macrocycle is cleaved. The mechanical bond for maintaining the catenane entanglement in this case is considered to be broken and converted to another mechanical bond that maintains the (pseudo)rotaxane structure. The size of the bulky groups and strength of inter-component interactions may affect the strength of the mechanical bond of the (pseudo)rotaxane but not that of the parent Hopf link (Fig. 11).
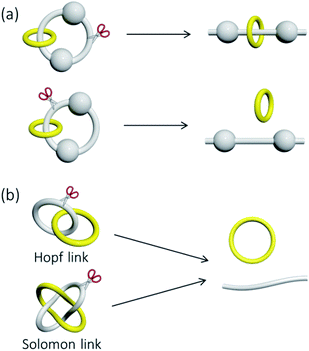 |
| Fig. 11 Breaking a covalent bond in (a) a Hopf link could result in either a [2]rotaxane that contains a mechanical bond or separated derivatives from the dumbbell and macrocycle; (b) a Hopf link and a Solomon link could both result in the breaking of the mechanical bond. | |
While the strength of a covalent bond generally increases with the bond order, the level of entanglement between two interlocked rings in a catenane may not necessarily correlate with the strength of the mechanical bond (i.e. strength of the covalent bond/interactions that maintain the entanglement). The entanglement may or may not influence the strength of the weakest covalent bond that is essential for maintaining the mechanical bond, which is dependent on the overall structure and chemical composition of the specific MIMs. For example, although the two interlocked macrocycles in a 4-crossing Solomon link is “more entangled” than that in a 2-crossing Hopf link, the mechanical bond between the macrocycles in both MIMs will be broken once a ring is cleaved. While a mechanical bond and the concept of mechanical bond strength are not applicable in knots, the mechanical bond in a rotaxane can be “broken” by a number of ways in addition to covalent bond cleavage because of the trivial topology (e.g. slippage of macrocycle over the dumbbell), and hence there are more factors that determine the mechanical bond strength in rotaxanes.
4.2 Propensity to undergo co-conformational change
The ability of the interlocked macrocycles to undergo large-amplitude motions to result in co-conformational changes is another important feature that characterises the mechanical bond in a catenane. In fact, the development of rotaxane- and catenane-based molecular switches and machines is largely premised on stimuli-triggered co-conformational changes of the MIMs, and the dynamics of the interlocked components is therefore of critical importance in this regard.68 Although molecular knots cannot undergo co-conformational change, and the dynamics of knots are relatively unexplored, motions such as reptation (i.e. a “creep-like” movement similar to that of a snake where segments of the knot move within the entangled knot) have been suggested and identified.44b,69
There are a few potential factors that would affect co-conformation exchange in a catenane and rotaxane. The first one is the “tightness” of the mechanical bond that is related to the topology, physical dimension and rigidity of the interlocked components which are largely kinetic in origin.70 Generally, with a higher level of entanglement (i.e. more crossings) between two interlocked macrocycles, undergoing co-conformational change would be expected to be more difficult. For example, a Solomon link would be tighter than a Hopf link that is composed of the same macrocycles, and it is therefore easier to develop switchable catenanes based on a Hopf link than a Solomon link (Fig. 12).
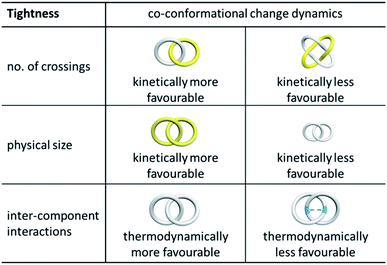 |
| Fig. 12 Effect of tightness (top) and stickiness (bottom) of interlocked rings on the kinetics and thermodynamics of catenane co-conformational change. | |
In fact, tightness of an MIM influences not only co-conformational changes but also conformations, and the physical and chemical properties of the MIM as well. For example, in a study reported by Leigh and co-workers, three 819 knots of different sizes and tightnesses were found to display properties, including dynamics, CD and NMR features, and chemical reactivity, which are correlated with the tightness of the knots.71 Of note, it would often be difficult to compare the overall tightness between different MIMs as it is contributed by a number of factors including the number of crossings, physical dimension, and chemical composition of the MIMs. Mass spectrometric techniques may provide a comparative assessment of tightness between different MIMs, but the tightness of individual components in an MIM may need to be characterised by their dynamic behaviour.72
Another factor that affects co-conformational exchange dynamics in a catenane is the strength of the interactions between segments of two interlocked macrocycles. As a majority of catenanes (and rotaxanes) are synthesised using a templated method, the interactions that template macrocycle interlocking often remain in the resulting MIMs in addition to those that exist between other parts of the interlocked macrocycles. These interactions may stabilise a particular co-conformation of the catenane, and contribute to the thermodynamics and kinetics of the co-conformational exchange. If the interactions that stabilise a particular co-conformation of a catenane are very strong (relative to the average van der Waals interactions between the macrocycles), co-conformational exchange may not be favourable as the macrocycles are “stuck” firmly together. For example, in transition metal-templated catenanes, due to the presence of strong coordination bonds, the catenanes usually exist as one stable co-conformation in the presence of the templating metal, and thermal motions may not be sufficient to result in large-amplitude co-conformational change. On the other hand, facile co-conformation exchange can be observed if the interlocked macrocycles are not bound by strong interactions. For example, β-CDs interlocked by hydrophobic interactions are usually more mobile as the hydrophobic interactions involving β-CDs are only of moderate strength (log
K ∼ 3).17
A catenane can exchange between different stable co-conformations when additional binding sites are present in the interlocked macrocycles. In fact, the incorporation of binding sites other than that for the templating purpose is a common strategy to obtain switchable catenanes and rotaxanes for developing molecular switches and molecular machines. Co-conformational switching in these bistable or multistable MIMs can be triggered by a chemical, photo, thermal or environmental stimulus that modulates the stability of the co-conformations.68
4.3 Examples of catenane application in relation to the properties of the mechanical bond
4.3.1 Stable co-conformations for switchable systems.
The development of molecular machines that exploits co-conformational switching under controlled conditions is perhaps one most appreciated application of catenanes and rotaxanes. Various kinds of stimuli-responsive systems, such as molecular shuttles, rotors, pumps, sensors, switchable catalysts… etc., have been developed based on the ability of the interlocked components to undergo large-amplitude intramolecular motions and switch between different stable co-conformations.68 If a specific change in a MIM property (e.g. photophysical or catalytic property) is targeted for functions or applications, dependence of that particular property on the MIM co-conformations will have to be considered in the molecular design. For example, in a recent report by Qu and co-workers, a reversible switching of the fluorescence properties of a [2]catenane is demonstrated. Fluorescence properties of the 9,14-diphenyl-9,14-dihydrodibenzo[a,c]phenazine (DPAC) fluorophore are sensitive to its conformation, which in turn is dependent on the co-conformation of the [2]catenane, and therefore switching between stable co-conformations results in a change in the observed fluorescence (Fig. 13).73 The different reactivities of catenane co-conformations have also been exploited in the development of switchable catalysts. For example, Willner and co-workers have developed DNA catenanes that can be switched between the catalytically active and inactive states.74 Examples of switching of chirality, optical rotations and other properties in catenanes due to co-conformational change have also been reported.75
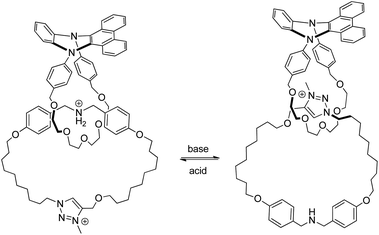 |
| Fig. 13 Co-conformational switching of an acid–base responsive DPAC-containing [2]catenane with different fluorescence properties.73 | |
In addition to the stimuli that bring about the co-conformational change and their associated kinetics and thermodynamics, another noteworthy point in exploiting the co-conformational change of MIMs in developing molecular switches and machines is the direction of the movement of the interlocked components. For example, a molecular shuttle can be developed from the translational motions of the interlocked macrocycle along a rotaxane axle, whereas a molecular rotor may require rotatory motions that are available in a catenane; the one-dimensional length extension/contraction from a switchable polymeric linear daisy chain could be employed as molecular muscles, whereas similar co-conformational switching of a cyclic daisy chain will lead to a two-dimensional area change of the internal opening (Fig. 14).76,77
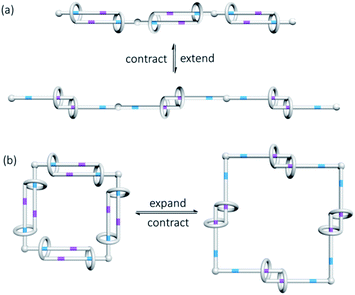 |
| Fig. 14 Co-conformational switching in (a) linear and (b) cyclic daisy chains results in length and aperture control. | |
With the relatively higher level of entanglement and more rigid structure, switchable systems derived from knots are rarely explored although a flexible knot may be able to switch between different conformations.78 Currently, rotaxane-based switchable systems are more developed than those based on catenanes, yet the availability of a diverse selection and variety of MIMs will be necessary to further advance MIM-based molecular machineries and applications. Similar to biomolecular machines or machines in the macroscopic world, the presence of multiple components of different chemical, physical and dynamic properties, and strategies to control the thermodynamics and kinetics of co-conformational changes in a coordinated and orchestrated fashion will be essential for developing complex MIM-based molecular machines. One recent example of such a complex catenane-based molecular machine is the rotatory motor powered by a chemical fuel reported by Leigh and co-workers (Fig. 15).79a Both the strength of macrocycle binding at different stations and the size of the bulky speedbumps can be switched by pulses of trichloroacetic acids which initially acidify but then slowly decarboxylate and neutralise the system. A unidirectional rotatory movement of the interlocked crown ether is achieved as only one of the base-labile disulfide or acid-labile hydrazone speedbumps can be exchanged to allow the macrocycle to pass through at a time. Other molecular machines based on catenanes with sophisticated mechanisms for motion control have also been reported.79
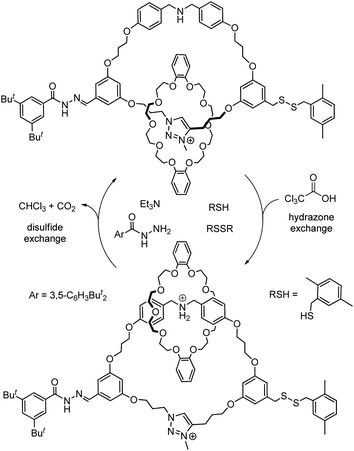 |
| Fig. 15 Co-conformational switching in a chemically fuelled molecular motor results in a unidirectional motion.79a | |
4.3.2 Exploiting co-conformational dynamics in materials.
Dynamics due to co-conformational changes in catenanes, rotaxanes and other MIMs are also implicated in material properties when they are incorporated into materials. For example, by crosslinking the interlocked macrocycles in polymers, Ito has obtained slide-ring gels (polyrotaxane) in which forces applied on the polymer can be equalised through sliding of the interlocked macrocyclic crosslinks along the linear polymer chains, and the elasticity and softness of the slide-ring gel are very different from those of covalently crosslinked polymers (Fig. 16a).80 A study by De Bo has shown that ring circumrotation in a catenane containing polymer can divert tension away from the catenane and stabilise a mechanophore incorporated in the ring over that in the polymer chain (Fig. 16b).67 Huang and co-workers have also demonstrated that polymers crosslinked by [2]catenanes with hydrogen bonding capability can switch between rigid and soft states when the dynamics of the catenane crosslinkers is modulated by pH (Fig. 16c).81
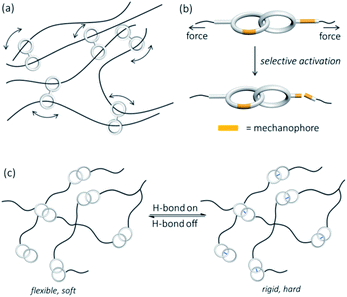 |
| Fig. 16 Examples of polymer materials that incorporate MIMs with new properties: (a) slide-ring gel of unique elasticity and softness;80 (b) a catenane polymer that shows selective mechanophore activation;67 and (c) a catenane-crosslinked polymer with switchable rigidity and hardness.81 | |
4.3.3 Binding sites created by molecular entanglements.
The strong and flexible mechanical bond in catenanes also provides an efficient mechanical confinement and stabilisation effect that are implicated in host–guest binding, catalysis and other applications. In fact, templated catenane synthesis using an external template will result in catenane hosts that are predisposed with a highly complementary cavity for binding to the template. Co-conformational change could also provide an additional, low-energy mechanism for structural adaption beyond that restricted by the covalent backbone. A wealth of catenane-based hosts and sensors for cations, anions and other guests have therefore been developed using the targeted guest as a template in the synthesis.82 In addition to the binding site crafted by the template, the well-defined entanglements in molecular links and knots can also result in a symmetrical, highly preorganised cavity/binding site with unique stereochemistry from the topology for guest binding. For example, the Star of David catenane and pentafoil knot reported by Leigh and co-workers can bind to anions in their internal cavity, and this anion-binding ability of the MIMs has been employed as cross-membrane ion channels and anion-binding catalysts (Fig. 17a).15c,65 Cougnon and co-workers have also reported a Solomon link with an internal cavity that can bind to four water molecules (Fig. 17b).83 On the other hand, while rotaxane-based hosts with a binding pocket from mechanical bonding have also been developed, the level of mechanical confinement in rotaxanes may not be as strong as that in catenanes and knots owing to their relatively more open binding pocket. Topological chirality from molecular knots and links (catenanes) can also be exploited for stereoselective guest binding, but additional structural elements will be needed for creating a chiral rotaxane host.19
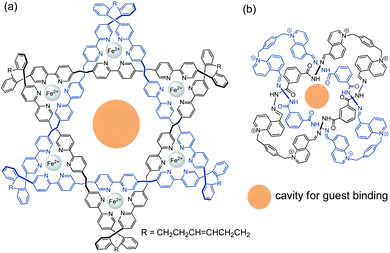 |
| Fig. 17 Examples of guest-binding catenanes with a well-defined cavity: (a) an anion-binding Star-of-David catenane;15c and (b) a Solomon link that binds to four water molecules.83 | |
4.3.4 Stabilisation and confinement of reactive species.
Highly reactive species and weakly associating complexes can also be stabilised by binding to or being confined within a catenane host. For example, air-stable radical species can be generated when being incorporated within the interlocked framework of catenanes (Fig. 18a).84 Charge separated radical pairs generated upon photoexcitation have been shown to have longer lifetime when the donor and acceptor are mechanically bonded as rotaxanes or catenanes (Fig. 18b).85 Incorporation of reactive carbene species with an MIM has also been recently reported (Fig. 18c).86 Metal ions are kinetically stabilised by coordinating to catenane (and rotaxane) ligands.87 Complete ligand dissociation is prohibited by the mechanical bond and adaption in coordination geometry is kinetically restricted. Metal complexes of unusual coordination geometry and hence electronic structure, redox properties, and chemical reactivity could therefore be produced. Recently, it has been demonstrated that weakly associating polyiodides can be stabilised as networks of rotaxanes and catenanes.88
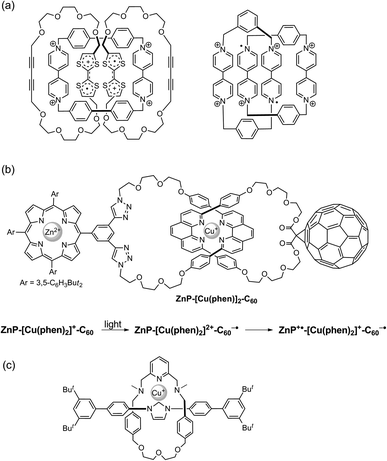 |
| Fig. 18 Examples of reactive species confined and stabilised within an MIM: (a) a cation radical pair;84 (b) a charge-separated radical pair generated upon photoexcitation;85 and (c) a metal carbene species.86 | |
By stabilising these otherwise unstable radical and metal species, new MIM-based catalysts can be developed.89 Recently, the classical aromatic donor–acceptor [2]catenane developed by Stoddart et al. has been studied for photoredox catalysis. Being confined within the [2]catenane framework, the charge transfer complex remains intact even at low concentrations with a strong light absorption, and the cation radicals generated can be employed in catalysing both photo-oxidation and photo-reduction reactions (Fig. 19a).90 Our group has reported the use of a catenane-coordinated copper(I) for catalytic C–O cross-coupling reactions.91 The copper is kinetically stabilised by the catenane ligands with a coordinatively saturated resting state, but the coordination sphere can also be accessed by the substrates due to the dynamic co-conformation changes of the catenane ligands. As a result, the catalyst enjoys both a long lifetime and a good reactivity (Fig. 19b). A study by Niemeyer et al. has shown that confining two chiral phosphoric acid groups within a [2]catenane can lead to a high local concentration of the acidic catalytic sites for a high catalytic efficiency and an appropriate substrate orientation for a good stereoselectivity (Fig. 19c).92 Together with the switchable catalysts that are based on co-conformational switching,74,93 and examples of asymmetric catalysis by virtue of the topological chirality,94 these catenane catalysts that exploit mechanical bond stabilisation highlight the versatility of catenanes that can be exploited to address different challenges in catalysis.
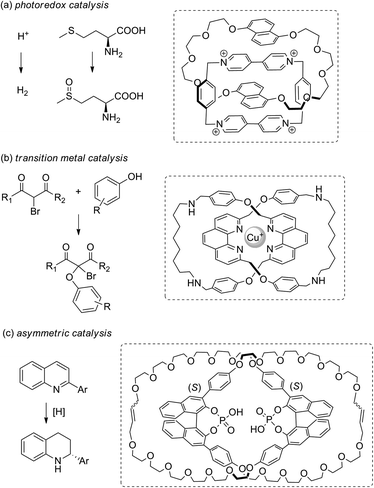 |
| Fig. 19 Recent examples of catenane catalysts for (a) photoredox,90 (b) transition metal91 and (c) asymmetric catalysis.92 | |
The efficient stabilisation provided by MIMs is also attractive for applications in complex biological matrices and the use of MIMs as carriers in biological systems for drug delivery and triggered release has been recently reported.95–97
5. Future outlook
Catenane chemistry has rapidly evolved since the introduction of templated synthesis. Yet, the synthesis of simple catenanes (e.g. Hopf link) and control of catenane co-conformations are only the first step of our exploration in the new chemical space of mechanical bond and molecular topology. From fundamental synthesis, structure and characterisation, to innovations and applications of catenanes for new properties and functions, there are many directions to further develop the chemistry of catenanes.98 Here, we present some of our own views on the challenges and opportunities in catenane chemistry.
While templated synthesis overcomes the unfavourable entropy in macrocycle interlocking, there are other aspects in the interlocking process that need to be controlled if catenanes of diverse structural variety are to be obtained. One longstanding challenge is the synthesis of [n]catenanes with a large number of interlocked rings. Although [n]catenane synthesis/assembly has been reported, the reported methods are still far from being controllable and predictable. Strategies to control the number, topology (i.e. number and way of crossings) and connectivity (e.g. linear and branched catenanes) of the interlocked rings that allow a rational design to obtain high-order [n]catenanes in a predictable and reliable fashion will be necessary.26 Chirality is another attribute that needs further attention particularly when catenanes are to be employed in guest recognition and catalysis. Although Hopf links can be efficiently obtained in a relatively large quantity and variety, and are therefore more suitable than other high-order [n]catenanes and molecular links for exploiting properties and applications, topologically chiral Hopf links are much more difficult to prepare due to the achiral topology. New strategies to direct the orientation of directional macrocycles, such as the use of chiral auxiliaries for enantioselective mechanical interlocking developed by Goldup, will be necessary.99
Physical methods for efficient characterisation of catenanes are also very important, especially as more catenanes of high structural and topological complexity are now becoming synthetically accessible. Currently, X-ray crystallography is one highly reliable technique for topology assignment, but obtaining suitable single crystals can be very difficult; in particular, complex molecular links and knots are usually available in only tiny amounts. The mechanically bonded macrocycles in catenanes can also be characterised by analysing the fragmentation patterns in tandem MS, and a recent study by Schalley et al. has shown that ion mobility can be an additional parameter that reflects the tightness of the MIMs.72 Symmetry and structural analyses by two-dimensional NMR techniques are also common in the structural characterisation of catenanes and other interlocked structures. Methods for characterising the dynamics and motions of the interlocked components such as variable temperature NMR, vibrational spectroscopies and microscopies will also be necessary.100 Computational studies and simulations on the effects and properties of mechanical bonds in catenanes, on the other hand, are an area that needs more attention. Due to the relatively large molecular size of catenanes and MIMs, calculations on interlocked systems are usually of a high computational cost.101
Regarding properties and applications, synthesis and studies on the switching behaviour in switchable catenanes with two or more stable co-conformations remain one of the more studied directions. In addition to the various mechanisms and stimuli that have been developed to trigger co-conformational change, strategies in controlling the switching dynamics and coordinating the motions of different interlocked components will be important. Switchable systems will also need to expand from simple [2]- and [3]catenanes to high order [n]catenanes for more complex motions.
Studies of catenanes and mechanical bond properties in different materials and applications are also one important direction for further development of catenane chemistry. The non-trivial topology of catenanes may offer an interesting structural scaffold for unusual electronic structures and conjugated systems which are implicated in electronic, luminescent and magnetic materials.102 Strategies for catenane functionalisation and incorporation in extended networks and interfaces such as surfaces, monolayers, thin films, polymers, nanoparticles and metal–organic frameworks (MOFs) will also be necessary for expressing the unique features of catenanes in different materials and interfaces.103 On the other hand, the good stability of catenanes provided by the mechanical bond not only will allow studies and explorations of short-lived, reactive species that are implicated in catalysis, energy/electron transfer and material research, but also render catenanes particularly suitable for applications in competitive environments such as biological systems, interfaces, devices and other complex matrices.
Overall, with the unique properties arising from the non-trivial topology, diverse structural variety and rich dynamics, the realm of catenane chemistry overlaps much with that of knots and rotaxanes, and as such the potential and opportunities of knots and rotaxanes are also available to catenanes. Of course, what can be achieved by catenanes, rotaxanes and knots, and properties that are unique to mechanical interlocking are not exclusive to any one of these kinds of MIMs, and are largely dependent on the specific topology, structural characteristics, and availability of synthetic methods to obtain a particular MIM structure. A rigid rotaxane could be designed with a highly elaborate cavity for efficient guest binding, and a loose and flexible knot could also display dynamic conformational changes similar to catenanes and rotaxanes. Nevertheless, with a thorough and fundamental understanding of the nature of molecular entanglement in catenanes, it is hoped that the boundary between different classes of MIMs is becoming more clear, and that the different and unique properties of catenanes and other MIMs can be strategically integrated with the challenges and opportunities in various areas, where fruitful and diverse discoveries and innovations are to be expected.
Author contributions
H. Y. Au-Yeung and Y. Deng wrote the paper.
Conflicts of interest
There are no conflicts to declare.
Acknowledgements
We are grateful for the support from the Croucher Foundation, CAS-Croucher Funding Scheme for Joint Laboratories and a grant from the Research Grants Council of the Hong Kong Special Administrative Region, China. We also thank Dr Lihui Zhu for helpful discussion on the manuscript.
Notes and references
-
(a) G. Gil-Ramírez, D. A. Leigh and A. J. Stephens, Angew. Chem., Int. Ed., 2015, 54, 6110 CrossRef;
(b) N. H. Evans and P. D. Beer, Chem. Soc. Rev., 2014, 43, 4658 RSC.
-
(a)
C. J. Bruns and J. F. Stoddart, The Nature of Mechanical Bond: from Molecules to Machines, John Wiley & Sons, New Jersey, 2017 Search PubMed;
(b) Q.-H. Guo, Y. Jiao, Y. Feng and J. F. Stoddart, CCS Chem., 2021, 3, 1542 CrossRef CAS;
(c) D. Sluysmans and J. F. Stoddart, Trends Chem., 2019, 1, 185 CrossRef CAS;
(d) C. O. Dietrich-Buchecker and J. P. Sauvage, Chem. Rev., 1987, 87, 795 CrossRef CAS.
-
(a) S. D. P. Fielden, D. A. Leigh and S. L. Woltering, Angew. Chem., Int. Ed., 2017, 56, 11166 CrossRef CAS;
(b) J. E. Beves, B. A. Blight, C. J. Campbell, D. A. Leigh and R. T. McBurney, Angew. Chem., Int. Ed., 2011, 50, 9260 CrossRef CAS;
(c) S.-L. Huang, T. S. A. Hor and G.-X. Jin, Coord. Chem. Rev., 2017, 333, 1 CrossRef CAS;
(d) W.-X. Gao, H.-J. Feng, B.-B. Guo, Y. Lu and G.-X. Jin, Chem. Rev., 2020, 120, 6288 CrossRef CAS PubMed.
- P. J. Altmann and A. Pöthig, Angew. Chem., Int. Ed., 2017, 56, 15733 CrossRef CAS PubMed.
-
(a) D. M. P. Mingos, J. Yau, S. Menzer and D. J. Williams, Angew. Chem., Int. Ed. Engl., 1995, 34, 1894 CrossRef CAS;
(b) C. P. McArdle, M. C. Jennings, J. J. Vittal and R. J. Puddephatt, Chem.–Eur. J., 2001, 7, 3572 CrossRef CAS;
(c) S. S.-Y. Chui, R. Chen and C. M. Che, Angew. Chem., Int. Ed., 2006, 45, 1621 CrossRef CAS;
(d) G.-T. Xu, L.-L. Wu, X.-Y. Chang, T. W. H. Ang, W.-Y. Wong, J.-S. Huang and C.-M. Che, Angew. Chem., Int. Ed., 2019, 58, 16297 CrossRef CAS.
-
(a) H. Kawai, T. Umehara, K. Fujiwara, T. Tsuji and T. Suzuki, Angew. Chem., Int. Ed., 2006, 45, 4281 CrossRef CAS PubMed;
(b) T. Umehara, H. Kawai, K. Fujiwara and T. Suzuki, J. Am. Chem. Soc., 2008, 130, 13981 CrossRef CAS PubMed;
(c) H. Sugino, H. Kawai, T. Umehara, K. Fujiwara and T. Suzuki, Chem.–Eur. J., 2012, 18, 13722 CrossRef CAS PubMed.
- D. M. Walba, Tetrahedron, 1985, 41, 3161 CrossRef CAS.
-
(a) O. Lukin and F. Vögtle, Angew. Chem., Int. Ed., 2005, 44, 1456 CrossRef CAS PubMed;
(b) R. S. Forgan, J.-P. Sauvage and J. F. Stoddart, Chem. Rev., 2011, 111, 5434 CrossRef CAS PubMed.
- J. W. Alexander and G. B. Briggs, Ann. Math., 1926, 28, 562 CrossRef.
- For reviews and examples of high-order [n]rotaxanes, see:
(a) H.-Y. Zhou, Q.-S. Zong, Y. Han and C.-F. Chen, Chem. Commun., 2020, 56, 9916 RSC;
(b) X.-Q. Wang, W.-J. Li, W. Wang and H.-B. Yang, Chem. Commun., 2018, 54, 13303 RSC;
(c) S.-J. Rao, Q. Zhang, J. Mei, X.-H. Ye, C. Gao, Q.-C. Wang, D.-H. Qu and H. Tian, Chem. Sci., 2017, 8, 6777 RSC;
(d) J. E. M. Lewis, J. Winn, L. Cera and S. M. Goldup, J. Am. Chem. Soc., 2016, 138, 16329 CrossRef CAS PubMed;
(e) T. A. Barendt, I. Rašović, M. A. Lebedeva, G. A. Farrow, A. Auty, D. Chekulaev, I. V. Sazanovich, J. A. Weinstein, K. Porfyrakis and P. D. Beer, J. Am. Chem. Soc., 2018, 140, 1924 CrossRef CAS;
(f) Z. Meng, J.-F. Xiang and C.-F. Chen, Chem. Sci., 2014, 5, 1520 RSC;
(g) L.-S. Zheng, J.-S. Cui and W. Jiang, Angew. Chem., Int. Ed., 2019, 58, 15136 CrossRef CAS;
(h) Y. Wang, M. Frasconi, W.-G. Liu, J. Sun, Y. Wu, M. S. Nassar, Y. Y. Botros, W. A. Goddard, III, M. R. Wasielewski and J. F. Stoddart, ACS Cent. Sci., 2016, 2, 89 CrossRef CAS;
(i) H. V. Schröder, F. Stein, J. M. Wollschläger, S. Sobottka, M. Gaedke, B. Sarkar and C. A. Schalley, Angew. Chem., Int. Ed., 2019, 58, 3496 CrossRef PubMed;
(j) J. C. Chambron, V. Heitz and J. P. Sauvage, J. Am. Chem. Soc., 1993, 115, 12378 CrossRef CAS;
(k) S. Lee, C.-H. Chen and A. H. Flood, Nat. Chem., 2013, 5, 704 CrossRef CAS PubMed;
(l) Y.-D. Yang, C.-C. Fan, B. M. Rambo, H.-Y. Gong, L.-J. Xu, J.-F. Xiang and J. L. Sessler, J. Am. Chem. Soc., 2015, 137, 12966 CrossRef CAS PubMed.
- For examples see:
(a) H. M. Cheng, D. A. Leigh, F. Maffei, P. R. McGonigal, A. M. Z. Slawin and J. Wu, J. Am. Chem. Soc., 2011, 133, 12298 CrossRef CAS;
(b) M. Inouye, K. Hayashi, Y. Yonenaga, T. Itou, K. Fujimoto, T. Uchida, M. Iwamura and K. Nozaki, Angew. Chem., Int. Ed., 2014, 53, 14392 CrossRef CAS;
(c) J. J. Danon, D. A. Leigh, P. R. McGonigal, J. W. Ward and J. Wu, J. Am. Chem. Soc., 2016, 138, 12643 CrossRef CAS PubMed;
(d) L. D. Movsisyan, M. Franz, F. Hampel, A. L. Thompson, R. R. Tykwinski and H. L. Anderson, J. Am. Chem. Soc., 2016, 138, 1366 CrossRef CAS PubMed;
(e) K. Yamamoto, R. Nameki, H. Sogawa and T. Takata, Angew. Chem., Int. Ed., 2020, 59, 18023 CrossRef CAS PubMed;
(f) Y. Yamashita, Y. Mutoh, R. Yamasaki, T. Kasama and S. Saito, Chem.–Eur. J., 2015, 21, 2139 CrossRef CAS PubMed;
(g) A. I. Prikhod'ko, F. Durola and J.-P. Sauvage, J. Am. Chem. Soc., 2008, 130, 448 CrossRef PubMed;
(h) E. J. F. Klotz, T. D. W. Claridge and H. L. Anderson, J. Am. Chem. Soc., 2006, 128, 15374 CrossRef CAS PubMed;
(i) Z.-J. Zhang, H.-Y. Zhang, H. Wang and Y. Liu, Angew. Chem., Int. Ed., 2011, 50, 10834 CrossRef CAS PubMed;
(j) C.-F. Lee, D. A. Leigh, R. G. Pritchard, D. Schultz, S. J. Teat, G. A. Timco and R. E. P. Winpenny, Nature, 2009, 458, 314 CrossRef CAS PubMed.
- For examples of catenane-based molecular switches, see:
(a) D. B. Amabilino, C. O. Dietrich-Buchecker, A. Livoreil, L. Pérez-García, J.-P. Sauvage and J. F. Stoddart, J. Am. Chem. Soc., 1996, 118, 3905 CrossRef CAS PubMed;
(b) D. J. Cárdenas, A. Livoreil and J.-P. Sauvage, J. Am. Chem. Soc., 1996, 118, 11980 CrossRef;
(c) S. Grunder, P. L. McGrier, A. C. Whalley, M. M. Boyle, C. Stern and J. F. Stoddart, J. Am. Chem. Soc., 2013, 135, 17691 CrossRef CAS PubMed;
(d) I. R. Fernando, M. Frasconi, Y. Wu, W.-G. Liu, M. R. Wasielewski, W. A. Goddard III and J. F. Stoddart, J. Am. Chem. Soc., 2016, 138, 10214 CrossRef CAS PubMed;
(e) D. A. Leigh, K. Moody, J. P. Smart, K. J. Watson and A. M. Z. Slawin, Angew. Chem., Int. Ed., 1996, 35, 306 CrossRef CAS;
(f) D. A. Leigh, P. J. Lusby, A. M. Z. Slawin and D. B. Walker, Chem. Commun., 2005, 4919 RSC;
(g) T. A. Barendt, L. Ferreira, I. Marques, V. Félix and P. D. Beer, J. Am. Chem. Soc., 2017, 139, 90260 CrossRef PubMed;
(h) C.-Y. Tsai, C.-C. Lai, Y.-H. Liu, S.-M. Peng, R. P. Cheng and S.-H. Chiu, J. Org. Chem., 2018, 83, 5619 CrossRef CAS PubMed;
(i) Y. Zhang, Q. Chen, Y. Wang, X. Zheng, H. Wang, F. Cao, A. C.-H. Sue and H. Li, Chem. Commun., 2020, 56, 11887 RSC.
- For examples of rotaxane-based molecular machines, see:
(a) J. D. Badjic, C. M. Ronconi, J. F. Stoddart, V. Balzani, S. Silvi and A. Credi, J. Am. Chem. Soc., 2006, 128, 1489 CrossRef CAS PubMed;
(b) Y. Yamada, M. Okamoto, K. Furukawa, T. Kato and K. Tanaka, Angew. Chem., Int. Ed., 2012, 51, 709 CrossRef CAS;
(c) B. Lewandowski, G. De Bo, J. W. Ward, M. Papmeyer, S. Kuschel, M. J. Aldegunde, P. M. E. Gramlich, D. Heckmann, S. M. Goldup, D. M. D'Souza, A. E. Fernandes and D. A. Leigh, Science, 2013, 339, 189 CrossRef CAS;
(d) S. Amano, S. D. P. Fielden and D. A. Leigh, Nature, 2021, 594, 529 CrossRef CAS PubMed;
(e) Q.-H. Guo, Y. Qiu, X. Kuang, J. Liang, Y. Feng, L. Zhang, Y. Jiao, D. Shen, R. D. Astumian and J. F. Stoddart, J. Am. Chem. Soc., 2020, 142, 14443 CrossRef CAS PubMed;
(f) Y. Qiu, B. Song, C. Pezzato, D. Shen, W. Liu, L. Zhang, Y. Feng, Q.-H. Guo, K. Cai, W. Li, H. Chen, M. T. Nguyen, Y. Shi, C. Cheng, R. D. Astumian, X. Li and J. F. Stoddart, Science, 2020, 368, 1247 CrossRef CAS PubMed;
(g) M. Canton, J. Groppi, L. Casimiro, S. Corra, M. Baroncini, S. Silvi and A. Credi, J. Am. Chem. Soc., 2021, 143, 10890 CrossRef CAS PubMed.
- For examples of Solomon link:
(a) J. E. Beves, C. J. Campbell, D. A. Leigh and R. G. Pritchard, Angew. Chem., Int. Ed., 2013, 52, 6464 CrossRef CAS PubMed;
(b) C. D. Pentecost, K. S. Chichak, A. J. Peters, G. W. V. Cave, S. J. Cantrill and J. F. Stoddart, Angew. Chem., Int. Ed., 2007, 46, 218 CrossRef CAS PubMed;
(c) C. Schouwey, J. J. Holstein, R. Scopelliti, K. O. Zhurov, K. O. Nagornov, Y. O. Tsybin, O. S. Smart, G. Bricogne and K. Severin, Angew. Chem., Int. Ed., 2014, 53, 11261 CrossRef CAS PubMed;
(d) H.-J. Feng, W.-X. Gao, Y.-J. Lin and G.-X. Jin, Chem.–Eur. J., 2019, 25, 15687 CrossRef CAS PubMed.
- For Star-of-David catenanes and 8 and 10-crossing torus link:
(a) D. A. Leigh, R. G. Pritchard and A. J. Stephens, Nat. Chem., 2014, 6, 978 CrossRef CAS PubMed;
(b) D. P. August, J. Jaramillo-Garcia, D. A. Leigh, A. Valero and I. J. Vitorica-Yrezabal, J. Am. Chem. Soc., 2021, 143, 1154 CrossRef CAS PubMed;
(c) D. P. August, S. Borsley, S. L. Cockroft, F. Della Sala, D. A. Leigh and S. J. Webb, J. Am. Chem. Soc., 2020, 142, 18859 CrossRef CAS PubMed;
(d) Y. Inomata, T. Sawada and M. Fujita, Chem, 2020, 6, 294 CrossRef CAS;
(e) Y. Inomata, T. Sawada and M. Fujita, J. Am. Chem. Soc., 2021, 143, 16734 CrossRef CAS PubMed.
-
(a) A.-M. L. Fuller, D. A. Leigh and P. J. Lusby, J. Am. Chem. Soc., 2010, 132, 4954 CrossRef CAS PubMed;
(b) R. Hayashi, P. Slavík, Y. Mutoh, T. Kasama and S. Saito, J. Org. Chem., 2016, 81, 1175 CrossRef CAS PubMed;
(c) J. Y. H. Man and H. Y. Au-Yeung, Beilstein J. Org. Chem., 2019, 15, 1829 CrossRef CAS PubMed.
-
(a) D. Armspach, P. R. Ashton, C. P. Moore, N. Spencer, J. F. Stoddart, T. J. Wear and D. J. Williams, Angew. Chem., Int. Ed. Engl., 1993, 32, 854 CrossRef;
(b) J. Li, P. Nowak, H. Fanlo-Virgós and S. Otto, Chem. Sci., 2014, 5, 4968 RSC.
-
(a) A. W. H. Ng, C.-C. Yee and H. Y. Au-Yeung, Angew. Chem., Int. Ed., 2019, 58, 17375 CrossRef CAS PubMed;
(b) H. Y. Au-Yeung and A. W. H. Ng, Synlett, 2020, 31, 309 CrossRef CAS;
(c) A. W. H. Ng, Y. H. Leung and H. Y. Au-Yeung, Org. Chem. Front., 2021, 8, 2182 RSC.
-
(a) E. M. G. Jamieson, F. Modicom and S. M. Goldup, Chem. Soc. Rev., 2018, 47, 5266 RSC;
(b) J. R. J. Maynard and S. M. Goldup, Chem, 2020, 6, 1914 CrossRef CAS;
(c) N. H. Evans, Chem.–Eur. J., 2018, 24, 3101 CrossRef CAS PubMed.
- For examples, see:
(a) Y. Kaida, Y. Okamoto, J.-C. Chambron, D. K. Mitchell and J.-P. Sauvage, Tetrahedron Lett., 1993, 34, 1019 CrossRef CAS;
(b) M.-K. Chung, P. S. White, S. J. Lee and M. R. Gagné, Angew. Chem., Int. Ed., 2009, 48, 8683 CrossRef CAS PubMed;
(c) H. V. Schröder, Y. Zhang and A. J. Link, Nat. Chem., 2021, 13, 850 CrossRef PubMed;
(d) D. K. Mitchell and J.-P. Sauvage, Angew. Chem., Int. Ed. Engl., 1988, 27, 930 CrossRef;
(e) T. Prakasam, M. Lusi, E. Nauha, J.-C. Olsen, M. Sy, C. Platas-Iglesias, L. J. Charbonnière and A. Trabolsi, Chem. Commun., 2015, 51, 5840 RSC.
-
(a) C. P. McArdle, S. Van, M. C. Jennings and R. J. Puddephatt, J. Am. Chem. Soc., 2002, 124, 3959 CrossRef CAS PubMed;
(b) A. Theil, C. Mauve, M.-T. Adeline, A. Marinetti and J.-P. Sauvage, Angew. Chem., Int. Ed., 2006, 45, 2104 CrossRef CAS PubMed.
-
(a) C. O. Dietrich-Buchecker, A. Edel, J. P. Kintzinger and J. P. Sauvage, Tetrahedron, 1987, 43, 333 CrossRef CAS;
(b) S. A. Vignon, J. Wong, H.-R. Tseng and J. F. Stoddart, Org. Lett., 2004, 6, 1095 CrossRef CAS PubMed.
-
(a) I. T. Harrison and S. Harrison, J. Am. Chem. Soc., 1967, 89, 5723 CrossRef CAS;
(b) P. R. Aston, I. Baxter, M. C. T. Fyfe, F. M. Raymo, N. Spencer, J. F. Stoddart, A. J. P. White and D. J. Williams, J. Am. Chem. Soc., 1998, 120, 2297 CrossRef;
(c) M. Xue, Y. Yang, X. Chi, X. Yan and F. Huang, Chem. Rev., 2015, 115, 7398 CrossRef CAS PubMed.
-
(a) J.-F. Ayme, J. E. Beves, C. J. Campbella and D. A. Leigh, Chem. Soc. Rev., 2013, 42, 1700 RSC;
(b) K. E. Horner, M. A. Miller, J. W. Steed and P. M. Sutcliffe, Chem. Soc. Rev., 2016, 45, 6432 RSC;
(c)
C. Dietrich-Buchecker, B. X. Colasson and J. P. Sauvage, Molecular Knots, Templates in Chemistry II. Topics in Current Chemistry, ed. C. A. Schalley, F. Vögtle and K. H. Dötz, Springer, Berlin, Heidelberg, 2005, vol. 249, p. 261 Search PubMed.
-
(a) D. A. Leigh, J. J. Danon, S. D. P. Fielden, J.-F. Lemonnier, G. F. S. Whitehead and S. L. Woltering, Nat. Chem., 2021, 13, 117 CrossRef CAS PubMed;
(b) D. P. August, R. A. W. Dryfe, S. J. Haigh, P. R. C. Kent, D. A. Leigh, J.-F. Lemonnier, Z. Li, C. A. Muryn, L. I. Palmer, Y. Song, G. F. S. Whitehead and R. J. Young, Nature, 2020, 588, 429 CrossRef CAS PubMed;
(c) D. A. Leigh, F. Schaufelberger, L. Pirvu, J. H. Stenlid, D. P. August and J. Segard, Nature, 2020, 584, 562 CrossRef CAS PubMed;
(d) D. A. Leigh, L. Pirvu and F. Schaufelberger, J. Am. Chem. Soc., 2019, 141, 6054 CrossRef CAS PubMed;
(e) J. J. Danon, D. A. Leigh, S. Pisano, A. Valero and I. J. Vitorica-Yrezabal, Angew. Chem., Int. Ed., 2018, 57, 13833 CrossRef CAS PubMed;
(f) L. Zhang, A. J. Stephens, A. L. Nussbaumer, J.-F. Lemonnier, P. Jurček, I. J. Vitorica-Yrezabal and D. A. Leigh, Nat. Chem., 2018, 10, 1083 CrossRef CAS PubMed.
-
(a) H. Y. Au-Yeung, C.-C. Yee, A. W. H. Ng and K. Hu, Inorg. Chem., 2018, 57, 3475 CrossRef CAS PubMed;
(b) N. H. Pérez and J. E. M. Lewis, Org. Biomol. Chem., 2020, 18, 6757 RSC.
- E. Wasserman, J. Am. Chem. Soc., 1960, 82, 4433 CrossRef CAS.
- I. T. Harrison and S. Harrison, J. Am. Chem. Soc., 1967, 89, 5723 CrossRef CAS.
-
(a) J. F. Stoddart and H.-R. Tseng, Proc. Natl. Acad. Sci. U. S. A., 2002, 99, 4797 CrossRef CAS PubMed;
(b) S. J. Loeb, Chem. Soc. Rev., 2007, 36, 226 RSC;
(c) M. S. Vickers and P. D. Beer, Chem. Soc. Rev., 2007, 36, 211 RSC.
- For examples see:
(a) K. D. Hänni and D. A. Leigh, Chem. Soc. Rev., 2010, 39, 1240 RSC;
(b) O. Š. Miljanić, W. R. Dichtel, S. I. Khan, S. Mortezaei, J. R. Heath and J. F. Stoddart, J. Am. Chem. Soc., 2007, 129, 8236 CrossRef PubMed;
(c) R. Kumar, A. El-Sagheer, J. Tumpane, P. Lincoln, L. M. Wilhelmsson and T. Brown, J. Am. Chem. Soc., 2007, 129, 6859 CrossRef CAS PubMed.
- For examples see:
(a) C. Dietrich-Buchecker, G. Rapenne and J.-P. Sauvage, Coord. Chem. Rev., 1999, 185–186, 167 CrossRef CAS;
(b) B. Mohr, J.-P. Sauvage, R. H. Grubbs and M. Weck, Angew. Chem., Int. Ed. Engl., 1997, 36, 1308 CrossRef CAS;
(c) M. Weck, B. Mohr, J.-P. Sauvage and R. H. Grubbs, J. Org. Chem., 1999, 64, 5463 CrossRef CAS PubMed;
(d) E. N. Guidry, S. J. Cantrill, J. F. Stoddart and R. H. Grubbs, Org. Lett., 2005, 7, 2129 CrossRef CAS PubMed.
- For examples see:
(a) C. D. Meyer, C. S. Joiner and J. F. Stoddart, Chem. Soc. Rev., 2007, 36, 1705 RSC;
(b) D. A. Leigh, P. J. Lusby, S. J. Teat, A. J. Wilson and J. K. Y. Wong, Angew. Chem., Int. Ed., 2001, 40, 1538 CrossRef CAS;
(c) G. Koshkakaryan, D. Cao, L. M. Klivansky, S. J. Teat, J. L. Tran and Y. Liu, Org. Lett., 2010, 12, 1528 CrossRef CAS PubMed;
(d) Z. Li, F. Hu, G. Liu, W. Xue, X. Chen, S. H. Liu and J. Yin, Org. Biomol. Chem., 2014, 12, 7702 RSC;
(e) K. Caprice, M. Pupier, A. Kruve, C. A. Schalley and F. B. L. Cougnon, Chem. Sci., 2018, 9, 1317 RSC.
- For examples see:
(a) K. R. West, R. F. Ludlow, P. T. Corbett, P. Besenius, F. M. Mansfeld, P. A. G. Cormack, D. C. Sherrington, J. M. Goodman, M. C. A. Stuart and S. Otto, J. Am. Chem. Soc., 2008, 130, 10834 CrossRef CAS PubMed;
(b) H. Y. Au-Yeung, G. D. Pantoş and J. K. M. Sanders, J. Am. Chem. Soc., 2009, 131, 16030 CrossRef CAS PubMed;
(c) H. Y. Au-Yeung, G. D. Pantoş and J. K. M. Sanders, Angew. Chem., Int. Ed., 2010, 49, 5331 CrossRef CAS PubMed;
(d) H. Y. Au-Yeung, G. D. Pantoş and J. K. M. Sanders, J. Org. Chem., 2011, 76, 1257 CrossRef CAS PubMed;
(e) F. B. L. Cougnon, H. Y. Au-Yeung, G. D. Pantoş and J. K. M. Sanders, J. Am. Chem. Soc., 2011, 133, 3198 CrossRef CAS PubMed;
(f) T.-M. Gianga, E. Audibert, A. Trandafir, G. Kociok-Köhn and G. D. Pantoş, Chem. Sci., 2020, 11, 9685 RSC;
(g) T.-M. Gianga and G. D. Pantoş, Nat. Commun., 2020, 11, 3528 CrossRef CAS PubMed.
-
(a) J. D. Crowley, S. M. Goldup, A.-L. Lee, D. A. Leigh and R. T. McBurney, Chem. Soc. Rev., 2009, 38, 1530 RSC;
(b) M. Denis and S. M. Goldup, Nat. Rev. Chem., 2017, 1, 61 CrossRef CAS;
(c) Y. Sato, R. Yamasaki and S. Saito, Angew. Chem., Int. Ed., 2009, 48, 504 CrossRef CAS PubMed;
(d) J. E. M. Lewis, F. Modicom and S. M. Goldup, J. Am. Chem. Soc., 2018, 140, 4787 CrossRef CAS PubMed;
(e) P. E. Barran, H. L. Cole, S. M. Goldup, D. A. Leigh, P. R. McGonigal, M. D. Symes, J. Wu and M. Zengerle, Angew. Chem., Int. Ed., 2011, 50, 12280 CrossRef CAS PubMed.
-
(a) X. Hou, C. Ke and J. F. Stoddart, Chem. Soc. Rev., 2016, 45, 3766 RSC;
(b) W. L. Mock, T. A. Irra, J. P. Wepsiec and T. L. Manimaran, J. Org. Chem., 1983, 48, 3619 CrossRef CAS;
(c) D. Tuncel and J. H. G. Steinke, Chem. Commun., 2002, 496 RSC;
(d) C. Ke, R. A. Smaldone, T. Kikuchi, H. Li, A. P. Davis and J. F. Stoddart, Angew. Chem., Int. Ed., 2013, 52, 381 CrossRef CAS PubMed;
(e) C. Ke, N. L. Strutt, H. Li, X. Hou, K. J. Hartlieb, P. R. McGonigal, Z. Ma, J. Iehl, C. L. Stern, C. Cheng, Z. Zhu, N. A. Vermeulen, T. J. Meade, Y. Y. Botros and J. F. Stoddart, J. Am. Chem. Soc., 2013, 135, 17019 CrossRef CAS PubMed;
(f) M. E. Belowich, C. Valente, R. A. Smaldone, D. C. Friedman, J. Thiel, L. Cronin and J. F. Stoddart, J. Am. Chem. Soc., 2012, 134, 5243 CrossRef CAS PubMed;
(g) A. W. H. Ng, C.-C. Yee, K. Wang and H. Y. Au-Yeung, Beilstein J. Org. Chem., 2018, 14, 1846 CrossRef CAS PubMed.
-
(a) G. Schill and A. Lüttringhaus, Angew. Chem., Int. Ed. Engl., 1964, 3, 546 CrossRef;
(b) Ö. Ünsal and A. Godt, Chem.–Eur. J., 1999, 5, 1728 CrossRef;
(c) M. R. Shah, S. Duda, B. Müller, A. Godt and A. Malik, J. Am. Chem. Soc., 2003, 125, 5408 CrossRef PubMed;
(d) Y. Nagawa, J.-I. Suga, K. Hiratani, E. Koyama and M. Kanesato, Chem. Commun., 2005, 749 RSC;
(e) K. Hirose, K. Nishihara, N. Harada, Y. Nakamura, D. Masuda, M. Araki and Y. Tobe, Org. Lett., 2007, 9, 2969 CrossRef CAS PubMed;
(f) S. Pilon, S. I. Jørgensen and J. H. van Maarseveen, Chem.–Eur. J., 2021, 27, 2310 CrossRef CAS PubMed;
(g) Y. Segawa, M. Kuwayama, Y. Hijikata, M. Fushimi, T. Nishihara, J. Pirillo, J. Shirasaki, N. Kubota and K. Itami, Science, 2019, 365, 272 CrossRef CAS PubMed;
(h) Y. Segawa, M. Kuwayama and K. Itami, Org. Lett., 2020, 22, 1067 CrossRef CAS PubMed;
(i) S. Pilon, S. I. Jørgensen and J. H. van Maarseveen, ACS Org. Inorg. Au, 2021, 1, 37 CrossRef CAS PubMed.
- J. E. M. Lewis, P. D. Beer, S. J. Loeb and S. M. Goldup, Chem. Soc. Rev., 2017, 46, 2577 RSC.
- For examples see:
(a) C. O. Dietrich-Buchecker, J.-P. Kintzinger and J.-P. Sauvage, Tetrahedron Lett., 1983, 24, 5095 CrossRef CAS;
(b) J.-P. Sauvage, Acc. Chem. Res., 1990, 23, 319 CrossRef CAS;
(c) J. D. Megiatto Jr and D. I. Schuster, J. Am. Chem. Soc., 2008, 130, 12872 CrossRef PubMed;
(d) J. A. Berrocal, M. M. L. Nieuwenhuizen, L. Mandolini, E. W. Meijer and S. Di Stefano, Org. Biomol. Chem., 2014, 12, 6167 RSC;
(e) K.-L. Tong, C.-C. Yee, Y. C. Tse and H. Y. Au-Yeung, Inorg. Chem. Front., 2016, 3, 348 RSC.
-
(a) C. Dietrich-Buchecker, G. Rapenne and J.-P. Sauvage, Chem. Commun., 1997, 2053 RSC;
(b) G. Rapenne, C. Dietrich-Buchecker and J.-P. Sauvage, J. Am. Chem. Soc., 1999, 121, 994 CrossRef CAS.
- For examples see:
(a) P. Mobian, J.-M. Kern and J.-P. Sauvage, Angew. Chem., Int. Ed., 2004, 43, 2392 CrossRef CAS PubMed;
(b) C. Dietrich-Buchecker, B. Colasso, D. Jouvenot and J.-P. Sauvage, Chem.–Eur. J., 2005, 11, 4374 CrossRef CAS PubMed;
(c) D. A. Leigh, P. J. Lusby, R. T. McBurney, A. Morelli, A. M. Z. Slawin, A. R. Thomson and D. B. Walker, J. Am. Chem. Soc., 2009, 131, 3762 CrossRef CAS PubMed;
(d) S. M. Goldup, D. A. Leigh, P. J. Lusby, R. T. McBurney and A. M. Z. Slawin, Angew. Chem., Int. Ed., 2008, 47, 6999 CrossRef CAS PubMed;
(e) C. Browne, T. K. Ronson and J. R. Nitschke, Angew. Chem., Int. Ed., 2014, 53, 10701 CrossRef CAS PubMed;
(f) C. Lincheneau, B. Jean-Denisa and T. Gunnlaugsson, Chem. Commun., 2014, 50, 2857 RSC;
(g) S.-T. Tung, C.-C. Lai, Y.-H. Liu, S.-M. Peng and S.-H. Chiu, Angew. Chem., Int. Ed., 2013, 52, 13269 CrossRef CAS PubMed;
(h) R. Mitra, M. Thiele, F. Octa-Smolin, M. C. Letzel and J. Niemeyer, Chem. Commun., 2016, 52, 5977 RSC.
-
(a) N. Ponnuswamy, F. B. L. Cougnon, G. D. Pantoş and J. K. M. Sanders, J. Am. Chem. Soc., 2014, 136, 8243 CrossRef CAS PubMed;
(b) F. B. L. Cougnon, K. Caprice, M. Pupier, A. Bauza and A. Frontera, J. Am. Chem. Soc., 2018, 140, 12442 CrossRef CAS PubMed.
- For examples of Solomon link obtained from trinuclear helicate, see:
(a) J. F. Nierengarten, C. O. Dietrich-Buchecker and J.-P. Sauvage, J. Am. Chem. Soc., 1994, 116, 375 CrossRef CAS PubMed;
(b) C. Dietrich-Buchecker and J.-P. Sauvage, Chem. Commun., 1999, 615 RSC.
- For examples of Solomon link obtained from 2 × 2 grid, see: J. E. Beves, J. J. Danon, D. A. Leigh, J.-F. Lemonnier and I. J. Vitorica-Yrezabal, Angew. Chem., Int. Ed., 54, 2015, 54, 7555 Search PubMed.
- For examples see:
(a) C. Dietrich-Buchecker, B. Colasson, D. Jouvenot and J.-P. Sauvage, Chem.–Eur. J., 2005, 11, 4374 CrossRef CAS PubMed;
(b) J. J. Danon, A. Krüger, D. A. Leigh, J.-F. Lemonnier, A. J. Stephens, I. J. Vitorica-Yrezabal and S. L. Woltering, Science, 2017, 355, 159 CrossRef CAS PubMed;
(c) L. Zhang, D. P. August, J. Zhong, G. F. S. Whitehead, I. J. Vitorica-Yrezabal and D. A. Leigh, J. Am. Chem. Soc., 2018, 140, 4982 CrossRef CAS PubMed;
(d) J. Zhong, L. Zhang, D. P. August, G. F. S. Whitehead and D. A. Leigh, J. Am. Chem. Soc., 2019, 141, 14249 CrossRef CAS PubMed.
-
(a) N. Belfrekh, C. Dietrich-Buchecker and J.-P. Sauvage, Inorg. Chem., 2000, 39, 5169 CrossRef CAS PubMed;
(b) D. A. Leigh, P. J. Lusby, R. T. McBurney, A. Morelli, A. M. Z. Slawin, A. R. Thomson and D. B. Walker, J. Am. Chem. Soc., 2009, 131, 3762 CrossRef CAS PubMed;
(c) A.-M. L. Fuller, D. A. Leigh, P. J. Lusby, A. M. Z. Slawin and D. B. Walker, J. Am. Chem. Soc., 2005, 127, 12612 CrossRef CAS PubMed;
(d) C.-C. Yee, A. W. H. Ng and H. Y. Au-Yeung, Chem. Commun., 2019, 55, 6169 RSC.
- For examples see:
(a) A. Westcott, J. Fisher, L. P. Harding, P. Rizkallah and M. J. Hardie, J. Am. Chem. Soc., 2008, 130, 2950 CrossRef CAS PubMed;
(b) C. S. Wood, T. K. Ronson, A. M. J. J. Belenguer Holstein and J. R. Nitschke, Nat. Chem., 2015, 7, 354 CrossRef CAS PubMed;
(c) S. P. Black, A. R. Stefankiewicz, M. M. J. Smulders, D. Sattler, C. A. Schalley, J. R. Nitschke and J. K. M. Sanders, Angew. Chem., Int. Ed., 2013, 52, 5749 CrossRef CAS PubMed;
(d) S. P. Black, D. M. Wood, F. B. Schwarz, T. K. Ronson, J. J. Holstein, A. R. Stefankiewicz, C. A. Schalley, J. K. M. Sanders and J. R. Nitschke, Chem. Sci., 2016, 7, 2614 RSC;
(e) K. S. Chichak, S. J. Cantrill, A. R. Pease, S. H. Chiu, G. W. Cave, J. L. Atwood and J. F. Stoddart, Science, 2004, 304, 1308 CrossRef CAS PubMed;
(f) C. D. Pentecost, A. J. Peter, K. S. Chichak, G. W. V. Cave, S. J. Cantril and J. F. Stoddart, Angew. Chem., Int. Ed., 2006, 45, 4099 CrossRef CAS PubMed;
(g) S.-L. Huang, Y.-J. Lin, T. S. A. Hor and G.-X. Jin, J. Am. Chem. Soc., 2013, 135, 8125 CrossRef CAS PubMed;
(h) S.-L. Huang, Y.-J. Lin, Z.-H. Li and G.-X. Jin, Angew. Chem., Int. Ed., 2014, 53, 11218 CrossRef CAS PubMed;
(i) Y. Lu, Y.-X. Deng, Y.-J. Lin, Y.-F. Han, L.-H. Weng, Z.-H. Li and G.-X. Jin, Chem, 2017, 3, 110 CrossRef CAS;
(j) T. Kim, N. Singh, J. Oh, E.-H. Kim, J. Jung, H. Kim and K.-W. Chi, J. Am. Chem. Soc., 2016, 138, 8368 CrossRef CAS PubMed.
-
(a) A. Harada, A. Hashidzume, H. Yamaguchi and Y. Takashima, Chem. Rev., 2009, 109, 5974 CrossRef CAS PubMed;
(b)
K. Ito, K. Mayumi and K. Kato, Polyrotaxane and Slide-Ring Materials, The Royal Society of Chemistry, Cambridge, 2016 Search PubMed;
(c) Y. Qiu, B. Song, C. Pezzato, D. Shen, W. Liu, L. Zhang, Y. Feng, Q. H. Guo, K. Cai, W. Li, H. Chen, M. T. Nguyen, Y. Shi, C. Cheng, R. D. Astumian, X. Li and J. F. Stoddart, Science, 2020, 368, 1247 CrossRef CAS PubMed;
(d) M. E. Belowich, C. Valente, R. A. Smaldone, D. C. Friedman, J. Thiel, L. Cronin and J. F. Stoddart, J. Am. Chem. Soc., 2012, 134, 5243 CrossRef CAS PubMed;
(e) N. Momčilović, P. G. Clark, A. J. Boydston and R. H. Grubbs, J. Am. Chem. Soc., 2011, 133, 19087 CrossRef PubMed;
(f) M. D. Cornelissen, S. Pilon, L. Steemers, M. J. Wanner, S. Frölke, E. Zuidinga, S. I. Jørgensen, J. I. van der Vlugt and J. H. van Maarseveen, J. Org. Chem., 2020, 85, 3146 CrossRef CAS PubMed.
- For examples of radial [n]catenanes (n ≥ 5):
(a) F. Bitsch, C. O. Dietrich-Buchecker, A.-K. Khémiss, J.-P. Sauvage and A. Van Dorsselaer, J. Am. Chem. Soc., 1991, 113, 4023 CrossRef CAS;
(b) D. Whang, K.-M. Park, J. Heo, P. Ashton and K. Kim, J. Am. Chem. Soc., 1998, 120, 4899 CrossRef CAS;
(c) S. Li, J. Huang, F. Zhou, T. R. Cook, X. Yan, Y. Ye, B. Zhu, B. Zheng and P. J. Stang, J. Am. Chem. Soc., 2014, 136, 5908 CrossRef CAS PubMed;
(d) S. Dasgupta and J. Wu, Org. Biomol. Chem., 2011, 9, 3504 RSC;
(e) K.-M. Park, S.-Y. Kim, J. Heo, D. Whang, S. Sakamoto, K. Yamaguchi and K. Kim, J. Am. Chem. Soc., 2002, 124, 2140 CrossRef CAS PubMed;
(f) M. Okada and A. Harada, Macromolecules, 2003, 36, 9701 CrossRef CAS;
(g) T. Higashi, K. Morita, X. Song, J. Zhu, A. Tamura, N. Yui, K. Motoyama, H. Arima and J. Li, Commun. Chem., 2019, 2, 78 CrossRef;
(h) S.-G. Roh, K.-M. Park, G.-J. Park, S. Sakamoto, K. Yamaguchi and K. Kim, Angew. Chem., Int. Ed., 1999, 38, 637 CrossRef;
(i) P. Gawel, S. L. Woltering, Y. Xiong, K. E. Christensen and H. L. Anderson, Angew. Chem., Int. Ed., 2021, 60, 5941 CrossRef CAS PubMed.
-
(a) T. Takata, N. Kihara and Y. Furusho, Adv. Polym. Sci., 2004, 171, 1 CrossRef CAS;
(b) Z. Niu and H. W. Gibson, Chem. Rev., 2009, 109, 6024 CrossRef CAS PubMed;
(c) L. Fang, M. A. Olson, D. Benítez, E. Tkatchouk, W. A. Goddard III and J. F. Stoddart, Chem. Soc. Rev., 2010, 39, 17 RSC;
(d) M. Arunachalam and H. W. Gibson, Prog. Polym. Sci., 2014, 39, 1043 CrossRef CAS;
(e) L. F. Hart, J. E. Hertzog, P. M. Rauscher, B. W. Rawe, M. M. Tranquilli and S. J. Rowan, Nat. Rev. Mater., 2021, 6, 508 CrossRef CAS.
- D. B. Amabilino, P. R. Ashton, A. S. Reder, N. Spencer and J. F. Stoddart, Angew. Chem., Int. Ed. Engl., 1994, 33, 1286 CrossRef.
- P. R. Ashton, V. Baldoni, V. Balzani, C. G. Claessens, A. Credi, H. D. A. Hoffmann, F. M. Raymo, J. F. Stoddart, M. Venturi, A. J. P. White and D. J. Williams, Eur. J. Org. Chem., 2000, 1121 CrossRef CAS.
- F. Schwanke, O. Safarowsky, C. Heim, G. Silva and F. Vögtle, Helv. Chim. Acta, 2000, 83, 3279 CrossRef CAS.
- H. Iwamoto, S. Tafuku, Y. Sato, W. Takizawa, W. Katagiri, E. Tayama, E. Hasegawa, Y. Fukazawa and T. Haino, Chem. Commun., 2016, 52, 319 RSC.
- N. D. Colley, M. A. Nosiglia, L. Li, F. Amir, C. Chang, A. F. Greene, J. M. Fisher, R. Li, X. Li and J. C. Barnes, Inorg. Chem., 2020, 59, 10450 CrossRef CAS PubMed.
- Y. Lu, D. Liu, Y.-J. Lin, Z.-H. Li, F. E. Hahn and G.-X. Jin, J. Am. Chem. Soc., 2021, 143, 12404 CrossRef CAS PubMed.
- Q. Wu, P. M. Rauscher, X. Lang, R. J. Wojtecki, J. J. de Pablo, M. J. A. Hore and S. J. Rowan, Science, 2017, 358, 1434 CrossRef CAS PubMed.
- D. B. Amabilino, P. R. Ashton, S. E. Boyd, J. Y. Lee, S. Menzer, J. F. Stoddart and D. J. Williams, Angew. Chem., Int. Ed. Engl., 1997, 36, 2070 CrossRef CAS.
-
(a) K. Wang, C.-C. Yee and H. Y. Au-Yeung, Chem. Sci., 2016, 7, 2787 RSC;
(b) A. W. H. Ng, S. K.-M. Lai, C.-C. Yee and H. Y. Au-Yeung, Angew. Chem., Int. Ed., 2022, 61, e202110200 CrossRef CAS PubMed.
- For examples see:
(a) C.-H. Lu, A. Cecconello, X.-J. Qi, N. Wu, S.-S. Jester, M. Famulok, M. Matthies, T.-L. Schmidt and I. Willner, Nano Lett., 2015, 15, 7133 CrossRef PubMed;
(b) K. Endo, T. Shiroi, N. Murata, G. Kojima and T. Yamanaka, Macromolecules, 2004, 37, 3143 CrossRef CAS;
(c) K. Endo and T. Yamanaka, Macromolecules, 2006, 39, 4038 CrossRef CAS;
(d) A. Peil, P. Zhan and N. Liu, Small, 2020, 16, 1905987 CrossRef CAS PubMed.
- For an example of a [2]catenane with dendritic substituents: C. Reuter, G. Pawlitzki, U. Wörsdörfer, M. Plevoets, A. Mohry, T. Kubota, Y. Okamoto and F. Vögtle, Eur. J. Org. Chem., 2000, 3059 CrossRef CAS.
- For examples of rotaxane dendrimers see:
(a) J. W. Lee and K. Kim, Top. Curr. Chem., 2003, 228, 111 CrossRef CAS PubMed;
(b) K. C.-F. Leung, F. Aricó, S. J. Cantrill and J. F. Stoddart, J. Am. Chem. Soc., 2005, 127, 5808 CrossRef CAS PubMed;
(c) W. Wang, L.-J. Chen, X.-Q. Wang, B. Sun, X. Li, Y. Zhang, J. Shi, Y. Yu, L. Zhang, M. Liu and H.-B. Yang, Proc. Natl. Acad. Sci. U. S. A., 2015, 112, 5597 CrossRef CAS PubMed;
(d) C.-S. Kwan, R. Zhao, M. A. V. Hove, Z. Cai and K. C.-F. Leung, Nat. Commun., 2018, 9, 497 CrossRef PubMed;
(e) X.-Q. Wang, W. Wang, W.-J. Li, L.-J. Chen, R. Yao, G.-Q. Yin, Y.-X. Wang, Y. Zhang, J. Huang, H. Tan, Y. Yu, X. Li, L. Xu and H.-B. Yang, Nat. Commun., 2018, 9, 3190 CrossRef PubMed;
(f) X.-Q. Wang, W.-J. Li, W. Wang, J. Wen, Y. Zhang, H. Tan and H.-B. Yang, J. Am. Chem. Soc., 2019, 141, 13923 CrossRef CAS PubMed;
(g) W.-J. Li, W. Wang, X.-Q. Wang, M. Li, Y. Ke, R. Yao, J. Wen, G.-Q. Yin, B. Jiang, X. Li, P. Yin and H.-B. Yang, J. Am. Chem. Soc., 2020, 142, 8473 CrossRef CAS PubMed.
-
(a) T. Sawada, M. Yamagami, K. Ohara, K. Yamaguchi and M. Fujita, Angew. Chem., Int. Ed., 2016, 55, 4519 CrossRef CAS PubMed;
(b) T. Sawada, A. Saito, K. Tamiya, K. Shimokawa, Y. Hisada and M. Fujita, Nat. Commun., 2019, 10, 921 CrossRef PubMed.
- T. Sawada, Y. Inomata, K. Shimokawa and M. Fujita, Nat. Commun., 2019, 10, 5687 CrossRef CAS PubMed.
- S. Datta, Y. Kato, S. Higashiharaguchi, K. Aratsu, A. Isobe, T. Saito, D. D. Prabhu, Y. Kitamoto, M. J. Hollamby, A. J. Smith, R. Dalgliesh, N. Mahmoudi, L. Pesce, C. Perego, G. M. Pavan and S. Yagai, Nature, 2020, 583, 400 CrossRef CAS PubMed.
- V. Marcos, A. J. Stephens, J. Jaramillo-Garcia, A. L. Nussbaumer, S. L. Woltering, A. Valero, J.-F. Lemonnier, I. J. Vitorica-Yrezabal and D. A. Leigh, Science, 2016, 352, 1555 CrossRef CAS PubMed.
- H.-W. Schmidt and F. Würthner, Angew. Chem., Int. Ed., 2020, 59, 8766 CrossRef CAS PubMed.
- M. Zhang and G. De Bo, J. Am. Chem. Soc., 2020, 142, 5029 CrossRef CAS PubMed.
- For reviews on MIM-based molecular switches and machines, see:
(a) S. Kassem, T. van Leeuwen, A. S. Lubbe, M. R. Wilson, B. L. Feringa and D. A. Leigh, Chem. Soc. Rev., 2017, 46, 2592 RSC;
(b) J. J. Davis, G. A. Orlowski, H. Rahman and P. D. Beer, Chem. Commun., 2010, 46, 54 RSC;
(c) H.-Y. Zhou, Y. Han and C.-F. Chen, Mater. Chem. Front., 2020, 4, 12 RSC;
(d) M. Baroncini, S. Silvi and A. Credi, Chem. Rev., 2020, 120, 200 CrossRef CAS PubMed;
(e) Y. Feng, M. Ovalle, J. S. W. Seale, C. K. Lee, D. J. Kim, R. D. Astumian and J. F. Stoddart, J. Am. Chem. Soc., 2021, 143, 5569 CrossRef CAS PubMed;
(f) K. Cai, L. Zhang, R. D. Astumian and J. F. Stoddart, Nat. Rev. Chem., 2021, 5, 447 CrossRef CAS;
(g) E. Moulin, L. Faour, C. C. Carmona-Vargas and N. Giuseppone, Adv. Mater., 2020, 32, 1906036 CrossRef CAS PubMed;
(h) A. W. Heard and S. M. Goldup, ACS Cent. Sci., 2020, 6, 117 CrossRef CAS PubMed;
(i) K. Zhu, G. Baggi and S. J. Loeb, Nat. Chem., 2018, 10, 625 CrossRef CAS PubMed.
-
(a) C. O. Dietrich-Buchecker and J.-P. Sauvage, Angew. Chem., Int. Ed. Engl., 1989, 28, 189 CrossRef;
(b) M. Meyer, A.-M. Albrecht-Gary, C. O. Dietrich-Buchecker and J.-P. Sauvage, J. Am. Chem. Soc., 1997, 119, 4599 CrossRef CAS;
(c) O. Lukin and F. Vögtle, Angew. Chem., Int. Ed., 2005, 44, 1456 CrossRef CAS PubMed.
- For examples see:
(a) Y.-J. Lee, T.-H. Ho, C.-C. Lai and S.-H. Chiu, Org. Biomol. Chem., 2016, 14, 1153 RSC;
(b) A. M. Albrecht-Gary, Z. Saad, C. O. Dietrich-Buchecker and J. P. Sauvage, J. Am. Chem. Soc., 1985, 107, 3205 CrossRef CAS;
(c) S. Duda and A. Godt, Eur. J. Org. Chem., 2003, 3412 CrossRef CAS;
(d) S. Dasgupta and J. Wu, Chem. Sci., 2012, 3, 425 RSC;
(e) C.-C. Hsu, N.-C. Chen, C.-C. Lai, Y.-H. Liu, S.-M. Peng and S.-H. Chiu, Angew. Chem., Int. Ed., 2008, 47, 7475 CrossRef CAS PubMed;
(f) H. Iwamoto, W. Takizawa, K. Itoh, T. Hagiwara, E. Tayama, E. Hasegawa and T. Haino, J. Org. Chem., 2013, 78, 5205 CrossRef CAS PubMed.
- L. Zhang, J.-F. Lemonnier, A. Acocella, M. Calvaresi, F. Zerbetto and D. A. Leigh, Proc. Natl. Acad. Sci. U. S. A., 2019, 116, 2452 CrossRef CAS PubMed.
- A. Kruve, K. Caprice, R. Lavendomme, J. M. Wollschläger, S. Schoder, H. V. Schröder, J. R. Nitschke, F. B. L. Cougnon and C. A. Schalley, Angew. Chem., Int. Ed., 2019, 58, 11324 CrossRef CAS PubMed.
- S. Yang, C.-X. Zhao, S. Crespi, X. Li, Q. Zhang, Z.-Y. Zhang, J. Mei, H. Tian and D.-H. Qu, Chem, 2021, 7, 1544 CAS.
- L. Hu, C.-H. Lu and I. Willner, Nano Lett., 2015, 15, 2099 CrossRef CAS PubMed.
-
(a) T.-Y. Tai, Y.-H. Liu, C.-C. Lai, S.-M. Peng and S.-H. Chiu, Org. Lett., 2019, 21, 5708 CrossRef CAS PubMed;
(b) K. Caprice, D. Pál, C. Besnard, B. Galmés, A. Frontera and F. B. L. Cougnon, J. Am. Chem. Soc., 2021, 143, 11957 CrossRef CAS PubMed;
(c) Y. Deng, S. K.-M. Lai, L. Kong and H. Y. Au-Yeung, Chem. Commun., 2021, 57, 2931 RSC.
- For examples of linear daisy chain, see:
(a) K. Iwaso, Y. Takashima and A. Harada, Nat. Chem., 2016, 8, 625 CrossRef CAS PubMed;
(b) A. Goujon, G. Mariani, T. Lang, E. Moulin, M. Rawiso, E. Buhler and N. Giuseppone, J. Am. Chem. Soc., 2017, 139, 4923 CrossRef CAS PubMed;
(c) Q. Zhang, S.-J. Rao, T. Xie, X. Li, T.-Y. Xu, D.-W. Li, D.-H. Qu, Y.-T. Long and H. Tian, Chem, 2018, 4, 2670 CrossRef CAS;
(d) A. Goujon, E. Moulin, G. Fuks and N. Giuseppone, CCS Chem., 2019, 1, 83 CAS.
- For examples of cyclic daisy chain, see:
(a) J.-C. Chang, S.-H. Tseng, C.-C. Lai, Y.-H. Liu, S.-M. Peng and S.-H. Chiu, Nat. Chem., 2016, 9, 128 CrossRef;
(b) T. Hoshino, M. Miyauchi, Y. Kawaguchi, H. Yamaguchi and A. Harada, J. Am. Chem. Soc., 2000, 122, 9876 CrossRef CAS;
(c) C. C. Carmona-Vargas and N. Giuseppone, Chem, 2021, 7, 174 CrossRef;
(d) K. Cai, B. Cui, B. Song, H. Wang, Y. Qiu, L. O. Jones, W. Liu, Y. Shi, S. Vemuri, D. Shen, T. Jiao, L. Zhang, H. Wu, H. Chen, Y. Jiao, Y. Wang, C. L. Stern, H. Li, G. C. Schatz, X. Li and J. F. Stoddart, Chem, 2021, 7, 174 CrossRef CAS.
- D. A. Leigh, L. Pirvu, F. Schaufelberger, D. J. Tetlow and L. Zhang, Angew. Chem., Int. Ed., 2018, 57, 10484 CrossRef CAS PubMed.
- For examples of catenane-based molecular machines, see:
(a) S. Erbas-Cakmak, S. D. P. Fielden, U. Karaca, D. A. Leigh, C. T. McTernan, D. J. Tetlow and M. R. Wilson, Science, 2017, 358, 340 CrossRef CAS PubMed;
(b) M. R. Wilson, J. Solà, A. Carlone, S. M. Goldup, N. Lebrasseur and D. A. Leigh, Nature, 2016, 534, 235 CrossRef CAS PubMed;
(c) D. A. Leigh, J. K. Y. Wong, F. Dehez and F. Zerbetto, Nature, 2003, 424, 174 CrossRef CAS PubMed;
(d) J. V. Hernández, E. R. Kay and D. A. Leigh, Science, 2004, 306, 1532 CrossRef PubMed;
(e) C. Biagini, S. Albano, R. Caruso, L. Mandolini, J. A. Berrocal and S. Di Stefano, Chem. Sci., 2018, 9, 181 RSC;
(f) J. Valero and M. Famulok, Angew. Chem., Int. Ed., 2020, 59, 16366 CrossRef CAS PubMed.
-
(a) Y. Okumura and K. Ito, Adv. Mater., 2001, 13, 485 CrossRef CAS;
(b) K. Ito, Polym. J., 2012, 44, 38 CrossRef CAS.
-
(a) H. Xing, Z. Li, W. Wang, P. Liu, J. Liu, Y. Song, Z. L. Wu, W. Zhang and F. Huang, CCS Chem., 2019, 1, 513 Search PubMed;
(b) H. Xing, Z. Li, Z. L. Wu and F. Huang, Macromol. Rapid Commun., 2018, 39, 1700361 CrossRef PubMed.
- For examples see:
(a) A. Caballero, F. Zapata, N. G. White, P. J. Costa, V. Félix and P. D. Beer, Angew. Chem., Int. Ed., 2012, 51, 1876 CrossRef CAS PubMed;
(b) N. H. Evans, H. Rahman, A. V. Leontiev, N. D. Greenham, G. A. Orlowski, Q. Zeng, R. M. J. Jacobs, C. J. Serpell, N. L. Kilah, J. J. Davis and P. D. Beer, Chem. Sci., 2012, 3, 1080 RSC;
(c) Y. Nakatani, Y. Furusho and E. Yashima, Angew. Chem., Int. Ed., 2010, 49, 5463 CrossRef CAS PubMed;
(d) G. Barin, M. Frasconi, S. M. Dyar, J. Iehl, O. Buyukcakir, A. A. Sarjeant, R. Carmieli, A. Coskun, M. R. Wasielewski and J. F. Stoddart, J. Am. Chem. Soc., 2013, 135, 2466 CrossRef CAS PubMed;
(e) J. P. Byrne, S. Blasco, A. B. Aletti, G. Hessman and T. Gunnlaugsson, Angew. Chem., Int. Ed., 2016, 55, 8938 CrossRef CAS PubMed;
(f) F. B. L. Cougnon, N. A. Jenkins, G. D. Pantoş and J. K. M. Sanders, Angew. Chem., Int. Ed., 2012, 51, 1443 CrossRef CAS PubMed;
(g) H. Y. Au-Yeung, G. D. Pantoş and J. K. M. Sanders, Proc. Natl. Acad. Sci. U. S. A., 2009, 106, 10466 CrossRef CAS PubMed;
(h) R. T. S. Lam, A. Belenguer, S. L. Roberts, C. Naumann, T. Jarrosson, S. Otto and J. K. M. Sanders, Science, 2005, 308, 667 CrossRef CAS PubMed.
- K. Caprice, M. Pupier, A. Bauzá, A. Frontera and F. B. L. Cougnon, Angew. Chem., Int. Ed., 2019, 58, 8053 CrossRef CAS PubMed.
- For examples see:
(a) A. Coskun, J. M. Spruell, G. Barin, A. C. Fahrenbach, R. S. Forgan, M. T. Colvin, R. Carmieli, D. Benítez, E. Tkatchouk, D. C. Friedman, A. A. Sarjeant, M. R. Wasielewski, W. A. Goddard III and J. F. Stoddart, J. Am. Chem. Soc., 2011, 133, 4538 CrossRef CAS PubMed;
(b) M. Frasconi, T. Kikuchi, D. Cao, Y. Wu, W.-G. Liu, S. M. Dyar, G. Barin, A. A. Sarjeant, C. L. Stern, R. Carmieli, C. Wang, M. R. Wasielewski, W. A. Goddard III and J. F. Stoddart, J. Am. Chem. Soc., 2014, 136, 11011 CrossRef CAS PubMed;
(c) J. M. Spruell, A. Coskun, D. C. Friedman, R. S. Forgan, A. A. Sarjeant, A. Trabolsi, A. C. Fahrenbach, G. Barin, W. F. Paxton, S. K. Dey, M. A. Olson, D. Benítez, E. Tkatchouk, M. T. Colvin, R. Carmielli, S. T. Caldwell, G. M. Rosair, S. G. Hewage, F. Duclairoir, J. L. Seymour, A. M. Z. Slawin, W. A. Goddard III, M. R. Wasielewski, G. Cooke and J. F. Stoddart, Nat. Chem., 2010, 2, 870 CrossRef CAS PubMed;
(d) M. T. Nguyen, D. P. Ferris, C. Pezzato, Y. Wang and J. F. Stoddart, Chem, 2018, 4, 2329 CrossRef CAS;
(e) K. Cai, H. Mao, W.-G. Liu, Y. Qiu, Y. Shi, L. Zhang, D. Shen, H. Chen, Y. Jiao, H. Wu, Z. Liu, Y. Feng, C. L. Stern, M. R. Wasielewski, W. A. Goddard III and J. F. Stoddart, J. Am. Chem. Soc., 2020, 142, 7190 CrossRef CAS PubMed.
-
(a) S. V. Kirner, D. M. Guldi, J. D. Megiatto, Jr and D. I. Schuster, Nanoscale, 2015, 7, 1145 RSC;
(b) J. D. Megiatto, Jr, D. I. Schuster, S. Abwandner, G. de Miguel and D. M. Guldi, J. Am. Chem. Soc., 2010, 132, 3847 CrossRef PubMed.
- F.-C. Hsueh, C.-Y. Tsai, C.-C. Lai, Y.-H. Liu, S.-M. Peng and S.-H. Chiu, Angew. Chem., Int. Ed., 2020, 59, 11278 CrossRef CAS PubMed.
-
(a) C. Dietrich-Buchecker, J.-P. Sauvage and J. M. Kern, J. Am. Chem. Soc., 1989, 111, 7791 CrossRef CAS;
(b) N. Armaroli, L. De Cola, V. Balzani, J.-P. Sauvage, C. O. Dietrich-Buchecker, J.-M. Kern and A. Bailal, J. Chem. Soc., Dalton Trans., 1993, 3241 RSC;
(c) C. Hamann, J.-M. Kern and J.-P. Sauvage, Inorg. Chem., 2003, 42, 1877 CrossRef CAS PubMed;
(d) M. Cirulli, A. Kaur, J. E. M. Lewis, Z. Zhang, J. A. Kitchen, S. M. Goldup and M. M. Roessler, J. Am. Chem. Soc., 2019, 141, 879 CrossRef CAS PubMed.
- M. Savastano, C. Bazzicalupi, C. Gellini and A. Bianchi, Chem. Commun., 2020, 56, 551 RSC.
-
(a) A. Martinez-Cuezva, A. Saura-Sanmartin, M. Alajarin and J. Berna, ACS Catal., 2020, 10, 7719 CrossRef CAS;
(b) N. Pairault and J. Niemeyer, Synlett, 2018, 29, 689 CrossRef CAS.
- Y. Jiao, L. Đorđević, H. Mao, R. M. Young, T. Jaynes, H. Chen, Y. Qiu, K. Cai, L. Zhang, X.-Y. Chen, Y. Feng, M. R. Wasielewski, S. I. Stupp and J. F. Stoddart, J. Am. Chem. Soc., 2021, 143, 8000 CrossRef CAS PubMed.
- L. Zhu, J. Li, J. Yang and H. Y. Au-Yeung, Chem. Sci., 2020, 11, 13008 RSC.
-
(a) R. Mitra, H. Zhu, S. Grimme and J. Niemeyer, Angew. Chem., Int. Ed., 2017, 56, 11456 CrossRef CAS PubMed;
(b) D. Jansen, J. Gramüller, F. Niemeyer, T. Schaller, M. C. Letzel, S. Grimme, H. Zhu, R. M. Gschwind and J. Niemeyer, Chem. Sci., 2020, 11, 4381 RSC.
- For reviews and examples of switchable rotaxane catalysts, see:
(a) D. A. Leigh, V. Marcos and M. R. Wilson, ACS Catal., 2014, 4, 4490 CrossRef CAS;
(b) C. Kwamen and J. Niemeyer, Chem.–Eur. J., 2021, 27, 175 CrossRef CAS PubMed;
(c)
C.-X. Zhao, Q. Zhang, G. London and D.-H. Qu, Functional Rotaxanes, Handbook of Macrocyclic Supramolecular Assembly, ed. Y. Liu, Y. Chen and H.-Y. Zhang, Springer, Singapore, 2020, p. 277 Search PubMed;
(d) K. Eichstaedt, J. J-Garcia, D. A. Leigh, V. Marcos, S. Pisano and T. A. Singleton, J. Am. Chem. Soc., 2017, 139, 9376 CrossRef CAS PubMed;
(e) J. Beswick, V. Blanco, G. De Bo, D. A. Leigh, U. Lewandowska, B. Lewandowski and K. Mishiro, Chem. Sci., 2015, 6, 140 RSC;
(f) C.-S. Kwan, A. S. C. Chan and K. C.-F. Leung, Org. Lett., 2016, 18, 976 CrossRef CAS PubMed;
(g) A. Martinez-Cuezva, A. Saura-Sanmartin, T. Nicolas-Garcia, C. Navarro, R.-A. Orenes, M. Alajarin and J. Berna, Chem. Sci., 2017, 8, 3775 RSC;
(h) J. Y. C. Lim, N. Yuntawattana, P. D. Beer and C. K. Williams, Angew. Chem., Int. Ed., 2019, 58, 6007 CrossRef CAS PubMed.
- G. Gil-Ramírez, S. Hoekman, M. O. Kitching, D. A. Leigh, I. J. Vitorica-Yrezabal and G. Zhang, J. Am. Chem. Soc., 2016, 138, 13159 CrossRef PubMed.
- F. Benyettou, T. Prakasam, A. Ramdas Nair, I.-I. Witzel, M. Alhashimi, T. Skorjanc, J.-C. Olsen, K. C. Sadler and A. Trabolsi, Chem. Sci., 2019, 10, 5884 RSC.
- G.-Y. Wu, X. Shi, H. Phan, H. Qu, Y.-X. Hu, G.-Q. Yin, X.-L. Zhao, X. Li, L. Xu, Q. Yu and H.-B. Yang, Nat. Commun., 2020, 11, 3178 CrossRef CAS PubMed.
- T. Kench, P. A. Summers, M. K. Kuimova, J. E. M. Lewis and R. Vilar, Angew. Chem., Int. Ed., 2021, 60, 10928 CrossRef CAS PubMed.
- E. A. Neal and S. M. Goldup, Chem. Commun., 2014, 50, 5128 RSC.
-
(a) M. Denis, J. E. M. Lewis, F. Modicom and S. M. Goldup, Chem, 2019, 5, 1357 CrossRef PubMed;
(b) A. de Juan, D. Lozano, A. W. Heard, M. A. Jinks, J. M. Suarez, G. J. Tizzard and S. M. Goldup, Nat. Chem., 2022, 14, 179 CrossRef CAS PubMed.
-
(a) A. van Quaethem, P. Lussis, D. A. Leigh, A.-S. Duwez and C.-A. Fustin, Chem. Sci., 2014, 5, 1449 RSC;
(b) C. He, G. Lamour, A. Xiao, J. Gsponer and H. Li, J. Am. Chem. Soc., 2014, 136, 11946 CrossRef CAS PubMed;
(c) H. Wang and H. Li, Chem. Sci., 2020, 11, 12512 RSC;
(d) M. Janke, Y. Rudzevich, O. Molokanova, T. Metzroth, I. Mey, G. Diezemann, P. E. Marszalek, J. Gauss, V. Böhmer and A. Janshoff, Nat. Nanotechnol., 2009, 4, 225 CrossRef CAS PubMed;
(e) G. D. Scott, K. S. Chichak, A. J. Peters, S. J. Cantrill, J. F. Stoddart and H. W. Jiang, Phys. Rev. B: Condens. Matter Mater. Phys., 2006, 74, 113404 CrossRef;
(f) C. Romero-Muñiz, D. Paredes-Roibás, C. López, A. Hernanz and J. M. Gavira-Vallejo, J. Phys. Chem. C, 2018, 122, 18102 CrossRef;
(g) C. Romero-Muñiz, D. Paredes-Roibás, A. Hernanz and J. M. Gavira-Vallejo, Phys. Chem. Chem. Phys., 2019, 21, 19538 RSC.
-
(a) N. Fedik, M. Kulichenko, D. Steglenko and A. I. Boldyrev, Chem. Commun., 2020, 56, 2711 RSC;
(b) L.-L. Dang, Z.-B. Sun, W.-L. Shan, Y.-J. Lin, Z.-H. Li and G.-X. Jin, Nat. Commun., 2019, 10, 2057 CrossRef PubMed;
(c) P. M. Rauscher, K. S. Schweizer, S. J. Rowan and J. J. de Pablo, Macromolecules, 2020, 53, 3390 CrossRef CAS;
(d) P. M. Rauscher, S. J. Rowan and J. J. de Pablo, ACS Macro Lett., 2018, 7, 938 CrossRef CAS;
(e) X. Feng, J. Gu, Q. Chen, J.-H. Lii, N. L. Allinger, Y. Xie and H. F. Schaefer III, J. Chem. Theory Comput., 2014, 10, 1511 CrossRef CAS PubMed;
(f) J.-H. Lii, N. L. Allinger, C.-H. Hu and H. F. Schaefer III, J. Comput. Chem., 2016, 37, 124 CrossRef CAS PubMed;
(g) Q. Liang and J. Yang, J. Chem. Theory Comput., 2021, 17, 6841 CrossRef CAS PubMed.
-
(a) Y.-Y. Fan, D. Chen, Z.-A. Huang, J. Zhu, C.-H. Tung, L.-Z. Wu and H. Cong, Nat. Commun., 2018, 9, 3037 CrossRef PubMed;
(b) S. Yu, A. Kupryakov, J. E. M. Lewis, V. Martí-Centelles, S. M. Goldup, J.-L. Pozzo, G. Jonusauskas and N. D. McClenaghan, Chem. Sci., 2021, 12, 9196 RSC;
(c) P. Rajamalli, F. Rizzi, W. Li, M. A. Jinks, A. K. Gupta, B. A. Laidlaw, I. D. W. Samuel, T. J. Penfold, S. M. Goldup and E. Zysman-Colman, Angew. Chem., Int. Ed., 2021, 60, 12066 CrossRef CAS PubMed;
(d) A. Diac, M. Matache, I. Grosu and N. D. Hădade, Adv. Synth. Catal., 2018, 360, 817 CrossRef CAS;
(e) A. W. H. Ng and H. Y. Au-Yeung, Chem.–Asian J., 2019, 14, 1602 CrossRef CAS PubMed;
(f) M. Cirulli, E. Salvadori, Z.-H. Zhang, M. Dommett, F. Tuna, H. Bamberger, J. E. M. Lewis, A. Kaur, G. J. Tizzard, J. van Slageren, R. Crespo-Otero, S. M. Goldup and M. M. Roessler, Angew. Chem., Int. Ed., 2021, 60, 16051 CrossRef CAS PubMed;
(g) B. H. Wilson, J. S. Ward, D. C. Young, J.-L. Liu, C. Mathonière, R. Clérac and P. E. Kruger, Angew. Chem., Int. Ed., 2022, 61, e202113837 CrossRef CAS PubMed.
- For examples see:
(a) E. D. Baranoff, J. Voignier, T. Yasuda, V. Heitz, J.-P. Sauvage and T. Kato, Angew. Chem., Int. Ed., 2007, 46, 4680 CrossRef CAS PubMed;
(b) C. P. Collier, G. Mattersteig, E. W. Wong, Y. Luo, K. Beverly, J. Sampaio, F. M. Raymo, J. F. Stoddart and J. R. Heath, Science, 2000, 289, 1172 CrossRef CAS PubMed;
(c) M. Asakawa, M. Higuchi, G. Mattersteig, T. Nakamura, A. R. Pease, F. M. Raymo, T. Shimizu and J. F. Stoddart, Adv. Mater., 2000, 12, 1099 CrossRef CAS;
(d) B. N. Ahamed, P. van Velthem, K. Robeyns and C.-A. Fustin, ACS Macro Lett., 2017, 6, 468 CrossRef CAS;
(e) J.-J. Lee, A. G. White, D. R. Rice and B. D. Smith, Chem. Commun., 2013, 49, 3016 RSC;
(f) B. H. Wilson and S. J. Loeb, Chem, 2020, 6, 1604 CrossRef CAS;
(g) Q. Li, C.-H. Sue, S. Basu, A. K. Shveyd, W. Zhang, G. Barin, L. Fang, A. A. Sarjeant, J. F. Stoddart and O. M. Yaghi, Angew. Chem., Int. Ed., 2010, 49, 6751 CrossRef CAS PubMed;
(h) Q. Chen, J. Sun, P. Li, I. Hod, P. Z. Moghadam, Z. S. Kean, R. Q. Snurr, J. T. Hupp, O. K. Farha and J. F. Stoddart, J. Am. Chem. Soc., 2016, 138, 14242 CrossRef CAS PubMed;
(i) W. Meng, S. Kondo, T. Ito, K. Komatsu, J. Pirillo, Y. Hijikata, Y. Ikuhara, T. Aida and H. Sato, Nature, 2021, 598, 298 CrossRef CAS PubMed.
|
This journal is © The Royal Society of Chemistry 2022 |