DOI:
10.1039/C9AY02246E
(Critical Review)
Anal. Methods, 2020,
12, 717-746
Loop-mediated isothermal amplification (LAMP) – review and classification of methods for sequence-specific detection
Received
17th October 2019
, Accepted 5th December 2019
First published on 9th January 2020
Abstract
In the course of the last 20 years, isothermal nucleic acid amplification tests have emerged as an important diagnostic tool, not only for clinical applications, but also for food quality control and environmental monitoring. Loop-mediated isothermal amplification (LAMP) is well known for its robust and highly sensitive and specific amplification of target DNA, which is achieved by utilizing up to six primers. Moreover, LAMP excels through its isothermal and energy efficient amplification requirements, rendering it a prime candidate for low-cost diagnostics and analysis at the point of need. Recently, methods for sequence-specific detection have gained more importance because, unlike sequence-independent detection methods, they are highly specific towards the target DNA. In the last 13 years, a variety of sequence-specific methods have emerged, based on a very diverse range of sensing techniques, including optical, magnetic, piezoelectric, electrochemical and magnetoresistive sensing. To give structure to the diverse multitude of sequence-specific methods, we created a systematic classification and provide a critical comparative evaluation according to a catalogue of criteria (analytical performance, multiplexing, quantification and instrumental requirements). Fluorescence-based detection, making up half of the methods, can be processed on open platforms and satisfies all the criteria listed before. Instrumental requirements are discussed in terms of complexity, portability and fluidic cartridges. In addition, the technological readiness level and the kind of platform (open versus method-tailored) are evaluated, the latter playing an important role in the miniaturization and automation of operational process steps. We also observe an increase in the use of smartphone-integrated sensors to improve LAMP-based point-of-need testing. In summary, recent developments in methods for the sequence-specific detection of LAMP demonstrate high potential for many future applications.
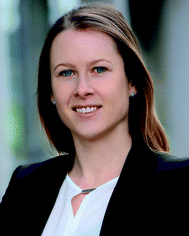 Lisa Becherer | Lisa Becherer studied chemistry at the University of Freiburg. In 2015 she graduated as a Master of Science with a master's thesis on spin–spin interactions on DNA aptamer complexes. She currently works as a PhD candidate at Hahn-Schickard and at the Laboratory for MEMS Applications at IMTEK, University of Freiburg. Her research involves nucleic acid analysis with focus on isothermal amplification and digital amplification on centrifugal microfluidic cartridges. |
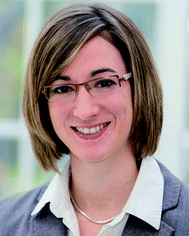 Nadine Borst | Dr Nadine Borst studied biochemistry at the University of Regensburg and completed her PhD at the Technical University of Munich in cooperation with the Fraunhofer Institute for Interfacial Engineering and Biotechnology. There, she worked in the field of enzyme engineering and assay development. In 2016, Nadine Borst joined Hahn-Schickard and since February 2017 she is heading the Nucleic Acid Analysis group. Her current research is focused on real-time and digital assays, isothermal amplification and single cell analysis on lab-on-a-chip platforms. |
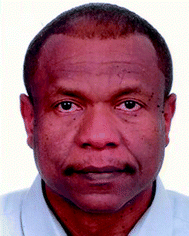 Mohammed Bakheit | Dr Mohammed Bakheit obtained his Bachelor and Master's degrees in the Faculty of Veterinary Medicine, University of Khartoum and doctoral degree in the Free University of Berlin. As a postdoc, he worked in diagnostics (both serological and molecular), first in the National Reference Center for Protozoan Diseases (NRCPD), Obihiro University focusing on methods for the diagnosis of Cryptosporidium species infection using LAMP. In his second postdoc term at Research Center Borstel he participated further in development of isothermal diagnostics for tick-borne diseases. Since 2014, he has been working for the product development team of Mast Diagnostica GmbH using LAMP. |
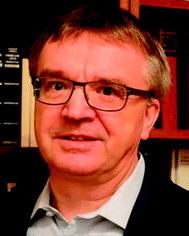 Sieghard Frischmann | Dr Sieghard Frischmann studied biology at the Friedrich-Alexander-Universität Erlangen and completed his PhD at the University of Hamburg, Universitätsklinik Eppendorf. Sieghard Frischmann joined Mast in 1992 and now he is head of product development and production at Mast. He is leading a team for the development of molecular diagnostic (based on the LAMP) and protein technologies. He works as a Medical Product Safety Manager in the terms of the EN ISO 13485 standard and the German Medical Product Law. He leads the Regulatory Affairs Team at Mast and is responsible for product registration worldwide. |
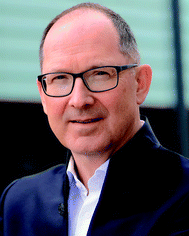 Roland Zengerle | Prof. Dr-Ing. Roland Zengerle is full professor at the Department of Microsystems Engineering at the University of Freiburg, director at the “Hahn-Schickard-Institut für Mikroanalysesysteme”. The research of Dr Zengerle is focused on Microfluidics, lab-on-a-chip as well as Electrochemical Energy Systems. Dr Zengerle co-authored more than 380 papers. From 2004 until 2014, he was member of the editorial board of the Springer Journal of Microfluidics and Nanofluidics and since 2017, he is a Member of the Advisory Board of the scientific journal “Lab on a Chip”. Since 2011, Dr Zengerle is a member of the German national academy of sciences, Leopoldina. |
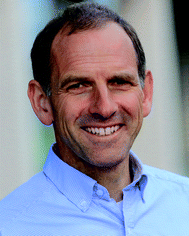 Felix von Stetten | Apl. Prof. Dr Felix von Stetten completed his PhD in Microbiology in 1999 from the Technical University of Munich. Thereafter, he joined in the diagnostic industry, where he was involved in the development of methods for sample preparation, real-time PCR and DNA-arrays. Subsequently, he joined the Laboratory for MEMS Applications at IMTEK, University of Freiburg, where he was involved in lab-on-a-chip-research and the development of molecular diagnostic techniques. In 2008, he became head of the Hahn-Schickard lab-on-a-chip division and in 2016 associate director of the Hahn-Schickard-Institut für Mikroanalysesysteme. |
1. Introduction
Since the revolutionary development of the polymerase chain reaction (PCR) in the 1980s,1,2 nucleic acid amplification tests (NAATs) have become an indispensable tool throughout the entire life sciences field, and have even grown to be the gold standard of nucleic acid analysis, especially in clinical applications,3–6 but also for food quality control7 and environmental monitoring.8 A remarkable trend, emerging between 1995 and 2005, can also be observed in the development of isothermal NAATs, which was provoked by the limitations of PCR. The complex and expensive devices required for thermal cycling and real-time detection during PCR restrict the use of this amplification method. Isothermal NAATs enable amplification reactions at constant and moderate temperatures. Simple and low-cost devices, as well as fast processing times compared to PCR, make isothermal NAATs increasingly attractive and open up new application opportunities in the field of point-of-care (POC)/point-of-need (PON) testing.9–11
The development of rolling circle amplification (RCA),12 the pioneering isothermal NAAT method, laid the cornerstone for ongoing research into numerous alternative methods enabling isothermal amplification of DNA. These include loop-mediated isothermal amplification (LAMP),13 recombinase polymerase amplification (RPA),14 nucleic acid sequence based amplification (NASBA),15 strand displacement amplification (SDA),16 helicase dependent amplification (HDA)17 and transcription-mediated amplification (TMA)18 to just name the most important ones. LAMP and RCA rank among the most frequently used methods described in literature. The percentage of publications listed in Web of Science that have LAMP in their title, illustrated in Fig. 1, demonstrates the prevalence of LAMP among isothermal NAATs (status as of 2019).
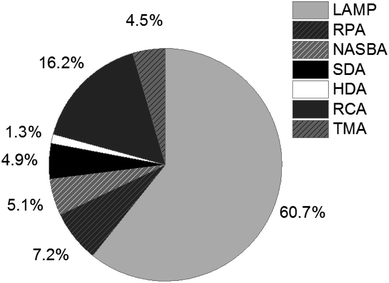 |
| Fig. 1 Percentage of publications listed in Web of Science (status as of 2019), refined search for appearance in title. | |
LAMP was first described by Notomi et al. in 200019 and was optimized with additional primers for accelerated amplification by Nagamine et al.20 It has proven itself through its high sensitivity and specificity and its tolerance to PCR-inhibiting substances.21,22 The outstanding specificity is accomplished by using four to six specific primers, which recognize six to eight different regions in the target DNA sequence. A standard method for the detection of LAMP is the measurement of the turbidity caused by precipitated magnesium pyrophosphate,23,24 as well as endpoint detection with the naked eye.25–27 Other target sequence-independent detection methods rely on gel electrophoresis,28 metal indicators for calcium,29 colorimetric LAMP,30 coffee-ring effect on colloid-crystal substrates,31 paper-based rapid detection of LAMP–magnetic bead aggregates,32 melting and annealing curve analysis,33 intercalating fluorescent dyes such as SYBR green,34–36 bioluminescence through pyrophosphate conversion37,38 or electrochemiluminescence.39,40
LAMP stands out in terms of its high analytical specificity towards the target analyte due to the utilization of six primers. However, the large number of long primers means there is a risk of primer dimers forming.41,42 Sequence-independent detection methods, listed above, indicate extended primer dimers as false positive results. In contrast, methods for sequence-specific detection excel through the use of target-specific probes or modified primers as biorecognition elements. Such sequence-specific detection enables the accurate identification of amplicons without being affected by unspecific products. Moreover, sequence-specific detection allows for differentiation between and identification of multiple targets simultaneously in one assay (one-pot multiplexing43). Appropriate selection and adaption of biorecognition elements plays an essential role in biosensing performance, especially with respect to sensitivity and specificity.44 Recent advancements have provided automated systems with integrated sample handling, sample preparation and biosensing.45 This integration of several laboratory processes into miniaturized total chemical analysis systems (μTAS) offers tremendous advantages, such as lower costs due to reduced volumes and consumption of reagents, compact system size, and high throughput.46,47 Furthermore, miniaturization reduces the complexity of the instrumentation and its operation and, combined with the isothermal reaction conditions enabled by LAMP, paves the way for on-site testing in the environmental field48,49 or close to the patient at the PON.50,51
Methods for real-time monitoring and endpoint detection of LAMP were summarized in a brief review by Zhang et al.52 These methods comprise diverse sequence-independent and sequence-specific methods. Furthermore, Mayboroda et al.43 reviewed multiplex methods for isothermal amplification in general. As a completion of the previous work and in consideration of the novel methods published after 2014, we provide the first comprehensive, systematic classification and critical analysis of such methods. In this review, we place particular focus on methods for sequence-specific detection. First, we give a short introduction to the principle of a LAMP reaction. The terms used throughout this review are defined in Table 1. In chapter 3, we classify the sequence-specific detection methods for LAMP according to the transducing elements and sensing techniques they use. We define the assessment criteria which we use to evaluate the different methods at the beginning of chapter 4. The main section of chapter 4 gives a detailed description and illustration of the individual methods, and related instrumentation is discussed in chapter 5. We compare the different methods according to our assessment criteria and their related instrumentation in a general discussion in chapter 6, and provide a summarizing overview in Table 2.
Table 1 Definitions. Terms used throughout the review are defined
Term |
Definition |
Analytical sensitivity |
The analytical sensitivity of an NAAT describes the minimum number of target copies in a sample that can be measured accurately.53 It is expressed as the limit of detection (LOD), describing the number of copies which can be detected with a probability of 95% |
Analytical specificity |
The analytical specificity of an NAAT describes the probability of detecting the correct target sequence rather than non-specific targets53 |
Biorecognition element |
Element in a biosensor which specifically interacts with the target analyte |
Biorecognition event |
Interaction between biorecognition element and target analyte |
Biosensor |
“A device that uses specific biochemical reactions mediated by isolated enzymes, immunosystems, tissues, organelles or whole cells to detect chemical compounds usually by electrical, thermal or optical signals”. (B. Nagel, H. Dellweg and L. M. Gierasch 1992)54,55 |
Diagnostic sensitivity |
This is also referred to as clinical sensitivity, and describes the percentage of positive test results which are correctly identified as positive53 |
Diagnostic specificity |
This is also referred to as clinical specificity and describes the percentage of negative test results which are correctly identified as negative53 |
FRET |
Förster resonance energy transfer. The energy of an excited fluorophore (donor) is transferred radiation-free to a second light-sensitive molecule (acceptor), also called a quencher |
Heterogeneous detection |
Planar sensor surfaces, connected to the transducing element, are used for heterogeneous detection. During signal detection, biorecognition elements capturing the target or a mediator, are in close proximity or linked to the sensing surface |
Homogeneous detection |
Signals are generated within and detected from the entire volume of the reaction mix. During signal transduction, biorecognition elements are homogeneously distributed in the reaction volume, either dissolved in solution or immobilized on suspended particles |
Imprecision |
Describes the repeatability (intraassay variance) and reproducibility (interassay variance) of an NAAT53 |
Method-tailored demonstrators |
Custom-made instrumentation, comprising devices and/or disposables, which was developed for a special method and application |
Miniaturized total chemical analysis systems (μTAS) |
μTAS are devices which provide automated sample handling. All steps for the analysis of a sample are included (sample preparation, (bio)chemical reaction and detection) |
One-pot multiplexing |
Multiple target analytes are parallelly amplified in parallel in a single reaction, comprising multiple primer sets, and detected simultaneously43 |
Open platforms |
Commercially available instrumentation, which can beis adaptative to different protocols and reagents. The instrumentation comprises devices and related disposable cartridges, such as chips or disks |
Signal transduction |
The process of converting a biorecognition event into an electrical signal with a transducing element |
Transducing element |
Element in a biosensor, which converts the biorecognition event into an electrical measurable electrical signal |
TRL |
Technological readiness level. This scale indicates the development status of a technology |
2. LAMP mechanism
The principle of LAMP is depicted in Fig. 2. The optimum temperature range of LAMP is between 60–65 °C. LAMP utilizes two outer primers (forward outer primer, F3, and backward outer primer, B3), two inner primers (forward inner primer, FIP, and backward inner primer, BIP) and a DNA polymerase with strand-displacement activity.19 LAMP can be accelerated by a factor of two by using an additional pair of primers, the loop primers (forward loop primer, LF, and backward loop primer, LB).20 Initially, the inner primer FIP, which contains two target sequences specific to two different regions in the template DNA, hybridizes to the target DNA and starts complementary strand synthesis (A). The outer primer F3 starts strand displacement of the elongated FIP primer, releasing single stranded (ss) DNA that serves as a template for the backward primers (B). The BIP primer starts strand synthesis at the ssDNA and is subsequently displaced by the B3 primer (C). Both the 3′ and the 5′ end are complementary to sequences further inwards, enabling the formation of a stem-loop DNA structure (C and D). The stem-loop structure is the starting point for exponential amplification. Self-priming and the elongation of its 3′ end (F1) induces displacement of the 5′ end (B1c), subsequent unfolding of the hairpin structure, and backfolding of the newly synthesized strand. Repetition of the self-priming pathway generates long amplicons with cauliflower-like structures. Additionally, inner and outer primers hybridize to the loop structures and initialize strand synthesis and subsequent displacement. Loop primers, annealing to the loops in the stem-loop structure (D), further accelerate LAMP.
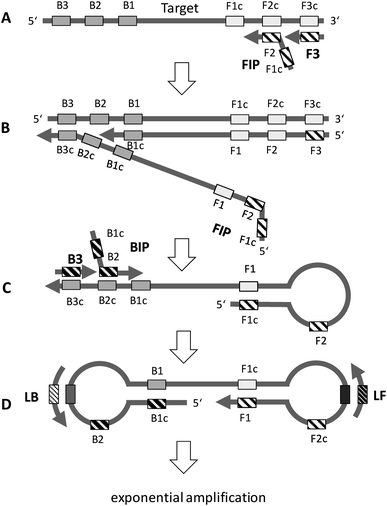 |
| Fig. 2 General principle of LAMP. See text for explanation (redrawn according to ref. 19 and 20). | |
3. Classification of detection methods
We have divided the methods used for sequence-specific detection of LAMP into groups and sub-groups according to the techniques used for signal detection. For a visual illustration of this classification, we provide a graphical overview of all the methods discussed within this review (Fig. 3). The methods are classified into homogeneous and heterogeneous detection (Fig. 3, black boxes). We further differentiate between particle-free and particle-based methods (Fig. 3, dark grey boxes) and classify the methods according to the transducing elements (Fig. 3, grey boxes) and sensing techniques (Fig. 3, light grey boxes) used. This systematic scheme is applicable to real-time as well as to endpoint detection of LAMP.
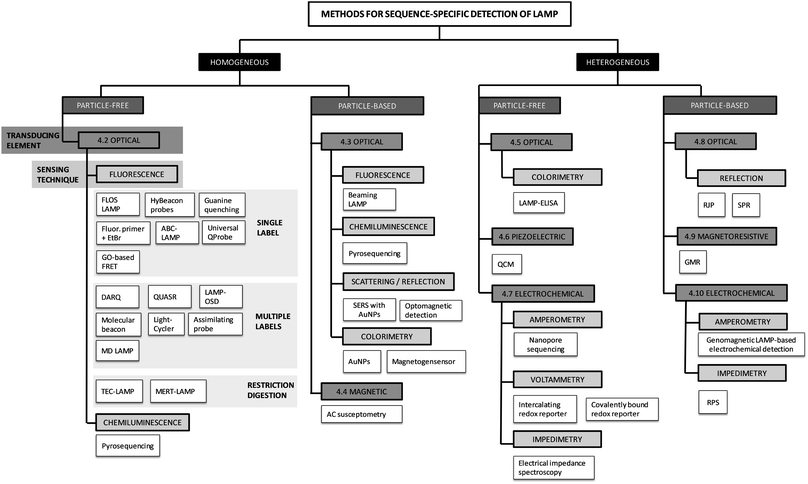 |
| Fig. 3 Overview and grouping of the methods for sequence-specific detection of LAMP. The abbreviations used herein are explained in the following: fluorescence of loop primer upon self-dequenching (FLOS), alternately binding quenching probe competitive LAMP (ABC-LAMP), quenching probe (QProbe), graphene oxide (GO), detection of amplification by release of quenching (DARQ), quenching of unincorporated amplification signal reporters (QUASR), one-step strand displacement (OSD), mediator displacement (MD), multiple endonuclease restriction real-time (MERT), Tth endonuclease cleavage (TEC), surface-enhanced Raman spectroscopy (SERS), gold nanoparticles (AuNPs), enzyme-linked immunosorbent assay (ELISA), quartz crystal microbalance (QCM), retroreflective Janus particle (RJP), surface plasmon resonance (SPR), giant magnetoresistive (GMR) measurement, resistive pulse sensing (RPS). | |
The major divide is into homogeneous and heterogeneous methods. Homogeneous detection methods rely on signals generated within and detected from the entire volume of the reaction mix. During signal transduction (see definitions), biorecognition elements (see definitions) are homogeneously distributed in the reaction volume, either dissolved in solution (particle-free detection) or immobilized on suspended particles (particle-based detection). In contrast, heterogeneous detection methods make use of planar sensor surfaces connected to the transducing element. For signal detection, biorecognition elements which capture the target analyte are in close proximity or linked to the sensing surface. For the next two levels of the systematic classification of the methods, we rely on the differentiation of the signal transduction and sensing techniques. Signal transduction, the conversion of the biorecognition event into a measurable signal, can be classified according to the transducing element (Fig. 3, grey boxes): optical, piezoelectric, magnetic, magnetoresistive or electrochemical. We further divided the methods using each type of transducer into sub-groups depending on the sensing technique used (Fig. 3, light grey boxes).
4. Methods for sequence-specific detection of LAMP
4.1. Assessment criteria
In this review we assess 34 different methods for sequence-specific detection of LAMP. In order to compare these methods, we define assessment criteria, listed in Table 2. Data that is available and published for each method is indicated with an “X”. The first criterion is the analytical performance. This was assessed according to the MIQE guidelines,53 which define the following criteria: analytical sensitivity, analytical specificity and imprecision (accuracy, repeatability and reproducibility). The second criterion is the ability to cope with complex sample material. To assess the second criterion, we evaluated published clinical studies reporting on the diagnostic sensitivity and diagnostic specificity, or demonstrating proof of concept with real sample material. Organisms and target genes utilized to assess the analytical performance or the diagnostic sensitivity and specificity are listed in Table 3. The third, fourth and fifth assessment criteria were the capability regarding multiplexing, quantification and detection by naked eye. The final criterion was the instrumental requirements. Method-tailored and open platforms that are used to apply a certain method are considered separately (platform section in Table 2) and are evaluated in chapters 5 and 6.4.
Table 2 Assessmenta of the methods for sequence-specific detection of LAMP according to analytical performance, handling of complex sample material and the capability of multiplexing and quantification. Instrumental platforms for the implementation of the methods is listed in a separate column and evaluated according to compatible methods, technological readiness level (TRL), portability, required cartridges, reusability and complexityb of practical operation. Further, we differentiate between open (O) and method-tailored (M) platforms
Methods for sequence-specific detection of LAMP |
Analytical performance |
Complex sample material |
References validation |
Multiplexing |
Quantification |
Detection by naked eye |
Instrumental platform |
Compatible method(s) |
TRL |
Open (O)/method-tailored (M) |
Portable devices |
Cartridge |
Reusable sensor/cartridges |
Complexity |
Sensitivity |
Specificity |
Imprecision |
Proof of concept |
Diag. sensitivity |
Diag. specificity |
Reagents preparation |
Operational steps |
Training, expertise |
A “X” indicates available data, published in literature. Brackets illustrate, that quantification and/or multiplexing is possible and has been shown for similar sensing techniques.
The complexity of the practical operation on the platform is rated by score factors. Score factor 1 indicates a low level of complexity and score factor 3 a high level of complexity.
|
4.2.1. FLOS LAMP & 4.2.2. HyBeacon probes56–62 |
X |
|
|
X |
X |
X |
59 and 61
|
X |
X |
X |
Standard isothermal amplification device with integrated fluorescence reader |
4.2.1.–4.2.16. |
9 |
O |
X |
|
|
1 |
1 |
1 |
Visual detection by adding polyethylenimine (PEI)60,62 |
4.2.3. Guanine quenching63 |
X |
|
|
X |
X |
X |
63
|
(X) |
X |
|
Standard isothermal amplification device with integrated fluorescence reader (see Table 2, row 4.2.1., instrumental platform) |
4.2.4. ABC-LAMP64 |
|
|
|
|
|
|
|
(X) |
X |
|
4.2.5. Fluoro-primer with EtBr65 |
X |
|
|
|
|
|
65
|
X |
X |
|
4.2.6. Universal QProbe66 |
X |
|
|
|
|
|
66
|
(X) |
(X) |
|
4.2.7. GO-based FRET67 |
X |
X |
|
|
|
|
67
|
(X) |
X |
|
4.2.8. DARQ27,68–70,72 |
X |
X |
|
X |
X |
X |
69
|
X |
X |
|
4.2.9. QUASR73–82 |
X |
X |
|
|
|
|
74–76
|
X |
|
X |
Custom-made portable “LAMP box” with smartphone readout74,75 |
4.2.1.–4.2.16. |
4 |
M |
X |
|
|
1 |
1 |
1 |
Custom-made centrifugal microfluidic platform for the orthogonal detection of proteins and nucleic acids82 |
4.2.1.–4.2.16. |
4 |
M |
X |
X |
|
1 |
1 |
1 |
4.2.10. LAMP-OSD83–86 |
X |
X |
|
X |
X |
X |
86
|
X |
X |
|
Custom-made filter-based sample-to-answer platform by visual endpoint detection with smartphones85,86 |
4.2.1.–4.2.16. |
4 |
M |
X |
|
|
1 |
2 |
2 |
4.2.11. Molecular beacon87–91 |
X |
X |
|
X |
X |
X |
87
|
X |
X |
|
Custom-made digital microfluidic (DMF) system with readout under a fluorescence microscope89 |
4.2.1.–4.2.16. |
4 |
M |
|
X |
|
1 |
2 |
2 |
4.2.12. LightCycler94,95 |
X |
X |
|
X |
|
|
94 and 95
|
(X) |
X |
|
Standard isothermal amplification device with integrated fluorescence reader (see Table 2, row 4.2.1., instrumental platform) |
4.2.13. Assimilating probe96–100 |
X |
|
|
|
|
|
97 and 98
|
X |
X |
|
Custom-made power-free device for incubation at LAMP conditions combined with fluorometer97,98 |
4.2.1.–4.2.16. |
4 |
M |
X |
|
|
1 |
1 |
1 |
Custom-made small handheld device for incubation and detection99 |
4.2.1.–4.2.16. |
4 |
M |
X |
|
|
1 |
1 |
1 |
4.2.14. MD LAMP101,102 |
X |
X |
X |
X |
X |
X |
101 and 102
|
X |
X |
|
Standard isothermal amplification device with integrated fluorescence reader (see Table 2, row 4.2.1., instrumental platform) |
4.2.15. MERT-LAMP103,104 |
X |
X |
|
X |
|
|
103 and 104
|
X |
X |
X |
4.2.16. TEC-LAMP105 |
X |
X |
|
X |
X |
X |
105
|
X |
X |
|
4.2.17. Pyrosequencing106 |
|
|
|
|
|
|
|
X |
|
|
Pyrosequencer (Hitachi, Ltd., Central Research Laboratory, Japan)106 |
4.2.17. |
9 |
O |
X |
X |
|
1 |
3 |
3 |
4.3.1. BEAMing LAMP107 |
X |
|
X |
X |
|
|
107
|
(X) |
X |
|
Emulsification in a 48-well TissueLyser, Flow cytometry107 |
4.3.1. |
9 |
O |
|
|
|
2 |
3 |
3 |
4.3.2. Surface enhanced Raman spectroscopy (SERS) with AuNPs111 |
X |
X |
|
X |
|
|
111
|
|
X |
|
Confocal micro-Raman spectroscopic system equipped with a Leica microscope111 |
4.3.2. |
9 |
O |
|
|
|
2 |
3 |
3 |
4.3.3. Optomagnetic detection112,113 |
X |
|
|
X |
|
|
113
|
|
X |
|
Custom made chip and measuring station for optical detection112 |
4.3.3. |
4 |
M |
|
X |
|
2 |
1 |
2 |
4.3.4. AuNPs114–124 |
X |
|
|
X |
X |
X |
114
|
|
|
X |
Visual detection, in tube assay |
4.3.5. Magnetogenosensor148 |
X |
X |
|
|
|
|
148
|
|
|
X |
Visual detection, lateral flow dipsticks 4.3.4.1. |
4.4.1. AC susceptometry150 |
X |
X |
|
|
|
|
150
|
|
X |
|
AC susceptometer150 |
4.4.1. |
9 |
O |
X |
|
|
2 |
2 |
2 |
4.5.1. LAMP-ELISA151,152 |
X |
X |
|
X |
|
|
151 and 152
|
|
X |
|
Microtiter plate reader152 |
4.5.1. |
9 |
O |
X |
|
|
1 |
3 |
3 |
4.6.1. Quartz crystal microbalance (QCM)153 |
X |
|
|
X |
X |
X |
153
|
|
X |
|
Commercially available quartz crystals enclosed by electrodes and custom-made QCM system for LAMP and readout153 |
4.6.1. |
4 |
M/O |
X |
|
X |
1 |
2 |
2 |
4.7.1. Nanopore sequencing154–157 |
X |
|
|
X |
X |
|
154–157
|
X |
|
|
(Minion) nanopore sequencer154–157 |
4.7.1. |
9 |
O |
X |
X |
|
1 |
3 |
3 |
4.7.2. Covalently bound redox reporter158 |
X |
|
|
X |
|
|
158
|
X |
(X) |
|
Custom-made microfluidic chip and a potentiostat for square-wave voltammetry158 |
4.7.2. and 4.7.3. |
4 |
M/O |
X |
X |
X |
2 |
1 |
2 |
4.7.3. Intercalating redox reporter159,160,162 |
X |
|
|
X |
|
|
159, 160 and 162
|
X |
(X) |
|
Custom-made microfluidic chip and Genelyzer™ (Toshiba) for linear sweep voltammetry159,160 |
4.7.2. and 4.7.3. |
4 |
M/O |
X |
X |
|
2 |
2 |
2 |
4.7.4. Electrical impedance spectroscopy163 |
|
|
|
X |
X |
X |
163
|
(X) |
X |
|
Custom-made measuring station for electrical impedance spectroscopy163 |
4.7.4. |
4 |
M |
X |
X |
|
2 |
2 |
2 |
4.8.1. Retroreflective Janus particle (RJP)164,165 |
X |
|
|
|
|
|
164
|
|
X |
|
Commercially available RJP-quantifying chips (from AMED, Seoul, Korea) and custom-made optical detection system164,165 |
4.8.1. |
4 |
M/O |
X |
X |
|
3 |
2 |
2 |
4.8.2. Surface plasmon resonance (SPR)166–169 |
X |
|
|
X |
X |
X |
166
|
X |
X |
|
Commercially available Au sensor chips (from Ssens, Netherland) and custom-made optical detection system166 |
4.8.2. |
4 |
M/O |
X |
X |
X |
3 |
2 |
2 |
4.9.1. Giant magnetoresistive (GMR) measurement170 |
X |
|
|
|
|
|
170
|
|
X |
|
Custom made GMR detecting system, custom made microfluidic chips170 |
4.9.1. |
4 |
M |
X |
X |
X |
3 |
2 |
2 |
4.10.1. Genomagnetic LAMP-based electrochemical detection171,172 |
X |
X |
|
X |
|
|
171 and 172
|
|
X |
|
Commercially available electrochemical chips, magnetic support beneath the chip and a multi potentiostat/galvanostat (all from DropSens, Spain)171,172 |
4.10.1. |
9 |
O |
X |
X |
|
2 |
3 |
3 |
4.10.2. Resistive pulse sensing (RPS)173 |
X |
|
|
|
|
|
173
|
|
X |
|
Nanopore sensor (qNano, IZON Science, Christchurch, New Zealand)173 |
4.10.2. |
9 |
O |
|
|
X |
2 |
2 |
2 |
Table 3 List of referenced organisms and target genes (alphabetical order)
Aedes aegypti (Wolbachia infection)85,86 |
Ammonia-oxidizing enzyme in environmental bacteria64 |
Avian reovirus70 |
Bacteriophage MS2 73 |
Bemisia tabaci
60
|
BRAF allele (V600E)83 |
Brucella
72
|
Caenorhabditis elegans
68
|
Chikungunya virus73 |
Chlamydia trachomatis
61
|
Dengue virus87,112,156 |
Diarrheal disease82 |
Escherichia coli
68
|
Fomitiporia torreyae
59
|
Fulviformes umbrinellus
59
|
Fusarium oxysporum f. sp. lycopersici (point mutations in xylem 3 (SIX3) gene)66 |
Genetically modified maize77–81 |
Haemophilus ducreyi
101
|
Haemophilus influenzae
105
|
hBRCA1 68 |
HeLa68 |
Hepatitis B and C virus62,87,107 |
Herpes simplex virus 1 (HSV1) US4 83 |
Human immunodeficiency virus87,101 |
HLA-B*15:02 allele163 |
Human papillomavirus153,171 |
Human T-lymphotropic virus 1 101 |
Influenza virus63,114 |
Lambda DNA68 |
Leptospira
148
|
Leishmania
155
|
Listeria ivanovii
103
|
Listeria monocytogenes
103
|
Methicillin-resistant Staphylococcus aureus (MRSA)71,166 |
Middle east respiratory syndrome coronaviruses84 |
Neisseria meningitide
105
|
Newcastle disease virus113 |
Panton–Valentine leukocidin toxin of MRSA173 |
Plasmodium falciparum
83,154,157 and, P. vivax, P. ovale wallikeri, P. ovale curtisi, P. knowlesi and P. malariae154 |
Pseudomonas aeruginosa
125
|
Ralstonia solanacearum
96
|
Respiratory syncytial virus63 |
Rheumatoid arthritis (SNPs associated with)159,160 |
Salmonella
69,111,151,152,158,164
|
Shiga toxigenic Escherichia coli65 |
Streptococcus pneumoniae
105
|
Treponema pallidum
101,106
|
Trialeurodes vaporariorum
60
|
Trypanosoma brucei
89
|
Varicella-zoster virus58 |
Vibrio cholera
88
|
Vibrio parahaemolyticus
104
|
Vibrio vulnificus
104
|
Vitamin K epoxide reductase 1 (SNP)61 |
West Nile virus73,87 |
White spot syndrome virus67,94 |
Yersinia enterocolitica
162
|
Zika virus74,150 |
β2-Tubulin gene region of causal pathogens of Fusarium head blight of wheat (mutant genotypes F167Y, E198Q, and F200Y)95 |
4.2. Homogeneous, particle-free detection – optical
Sections 4.2.1.–4.2.7. relate to single-modified fluorogenic primers and probes.
4.2.1. Fluorescence of loop primer upon self-dequenching (FLOS) LAMP.
An inner or loop primer is modified with a fluorophore capable of self-quenching. No further fluorogenic molecules are required, and therefore this is a non-FRET based quenching mechanism. The mechanism of the dequenching of an internally conjugated label is still unclear, but one possible approach is based on interactions with surrounding nucleobases.56,57 Initially, the fluorophore-modified primer is unbound and free in solution, and shows self-quenching. During amplification, the modified primer is incorporated into the double stranded amplicon, resulting in dequenching and consequently, increased fluorescence (Fig. 4). Analytical and clinical validation of FLOS-LAMP was conducted for the human pathogen Varicella-zoster virus by Gadkar et al.58 They used both crude sample material (direct specimen addition, no pre-extraction protocol) and extracted DNA, with the latter showing a more robust detection signal. Analytical sensitivity was 500 copies per reaction and the diagnostic sensitivity and specificity were 96.8% and 100% respectively.58 One challenge is to find fluorophores showing high self-quenching and a primer appropriate for modification with a fluorophore. Primer sequences are highly variable and can lead to high background fluorescence signals. The self-quenching method was utilized in a biplex assay for the diagnosis of Japanese pear dwarf,59 caused by the fungi Fomitiporia torreyae or Fulviformes umbrinellus. Visual detection was made possible by adding polyethylenimine (PEI), leading to the formation of an insoluble LAMP amplicon-PEI complex. The fluorescence-modified probes are incorporated into the precipitation, enabling easy detection by naked eye on a conventional UV illuminator. Visual differentiation between the two targets is possible, but only when just one of the targets is present. Furthermore, the same method was applied to the identification of three species of whitefly, Trialeurodes vaporariorum, Bemisia tabaci Middle East-Asia Minor 1 (MEAM1), and Mediterranean (MED).60 All three targets were identified with the naked eye in a multiplex assay by the three different colours of the precipitate.
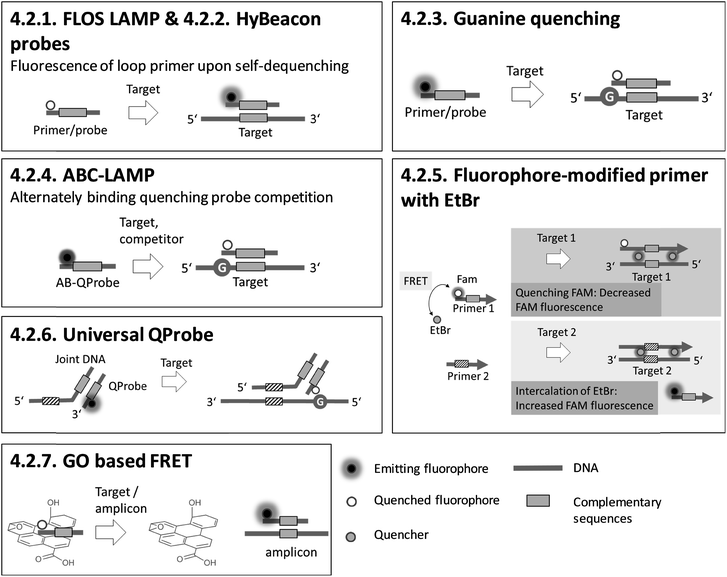 |
| Fig. 4 Schematic illustrations of 4.2.1. FLOS LAMP,58 4.2.2. HyBeacon probes,61 4.2.3. guanine quenching,63 4.2.4. ABC-LAMP,64 4.2.5. fluorophore-modified primer + EtBr,65 4.2.6. universal QProbe66 and 4.2.7. GO based FRET67 (redrawn according to the references). | |
4.2.2. HyBeacon probes.
In contrast to FLOS-LAMP, HyBeacon probes are non-extending reporter molecules and are placed between the inner primers, FIP and BIP (Fig. 4). Detection occurs after LAMP through annealing curve analysis. The described method was used for the detection of Chlamydia trachomatis and for the simultaneous detection of both alleles of the vitamin K epoxide reductase 1 (VKORC1)1639 G > A single nucleotide polymorphism (SNP) (rs9923231).61 Analytical sensitivity was determined with C. trachomatis DNA to be 18 copies per reaction. Mori et al.62 described a similar approach to the visual multiplex detection of hepatitis B and C viruses (HBV and HCV). Precipitation was generated by adding PEI after LAMP, and the LAMP result was observed by naked eye on a conventional UV illuminator. Discrimination between HBV and HCV and the observation of the simultaneous presence of both targets was possible.
4.2.3. Guanine quenching principle.
Probes or primers are modified with a fluorescent dye at a cytosine residue at the 5′ end. When the probe or primer anneals to the target sequence, fluorescence is quenched by electron transfer between the fluorescent dye and the guanine residue (Fig. 4). So-called QPrimers63 were used for the detection of influenza virus and respiratory syncytial virus in singleplex assays.63 The analytical sensitivity was between 25–250 copies per reaction, and the diagnostic sensitivity and specificity were determined to be 85.0% and 100% respectively. One big drawback is that signal generation depends heavily on the target sequence. Fluorophores must be attached to a cytosine to enable efficient quenching in the target–probe-complex. Furthermore, the signal of a free probe could be influenced by there being any guanine bases in close proximity.
4.2.4. Alternately binding quenching probe competitive LAMP (ABC-LAMP).
ABC-LAMP, mainly developed for the quantification of DNA, also makes use of guanine quenching, and is further based on a competing reaction.64 The LAMP mix comprises two targets, the amplicon of interest and an internal amplification standard (competitor). Both targets include a sequence in the loop region that is complementary to the alternately binding quenching probe (AB-QProbe). Binding of the probe to the target amplicon of interest attenuates the fluorescence intensity through guanine quenching. In contrast, binding to the competitor maintains the fluorescence of the AB-QProbe label because the guanine residues in the competitor have been replaced with cytosine (Fig. 4). The ratio of LAMP products from the target to those from the competitor can be calculated from the fluorescence intensity. ABC-LAMP was characterized for a model target, amoA, which encodes the ammonia-oxidizing enzyme in environmental bacteria. Analytical parameters were not assessed, but accurate quantification was demonstrated. The advantage of ABC-LAMP for the quantification of target DNA is that any inhibitors present in the LAMP mix affect amplifications of the real target and of the competitor equally. The competitor serves as an internal standard and enables quantification from measurements of the fluorescence at the beginning and the end of the amplification process. No real-time monitoring is required. One disadvantage is that the amplification efficiencies of the target and the competitor must be balanced. Another is that a standard curve is needed.64
4.2.5. Fluorophore-modified primer with ethidium bromide (EtBr).
Kouguchi et al.65 presented a method linking fluorescence-modified primers with an intercalating dye for the identification of two different Shiga toxin genes in Shiga toxigenic Escherichia coli isolates. A BIP primer is modified with FAM, and an intercalating acceptor molecule (EtBr) is used to weaken the fluorescence intensity through FRET. The method has been developed for biplex detection in particular. The primer set specific to target 1 includes a FAM-modified primer, whereas the primer set for target 2 is label-free. Initially, FAM fluorescence is weak due to FRET with EtBr. During amplification of target 2, EtBr intercalates into double stranded amplicons. The amplicons do not contain FAM modifications as only the second primer set is involved. The consumption of EtBr lowers the concentration of free EtBr in solution and consequently reduces FRET, leading to increased FAM-fluorescence. In contrast, the amplification of target 1 generates amplicons modified with FAM fluorophores. Intercalation of EtBr leads to close proximity between FAM and EtBr, resulting in strong quenching of fluorescence compared to the initial conditions (Fig. 4). Analytical sensitivity for the detection of Shiga toxin genes in Shiga toxigenic E. coli isolates was determined to be 100 colony forming units per reaction. One drawback of this approach is that multiplexing is limited to two targets. Another is the use of EtBr, which is known to decrease the specificity of biplex LAMP due to its interaction with unspecific by-products. A third drawback is the difficulty distinguishing between the targets when they are both present initially due to complicated fluorescence plots. EtBr may also decrease the efficiency of amplification. Furthermore, EtBr is considered a strong mutagen, and is potentially carcinogenic and teratogenic. However, the modification of primers for signal generation is simple.
4.2.6. Universal quenching probe (QProbe).
A universal QProbe66 is used together with joint DNA. The single stranded joint DNA contains two regions. One of these is complementary to the QProbe and the other is complementary to the target sequence. The QProbe is a universal probe labelled with a fluorophore at its 3′ end. The joint DNA operates as a coupling between the QProbe and the target by hybridizing with the template during amplification. Electron transfer between the fluorophore and the guanine residue in the target sequence leads to fluorescence quenching (Fig. 4). SNPs can be detected by melting curve analysis following LAMP reaction. QProbes were used for the detection of point mutations in the xylem 3 (SIX3) gene of the tomato wilt pathogen Fusarium oxysporum f. sp. lycopersici. Analytical sensitivity was 30 pg per reaction. An advantage of this method is that the nucleotide sequence of the QProbe can be fixed and does not contain target-specific regions. Still, this is a target sequence-dependent mechanism because the fluorophore attached to the 3′ end of the universal sequence is quenched by a guanine in the target strand when it is in close proximity. This works only if guanine bases are present at this position in the target sequence, and therefore it is only a semi-universal method.
4.2.7. Graphene oxide (GO) based FRET.
Fluorescence quenching via FRET can be achieved by mixing with fluorogenic probes, as energy can be transferred between the fluorophore and GO. Single stranded probes are adsorbed onto the GO surface via π–π interactions, leading to fluorescence quenching due to the close proximity between the fluorophore and GO. After LAMP, the fluorogenic probes hybridize with the amplicon, and thus nucleotide bases are not accessible to the GO. Consequently, a fluorescence signal is recovered. LAMP product is added to a probe–GO mixture, and in the case of a positive LAMP reaction probes bind to amplicons, leading to signal recovery within 10 minutes (Fig. 4). FRET between the fluorophore and GO was first applied by Waiwijit et al. to detect white spot syndrome virus.67 Analytical sensitivity was 10 plasmid DNA copies per reaction and 0.6 fg infected shrimp total DNA. High analytical specificity (100%) was confirmed by testing against common shrimp viruses. GO was synthesized by the authors and not obtained commercially. A further weakness is that this method depends on opening LAMP reaction vials, which risks contamination with amplicons. However, high fluorescence quenching due to efficient FRET between GO and fluorophores is an advantage of this detection method.
Sections 4.2.8.–4.2.14. relate to multiple-modified fluorogenic primers and probes.
4.2.8. Detection of amplification by release of quenching (DARQ).
The DARQ probe consists of a modified FIP primer and an additional oligonucleotide (Fd) which is complementary to the 5′ overhang of the FIP primer. The FIP primer is modified with a quencher or a fluorophore at the 5′ end and anneals to the Fd, which is also modified with a fluorophore or quencher at the 3′ end. The new synthesized strand hybridizes to the Fd probe and serves as a template for subsequent primer elongation. Primer extension at the 3′ end of the newly generated strand leads to the displacement of the Fd and a release of quenching, resulting in a gain in the fluorescence signal (Fig. 5). DARQ detection was first described by Tanner et al.68 for the singleplex and quadruplex detection of model systems comprising E. coli, Caenorhabditis elegans, HeLa, lambda and hBRCA1 DNA. However, there was no consistent curve shape and the data showed deviations from a sigmoidal shape. Furthermore, early non-template amplification was observed in multiplex assays, resulting in a decreased specificity.27,68 DARQ LAMP was also applied to detect Salmonella,69 avian reovirus,70 methicillin-resistant Staphylococcus aureus (MRSA)71 and Brucella.72 Analytical sensitivity for Salmonella DARQ LAMP was 10 copies per reaction. High specificity against non-Salmonella strains was shown. Diagnostic sensitivity and specificity were 100% and 99.4% respectively.69
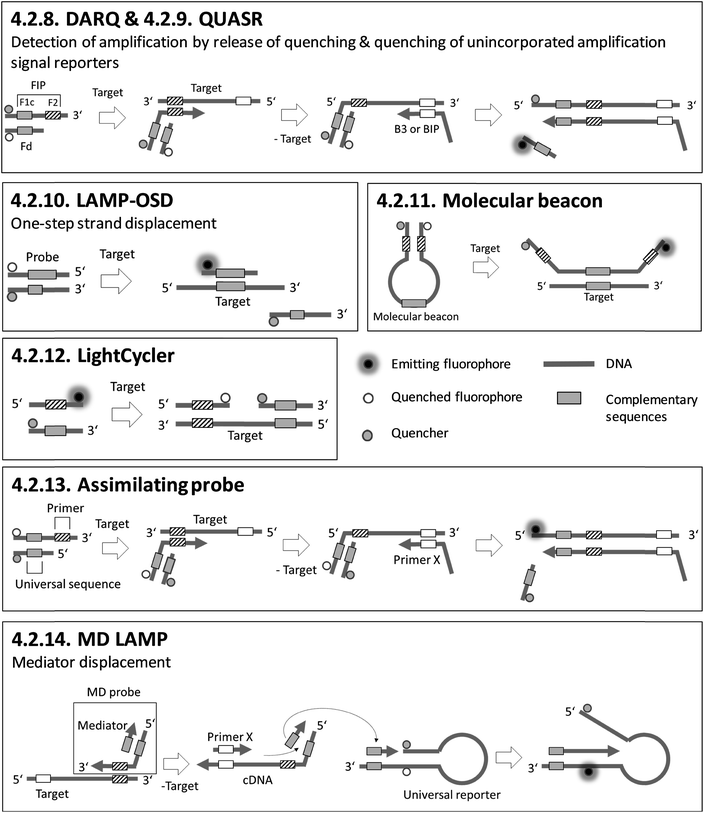 |
| Fig. 5 Schematic illustrations of 4.2.8. DARQ LAMP68 & 4.2.9. QUASR LAMP,73 4.2.10. LAMP-OSD,83 4.2.11. molecular beacon,88 4.2.12. LightCycler,94 4.2.13. assimilating probe96 and 4.2.14. MD LAMP101 (redrawn according to the references). | |
4.2.9. Quenching of unincorporated amplification signal reporters (QUASR).
QUASR is specifically designed for endpoint detection of LAMP. Similar to DARQ, the QUASR method, presented by Ball et al.,73 uses a primer modified with a fluorophore at its 5′ end and a short quencher probe of about 7–13 bases modified at the 3′ end. The quencher probe is complementary to the 5′ end of the modified primer. The melting temperature of the dimer, consisting of the modified primer and the quencher probe, must be below the temperature of LAMP to guarantee the presence of ssDNA during LAMP. After LAMP, the reaction is cooled down to room temperature, allowing hybridization of the free primer and the quencher probe. After the amplification of the target DNA, primers modified with fluorophores are incorporated into the amplicons and are inaccessible to the quencher probe (Fig. 5). Consequently, the fluorescence signal remains bright and can be observed visually with a UV lamp. Bacteriophage MS2 was used as a model RNA virus, and biplex detection of West Nile virus (WNV) and chikungunya virus RNA were also demonstrated.73 Technical applications of QUASR on a chip and with smartphone readout are described elsewhere.74–76 The analytical sensitivity for detection of cell-cultured zika virus by QUASR RT-LAMP was 44 copies per reaction. Furthermore, the assay showed high analytical specificity when tested against other flaviviruses and alphaviruses. Similar approaches allowing visual endpoint detection through the addition of quencher oligonucleotides after amplification were demonstrated for the human immunodeficiency virus (HIV) and genetically modified maize.77–81 Detection of diarrheal disease by QUASR was performed on a centrifugal microfluidic platform.82 QUASR is limited to biplex LAMP if visual detection is performed due to overlapping of the colours. Furthermore, no precise quantification is possible.
4.2.10. Toehold-mediated strand exchange reaction, termed one-step strand displacement (OSD).
The OSD probe comprises two complementary probes: the target-binding probe contains a fluorophore at its 5′ or 3′ end, and the second oligonucleotide is modified with a quencher at its 3′ or 5′ end. The target-binding probe is between 10 and 11 bases longer than the second oligonucleotide, resulting in a higher binding enthalpy between the target-binding probe and the amplicon. According to the principle of Le Chatelier, toehold-mediated strand exchange reaction occurs during the amplification of the target DNA. Consequently, separation of the fluorophore and quencher induces an increase in fluorescence intensity (Fig. 5). The probes are sensitive towards single mismatches in target amplicons due to their thermodynamic properties, and therefore OSD probes can distinguish SNPs in LAMP amplicons with a high signal-to-noise ratio. LAMP-OSD was applied for SNP detection in a mutant BRAF allele (V600E) in the presence of the wild-type gene. The mutant allele (V600E) was detected with an analytical sensitivity of 20 copies per reaction, and further, in the presence of 20 times as many wild-type gene copies, could deliver a yes/no result.83 The method was also applied for the detection of Middle East respiratory syndrome coronaviruses,84 as well as the detection of human faecal contamination of water on a portable platform by visual endpoint detection with unmodified smartphones, LEDs and filters, including the provision of lyophilized reagents and chemical heating.85 LAMP-OSD was also used to monitor Wolbachia infection frequencies in Aedes aegypti mosquito populations on a one-pot sample-to-answer nucleic acid diagnostic platform with visual endpoint detection.86 Blind field tests with field-caught mosquitoes revealed a diagnostic sensitivity and specificity for identifying Ae. aegypti of 98% and 97% respectively. Biplex detection was shown for the Plasmodium falciparum cytB gene and the genomic locus detection of herpes simplex virus 1 (HSV1) US4.83 A drawback is that LAMP-OSD requires elaborate assay design, as binding enthalpies must be calculated and balanced to guarantee successful exchange reactions.
4.2.11. Molecular beacon.
The target-specific dual labelled probe is modified with a fluorophore and a quencher at each end of its strand. Its 3′ end is complementary to its 5′ end, allowing the probe to form a hairpin structure.87,88 In the absence of amplicons, the molecular beacon exists in closed loop form due to intramolecular hybridization between the 5′ and 3′ ends. Consequently, the fluorophore and quencher are in close proximity, leading to fluorescence quenching. In the presence of amplicons, the probe hybridizes with an amplicon, causing the loop to open, separating the fluorophore and quencher, and resulting in release of fluorescence (Fig. 5). Molecular beacons were used for the viral nucleic acid detection by LAMP of HIV, HBV, HCV, hepatitis E (HEV), dengue virus (DENV) and WNV.87 Analytical sensitivity was between 50–100 viral particles per reaction for HCV and HBV detection, and analytical specificity was confirmed by testing against various viral nucleic acids. Furthermore, clinical validation was conducted with nucleic acids extracted from human clinical specimens. This revealed a diagnostic sensitivity and specificity of 97% and 100% respectively. Molecular beacons were used for the detection of a part of the 1031-bp ompW gene from Vibrio cholera88 and also, on a digital microfluidic system, for the detection of the human African trypanosomiasis pathogen Trypanosoma brucei.89 The hairpins are unstable under LAMP conditions, resulting in high signal-to-noise ratios. An approach to improving the thermal stability of molecular beacons is to use locked nucleic acids to decrease the background fluorescence signal. Locked nucleic acids comprise some kind of artificial bases, which generates high melting temperatures.90 A further method to monitor LAMP with molecular beacons is based on G-quadruplex molecular beacons.91 Due to the binding of the molecular beacon to an amplicon, a spatial G-quadruplex complex is formed. G-quadruplexes consist of G-rich nucleic acids. They are formed of stacked G-tetrads92 and can associate with hemin to form DNAzymes. The catalytic effect of the G-quadruplex/hemin complex upon peroxidation reactions is exploited to induce chemiluminescence due to the oxidation of luminol.93
4.2.12. LightCycler.
LightCycler probes for LAMP were introduced by Chou et al.94 and are currently commercially available for PCR. LightCycler probes comprise an acceptor probe modified with a quencher at the 5′ end and a donor probe labelled with a fluorophore at the 3′ end. The distance between the 5′ end of the acceptor part and the 3′ end of the donor part of the target sequence is about two bases to guarantee close proximity between the fluorophore and quencher. During LAMP, amplicons are generated, providing probe-complementary sequences to which the probe system hybridizes, bringing the fluorophore and quencher into close proximity (Fig. 5). Analytical sensitivity was determined for white spot syndrome virus (WSSV) of penaeid shrimp and yielded 100 copies per reaction. Analytical specificity was confirmed by testing against host DNA and further DNA from virus pathogens found in shrimp. Clinical usefulness was demonstrated by testing genomic DNA from WSSV-infected Penaeus vannamei.94 A similar approach for endpoint detection of SNPs was presented by Komura et al. for the mutant genotypes F167Y, E198Q and F200Y in the β2-tubulin gene region of the causal pathogens of Fusarium head blight in wheat.95
4.2.13. Assimilating probe.
The assimilating probe96 is a dimer consisting of two partly complementary oligonucleotides. It is closely related to DARQ detection.68 A first oligonucleotide comprises a target-specific sequence at the 3′ end and a universal sequence carrying a fluorophore at its 5′ end. A second oligonucleotide, complementary to the universal sequence of the first oligonucleotide, is labelled with a quencher at its 5′ end. Initially, the quencher strand is annealed to the first oligonucleotide of the assimilating probe, resulting in fluorescence quenching. During LAMP, the target-specific 3′ end of the fluorogenic oligonucleotide anneals to the amplicon taking part in the amplification process. The binding and extension of the reverse primers induces the displacement of the quencher strand, resulting in a release of fluorescence (Fig. 5). It differs from DARQ detection in the universal quencher oligonucleotide, which is target-specific in DARQ detection. Assimilating probes were first presented for detection of the bacterial agricultural wilt pathogen Ralstonia solanacearum.96 The assay has also been incorporated into a portable device.97,98 This device does not require any power supply as it uses the exothermic hydration of calcium oxide for incubation at 61 °C. Assimilating probes were also used for the detection of Salmonella enterica in a handheld device, enabling real-time monitoring of LAMP with an analytical sensitivity of 15 copies per reaction.99 Samples processed from chicken rinsate showed a sensitivity of 25 CFU per assay.99 A biplex assay to sub-type specific quarantine strains of the bacterial wilt pathogen Ralstonia solanacearum is described in ref. 100. Again, this method is semi-universal, as the fluorogenic oligonucleotides contain target-specific regions. Adaption to different target sequences requires modifications of the fluorogenic oligonucleotide which may influence signal generation.
4.2.14. Mediator displacement (MD) LAMP.
MD LAMP features a MD probe and a universal reporter (UR).101 The mediator displacement probe is a bifunctional dimer comprised of two partly complementary oligonucleotides. The first oligonucleotide contains a target-specific sequence, and also a universal sequence at its 5′ end. The second oligonucleotide, called the mediator, is complementary to the universal sequence of the first oligonucleotide. The signal generating molecule, the UR, exhibits a hairpin-shaped secondary structure. It is labelled with a fluorophore and a quencher, and has a mediator-complementary part at its 3′ end. The UR is completely target-independent. Amplification of target DNA induces the displacement of the mediator from the MD probe. The displaced mediator then hybridizes with the UR and generates a fluorescence signal upon mediator elongation and the subsequent unfolding of the UR (Fig. 5). Biplex MD LAMP was demonstrated for HIV-1 and human T-lymphotropic virus 1 (HTLV-1), as well as for Treponema pallidum and Haemophilus ducreyi.101 Universal applicability of MD detection was demonstrated by utilizing the same generic mediator-reporter set for HIV-1/HTLV-1 and for T. pallidum and H. ducreyi detection. Analytical sensitivity was determined for HIV-1 RNA as 131 copies per reaction. Additionally, MD LAMP showed high analytical specificity (100%) and low inter- (34.0% for 1 × 103 copies per reaction, 0.7% for 1 × 106 copies per reaction) and intraassay variation (16.0% for 1 × 103 copies per reaction, 0.7% for 1 × 106 copies per reaction). Clinical validation of T. pallidum and H. ducreyi biplex detection with statistically valid number of patient samples yielded acceptable diagnostic sensitivities and specificities.102 Preconfigured mediators and URs can be used for various target panels. As signal generation is decoupled from target amplification, quenching and fluorescence intensity can be optimized independently from the design of the target-specific probe.
Sections 4.2.15.–4.2.17. relate to detection based on restriction enzymes.
4.2.15. Multiple endonuclease restriction real-time (MERT)-LAMP.
The signal generating unit is an inner primer with an overhang at its 5′ end. The 5′ overhang is modified with a fluorophore and a quencher, which flank an endonuclease recognition site. The modified inner primer is called the core MERT-LAMP primer.103 During LAMP, the core MERT-LAMP primer is inserted into the amplicons. Synthesis of a new strand containing the MERT-LAMP primer sequence generates a double stranded terminus. Consequently, a nicking enzyme, recognizing the endonuclease recognition site, digests the double stranded terminus. Separation of the fluorophore and quencher releases quenching (Fig. 6). One-step MERT-LAMP enables simultaneous LAMP and digestion reaction under constant conditions, allowing real-time monitoring, quantification and also visual endpoint detection via colour change. MERT-LAMP was first described by Wang et al. for biplex detection of Listeria monocytogenes and Listeria ivanovii103, revealing an analytical sensitivity of 250 fg DNA per reaction. Raw meat samples were evaluated successfully and high analytical specificity was confirmed by testing against various Listeria strains. Further, MERT-LAMP was applied for biplex detection of two marine seafood-borne pathogens Vibrio parahaemolyticus and Vibrio vulnificus.104
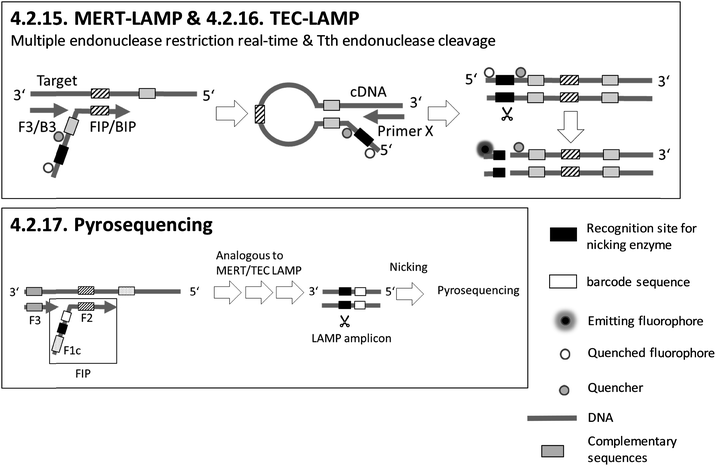 |
| Fig. 6 Schematic illustrations of 4.2.15. MERT-LAMP,103 4.2.16. TEC-LAMP105 and 4.2.17. pyrosequencing106 (redrawn according to the references). | |
4.2.16. Tth endonuclease cleavage (TEC)-LAMP.
TEC-LAMP is similar to MERT-LAMP, but instead of an endonuclease recognition site, an abasic site, cut by a Tth endonuclease IV enzyme, is incorporated (Fig. 6). The modified inner primers are called TEC primers.105 TEC-LAMP was applied for the detection of leading bacterial meningitis pathogens (Streptococcus pneumoniae, Neisseria meningitides and Haemophilus influenzae) in 3-plex LAMP and in 4-plex LAMP with an internal control. Analytical sensitivities of the above listed targets were between 17.3 and 39.5 genome copies per reaction, and high analytical specificity was shown. Clinical validation revealed a diagnostic sensitivity and specificity of 92.3% and 100% respectively. A drawback of MERT- and TEC-LAMP is that additional enzymes increase costs and also carry the risk of interactions with components in the reaction mix. Furthermore, nicking enzymes require specific conditions which must be made compatible with those of the LAMP reaction.
4.2.17. Pyrosequencing.
For pyrosequencing of amplicons generated during LAMP, a FIP primer is modified with a target-specific barcode and a recognition sequence for the nicking endonuclease (NEase) near the barcode, between the F2 and F1c regions. During LAMP, the target-specific barcode and the recognition sequence for the nicking endonuclease are incorporated into the amplicon. After LAMP, nicking of the amplicon occurs, followed by subsequent pyrosequencing (Fig. 6). Pyrosequencing in solution makes use of the nucleotide-degrading enzyme apyrase. The washing steps required in solid-phase pyrosequencing are therefore redundant. The registration of target-specific barcodes enables the identification of a large number of different targets. This method was presented by Liang et al.106 for 4-plex detection of HBV, HCV, HIV and T. pallidum. LAMP and the nicking reaction can be processed in a standard thermocycler, and pyrosequencing can be processed in a portable pyrosequencer (Hitachi, Ltd., Central Research Laboratory, Japan). A drawback is that this method, comprising LAMP, manual purification of LAMP amplicons with a PCR purification kit (about 30–45 minutes), nicking reaction (1 hour and 20 minutes) and pyrosequencing, is elaborate and requires many handling steps. Pyrosequencing is not quantitative, but it does enable a high degree of multiplexing.
4.3. Homogeneous, particle-based detection – optical
4.3.1. BEAMing LAMP.
LAMP is conducted in an emulsion on magnetic beads modified with target-complementary probes, and is subsequently measured by flow cytometry.107 Probes are immobilized on a magnetic bead surface via biotin–streptavidin interaction and bind to the FIP region of the target molecule. BIP primers free in solution are labelled with Cy5 for amplicon detection. In a first step, target molecules hybridize to probes immobilized on the bead surface. In the case of low target concentrations, the magnetic beads carry either zero or one template molecule. After the single-molecule capturing step, the beads are washed, mixed with LAMP reagents, and divided into droplets by the formation of a water-in-oil emulsion in a 48-well TissueLyser. Each aqueous droplet contains one magnetic bead carrying one or no template molecules. Droplets are incubated at LAMP conditions, and amplicons labelled with Cy5 accumulate on the bead surface. After in-droplet LAMP, the emulsion is broken by mixing with isopropyl alcohol and the beads are washed. In a final step, the beads are analyzed using flow cytometry (CytoFLEX, Beckman) (Fig. 7). By counting the number of positive beads, the initial target concentration can be determined precisely. Unlike most existing digital nucleic acid amplification platforms,108–110 BEAMing LAMP is a non-chip (cartridge-free) format. Furthermore, it allows parallel processing of multiple samples. The optimum time for on-bead LAMP is 1.5 hours, and the total turnaround time is about 2 hours. BEAMing LAMP has been demonstrated for the detection of HCV with an analytical sensitivity of 300 copies per reaction and high reproducibility (15% relative errors).107 Furthermore, clinical plasma samples were tested, revealing its potential for use in clinical applications. High-precision endpoint quantification due to digital readout makes DNA standards redundant. However, the complex workflow entails drawbacks.
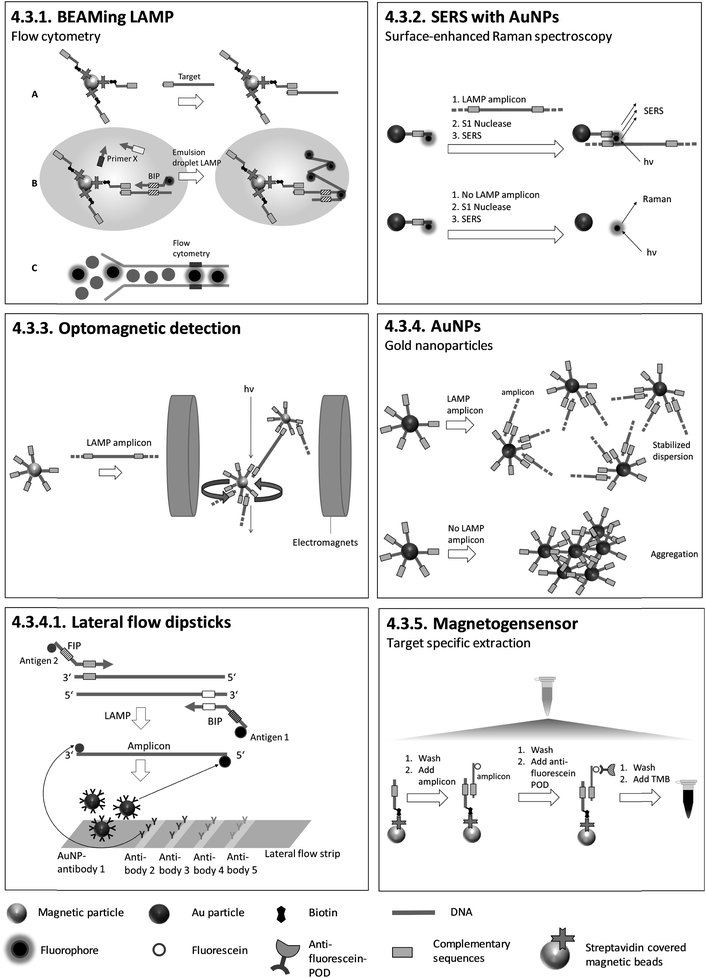 |
| Fig. 7 Schematic illustrations of 4.3.1. BEAMing LAMP,107 4.3.2. surface-enhanced Raman spectroscopy (SERS) with AuNPs,111 4.3.3. optomagnetic detection,112 4.3.4. AuNPs,115 4.3.4.1. lateral flow dipsticks125 and 4.3.5. magnetogenosensor148 (redrawn according to the references). | |
4.3.2. Surface-enhanced Raman spectroscopy (SERS) with gold nanoparticles (AuNPs).
Capture probes complementary to the LAMP amplicons and containing a Raman-active modification, e.g. Cy5 dyes are immobilized on the surface of AuNPs. The Raman-active label is in close proximity to the AuNP, causing the Raman signal intensity to increase significantly. LAMP amplicons hybridize to the capture probe, forming double stranded (ds) DNA. In the absence of the target DNA, the capture probes remain single stranded. Due to the addition of S1 nuclease, which specifically degrades ssDNA, ss capture probes are degraded into mononucleotides (dNTPs), whereas probes bound to amplicons and forming dsDNA are protected against degradation. In the absence of target DNA, Cy5 is released due to nuclease digestion, resulting in a reduction in the intensity of the Raman signal (Fig. 7). Two handling steps are needed, as digestion is conducted after LAMP. First, the LAMP (40 minutes) product is mixed with AuNPs and an S1 nuclease enzyme mix and incubated for 25 minutes. Second, the mix is washed twice and then analyzed with a confocal micro-Raman spectroscopic system, equipped with a Leica microscope. Total turnaround time is about 1 hour and 20 minutes. SERS was applied for the detection of Salmonella enterica serotype enteritidis and showed an analytical sensitivity of 66 CFU per reaction. High analytical specificity was demonstrated by testing against closely related bacterial species. Successful identification of the target in artificially spiked milk samples was also demonstrated.111 SERS spectroscopy is very sensitive and allows detection on a single molecule level.
4.3.3. Optomagnetic detection.
Two approaches for the functionalization of magnetic beads are used in literature: magnetic beads are either modified with target-specific capture probes or are coated with streptavidin and used in combination with amplicons carrying biotin due to the incorporation of biotinylated primers during LAMP. Clustering of the functionalized magnetic beads occurs in the presence of the target amplicons, as these contain many repetitions of the sequence complementary to the capture probe or many biotin modifications. Clustering leads to an increase in the hydrodynamic volume, resulting in a change in the optomagnetic spectra (Fig. 7). Optomagnetic methods rely on the modulation of the intensity of light in an external magnetic field, which is transmitted through the magnetic beads suspended in solution. Real-time monitoring was conducted in a custom-made chip processed in a specially established measuring station which was comprised of a light source, a photodetector, heaters, and poly(methyl methacrylate) rods to provide light access to the chip.112 DENV was detected in real time with an analytical sensitivity of 100 fM per reaction. Total turnaround time was only 20 minutes. The method was also applied for the endpoint detection of Newcastle disease virus113 with an analytical sensitivity of 10 aM. Clinical applicability was demonstrated by successfully analyzing viral RNA extracted from clinical specimens. A drawback is the sedimentation of magnetic beads during LAMP, which could influence the result. Furthermore, the modulation efficiency is not predictable or consistent, as the size distribution of LAMP amplicons is very broad. Another drawback is that the LAMP mix is not washed after incubation. Consequently, biotinylated primers and amplicons compete with each other in binding to the magnetic beads.
4.3.4. AuNPs.
The surfaces of AuNPs are modified with target-specific probes which bind to amplicons after LAMP. AuNPs enable the visual detection of target strands by their wine-red colour. The exact shade of red depends on particle size and the degree of aggregation. ssDNA-labelled AuNPs form larger clusters due to salt-induced self-aggregation, resulting in a blue-grey colour. Hybridization between amplicons and probes increases steric hindrance at the Au-surface, leading to repulsion between AuNPs. The AuNPs thus remain homogeneously distributed and dispersed in solution, leading to a wine-red colour (Fig. 7). In a manual step, LAMP product is mixed with AuNP solution and incubated for about 30 minutes. AuNP biosensors were applied for the visual endpoint detection of influenza viruses with an analytical sensitivity of 10–100 copies RNA per reaction and a diagnostic sensitivity and specificity of 98.3% and 100% respectively.114 AuNPs were also utilized for the detection of various pathogens,115–121 for forensic evidence,122 and for clinically relevant SNPs.123,124 In another approach, hybridization-induced aggregation due to cross linking between AuNPs and a template led to a colourless precipitation.124 This method does not require laboratory operations or expensive equipment and can be used directly at the PON. Furthermore, AuNPs are used for staining on lateral flow dipsticks. Lateral flow dipsticks find wide application in the detection of LAMP products and are therefore discussed in chapter 4.3.4.1.
4.3.4.1. Lateral flow dipsticks.
During LAMP, primers that are two-fold modified with two different antigens are incorporated into the amplicon. Antigen 1 (identical for each target) is for colour reaction and antigen 2 (individual for each target) is for capturing at the test line. Biotin in combination with streptavidin-modified AuNPs can be used instead of antigen 1. Antibodies for antigen 2 are pre-immobilized on the test lines and Au-NPs labelled with antibodies for antigen 1, for colour reaction, are pre-immobilized on the conjugate pad. After dipping the test strip into a running buffer which includes LAMP products, antibody 1-labeled AuNPs are rehydrated and transported in the running buffer. Antigen 2-tagged amplicons are trapped at specific test lines by antibodies for antigen 2, and AuNPs accumulate on the test lines due to their binding to antigen 1, which is attached to the amplicons. Positive amplification is indicated by a red band which can be observed visually (Fig. 7). The described lateral flow method was utilized by Chen et al.125 after triplex LAMP to identify three different toxin genes of Pseudomonas aeruginosa with an analytical sensitivity of 20 CFU per reaction. Similar lateral flow approaches were utilized to detect various other pathogens.126–146 Lateral flow tests have also been integrated into sample-to-answer microfluidic systems.147 Lateral flow tests are easy to handle and do not require additional equipment other than an incubator for LAMP. The strips are universally applicable as no target-specific probes are immobilized on the surface. These tests can therefore be produced on a large scale, minimizing fabrication costs. However, they are not quantitative, and the opening of LAMP reaction vials risks contamination with amplicons.
4.3.5. Magnetogenosensor.
After LAMP, fluorescein-labelled amplicons hybridize to probes immobilized on magnetic beads via biotin–streptavidin interaction. This hybridization takes about 20 minutes. Next, non-hybridized amplicons are washed away with a portable magnetic separator. Then, anti-fluorescein antibody peroxidase conjugate (POD) is added. This binds to fluorescein-labelled amplicons during the next incubation step (10 minutes). After a further washing step and the addition of 3,3′,5,5′-tetramethylbenzidine (TMB), a substrate of POD, there is a colour change from brown to blue, indicating a positive result (Fig. 7).148 Total turnaround time is about 2 hours. This method was applied for the detection of pathogenic Leptospira with an analytical sensitivity of 80 copies per reaction and 100% analytical specificity when tested against 172 bacterial strains.148 The portable magnetic sensor enables use at the PON, as no additional devices or power supply is required. Many manual steps and opening of the reaction vessels are drawbacks of this method.
4.4. Homogeneous, particle-based detection – magnetic
4.4.1. AC susceptometry.
Before LAMP, biotinylated primers are immobilized onto streptavidin-coated magnetic beads. These immobilized primers are extended during LAMP and the amplicons generated lead to an increase in the hydrodynamic volume of the magnetic beads. After incubation, the reaction vessel, containing LAMP product, is mounted in an AC susceptometer and the frequency-dependent AC susceptibility in an alternating magnetic field is measured (Fig. 8). The magnetic beads start to rotate (Brownian relaxation149) so that their magnetic moments are aligned with the direction of the applied magnetic field. The hydrodynamic volume of the magnetic beads influences the time taken for relaxation, which is reflected by the susceptibility. Time for readout is about 15 minutes. AC susceptometry was applied for the quantitative detection of synthetic Zika virus oligonucleotides with an analytical sensitivity of 1 aM.150 High specificity was demonstrated by testing against four relevant viruses. An advantage of this method is that no washing steps are required, which may allow real-time monitoring, provided that the time for readout can be reduced.
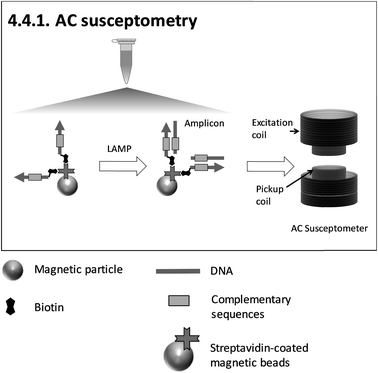 |
| Fig. 8 Schematic illustration of 4.4.1. AC susceptometry150 (redrawn according to the references). | |
4.5. Heterogeneous, particle-free detection – optical
4.5.1. LAMP detected by enzyme-linked immunosorbent assay (LAMP-ELISA).
Amplicons are tagged with digoxigenin during amplification due to the incorporation of digoxigenin-labelled nucleotides, and with biotin due to biotin-modified primers and probes. After LAMP, the amplicons are transferred to a streptavidin-coated microtiter plate well. The amplicons bind with the biotin tag to the streptavidin, which is immobilized on the surface of the well. Next, the wells are washed, POD-labelled anti-digoxigenin antibody Fab fragment is added, and the wells are incubated. During incubation, anti-digoxigenin Fab fragments bind to the digoxigenin incorporated into the amplicons. After 30 minutes of incubation, the wells are washed and an enzymatic reaction on the surface of the well is started after the addition of TMB. TMB is activated by the POD, resulting in an absorbance change. This absorbance change is detected using a standard microtiter plate reader (Fig. 9).151 LAMP-ELISA was applied for the detection of Salmonella with an analytical sensitivity of 4 CFU per reaction. The analytical specificity was evaluated with related Salmonella and non-Salmonella strains. LAMP-ELISA was also validated with DNA extracted and purified from blood samples that had been artificially contaminated with Salmonella enterica serovars Typhi and Paratyphi A.151,152 A big drawback is the elaborate handling. This could be circumvented by an automated system. A further disadvantage is the long processing time. Advantages are that it is suitable for low-resource settings, as it is nearly instrument-free, except for a water bath and a microtiter plate reader. It is also flexible, as it allows the processing of several hundred samples simultaneously.
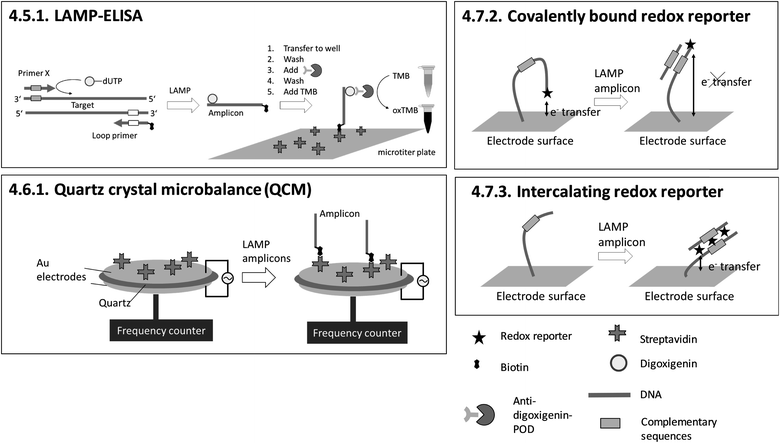 |
| Fig. 9 Schematic illustrations of 4.5.1. LAMP-ELISA,151 4.6.1. quartz crystal microbalance (QCM),153 4.7.2. covalently bound redox reporter158 and 4.7.3. intercalating redox reporter159 (redrawn according to the references). | |
4.6. Heterogeneous, particle-free detection – piezoelectric
4.6.1. Quartz crystal microbalance (QCM).
LAMP is conducted directly on an avidin-coated QCM surface. Biotinylated primers are incorporated into the amplicons and bind to the avidin-coated QCM surface, enabling real-time monitoring of LAMP. Changes in the frequency of the quartz crystal resonator due to the binding of amplicons to the sensor surface are measured by a frequency counter (Fig. 9). Further components needed are: a temperature control device to maintain LAMP conditions and a housing for the quartz crystal, enclosed by gold electrodes on both sides. For stable signal generation, a pre-incubation step is necessary, followed by the addition of polymerase using peristaltic pumps. It is a semi-automated process. The pre-incubation step and the baseline detection with HEPES buffer, which is needed to generate a stable signal before LAMP, are performed manually. The subsequent addition of polymerase to the LAMP mix and the immediate transfer into the QCM system are performed by pumps.153 QCM for the detection of LAMP was presented by Prakrankamanant et al.153 for the detection of high-risk human papillomavirus (HPV) type 58 with an analytical sensitivity of 100 copies per reaction and a diagnostic sensitivity and specificity of 100% and 90.5% respectively. Advantages are that the modified QCM surface is probe-free and universally applicable, which reduces fabrication costs and simplifies the adaption to different targets. Furthermore, quartz crystals enclosed by a gold electrode can be obtained commercially. A drawback is that the analyte is in contact with the sensor surface, which is reusable. This means there is a risk of carryover contamination.
4.7. Heterogeneous, particle-free detection – electrochemical
4.7.1. Nanopore sequencing.
After LAMP, amplicons are sequenced using a MinION™ nanopore sequencer (Oxford Nanopore Technologies, Oxford, UK) to identify different species. Nanopore sequencing was validated for the detection of Plasmodium species causing malaria 154 and showed an analytical sensitivity of 10–100 copies per reaction. It has also been applied and validated with clinical samples for the detection of cutaneous leishmaniasis 155, DENV156 and artemisinin-resistant Plasmodium falciparum.157 An advantage of this method is that it allows detailed genotyping analysis and a high degree of multiplexing. Furthermore, it requires no modification of oligonucleotides and no additional probes before LAMP. Therefore, this method can be used for any target sequence. MinION™ nanopore sequencers are portable and are not restricted to clinical or laboratory environments. Drawbacks are the opening of reaction vessels and expensive equipment, for example the consumable flow cells. Furthermore, the preparation of a DNA library for nanopore sequencing is elaborate, and sequencing is time-consuming, as the whole workflow, including a rapid sequencing protocol, takes 24 hours.
4.7.2. Covalently bound redox reporter.
The electrochemical probe comprises a linear oligonucleotide modified with a redox-reporter and is covalently attached to a gold surface which serves as an electrode. The custom-made microfluidic chip consists of an amplification chamber for LAMP and an electrochemical detection chamber connected to the LAMP chamber. After LAMP, the reaction mix is pushed into the detection chamber and onto the electrode. LAMP amplicons hybridize to the probes, which subsequently become rigid. Consequently, the movement of the redox-reporter is restricted, and thus the distance between the redox label and the gold surface increases, leading to a reduction in current. If the target is absent, the probe strand is flexible and moves freely on the gold surface. This enables the end-located redox-label to make contact with the gold surface and to be detected by square wave voltammetry (Fig. 9). This approach was applied for the biplex detection and discrimination of Salmonella enterica subsp. enterica serovars Typhimurium and Choleraesuis.158 The analytical sensitivity of the sensor for both pathogens was determined with synthetic target amplicons, revealing a limit of detection of 10 nM amplicons. Clinical utility was evaluated in a model experiment with blood from uninfected mice and mice infected with Yersinia pseudotuberculosis. Only endpoint detection was shown, but real-time monitoring, and therefore quantification, would be feasible if the assay conditions could be adapted to improve the speed of the reaction. Chips must be prepared individually for different targets as different probes have to be immobilized on the chip surface. The authors claim that the chips are reusable.
4.7.3. Intercalating redox reporter.
Unlabelled DNA probes are immobilized on a gold electrode surface and hybridize to amplicons after LAMP. An intercalating redox reporter, for example Hoechst 33258 or methylene blue, is used for signal generation. Hoechst 33258 intercalates in the dsDNA formed by amplicons and probes and immobilized on the electrode (Fig. 9). The anodic peak current derived from the redox reporter is then measured by linear sweep voltammetry. Nakamura et al. utilized the Genelyzer™ (Toshiba), a portable system for the analysis of an electrochemical DNA chip, for the detection of six SNPs associated with rheumatoid arthritis.159,160 LAMP reaction was conducted off-chip and the LAMP products were transferred manually to a custom-made chip161 for detection. First, the hybridization reaction is carried out for 20 minutes, after which the chip is washed with a buffer for 20 minutes. In the next step, phosphate buffer solution containing a redox reporter is transferred into the detection chamber and incubated for 10 minutes. Then the anodic peak current is measured. The process after hybridization (washing and incubation with a redox reporter) is completely automated. Total time to result is about two hours, as LAMP is incubated for one hour. Clinical samples were successfully analyzed to demonstrate the analysis of complex sample material.159,160 The electrochemical DNA chip comprises up to 13 types of immobilized probes for multiplex assays. A different approach, aimed at enlarging the electrochemical signal and increasing the sensitivity for DNA detection, was presented by Sun et al.162 They developed an electrochemical chip for the detection of Yersinia enterocolitica LAMP amplicons. Manual processing steps included the hybridization of target molecules, washing to remove unhybridized target ssDNA, the addition of a redox reporter, washing to remove excess redox reporter, and starting pulse voltammetry. The analytical sensitivity of the sensor was determined with LAMP amplicons and was around 1.8 pM.
4.7.4. Electrical impedance spectroscopy.
Impedance spectroscopy allows for the label- and modification-free endpoint detection of amplicons. Amplicons hybridize with probes immobilized on the surface of interdigitated electrodes. This induces a change in impedance, which is detected. The measuring station comprises a reference resistor, a function generator and a lock-in amplifier, and was assembled specially for this purpose. The total turnaround time including LAMP is 1 hour and 20 minutes.163
Electrical impedance spectroscopy was utilized for the detection of the HLA-B*15:02 allele responsible for adverse drug reactions in humans. Diagnostic sensitivity and specificity were determined with 27 crude blood samples to be 92.9% and 84.6% respectively.
The authors claim that the fabrication of the sensors is simple and low-cost, and that future miniaturization and integration into a PON device is possible.
4.8. Heterogeneous, particle-based detection – optical
4.8.1. Retroreflective Janus particle (RJP).
This retroreflection-based optical sensing approach uses RJP-quantifying chips, which can be obtained from AMED (Seoul, Korea). Probes complementary to the amplicon are immobilized on the sensing surface of the chip. After LAMP, the amplicons hybridize with surface-immobilized capture probes. Unbound LAMP products are subsequently washed away. In the next step, RJPs carrying probes are added, and these bind to the amplicons. Unbound RJPs are then washed away before the detection of retroreflection with an optical system comprising a white LED and a CMOS camera (Fig. 10). All steps after the chip is loaded with the amplicons are automated. The number of RJPs attached to the sensing surface through the loop region of the amplicons is proportional to the concentration of the amplified target DNA, and thus enables quantification of the target gene. RJPs were first used in combination with LAMP for the detection of Salmonella Typhimurium with an analytical sensitivity of 100 CFU per reaction.164 Retroreflection can be induced by various light sources, e.g. visible light, infrared light, and non-monochromatic light. The intensity of the light reflected at the particles is significantly brighter than the incoming light.165 Synthesis and the phenomenon of retroreflection are described in ref. 165.
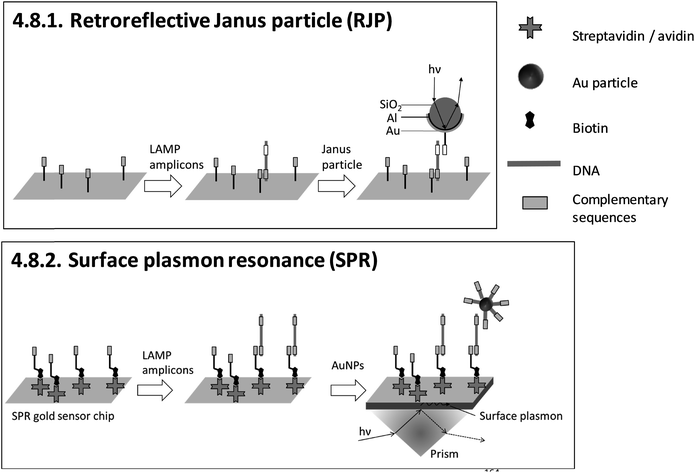 |
| Fig. 10 Schematic illustrations of 4.8.1. retroreflective Janus particle (RJP)164 and 4.8.2. surface plasmon resonance (SPR)166 (redrawn according to the references). | |
4.8.2. Surface plasmon resonance (SPR).
Gold sensor chips are modified with streptavidin and biotinylated target-specific probes. During LAMP, amplicons hybridize with probes immobilized on the sensor surface. AuNPs carrying target-specific probes can also be added to the LAMP mix for signal enhancement.166 AuNPs induce a pseudo mass increase, enhancing the refraction index change which is caused by the adsorption of amplicons onto the sensor surface (Fig. 10).167 The overall detection time after LAMP is about 30 minutes, including 10 minutes of initial baseline detection and 20 minutes of hybridization of the amplicons to the sensor surface. LAMP combined with SPR was first described for the detection of MRSA with an analytical sensitivity of 250 copies per reaction. Clinical performance was tested with a total of 90 Staphylococcus isolates from clinical specimens. All samples were identified correctly. Multiplex detection of S. aureus through the detection of femB and antibiotic resistance through the detection of mecA in MRSA, was demonstrated with a sensor array which enabled spatially resolved measurements.166 The authors claim that the sensors can be reused. The hybridization reaction between the amplicons and the probes is still too slow to allow real-time monitoring, but quantification by endpoint detection was demonstrated. Portable and handheld devices for SPR have already been addressed in literature168,169 and make this method also attractive for PON testing. However, implementation is still challenging as no low-cost commercial products are yet on the market.
4.9. Heterogeneous, particle-based detection – magnetoresistive
4.9.1. Giant magnetoresistive (GMR) measurement.
The detection system for GMR comprises a signal output instrument, a GMR detector and a disposable microfluidic chip. During LAMP, conducted in the microfluidic chip, a biotinylated primer is incorporated into the amplicon. The bottom of the channel on the chip is modified with target-specific probes. Amplicons generated during LAMP hybridize with the immobilized probes. After LAMP, streptavidin-conjugated magnetic nanoclusters are added to the mix, which is incubated for 15 minutes to allow binding between the streptavidin and the biotinylated amplicons. After a rinsing step, the microfluidic chip is transferred into the GMR detector and measurement of the resistance values of the GMR sensors begins. The signal intensity depends on the number of magnetic particles bound to the amplicons, which are immobilized on the bottom of the chip (Fig. 11).170 HBV was detected by GMR-LAMP with an analytical sensitivity of 10 copies HBV DNA per reaction. The GMR detecting system was custom made and is not commercially available. Advantages are that GMR detectors are inexpensive, small and portable. The detectors are universal and reusable, as no target-specific molecules are incorporated. Only endpoint detection was demonstrated. Real-time monitoring is difficult, as unbound magnetic nanoclusters must be washed away after LAMP.
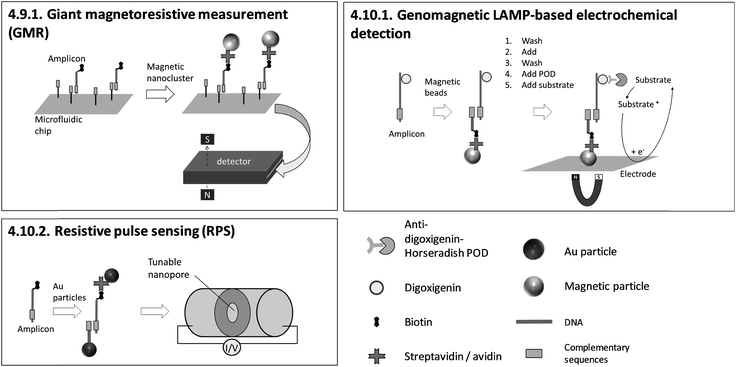 |
| Fig. 11 Schematic illustrations of 4.9.1. giant magnetoresistive (GMR) measurement,170 4.10.1. genomagnetic LAMP-based electrochemical detection171 and 4.10.2. resistive pulse sensing173 (redrawn according to the references). | |
4.10. Heterogeneous, particle-based detection – electrochemical
4.10.1. Genomagnetic LAMP-based electrochemical detection.
Digoxigenin-labelled nucleotides are incorporated in the amplicons during LAMP. After LAMP, the products are mixed with streptavidin-coated magnetic beads and biotinylated probes specific to the amplicons. The amplicons bind to the magnetic beads due to hybridization with the immobilized probe. Residual LAMP components are then washed away and anti-digoxigenin antibodies conjugated with horseradish POD are added to the mix. The beads are washed and transferred to an electrode on which they accumulate due to magnetic forces resulting from a magnet placed beneath the electrode. The addition of hydrogen peroxide and a substrate (hydroquinone) starts the enzymatic oxidation of the hydroquinone, which is subsequently reduced on the electrode surface (Fig. 11). The electrochemical reduction can be monitored chronoamperometrically. The turnaround time including LAMP is about 2.5 hours. This method was presented by Bartosik et al.171,172 for the detection of HPV types 16 and 18 on electrochemical chips using a multi potentiostat/galvanostat. The analytical sensitivity was described with a limit of detection of 0.1 ng DNA per reaction and a limit of quantification of 0.6 ng per reaction. The assay's specificity was confirmed with various cell lines, demonstrating discrimination between HPV16- and HPV18-positive cell lines. Three clinical samples were successfully analyzed. Advantages are that magnetic beads can be used for further purification steps, so that components which may inhibit the signal are washed away. Furthermore, inexpensive carbon electrodes are used, as no modification of the electrode surface is needed. The universal applicability of the electrodes and magnetic beads allows easy adaption to different targets.
4.10.2. Resistive pulse sensing (RPS).
LAMP amplicons carry biotin labels due to the incorporation of biotinylated primers. First, the amplicons are mixed with avidin-functionalized AuNPs. Then, AuNPs functionalized with target-specific probes are added. Interactions between the amplicons and the AuNPs generate clusters, which allows detection by resistive pulse sensing using a nanopore sensor. The nanopore separates two chambers which contain aqueous electrolytes and between which a constant voltage is applied. AuNP-amplicon clusters passing through the nanopore cause an ionic resistance, also called a ‘resistive pulse’ (Fig. 11). The nanopore platform is commercially available (qNano, IZON Science, Christchurch, New Zealand), whereas the AuNPs were synthesized and modified by the authors. LAMP-RPS comprises three manual steps: first, LAMP takes place, second, amplicons are mixed with AuNPs, and third, the AuNP-amplicon mix is transferred into the electrochemical chamber of the nanopore platform. Measurement duration after LAMP is about 10 minutes. LAMP-RPS was presented by Yang et al.173 for the detection of the Panton–Valentine leukocidin toxin encoding gene of MRSA. Analytical sensitivity was 530 copies per reaction. Turnaround time for LAMP and RPS was around 2 hours. The ionic resistance generated by AuNP-amplicon clusters passing through the nanopore depends on the size of the cluster and the NPs. Therefore, multiplexing could be achieved by using NPs with different, target-specific sizes. However, an accurate assignment may be difficult due to the undefined size of the clusters.
5. Instrumentation: open versus method-tailored platforms
In this chapter, we provide criteria for the assessment of the operational implementation of LAMP with sequence-specific detection. Table 2 shows platforms on which the individual methods can be implemented. Each platform comprises a device, as well as disposable tubes or cartridges.
The technological readiness level (TRL)174 of each platform is listed in a separate column and ranges from level three (demonstration of proof of concept) to nine (works in an operational environment and is ready for commercial deployment). Next, we differentiate between open platforms (O), which can be adapted to various protocols and reagents, and method-tailored platforms (M). All open platforms are already commercially available, and the methods have been adjusted to their requirements. In contrast, method-tailored platforms are custom-made and designed specifically for the applied method. As LAMP is predestined for on-site testing, another criterion for the assessment of a platform is its portability. We also indicated the use of disposable cartridges, like disks or chips, as these represent a substantial cost factor compared to the use of standard reaction tubes. Reusable cartridges or sensors have been shown to reduce costs, and therefore these are also marked in Table 2.
We next evaluated the practical operation. The evaluation was inspired by the key criteria given in the standards of the Clinical Laboratory Improvement Amendments (CLIA) program,175 which regulates clinical laboratory testing on patient specimens in the United States. Based on the available literature, we evaluated the complexity of the methods according to their CLIA score (Table 2). Scores range between one and three. A score of one indicates a low level of complexity, and score of three a high level of complexity.176 We provide scores for the following categories: preparation of reagents, characteristics of the operational steps, training, and expertise.175,176
6. Comparative general discussion
A brief description and visualization of the methods for sequence-specific detection of LAMP has been presented in chapter 4, and criteria for the evaluation of the platforms for the operational implementation of these methods have been presented in chapter 5. In the discussion chapter, we assess the methods and platforms according to the criteria specified in chapters 4.1 and 5 respectively. We also extend the assessment with additional criteria specific to homogeneous or heterogeneous detection methods.
First, we evaluate the status of the research with regard to the analytical performance of the methods and their ability to analyze complex sample material. We also highlight any possible shortcomings. In the second step, we evaluate the methods in a comparative manner. Homogeneous and heterogeneous detection methods are considered separately. Third, we focus on the operational implementation of various methods on the different instrumental platforms.
6.1. Analytical performance and analysis of complex sample material
The analytical performance of a large number of methods has been extensively examined in literature, as can be seen from Table 2. The majority of the methods (31 out of 34, 91%) have been evaluated according to analytical sensitivity. Moreover, the analysis of complex sample material has been demonstrated for more than half the methods (21 out of 34). Parameters from field studies, such as diagnostic sensitivity and diagnostic specificity, were reported for more than one third of the methods (12 out of 34). For future studies, we strongly suggest including investigations of repeatability (intraassay variance) and reproducibility (interassay variance), as these parameters were published in only 6% of the cases. The assay variance is a key parameter for the assessment of the precision and robustness of an assay and the variation in results between runs or between different laboratories.53
6.2. Homogeneous methods
Homogeneous sequence-specific methods are those which enable the detection of signals from the entire reaction volume due to the homogeneous distribution of biorecognition elements. Biorecognition elements are either dissolved in solution or immobilized on suspended particles. Homogeneous, particle-free methods make use of optical transducing elements (fluorescence) only, whereas particle-based methods can be differentiated by their use of optical (fluorescence, chemiluminescence, scattering, reflection or colorimetry) or magnetic transducing elements.
Since a large proportion of the homogeneous methods (18 out of 23) are based on fluorescence, we first discuss and compare fluorescence-based methods in detail. For this, we extend the general assessment criteria (quantification, multiplexing and detection by naked eye, chapter 4.1) and focus additionally on the modification of primers and probes, the optimization of fluorescence signals and the adaption to different targets.
Most of the fluorescence-based methods for sequence-specific detection of LAMP allow real-time monitoring of LAMP, and generate rapid and quantitative results using single- or multiple-fluorescence-labelled primers and probes. To obtain high amplification efficiencies and to maximize signal-to-noise ratios, the concentrations and ratios of fluorescence-labelled primers and probes must be adjusted carefully for each new target sequence. Semi-universal and universal methods like 4.2.6. universal QProbe, 4.2.13. assimilating probe and 4.2.14. MD LAMP render elaborate work to optimize the signal redundant, as the fluorophore and quencher are located at universal sequences. Consequently, these methods allow simple adaption to various target panels. 4.2.7. GO based FRET stands out for its excellent fluorescence yields due to efficient quenching properties. However, a drawback is an additional handling step required for adding graphene oxide (GO) after the LAMP reaction.
Adding PEI after LAMP enables instrument-free, visual endpoint detection by naked eye. This method was applied after 4.2.1. FLOS LAMP and 4.2.2. HyBeacon probes. Visual detection by naked eye was also demonstrated for 4.2.9. QUASR. All methods based on fluorescence enable multiplex detection and, apart from one exception, quantification of the target analytes, with 4.2.4. ABC-LAMP placing special focus on precise quantification. 4.2.17. pyrosequencing is particularly suitable for multiplexing. However, the simultaneous amplification of numerous targets by LAMP is not easy to implement, and therefore the efficiency of amplification is the limiting factor.
The next section deals with homogeneous methods based on suspended particles. The general assessment criteria (chapter 4.1) are extended to cover the modification of primers, probes or particles, real-time vs. endpoint detection, quantification, multiplexing and detection by naked eye. In contrast to fluorescence-based methods, only half of the homogeneous, particle-based methods require fluorescence-labelling or further modification of the primers or probes participating in the LAMP reaction. Target-specific probes are immobilized covalently or due to streptavidin-biotin interaction on particle surfaces. Except for wash-free methods like 4.3.3. optomagnetic detection and 4.4.1. AC susceptometry, all the particle-based methods focus on endpoint detection. 4.3.1. BEAMing LAMP and 4.3.2. SERS have a complex workflow comprising many individual manual steps. However, BEAMing LAMP stands out for its precise, digital quantification without the need of standards, while SERS is capable of single molecule detection and therefore enables highly sensitive measurements. Furthermore, BEAMing LAMP is the only homogeneous, particle-based method capable of multiplexing. Visual detection by naked eye is possible with 4.3.5. magnetogenosensor and 4.3.4. AuNPs. Moreover, a breakthrough was achieved by detecting LAMP products with AuNPs in 4.3.4.1. lateral flow dipsticks after LAMP. Lateral flow dipsticks are easy to handle and universally applicable due to their probe-free sensing surfaces.
6.3. Heterogeneous methods
Heterogeneous detection methods make use of planar sensing surfaces, in some instances combined with suspended particles. In contrast to homogeneous methods, the biorecognition elements are in close proximity or linked to the sensing surface during signal transduction. Heterogeneous methods make use of various transducing elements, namely optical (colorimetry, reflection), piezoelectric, electrochemical (amperometry, voltammetry, impedimetry) and magnetoresistive elements.
In addition to the general assessment criteria defined in chapter 4.1 (quantification, multiplexing and detection by naked eye), heterogeneous detection methods are evaluated according to the modification of primers, probes, particles or sensing surfaces, the hybridization time, and real-time vs. endpoint detection. These criteria apply equally to particle-free and particle-based methods, and these are therefore considered jointly.
Sensing and particle surfaces are coated either with streptavidin or with covalently binding, target-specific probes. The insertion of biotin into amplicons by biotin-labelled primers is unproblematic regarding the efficiency of amplification due to the small size of biotin. However, for 4.5.1. LAMP-ELISA and 4.10.1. genomagnetic LAMP-based electrochemical detection, digoxigenin-labelled dUTPs are inserted into amplicons. The use of digoxigenin-labelled dUTPs causes additional optimization work, since the amplification efficiency is decreased for high dUTP concentrations.177 In contrast, biotin- and fluorescence label-free methods (4.7.1.–4.7.4., 4.8.1., 4.8.2.) are advantageous with regard to assay optimization, as no modification of primers is required at all.
The dependency between signal intensity and target concentration allows rough quantification by endpoint detection. Precise quantification can be achieved by real-time monitoring. In general, slow diffusion rates of target molecules and amplicons migrating towards the sensing surface178 increase the time for hybridization. Long hybridization times pose a general obstacle for real-time monitoring and therefore for precise quantification. 4.10.1. genomagnetic LAMP-based electrochemical detection is limited to endpoint detection, but it circumvents long hybridization times by accumulating amplicons, bound to magnetic beads, on the electrode surfaces with the help of magnetic forces. A pioneer of real-time monitoring is 4.6.1. QCM, albeit with a pre-incubation step in a chamber separated from the sensing surface before polymerase is added. A further important issue concerning precise quantification arises when using biotinylated primers. For 4.5.1. LAMP-ELISA, 4.6.1. QCM, 4.10.1. GMR and 4.10.2. RPS, biotinylated amplicons compete with excess biotinylated primers for binding to streptavidin-coated sensing surfaces or particles, affecting the sensitivity of the assay.
An advantage of heterogeneous detection methods, which are based on planar sensing surfaces, is the simultaneous detection of multiple targets by spatially resolved measurements,158–160,166 or alternatively by wavelength division179,180 or spectral analysis of multiple light beams.181 Multiplex detection and differentiation between targets was demonstrated exclusively for 4.7.2. and 4.7.3. electrochemical detection with redox reporters, and 4.8.2. SPR. Another achievement is 4.7.1. nanopore sequencing, which provides detailed genotyping analysis. However, this is at the expense of requiring elaborate post-LAMP DNA library preparation. 4.5.1. LAMP-ELISA allows nearly instrument-free handling and surpasses almost all methods with regard to high throughput by using microtiter plates. No heterogeneous method allows visual detection by naked eye.
6.4. Instrumental platforms
More than two thirds of all methods are implemented on open instrumental platforms. Fluorescence-based methods make up a large proportion of these. Except for 4.3.1. BEAMing LAMP, all fluorescence-based methods are compatible with standard isothermal amplification devices with an integrated fluorescence reader. The open instrumental platforms listed in Table 2 are commercially available and work in operational environments (TRL 9). For method-tailored platforms, only demonstrations have been presented so far. These have only been validated in the laboratory environment and are still assigned low TRLs (TRL 4). Open instrumental platforms can also be combined with method-tailored cartridges (disks or chips), and method-tailored platforms can be combined with commercially available and universal cartridges (Table 2, O/M). In order to bring these methods to the market, higher TRLs, i.e. validation in operational environments must be achieved.
On-site testing at the PON requires portable instrumentation based on simple equipment. Platforms designed specifically to meet the demands for use at the PON182,183 have mainly been presented for fluorescence-based detection. Simple readout via smartphone, replacing bulky and expensive optical components, has been implemented by a portable LAMP box74,75 or combined with filter-based sample preparation.85,86 The integration of small smartphones into diagnostic platforms can reduce costs considerably. In future, exploitation of the variety of sensors hosted in smartphones, including optical sensors, wireless data transfer and a global positioning system, could also be beneficial in the context of telemedicine and e-health. Testing in the field requires devices which do not require electric power or any other external energy supply. The only devices which do not require power supply are so far method-tailored and comprise power-free devices for incubation at LAMP conditions combined with a fluorometer or smartphone readout.97,98 Both devices follow the same clever approach of generating heat for LAMP incubation with an exothermic reaction.
Devices with microfluidic cartridges enable fully automated LAMP with subsequent detection (4.2.9., 4.2.11., 4.7.2., 4.9.1.), facilitating on-site sample processing.82,89,158,170 Compared to methods based on fluorescence, electrochemical readout seems more amenable to miniaturization due to the independence of optical path lengths, but suffers from the additional costs of disposables with integrated electrodes.
Probe-free or streptavidin-coated universal sensor surfaces (4.5.1., 4.6.1., 4.7.1., 4.10.1., 4.10.2.) are attractive as these methods reduce costs by enabling cheap, large-scale fabrication of target-independent disposables. One highlight is 4.10.1. genomagnetic LAMP-based electrochemical detection, which uses unmodified and inexpensive carbon electrodes. Moreover, instrumentation providing reusable sensing surfaces (4.6.1., 4.7.2., 4.8.2., 4.9.1., 4.10.2.) enables further cost reduction. However, we would like to point out that carryover contamination with amplicons due to the reuse of sensors poses a tremendous risk to reliable results. Therefore, LAMP and detection should be conducted in separate chambers, as is demonstrated for all methods except 4.6.1. QCM.
We now evaluate the complexity of the practical operation of the methods. The majority of fluorescence-based detection methods used standard isothermal amplification devices with an integrated fluorescence reader and enable real-time detection of LAMP. These stand out due to a low degree of complexity. Scores for all three categories of complexity, comprising first the preparation of reagents, second the characteristics of the operational steps and third training and expertise, are one. First, biorecognition elements can be pre-stored in the primer mix. Second, real-time monitoring in a thermal cycler doesn't require post-LAMP steps. Consequently, the complexity of the operational steps and the required training and expertise is low.
Time-consuming operational steps comprising the addition of particles, incubation and washing steps, require trained staff and therefore have a higher level of complexity. Particle-based methods at a developmental stage involve elaborate particle modification, raising their complexity score for reagent preparation and expertise to two and two/three respectively. Furthermore, the modification of particles and sensor surfaces increases the complexity of the reagent preparation even more. Large-scale fabrication and the automation of operational steps in the course of a market launch will lower their complexity and increase their user-friendliness.
7. Conclusion and future prospects
LAMP is gaining more and more attention as field-friendly analytical tool, especially for PON applications, due to its fast processing times and simple instrumentation.9 Methods for sequence-specific detection of LAMP make full use of the specificity towards the target analyte for which LAMP is especially renowned, and are thus preferable to sequence-independent methods. For the assessment and comparison of sequence-specific methods, we provided a catalogue of assessment criteria covering the most important aspects (Table 2): analytical performance, handling of complex sample material, multiplex capacity, the ability to quantify the target analyte and detection by naked eye. Additionally, we evaluated the method-related platforms according to their TRL, accessibility (open/method-tailored platforms), portability, disposability of cartridges, reusability and complexity (reagent preparation, operational steps and training/expertise), as summarized in Table 2. The methods differ from each other in many aspects. Focus is placed on precise quantification (4.2.4. ABC-LAMP, 4.3.1. BEAMing LAMP), multiplex detection and genotyping (sequencing 4.2.17., 4.7.1.) or detection by naked eye (adding PEI after LAMP 4.2.1. and 4.2.2., 4.3.4. AuNPs, lateral flow dipsticks 4.3.4.1., 4.3.5. magnetogenosensor, 4.5.1. LAMP-ELISA).
Homogeneous detection methods capture the signal from the entire sample volume, predominantly in real time, enabling fast results and quantification without time-consuming washing steps. There is a large variety of homogeneous, fluorescence-based detection methods that have many similarities with regard to their required instrumental platforms and the complexity of their practical operation. Semi-universal (4.2.6., 4.2.13.) and universal (4.2.14.) homogeneous methods feature unique universal properties, and thus can be easily adapted to different targets. Heterogeneous, surface-based detection is mainly restricted to endpoint detection due to slow diffusion processes, but also has advantages, as it is more easily miniaturizable and integrable into μTAS systems. First steps towards real-time monitoring have been made by 4.6.1. QCM, a pioneering method which does not require post-LAMP modifications. Electrochemical detection is another heterogeneous method which is a promising candidate for real-time monitoring. In contrast to PCR, which suffers from thermally unstable bonds between the electrode surface and oligonucleotide probes during cycles,184,185 LAMP allows more moderate thermal conditions and paves the way for simultaneous amplification and heterogeneous detection.
The miniaturization and automation of operational steps in μTAS systems facilitates usage at the PON. So far, innovative solutions have been realized by fluorescence82 and electrochemical detection methods.158–160 In the context of miniaturization, electrochemical detection surpasses optical readout, as no long path lengths, lenses or waveguides are necessary. The latest trends in research show that bulky optical components can be replaced by smartphones.74,75,85,86 Further developments could lead to various heterogeneous detection methods being combined with simple smartphone readout, making expensive and sophisticated devices redundant and thus increasing user-friendliness. An exciting and stimulating demonstration of the smartphone approach is the detection of SPR, with a smartphone as the transducing element.186 In addition, novel technologies in the field of electrochemical detection make use of smartphones for signal processing and data transmission.187–189 Generally speaking, the combination of field-friendly LAMP and smart devices is a forward-looking approach that has the potential to bring diagnostics even closer to the patient.
Some methods, including mainly fluorescence-based methods but also sequencing 4.2.17. and 4.7.1., 4.3.2. SERS, 4.4.1. AC susceptometry, 4.5.1. LAMP-ELISA, 4.10.1. genomagnetic LAMP-based electrochemical detection and 4.10.2. resistive pulse sensing, can be implemented on open platforms which are already commercially available. Method-tailored platforms presented in this review are still in their youth and do not exceed TRL4, but convincing PON concepts have been shown in the laboratory environment. The next steps required to reach higher TRLs and aim for market launch will be the adaption to operational environments and the development of prototypes.
In summary, there are various methods for the sequence-specific detection of LAMP which distinguish themselves by their different intended uses. Our review intends to provide a comprehensive overview and a systematic evaluation of these methods with regard to different user requirements, ranging from the overall performance of the method to the related instrumental platforms. Ultimately, it may serve as a guideline for the selection of the most appropriate methods.
Abbreviations
ABC-LAMP | Alternately binding quenching probe competitive LAMP |
AuNPs | Gold nano particles |
B3 | Backward outer primer |
BIP | Backward inner primer |
CLIA | Clinical laboratory improvement amendments |
DARQ | Detection of amplification by release of quenching |
DENV | Dengue virus |
dUTP | 2′-Deoxyuridine, 5′-triphosphate |
EtBr | Ethidium bromide |
F3 | Forward outer primer |
FIP | Forward inner primer |
FLOS | Fluorescence of loop primer upon self-dequenching |
FRET | Förster resonance energy transfer |
GMR | Giant magnetoresistive |
GO | Graphene oxide |
HDA | Helicase dependent amplification |
H(B, C, E)V | Hepatitis B, C, E virus |
HIV | Human immunodeficiency virus |
HPV | Human papillomavirus |
LAMP | Loop-mediated isothermal amplification |
LAMP-ELISA | LAMP detected by enzyme-linked immunosorbent assay |
LB | Backward loop primer |
LF | Forward loop primer |
MD LAMP | Mediator displacement LAMP |
MERT-LAMP | Multiple endonuclease restriction real-time LAMP |
MNP | Magnet nano particle |
MRSA | Methicillin-resistant Staphylococcus aureus |
NAAT | Nucleic acid amplification test |
NASBA | Nucleic acid sequence based amplification |
NP | Nano particle |
PEI | Polyethylenimine |
POC | Point-of-care |
PON | Point-of-need |
QCM | Quartz crystal microbalance |
QUASR | Quenching of unincorporated amplification signal reporters |
LAMP-OSD | LAMP one-step strand displacement |
QProbe | Quenching probe |
RCA | Rolling circle amplification |
RJP | Retroreflective Janus particle |
PCR | Polymerase chain reaction |
RPA | Recombinase polymerase amplification |
RPS | Resistive pulse sensing |
SDA | Strand displacement amplification |
SERS | Surface enhanced Raman spectroscopy |
SNP | Single nucleotide polymorphism |
SPR | Surface plasmon resonance |
TEC-LAMP | Tth endonuclease cleavage LAMP |
TMA | Transcription-mediated amplification |
TRL | Technological readiness level |
UR | Universal reporter |
WNV | West Nile virus |
WSSV | White spot syndrome virus |
μTAS | Miniaturized total chemical analysis systems |
Conflicts of interest
The authors declare no conflict of interest.
References
- K. Mullis, F. Faloona, S. Scharf, R. Saiki, G. Horn and H. Erlich, Cold Spring Harbor Symp. Quant. Biol., 1986, 51, 263–273 CrossRef CAS PubMed.
- K. B. Mullis and F. A. Faloona, Methods Enzymol., 1987, 155, 335–350 CAS.
- S. Yang and R. E. Rothman, Lancet Infect. Dis., 2004, 4, 337–348 CrossRef CAS.
- K. Mann and C. M. Ogilvie, Prenatal Diagn., 2012, 32, 309–314 CrossRef CAS PubMed.
- L. S. Kristensen and L. L. Hansen, Clin. Chem., 2009, 55, 1471–1483 CrossRef CAS PubMed.
- S. E. Cavanaugh and A. S. Bathrick, Forensic Sci. Int.: Genet., 2018, 32, 40–49 CrossRef CAS PubMed.
- I. Laube, J. Zagon and H. Broll, Int. J. Food Sci. Technol., 2007, 42, 336–341 CrossRef CAS.
- H. A. Bowers, T. Tengs, H. B. Glasgow, J. M. Burkholder, P. A. Rublee and D. W. Oldach, Appl. Environ. Microbiol., 2000, 66, 4641–4648 CrossRef CAS PubMed.
- Z. K. Njiru, PLoS Neglected Trop. Dis., 2012, 6, e1572 CrossRef PubMed.
- X. Fang, Y. Liu, J. Kong and X. Jiang, Anal. Chem., 2010, 82, 3002–3006 CrossRef CAS PubMed.
- P. Craw and W. Balachandran, Lab Chip, 2012, 12, 2469–2486 RSC.
- M. M. Ali, F. Li, Z. Zhang, K. Zhang, D.-K. Kang, J. A. Ankrum, X. C. Le and W. Zhao, Chem. Soc. Rev., 2014, 43, 3324–3341 RSC.
- Y. Mori, H. Kanda and T. Notomi, J. Infect. Chemother., 2013, 19, 404–411 CrossRef CAS PubMed.
- J. Li, J. Macdonald and F. von Stetten, Analyst, 2018, 144, 31–67 RSC.
- A. Borst, J. Verhoef, E. Boel and A. C. Fluit, Clin. Lab., 2002, 48, 487–492 CAS.
- B. J. Toley, I. Covelli, Y. Belousov, S. Ramachandran, E. Kline, N. Scarr, N. Vermeulen, W. Mahoney, B. R. Lutz and P. Yager, Analyst, 2015, 140, 7540–7549 RSC.
- M. Vincent, Y. Xu and H. Kong, EMBO Rep., 2004, 5, 795–800 CrossRef CAS PubMed.
- L. Comanor, Am. J. Gastroenterol., 2001, 96, 2968–2972 CrossRef CAS PubMed.
- T. Notomi, H. Okayama, H. Masubuchi, T. Yonekawa, K. Watanabe, N. Amino and T. Hase, Nucleic Acids Res., 2000, 28, E63 CrossRef CAS PubMed.
- K. Nagamine, T. Hase and T. Notomi, Mol. Cell. Probes, 2002, 16, 223–229 CrossRef CAS PubMed.
- P. Francois, M. Tangomo, J. Hibbs, E.-J. Bonetti, C. C. Boehme, T. Notomi, M. D. Perkins and J. Schrenzel, FEMS Immunol. Med. Microbiol., 2011, 62, 41–48 CrossRef CAS PubMed.
- H. Kaneko, T. Kawana, E. Fukushima and T. Suzutani, J. Biochem. Biophys. Methods, 2007, 70, 499–501 CrossRef CAS PubMed.
- Y. Mori, K. Nagamine, N. Tomita and T. Notomi, Biochem. Biophys. Res. Commun., 2001, 289, 150–154 CrossRef CAS PubMed.
- Y. Mori, M. Kitao and T. Notomi, J. Biochem. Biophys. Methods, 2004, 59, 145–157 CrossRef CAS PubMed.
- N. Kuboki, N. Inoue, T. Sakurai, F. Di Cello, D. J. Grab, H. Suzuki, C. Sugimoto and I. Igarashi, J. Clin. Microbiol., 2003, 41, 5517–5524 CrossRef CAS PubMed.
- Y. Mori, H. Kanda and T. Notomi, Nat. Protoc., 2008, 3, 877–882 CrossRef PubMed.
- N. A. Tanner and T. C. Evans, Curr. Protoc. Mol. Biol., 2014, 105, 15.14 Search PubMed.
- T. Iwamoto, T. Sonobe and K. Hayashi, J. Clin. Microbiol., 2003, 41, 2616–2622 CrossRef CAS PubMed.
- M. Goto, E. Honda, A. Ogura, A. Nomoto and K.-I. Hanaki, BioTechniques, 2009, 46, 167–172 CrossRef CAS PubMed.
- S. J. Oh, B. H. Park, J. H. Jung, G. Choi, D. C. Lee, D. H. Kim and T. S. Seo, Biosens. Bioelectron., 2016, 75, 293–300 CrossRef CAS PubMed.
- D. Zhang, B. Gao, C. Zhao and H. Liu, Langmuir, 2019, 35, 248–253 CrossRef CAS PubMed.
- S. Roy, I. A. Rahman and M. U. Ahmed, Anal. Methods, 2016, 8, 2391–2399 RSC.
- A.-R. Cho, H.-J. Dong and S. Cho, Korean Journal for Food Science of Animal Resources, 2014, 34, 799–807 CrossRef PubMed.
- T. Cai, G. Lou, J. Yang, D. Xu and Z. Meng, J. Clin. Virol., 2008, 41, 270–276 CrossRef CAS PubMed.
- N. W. Lucchi, A. Demas, J. Narayanan, D. Sumari, A. Kabanywanyi, S. P. Kachur, J. W. Barnwell and V. Udhayakumar, PLoS One, 2010, 5, e13733 CrossRef PubMed.
- H. Maeda, S. Kokeguchi, C. Fujimoto, I. Tanimoto, W. Yoshizumi, F. Nishimura and S. Takashiba, FEMS Immunol. Med. Microbiol., 2005, 43, 233–239 CrossRef CAS PubMed.
- O. A. Gandelman, V. L. Church, C. A. Moore, G. Kiddle, C. A. Carne, S. Parmar, H. Jalal, L. C. Tisi and J. A. H. Murray, PLoS One, 2010, 5, e14155 CrossRef CAS PubMed.
- P. Hardinge, G. Kiddle, L. Tisi and J. A. H. Murray, Sci. Rep., 2018, 8, 17590 CrossRef PubMed.
- S. Roy, S. X. Wei, J. L. Z. Ying, M. Safavieh and M. U. Ahmed, Biosens. Bioelectron., 2016, 86, 346–352 CrossRef CAS PubMed.
- N. F. N. Azam, S. Roy, S. A. Lim and M. Uddin Ahmed, Food Chem., 2018, 248, 29–36 CrossRef CAS PubMed.
- D.-G. Wang, J. D. Brewster, M. Paul and P. M. Tomasula, Molecules, 2015, 20, 6048–6059 CrossRef CAS.
- D. Lee, M. La Mura, T. R. Allnutt and W. Powell, BMC Biotechnol., 2009, 9, 7 CrossRef PubMed.
- O. Mayboroda, I. Katakis and C. K. O'Sullivan, Anal. Biochem., 2018, 545, 20–30 CrossRef CAS PubMed.
- M. A. Morales and J. M. Halpern, Bioconjugate Chem., 2018, 29, 3231–3239 CrossRef CAS PubMed.
-
P. Sengupta, K. Khanra, A. R. Chowdhury and P. Datta, Bioelectronics and Medical Devices: From Materials to Devices - Fabrication, Applications and Reliability, 2019, ch. 4, pp. 47–95 Search PubMed.
- P. J. Asiello and A. J. Baeumner, Lab Chip, 2011, 11, 1420–1430 RSC.
- S. Shoji, Electron. Commun. Jpn. Part II Electron., 1999, 82, 21–29 CrossRef.
- H. Suzuki, Mater. Sci. Eng., C, 2000, 12, 55–61 CrossRef.
- L. Marle and G. M. Greenway, TrAC, Trends Anal. Chem., 2005, 24, 795–802 CrossRef CAS.
- A. J. Tudos, G. J. Besselink and R. B. Schasfoort, Lab Chip, 2001, 1, 83–95 RSC.
- P. K. Sorger, Nat. Biotechnol., 2008, 26, 1345–1346 CrossRef CAS PubMed.
- X. Zhang, S. B. Lowe and J. J. Gooding, Biosens. Bioelectron., 2014, 61, 491–499 CrossRef CAS PubMed.
- S. A. Bustin, V. Benes, J. A. Garson, J. Hellemans, J. Huggett, M. Kubista, R. Mueller, T. Nolan, M. W. Pfaffl, G. L. Shipley, J. Vandesompele and C. T. Wittwer, Clin. Chem., 2009, 55, 611–622 CrossRef CAS PubMed.
-
IUPAC, Compendium of Chemical Terminology, 2nd edn (the “Gold Book”), Compiled by A. D. McNaught and A. Wilkinson, Blackwell Scientific Publications, Oxford, 2019th edn, 1997 Search PubMed.
- B. Nagel, H. Dellweg and L. M. Gierasch, Pure Appl. Chem., 1992, 64, 143–168 CAS.
- I. Nazarenko, B. Lowe, M. Darfler, P. Ikonomi, D. Schuster and A. Rashtchian, Nucleic Acids Res., 2002, 30, e37 CrossRef PubMed.
- I. Nazarenko, R. Pires, B. Lowe, M. Obaidy and A. Rashtchian, Nucleic Acids Res., 2002, 30, 2089–2195 CrossRef CAS PubMed.
- V. J. Gadkar, D. M. Goldfarb, S. Gantt and P. A. G. Tilley, Sci. Rep., 2018, 8, 5548 CrossRef PubMed.
- S. Fukuta, H. Nagai, R. Suzuki, Y. Matsumoto, S. Kato, N. Saka, H. Horikawa and N. Miyake, Ann. Appl. Biol., 2017, 170, 170–178 CrossRef CAS.
- R. Suzuki, H. Tanaka, K. Suzuki, S. Fukuta, S. Kato and T. Ohno, J. Appl. Entomol., 2018, 142, 745–754 CrossRef CAS.
- R. L. Howard, D. J. French, J. A. Richardson, C. E. O'Neill, M. P. Andreou, T. Brown, D. Clark, I. N. Clarke, J. W. Holloway, P. Marsh and P. G. Debenham, Mol. Cell. Probes, 2015, 29, 92–98 CrossRef CAS PubMed.
- Y. Mori, T. Hirano and T. Notomi, BMC Biotechnol., 2006, 6, 3 CrossRef PubMed.
- I. Takayama, M. Nakauchi, H. Takahashi, K. Oba, S. Semba, A. Kaida, H. Kubo, S. Saito, S. Nagata, T. Odagiri and T. Kageyama, J. Virol. Methods, 2019, 267, 53–58 CrossRef CAS PubMed.
- H. Tani, T. Teramura, K. Adachi, S. Tsuneda, S. Kurata, K. Nakamura, T. Kanagawa and N. Noda, Anal. Chem., 2007, 79, 5608–5613 CrossRef CAS PubMed.
- Y. Kouguchi, T. Fujiwara, M. Teramoto and M. Kuramoto, Mol. Cell. Probes, 2010, 24, 190–195 CrossRef CAS PubMed.
- Y. Ayukawa, S. Hanyuda, N. Fujita, K. Komatsu and T. Arie, Sci. Rep., 2017, 7, 4253 CrossRef PubMed.
- U. Waiwijit, D. Phokaratkul, J. Kampeera, T. Lomas, A. Wisitsoraat, W. Kiatpathomchai and A. Tuantranont, J. Biotechnol., 2015, 212, 44–49 CrossRef CAS PubMed.
- N. A. Tanner, Y. Zhang and T. C. Evans, BioTechniques, 2012, 53, 81–89 CrossRef CAS PubMed.
- M. Mashooq, D. Kumar, A. K. Niranjan, R. K. Agarwal and R. Rathore, J. Microbiol. Methods, 2016, 126, 24–29 CrossRef CAS PubMed.
- D. Kumar, T. K. S. Chauhan, R. K. Agarwal, K. Dhama, P. P. Goswami, A. K. Mariappan, A. K. Tiwari and B. P. Mishra, Arch. Virol., 2017, 162, 979–985 CrossRef CAS PubMed.
- I. A. Nanayakkara and I. M. White, Analyst, 2019, 144, 3878–3885 RSC.
- I. A. Bhat, M. Mashooq, D. Kumar, R. Varshney and R. Rathore, J. Appl. Microbiol., 2018, 126(5), 1332–1339 CrossRef PubMed.
- C. S. Ball, Y. K. Light, C.-Y. Koh, S. S. Wheeler, L. L. Coffey and R. J. Meagher, Anal. Chem., 2016, 88, 3562–3568 CrossRef CAS PubMed.
- A. Priye, S. W. Bird, Y. K. Light, C. S. Ball, O. A. Negrete and R. J. Meagher, Sci. Rep., 2017, 7, 44778 CrossRef CAS PubMed.
- A. Priye, C. S. Ball and R. J. Meagher, Anal. Chem., 2018, 90, 12385–12389 CrossRef CAS PubMed.
- C. S. Ball, R. F. Renzi, A. Priye and R. J. Meagher, Lab Chip, 2016, 16, 4436–4444 RSC.
- K. A. Curtis, D. L. Rudolph, I. Nejad, J. Singleton, A. Beddoe, B. Weigl, P. LaBarre and S. M. Owen, PLoS One, 2012, 7, e31432 CrossRef CAS PubMed.
- A. Bektaş, Food Analytical Methods, 2018, 11, 686–692 CrossRef.
- K. A. Curtis, D. L. Rudolph and S. M. Owen, J. Med. Virol., 2009, 81, 966–972 CrossRef CAS PubMed.
- K. A. Curtis, D. L. Rudolph, D. Morrison, D. Guelig, S. Diesburg, D. McAdams, R. A. Burton, P. LaBarre and M. Owen, J. Virol. Methods, 2016, 237, 132–137 CrossRef CAS PubMed.
- D. L. Rudolph, V. Sullivan, S. M. Owen and K. A. Curtis, PLoS One, 2015, 10, e0126609 CrossRef PubMed.
- C. R. Phaneuf, B. Mangadu, H. M. Tran, Y. K. Light, A. Sinha, F. W. Charbonier, T. P. Eckles, A. K. Singh and C.-Y. Koh, Biosens. Bioelectron., 2018, 120, 93–101 CrossRef CAS PubMed.
- Y. S. Jiang, S. Bhadra, B. Li, Y. R. Wu, J. N. Milligan and A. D. Ellington, Anal. Chem., 2015, 87, 3314–3320 CrossRef CAS PubMed.
- S. Bhadra, Y. S. Jiang, M. R. Kumar, R. F. Johnson, L. E. Hensley and A. D. Ellington, PLoS One, 2015, 10, e0123126 CrossRef PubMed.
- Y. S. Jiang, T. E. Riedel, J. A. Popoola, B. R. Morrow, S. Cai, A. D. Ellington and S. Bhadra, Water Res., 2018, 131, 186–195 CrossRef CAS PubMed.
- S. Bhadra, T. E. Riedel, M. A. Saldaña, S. Hegde, N. Pederson, G. L. Hughes and A. D. Ellington, PLoS Neglected Trop. Dis., 2018, 12, e0006671 CrossRef PubMed.
- D.-C. Nyan and K. L. Swinson, Sci. Rep., 2015, 5, 17925 CrossRef CAS PubMed.
- W. Liu, S. Huang, N. Liu, D. Dong, Z. Yang, Y. Tang, W. Ma, X. He, D. Ao, Y. Xu, D. Zou and L. Huang, Sci. Rep., 2017, 7, 40125 CrossRef CAS PubMed.
- L. Wan, T. Chen, J. Gao, C. Dong, A. H.-H. Wong, Y. Jia, P.-I. Mak, C.-X. Deng and R. P. Martins, Sci. Rep., 2017, 7, 14586 CrossRef PubMed.
- P. Bakthavathsalam, G. Longatte, S. O. Jensen, M. Manefield and J. J. Gooding, Sens. Actuators, B, 2018, 268, 255–263 CrossRef CAS.
- J. Xu, Y. Hu, J. Guo, Y. Yang, J. Qiu, X. Li and Z. Xin, Food Analytical Methods, 2019, 12, 422–430 CrossRef.
- Y. Xu, Chem. Soc. Rev., 2011, 40, 2719–2740 RSC.
- P. Travascio, A. J. Bennet, D. Y. Wang and D. Sen, Chem. Biol., 1999, 6, 779–787 CrossRef CAS PubMed.
- P.-H. Chou, Y.-C. Lin, P.-H. Teng, C.-L. Chen and P.-Y. Lee, J. Virol. Methods, 2011, 173, 67–74 CrossRef CAS PubMed.
- R. Komura, T. Kawakami, K. Nakajima, H. Suzuki and C. Nakashima, J. Gen. Plant Pathol., 2018, 84, 247–253 CrossRef CAS.
- R. Kubota, A. M. Alvarez, W. W. Su and D. M. Jenkins, Biol. Eng. Trans., 2011, 4, 81–100 CAS.
- R. Kubota, P. LaBarre, J. Singleton, A. Beddoe, B. H. Weigl, A. M. Alvarez and D. M. Jenkins, Biol. Eng. Trans., 2011, 4, 69–80 CAS.
- R. Kubota, P. LaBarre, B. H. Weigl, Y. Li, P. Haydock and D. M. Jenkins, Chin. Sci. Bull., 2013, 58, 1162–1168 CrossRef CAS PubMed.
- D. M. Jenkins, R. Kubota, J. Dong, Y. Li and D. Higashiguchi, Biosens. Bioelectron., 2011, 30, 255–260 CrossRef CAS PubMed.
- R. Kubota and D. M. Jenkins, Int. J. Mol. Sci., 2015, 16, 4786–4799 CrossRef CAS.
- L. Becherer, M. Bakheit, S. Frischmann, S. Stinco, N. Borst, R. Zengerle and F. von Stetten, Anal. Chem., 2018, 90, 4741–4748 CrossRef CAS PubMed.
- L. Becherer, S. Knauf, M. Marks, S. Lueert, S. Frischmann, N. Borst, F. von Stetten, S. Bieb, Y. Adu-Sarkodie, K. Asiedu, O. Mitja and M. Bakheit, Emerging Infect. Dis., 2020, 26, 282–288 Search PubMed.
- Y. Wang, Y. Wang, R. Lan, H. Xu, A. Ma, D. Li, H. Dai, X. Yuan, J. Xu and C. Ye, J. Mol. Diagn., 2015, 17, 392–401 CrossRef CAS PubMed.
- Y. Wang, D. Li, Y. Wang, K. Li and C. Ye, Molecules, 2016, 21, E111 CrossRef PubMed.
- O. Higgins, E. Clancy, M. Cormican, T. W. Boo, R. Cunney and T. J. Smith, Int. J. Mol. Sci., 2018, 19, 524 CrossRef PubMed.
- C. Liang, Y. Chu, S. Cheng, H. Wu, T. Kajiyama, H. Kambara and G. Zhou, Anal. Chem., 2012, 84, 3758–3763 CrossRef CAS PubMed.
- J. Chen, X. Xu, Z. Huang, Y. Luo, L. Tang and J.-H. Jiang, Chem. Commun., 2018, 54, 291–294 RSC.
- L. Cao, X. Cui, J. Hu, Z. Li, J. R. Choi, Q. Yang, M. Lin, L. Ying Hui and F. Xu, Biosens. Bioelectron., 2017, 90, 459–474 CrossRef CAS PubMed.
- A. S. Basu, SLAS Technol., 2017, 22, 369–386 Search PubMed.
- P.-L. Quan, M. Sauzade and E. Brouzes, Sensors, 2018, 18, 1271 CrossRef.
- M. S. Draz and X. Lu, Theranostics, 2016, 6, 522–532 CrossRef CAS PubMed.
- G. A. S. Minero, C. Nogueira, G. Rizzi, B. Tian, J. Fock, M. Donolato, M. Strömberg and M. F. Hansen, Analyst, 2017, 142, 3441–3450 RSC.
- B. Tian, J. Ma, T. Zardán Gómez de la Torre, Á. Bálint, M. Donolato, M. F. Hansen, P. Svedlindh and M. Strömberg, ACS Sens., 2016, 1, 1228–1234 CrossRef CAS.
- Y. Ge, Q. Zhou, K. Zhao, Y. Chi, B. Liu, X. Min, Z. Shi, B. Zou and L. Cui, Int. J. Nanomed., 2017, 12, 2645–2656 CrossRef CAS PubMed.
- Y. Zhou, J. Xiao, X. Ma, Q. Wang and Y. Zhang, Appl. Microbiol. Biotechnol., 2018, 102, 5299–5308 CrossRef CAS PubMed.
- S. Wachiralurpan, T. Sriyapai, S. Areekit, P. Sriyapai, S. Augkarawaritsawong, S. Santiwatanakul and K. Chansiri, Front. Chem., 2018, 6, 90 CrossRef.
- R. Kumvongpin, P. Jearanaikool, C. Wilailuckana, N. Sae-Ung, P. Prasongdee, S. Daduang, M. Wongsena, P. Boonsiri, W. Kiatpathomchai, S. S. Swangvaree, A. Sandee and J. Daduang, J. Virol. Methods, 2016, 234, 90–95 CrossRef CAS.
- R. Suebsing, P. Prombun and W. Kiatpathomchai, Lett. Appl. Microbiol., 2013, 56, 428–435 CrossRef CAS PubMed.
- W. Jaroenram, N. Arunrut and W. Kiatpathomchai, J. Virol. Methods, 2012, 186, 36–42 CrossRef CAS.
- N. Arunrut, J. Kampeera, S. Sirithammajak, P. Sanguanrut, P. Proespraiwong, R. Suebsing and W. Kiatpathomchai, PLoS One, 2016, 11, e0151769 CrossRef PubMed.
- N. Arunrut, J. Kampeera, R. Suebsing and W. Kiatpathomchai, J. Virol. Methods, 2013, 193, 542–547 CrossRef CAS PubMed.
- K. Watthanapanpituck, W. Kiatpathomchai, E. Chu and N. Panvisavas, J. Leg. Med., 2014, 128, 923–931 CrossRef.
- F. F. Carlos, B. Veigas, A. S. Matias, G. Doria, O. Flores and P. V. Baptista, Biotechnology Reports, 2017, 16, 21–25 CrossRef PubMed.
- Y. Lu, X. Ma, J. Wang, N. Sheng, T. Dong, Q. Song, J. Rui, B. Zou and G. Zhou, Biosens. Bioelectron., 2017, 90, 388–393 CrossRef CAS PubMed.
- Y. Chen, N. Cheng, Y. Xu, K. Huang, Y. Luo and W. Xu, Biosens. Bioelectron., 2016, 81, 317–323 CrossRef CAS PubMed.
- A. B. Nurul Najian, E. A. R. Engku Nur Syafirah, N. Ismail, M. Mohamed and C. Y. Yean, Anal. Chim. Acta, 2016, 903, 142–148 CrossRef CAS PubMed.
- J. H. Jung, S. J. Oh, Y. T. Kim, S. Y. Kim, W.-J. Kim, J. Jung and T. S. Seo, Anal. Chim. Acta, 2015, 853, 541–547 CrossRef CAS PubMed.
- Y. Wang, H. Li, Y. Wang, L. Zhang, J. Xu and C. Ye, Front. Microbiol., 2017, 8, 192 CrossRef.
- J. Yu, F. Wang, X. Zhan, X. Wang, F. Zuo, Y. Wei, J. Qi and Y. Liu, Anal. Bioanal. Chem., 2019, 411, 647–658 CrossRef CAS.
- E. A. Phillips, T. J. Moehling, S. Bhadra, A. D. Ellington and J. C. Linnes, Anal. Chem., 2018, 90, 6580–6586 CrossRef CAS.
- P. C. Foo, Y. Y. Chan, M. Mohamed, W. K. Wong, A. B. Nurul Najian and B. H. Lim, Anal. Chim. Acta, 2017, 966, 71–80 CrossRef CAS.
- H. Y. Yin, T. J. Fang and H. W. Wen, Lett. Appl. Microbiol., 2016, 63, 16–24 CrossRef CAS PubMed.
- P. Prompamorn, P. Sithigorngul, S. Rukpratanporn, S. Longyant, P. Sridulyakul and P. Chaivisuthangkura, Lett. Appl. Microbiol., 2011, 52, 344–351 CrossRef CAS PubMed.
- W. Jaroenram, W. Kiatpathomchai and T. W. Flegel, Mol. Cell. Probes, 2009, 23, 65–70 CrossRef CAS PubMed.
- J. R. Choi, J. Hu, Y. Gong, S. Feng, A. Wan, W. Abu Bakar, B. Pingguan-Murphy and F. Xu, Analyst, 2016, 141, 2930–2939 RSC.
- L. A. Rigano, F. Malamud, I. G. Orce, M. P. Filippone, M. R. Marano, A. M. Do Amaral, A. P. Castagnaro and A. A. Vojnov, BMC Microbiol., 2014, 14, 86 CrossRef PubMed.
- L. A. Rigano, M. R. Marano, A. P. Castagnaro, A. M. Do Amaral and A. A. Vojnov, BMC Microbiol., 2010, 10, 176 CrossRef.
- Y. Ge, B. Wu, X. Qi, K. Zhao, X. Guo, Y. Zhu, Y. Qi, Z. Shi, M. Zhou, H. Wang and L. Cui, PLoS One, 2013, 8, e69941 CrossRef CAS PubMed.
- K. Roskos, A. I. Hickerson, H.-W. Lu, T. M. Ferguson, D. N. Shinde, Y. Klaue and A. Niemz, PLoS One, 2013, 8, e69355 CrossRef CAS PubMed.
- Y.-L. Sun, C.-H. Yen and C.-F. Tu, J. Vet. Med. Sci., 2014, 76, 509–516 CrossRef CAS PubMed.
- Z. Liu, Q. Zhang, N.-N. Yang, M.-G. Xu, J.-F. Xu, M.-L. Jing, W.-X. Wu, Y.-D. Lu, F. Shi and C.-F. Chen, J. Microbiol. Biotechnol., 2019, 29, 454–464 CrossRef PubMed.
- T. Kaewphinit, N. Arunrut, W. Kiatpathomchai, S. Santiwatanakul, P. Jaratsing and K. Chansiri, BioMed Res. Int., 2013, 2013, 926230 Search PubMed.
- Z. K. Njiru, Diagn. Microbiol. Infect. Dis., 2011, 69, 205–209 CrossRef CAS.
- J. Zhang, J. Cao, M. Zhu, M. Xu and F. Shi, World J. Microbiol. Biotechnol., 2019, 35, 31 CrossRef PubMed.
- D. Liu, W. He, M. Jiang, B. Zhao, X. Ou, C. Liu, H. Xia, Y. Zhou, S. Wang, Y. Song, Y. Zheng, Q. Chen, J. Fan, G. He and Y. Zhao, AMB Express, 2019, 9, 11 CrossRef PubMed.
- R. A. Waters, V. L. Fowler, B. Armson, N. Nelson, J. Gloster, D. J. Paton and D. P. King, PLoS One, 2014, 9, e105630 CrossRef PubMed.
- B. H. Park, S. J. Oh, J. H. Jung, G. Choi, J. H. Seo, D. H. Kim, E. Y. Lee and T. S. Seo, Biosens. Bioelectron., 2017, 91, 334–340 CrossRef CAS PubMed.
- A. B. Nurul Najian, P. C. Foo, N. Ismail, L. Kim-Fatt and C. Y. Yean, Mol. Cell. Probes, 2019, 44, 63–68 CrossRef CAS PubMed.
- J. Connolly and T. G. St Pierre, J. Magn. Magn. Mater., 2001, 225, 156–160 CrossRef CAS.
- B. Tian, Z. Qiu, J. Ma, T. La Zardán Gómez de Torre, C. Johansson, P. Svedlindh and M. Strömberg, Biosens. Bioelectron., 2016, 86, 420–425 CrossRef CAS PubMed.
- H. Ravan and R. Yazdanparast, Anal. Biochem., 2013, 439, 102–108 CrossRef CAS PubMed.
- H. Ravan and R. Yazdanparast, Anal. Chim. Acta, 2012, 733, 64–70 CrossRef CAS.
- P. Prakrankamanant, C. Leelayuwat, C. Promptmas, T. Limpaiboon, S. Wanram, P. Prasongdee, C. Pientong, J. Daduang and P. Jearanaikoon, Biosens. Bioelectron., 2013, 40, 252–257 CrossRef CAS.
- K. Imai, N. Tarumoto, K. Misawa, L. Runtuwene, J. Sakai, K. Hayashida, Y. Eshita, R. Maeda, J. Tuda, T. Murakami, S. Maesaki, Y. Suzuki, J. Yamagishi and T. Maeda, BMC Infect. Dis., 2017, 17(1), 621 CrossRef.
- K. Imai, N. Tarumoto, K. Amo, M. Takahashi, N. Sakamoto, A. Kosaka, Y. Kato, K. Mikita, J. Sakai, T. Murakami, Y. Suzuki, S. Maesaki and T. Maeda, Parasitol. Int., 2018, 67, 34–37 CrossRef CAS.
- J. Yamagishi, L. R. Runtuwene, K. Hayashida, A. E. Mongan, L. A. N. Thi, L. N. Thuy, C. N. Nhat, K. Limkittikul, C. Sirivichayakul, N. Sathirapongsasuti, M. Frith, W. Makalowski, Y. Eshita, S. Sugano and Y. Suzuki, Sci. Rep., 2017, 7, 3510 CrossRef.
- K. Imai, N. Tarumoto, L. R. Runtuwene, J. Sakai, K. Hayashida, Y. Eshita, R. Maeda, J. Tuda, H. Ohno, T. Murakami, S. Maesaki, Y. Suzuki, J. Yamagishi and T. Maeda, Malar. J., 2018, 17, 217 CrossRef.
- A. S. Patterson, D. M. Heithoff, B. S. Ferguson, H. T. Soh, M. J. Mahan and K. W. Plaxco, Appl. Environ. Microbiol., 2013, 79, 2302–2311 CrossRef CAS.
- N. Nakamura, K. Ito, M. Takahashi, K. Hashimoto, M. Kawamoto, M. Yamanaka, A. Taniguchi, N. Kamatani and N. Gemma, Anal. Chem., 2007, 79, 9484–9493 CrossRef CAS.
- N. Nakamura, K. Ito, M. Takahashi, S. Hongo, K. Hashimoto, M. Kawamoto, A. Taniguchi, N. Kamatani and N. Gemma, Clin. Biochem., 2009, 42, 1158–1161 CrossRef CAS.
- K. Hashimoto and Y. Ishimori, Lab Chip, 2001, 1, 61–63 RSC.
- W. Sun, P. Qin, H. Gao, G. Li and K. Jiao, Biosens. Bioelectron., 2010, 25, 1264–1270 CrossRef CAS PubMed.
- G. V. Soraya, J. Chan, T. C. Nguyen, D. H. Huynh, C. D. Abeyrathne, G. Chana, M. Todaro, E. Skafidas and P. Kwan, Biosens. Bioelectron., 2018, 111, 174–183 CrossRef CAS PubMed.
- H. J. Chun, S. Kim, Y. D. Han, K. R. Kim, J.-H. Kim, H. Yoon and H. C. Yoon, ACS Sens., 2018, 3, 2261–2268 CrossRef CAS PubMed.
- Y. D. Han, H.-S. Kim, Y. M. Park, H. J. Chun, J.-H. Kim and H. C. Yoon, ACS Appl. Mater. Interfaces, 2016, 8, 10767–10774 CrossRef CAS PubMed.
- K. Nawattanapaiboon, W. Kiatpathomchai, P. Santanirand, A. Vongsakulyanon, R. Amarit, A. Somboonkaew, B. Sutapun and T. Srikhirin, Biosens. Bioelectron., 2015, 74, 335–340 CrossRef CAS PubMed.
- H. H. Nguyen, J. Park, S. Kang and M. Kim, Sensors, 2015, 15, 10481–10510 CrossRef CAS PubMed.
- H. Šípová, M. Piliarik, M. Vala, K. Chadt, P. Adam, M. Bocková, K. Hegnerová and J. Homola, Procedia Eng., 2011, 25, 148–151 CrossRef.
- S. D. Soelberg, T. Chinowsky, G. Geiss, C. B. Spinelli, R. Stevens, S. Near, P. Kauffman, S. Yee and C. E. Furlong, J. Ind. Microbiol. Biotechnol., 2005, 32, 669–674 CrossRef CAS.
- X. Zhi, M. Deng, H. Yang, G. Gao, K. Wang, H. Fu, Y. Zhang, D. Chen and D. Cui, Biosens. Bioelectron., 2014, 54, 372–377 CrossRef CAS PubMed.
- M. Bartosik, L. Jirakova, M. Anton, B. Vojtesek and R. Hrstka, Anal. Chim. Acta, 2018, 1042, 37–43 CrossRef CAS.
- M. Bartosik and L. Jirakova, Curr. Opin. Electrochem., 2019, 14, 96–103 CrossRef CAS.
- A. K. L. Yang, H. Lu, S. Y. Wu, H. C. Kwok, H. P. Ho, S. Yu, A. K. L. Cheung and S. K. Kong, Anal. Chim. Acta, 2013, 782, 46–53 CrossRef CAS PubMed.
-
Space systems - Definition of the Technology Readiness Levels (TRLs) and their criteria of assessment, 2014, ISO 16290:2014-12 Search PubMed.
- Clinical Laboratory Improvement Amendments (CLIA), available at: https://www.cms.gov/Regulations-and-Guidance/Legislation/CLIA/index.html.
- CLIA Categorizations, available at: https://www.fda.gov/medical-devices/ivd-regulatory-assistance/clia-categorizations.
- L. He and H.-s. Xu, Aquaculture, 2011, 311, 94–99 CrossRef CAS.
- T. M. Squires, R. J. Messinger and S. R. Manalis, Nat. Biotechnol., 2008, 26, 417–426 CrossRef CAS PubMed.
- J. Dostálek, H. Vaisocherová and J. Homola, Sens. Actuators, B, 2005, 108, 758–764 CrossRef.
- J. Homola, H. B. Lu, G. G. Nenninger, J. Dostálek and S. S. Yee, Sens. Actuators, B, 2001, 76, 403–410 CrossRef CAS.
- G. G. Nenninger, J. B. Clendenning, C. E. Furlong and S. S. Yee, Sens. Actuators, B, 1998, 51, 38–45 CrossRef CAS.
- P. K. Drain, E. P. Hyle, F. Noubary, K. A. Freedberg, D. Wilson, W. R. Bishai, W. Rodriguez and I. V. Bassett, Lancet Infect. Dis., 2014, 14, 239–249 CrossRef PubMed.
- D. Mabey, R. W. Peeling, A. Ustianowski and M. D. Perkins, Nat. Rev. Microbiol., 2004, 2, 231–240 CrossRef CAS.
- N. Phares, R. J. White and K. W. Plaxco, Anal. Chem., 2009, 81, 1095–1100 CrossRef CAS PubMed.
- L. Civit, A. Fragoso and C. K. O'Sullivan, Electrochem. Commun., 2010, 12, 1045–1048 CrossRef CAS.
- Y. Liu, Q. Liu, S. Chen, F. Cheng, H. Wang and W. Peng, Sci. Rep., 2015, 5, 12864 CrossRef CAS PubMed.
- Z. Wei, X. Xiao, J. Wang and H. Wang, Sensors, 2017, 17, 2500 CrossRef PubMed.
- J. Jiang, X. Wang, R. Chao, Y. Ren, C. Hu, Z. Xu and G. L. Liu, Sens. Actuators, B, 2014, 193, 653–659 CrossRef CAS.
- D. Zhang, J. Jiang, J. Chen, Q. Zhang, Y. Lu, Y. Yao, S. Li, G. Logan Liu and Q. Liu, Biosens. Bioelectron., 2015, 70, 81–88 CrossRef CAS PubMed.
|
This journal is © The Royal Society of Chemistry 2020 |