DOI:
10.1039/D4NJ00624K
(Paper)
New J. Chem., 2024,
48, 6571-6581
Neat and rapid preparation of hydrophobic magnetic ionic liquids composed of transition metal chelates featuring in situ formation capabilities in aqueous matrices†
Received
5th February 2024
, Accepted 6th March 2024
First published on 6th March 2024
Abstract
Magnetic ionic liquids (MILs) form a subclass of ionic liquids (ILs) that possess paramagnetic properties and can respond to an external magnetic field, facilitating ease of manipulation in immiscible solvents. Despite being popular as solvents in catalysis, organic synthesis, and separations, MILs are obtained through complex and labor-intensive synthetic/purification routes that increase cost, are time-consuming, and require expertise in organic synthesis. To date, no study has successfully developed a straightforward and rapid procedure for MILs that also eliminates purification. In this study, transition metal-containing bis[(trifluoromethyl)sulfonyl]imide ([NTf2−]) salts and N-alkylimidazoles, diglycolamides, and O-donor ligands were used to produce hydrophobic MILs that could maintain their chemical integrity under water for over 6 months. A neat heat/stir method was employed to form MILs for long-term storage or later use while an in situ method was used for select combinations to generate the same MIL under 30 s in the bulk presence of water. Viscosities as low as 198.3 cP at 22.8 °C were obtained that were comparable to previous classes of paramagnetic solvents. In contrast to MILs comprised of O-donor ligands, those formed with alkylimidazoles and diglycolamides were found to be soluble in non-polar solvents such as hexane at concentrations of up to 50% (w/v) MIL-to-solvent ratio while being insoluble in water even at 0.01% (w/v). Effective magnetic moment values for MILs ranged from 2.78 to 5.16 Bohr magnetons (μB) and were observed to be dependent on the metal center in the [NTf2−] salts. The solvents possessed excellent thermal stabilities with diglycolamide-based MILs exhibiting structural resilience up to 345 °C. The synthetic design of these MILs has successfully achieved the primary objective of facilitating easy access to magnetoactive solvents by reducing their preparation to a simple step consisting of mixing two readily available reagents to expedite production in high-throughput laboratories where these compounds can be incorporated in automated separations and analytical testing systems where their magnetic properties can be conveniently exploited.
1. Introduction
Ionic liquids (ILs) are popular molten salts that are employed as green solvents in chemical separations and synthetic methodologies.1,2 Their typical chemical structure comprises an organic cation paired with a weakly coordinating anion that yields a conductive liquid having a melting point <100 °C.2,3 ILs exhibit desirable characteristics such as high chemical and thermal stability,4,5 negligible vapor pressure,1,4 tunable viscosities,6 and catalytic properties.7 Consequently, they are often used in applications such as organic reactions,1,8 CO2 capture,1,9 nucleic acid analysis,10 and electrochemistry.11 Incorporating a paramagnetic metal center in either the cation or anion and/or both produces magnetic ionic liquids (MILs) that can respond to an external magnetic field.12,13 MILs possess similar physio-chemical properties to ILs and can be easily manipulated when placed in various matrixes using a hand-held magnet.13,14
The design and development of MILs has undergone vast advancements over the years to produce unique combinations having distinct chemical structures.15,16 The first reported MIL comprised of 1-butyl-3-methylimidazolium tetrachloroferrate(III) ([C4mim+][FeCl4−]) provided the stepping stone,17 but offered limited chemical stability in aqueous environments.18,19 Since then, meticulous MIL design has enabled the possibility of integrating specific functional groups into the chemical structures of these solvents to impart desired characteristics.20,21 For example, exchanging halide anions to the bis[(trifluoromethyl)sulfonyl]imide ([NTf2−]) anion results in lowered MIL viscosity and improves thermal stability,22 while embedding trifluoromethyl moieties (–CF3) in the cation/anion enhances solvent hydrophobicity.13,22 Utilizing anions comprised of hexafluoroacetylacetonate ligands ([hfacac−])23 have enabled the formation of MILs containing multiple paramagnetic metal centers to produce combinations exhibiting stronger magnetic properties, higher water immiscibility, and robust thermal and chemical stabilities.24,25 Clearly, targeted modifications made in the design of these solvents have yielded several generations of MILs and profoundly demonstrate their designer aspect.
Given the complex chemical design of MILs, some of their components are acquired from commercial sources while others can be prepared using established synthetic routes. For example, tetrafluoroborate ([BF4−]), hexafluorophosphate ([PF6−]), and [NTf2−] anions are procured as alkali metal salts (i.e., Na+ and Li+)9,26 while ligands used to form the cations are often synthesized using cheap and readily accessible reagents.24,27 Although the synthetic methodologies used to prepare MILs and/or their starting materials are extensively reported in the literature, the ability to effectively reproduce these reported reaction schemes requires sufficient proficiency in organic/organometallic chemistry. Additionally, multistep synthesis and purification protocols employed in MIL preparation are time-consuming and often involve the use of dedicated glassware and lab equipment (i.e., rotary evaporator, reflux assembly, etc.) that may not be readily available to the end user of these solvents. Despite offering a non-volatile alternative to conventional organic solvents, MIL synthesis requires extensive purification steps that employ traditional solvents to remove unreacted species and by-products. Such factors can potentially hinder the mass adoption of MILs as extractants or reaction media in resource-limited settings.
In search of an improved class of sustainable solvents, green alternatives to ILs and MILs have gained widespread interest in the scientific community. One popular alternative is deep eutectic solvents (DESs) which are homogenous mixtures comprised of a hydrogen bond (H-bond) acceptor (HBA) and H-bond donor (HBD)28,29 capable of undergoing multiple types of intermolecular forces, including H-bonding, that result in a decrease in the melting point of the eutectic mixture.29,30 DESs typically offer biodegradability,28,31 low toxicity,28 and ease of preparation28,32 and can be formed using either the grinding or heat/stir method without subsequent purification steps.29,33 Additionally, paramagnetic metal centers can be impregnated in the HBAs to produce hydrophobic magnetic DESs (HMDESs).34,35 Given these desirable characteristics, DESs are increasingly employed in catalysis,36,37 organic synthesis,38 and chemical separations.39 However, their usefulness is often met with challenges in applications that require enhanced thermal and chemical stability. Hydrogen bonding between the HBA and HBD is adversely impacted at temperatures >70 °C40 or in the presence of a co-solvent above a certain concentration.41,42 Above all, DES components tend to leach into water over extended periods of time, thereby compromising their overall structural integrity.43
Despite possessing tremendous potential, DESs/HMDESs are clearly not viable replacements for ILs/MILs. Nevertheless, they demonstrate that non-traditional solvents can be prepared directly by mixing two requisite starting materials and requiring minimal or no synthetic effort. Although MILs have undergone massive advancements in their chemical design and synthetic strategy, no study has yet focused on simplifying their preparation with the aim of minimizing costs while ensuring high atom economy. To produce MILs with preparation methods used for HMDESs, it is essential that the reagents do not undergo chemical reactions to generate by-products29 but rather interact with each other to form pure stable cation/anion pairs when mixed. MILs comprised of [NTf2−] anions paired with cations formed using transition metals coordinated to N-alkylimidazole ligands offer a unique class of hydrophobic magnetoactive solvents having relatively low viscosities and tremendous potential for structural tunability.22 However, they are obtained through a two-pot synthetic scheme involving multiple purification steps and conversion of the halide-based precursor via an anion exchange mechanism. Employing transition metal-based [NTf2−] salts could eliminate the need for a halide-based intermediate salt and simplify MIL formation to a one-pot metal–ligand coordination reaction. Even though [Co2+][NTf2−]2 salts have been known to form inorganic complexes with monodentate ligands,44,45 these materials have never been explored in optimizing a neat synthetic route to obtain hydrophobic MILs that is as simple as mixing HBAs and HBDs to form HMDESs.
In this study, N- and O-donor ligands comprised of alkylimidazoles, tertiary (3°) amides, and diglycolamides were employed to form transition metal chelates in the synthetic preparation of 12 MILs. For the first time, these paramagnetic solvents were prepared using a neat synthesis route and their physico-chemical properties were thoroughly characterized and compared to previous classes of MILs. While MILs comprised of O-donor ligands solubilized in water, those formed with imidazoles and diglycolamides were observed to maintain their unique immiscibility in aqueous samples for over 6 months, be easily dispersed in water, and exhibit a low tendency of sticking to the walls of glass containers. In addition, diglycolamide and imidazole-based MILs can be rapidly formed in situ by solubilizing transition metal-based [NTf2−] salts and cationic ligands in water. Viscosities as low as 198.3 cP at 22.8 °C were obtained for these MILs while many combinations were found to be thermally stable at temperatures up to 345 °C. The distinct design of these MILs utilizes inexpensive reagents, significantly reduces time-consuming synthetic steps, and eliminates the need for organic solvents as reaction media or in purification steps, making their preparation faster and more energy efficient. These solvents represent one of the most green, versatile, and sustainable classes of magnetoactive designer solvents amongst MILs and HMDESs, making them promising candidates for applications where their magnetic properties can be readily exploited.
2. Experimental
2.1. Instrumentation and equipment
Nuclear magnetic resonance (NMR) spectroscopy was employed to characterize the compounds in this study using a Bruker 600 MHz spectrometer. Elemental analysis was conducted using a Thermo Scientific FlashSmart 2000 CHNS/O Combustion Elemental Analyzer (Waltham, MA, USA). A Brookfield DV1 cone and plate viscometer equipped with a CPA-51Z cone spindle was used to perform viscosity measurements at 22.8 °C using approximately 0.8 mL sample of each MIL after thoroughly drying for 48 h under vacuum. Magnetic properties were studied using a magnetic susceptibility balance (MSB, Sherwood Scientific, Cambridge, UK) and a Quantum Design Superconducting Quantum Interference Device (SQUID) magnetometer (MPMS XL-7). Thermal stability of MILs was analyzed by conducting thermogravimetric analysis (TGA) using a SETARAM Labsys Evo thermal analyzer (KEP Technologies, TX, USA). X-ray diffraction (XRD) analysis of metal salts was performed using a Philips X’Pert Pro MPD X-ray diffractometer (Goniometer = PW3050/60, Almelo, Netherlands). Differential scanning calorimetric (DSC) measurements were conducted using a Discovery DSC-250 instrument equipped with an autosampler and an RCS40 refrigeration cooling system (TA Instruments, DE, USA). All DSC data was analyzed using TA universal analysis software (TA Instruments).
2.2. Synthesis and characterization of transition metal-based [NTf2−] salts
A comprehensive list of reagents and starting materials is provided in the ESI.† All metal-containing [NTf2−] salts of Co2+ and Ni2+ were obtained through a previously reported synthesis scheme.44 In a 250 mL round bottom flask, hydrogen bis[(trifluoromethyl)sulfonyl]imide ([H+][NTf2−], 100 mmol) was added dropwise to 75 mmol (excess) of transition metal carbonates (CoCO3 and NiCO3) suspended in 50 mL of water. The resulting mixture was stirred under ambient conditions for 5 h and the contents of the flask were then filtered under gravity. Unreacted CoCO3/NiCO3 was collected as the residue and water was removed from the filtrate under reduced pressure using rotary evaporation to obtain [Co(H2O)6][NTf2]2 and [Ni(H2O)6][NTf2]2 salts. Hydrated transition metal-based [NTf2−] salts were subsequently dried under vacuum for 24 h prior to further characterization. All Co2+ and Ni2+ salts were examined using CHN elemental analysis for purity determination. In addition, XRD measurements were conducted on the hydrated metal salts to investigate their crystal lattice; detailed discussion of XRD patterns is provided in the ESI† (Fig. S1 and S2).
Characterization of hydrated metal-containing [NTf2−] salts.
[Co(H2O)6][NTf2]2 salt.
Pink amorphous powder. Elemental analysis calculated (%) for CoC4H12N2S4F12O14: C, 6.61; H, 1.66; N, 3.85. Found: C, 7.58; H, 1.24; N, 4.69.
[Ni(H2O)6][NTf2]2 salt.
Green amorphous powder. Elemental analysis calculated (%) for NiC4H12N2S4F12O14: C, 6.61; H, 1.66; N, 3.85. Found: C, 7.59; H, 1.23; N, 5.15.
2.3. Preparation and characterization of multidentate chelating cationic ligand
All monodentate cationic ligands comprised of alkylimidazoles and 3° amides were obtained from commercial sources while diglycolamide was prepared using a previously reported synthetic route.24 Diglycolyl chloride (10 mmol) was placed in a 100 mL round bottom flask and solubilized in 40 mL of diethyl ether. Triethylamine (45 mmol) was mixed with 20 mmol of dioctylamine and added dropwise to the contents of the flask. The resulting solution was stirred at room temperature for 5 h prior to being washed thrice with 40 mL of 10% HCl solution and water in a 250 mL separatory funnel. The organic layer was further washed with 40 mL of 5% (w/w) NaHCO3 solution and filtered under gravity to remove residual solids. Diethyl ether was removed under reduced pressure and the product was dried under vacuum for 48 h before being used in MIL synthesis. The following multidentate cationic ligand was prepared and characterized using 13C and 1H NMR; spectra are provided in the ESI.†
NMR analysis of cationic ligand N,N,N′,N′-tetraoctyl diglycolamide (TODGA).
A pale yellow liquid. Yield of 93%. 1H NMR (400 MHz, cdcl3) δ 4.31 (s, 3H), 3.34–3.22 (m, 4H), 3.22–3.09 (m, 4H), 1.60–1.43 (m, 8H), 1.33–1.18 (m, 40H), 0.86 (td, J = 6.8, 3.3 Hz, 12H). 13C NMR (101 MHz, CDCl3) δ 168.45, 77.27, 69.09, 46.92, 45.76, 31.80, 31.75, 29.51, 29.36, 29.32, 29.24, 29.20, 28.96, 28.79, 27.60, 27.41, 27.16, 27.03, 26.99, 26.96, 26.82, 22.66, 22.63, 22.61, 14.07, 14.05.
2.4. Neat heat and stir method for MIL preparation and characterization
A solvent-free one-pot synthesis approach was employed to prepare all MILs. [Co(H2O)6][NTf2]2 or [Ni(H2O)6][NTf2]2 salt (1 mmol) was placed in a 20 mL glass vial and mixed with 6 mmol of alkyimidazoles and 3° amides at low heat setting (<50 °C) to produce MILs 1 to 10 (Fig. 1A). A homogenous liquid was obtained over a duration of 12 h and water vapor was observed on the inner wall of the glass vial. The resulting product was dried under vacuum for 24 h to remove residual water introduced in the MIL from the hydrated salts. All MILs were further stirred for 1 h at room temperature to obtain the final neat product and Fig. 2A provides a pictorial representation of the heat/stir preparation method. In the case of MILs 11 and 12, 1 mmol of Co2+ and Ni2+ salts were mixed with 2 mmol of TODGA (Fig. 1B). The remaining synthetic procedure was identical to that employed for MILs comprised of monodentate ligands. The purity of all MILs was characterized using CHN elemental analysis.
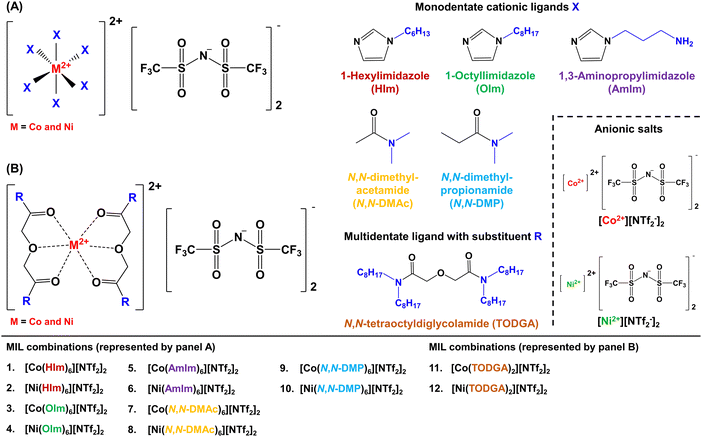 |
| Fig. 1 Numbering scheme and chemical structures used for MILs examined in this study. Abbreviations: 1-hexylimidazole (HIm), 1-octylimidazole (OIm), 1,3-aminopropylimidazole (AmIm), N,N-dimethylacetamide (N,N-DMac), N,N-dimethylpropionamide (N,N-DMP), N,N,N′,N′-tetraoctyl diglycolamide (TODGA), cobalt bis(trifluoromethanesulfonyl)imide hexahydrate ([Co(H2O)6][NTf2]2), and nickel bis(trifluoromethanesulfonyl)imide hexahydrate ([Ni(H2O)6][NTf2]2). (A) Chemical structure of MILs comprised of monodentate cationic ligands and (B) chemical structure of MILs prepared using diglycolamide ligand, respectively. | |
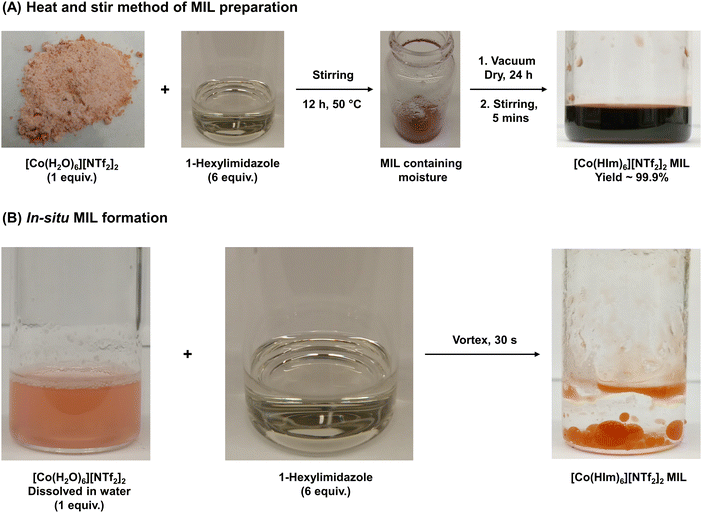 |
| Fig. 2 Pictorial representation of the (A) neat heat/stir method of MIL preparation and (B) the in situ formation of MILs under water. | |
Characterization of MILs prepared using neat method.
MIL 1.
Dark red, non-viscous liquid. Elemental analysis calculated (%) for CoC58H96N14S4F12O8: C, 45.45; H, 6.31; N, 12.79. Found: C, 44.50; H, 6.50; N, 12.19.
MIL 2.
Royal blue, non-viscous liquid. Elemental analysis calculated (%) for NiC58H96N14S4F12O8: C, 45.46; H, 6.31; N, 12.80. Found: C, 45.03; H, 6.65; N, 12.56.
MIL 3.
Dark purple, non-viscous liquid. Elemental analysis calculated (%) for CoC70H120N14S4F12O8: C, 49.43; H, 7.11; N, 11.53. Found: C, 49.81; H, 7.78; N, 10.69.
MIL 4.
Royal blue, non-viscous liquid. Elemental analysis calculated (%) for NiC70H120N14S4F12O8: C, 49.44; H, 7.11; N, 11.53. Found: C, 51.80; H, 8.09; N, 10.68.
MIL 5.
Dark red, non-viscous liquid. Elemental analysis calculated (%) for CoC40H70N20S4F12O10: C, 34.16; H, 5.02; N, 19.92. Found: C, 34.32; H, 5.89; N, 19.06.
MIL 6.
Bright blue, non-viscous liquid. Elemental analysis calculated (%) for NiC40H70N20S4F12O10: C, 34.17; H, 5.02; N, 19.92. Found: C, 34.41; H, 6.28; N, 19.01.
MIL 7.
Light blue, non-viscous liquid. Elemental analysis calculated (%) for CoC28H66N8S4F12O20: C, 26.90; H, 5.32; N, 8.96. Found: C, 27.69; H, 5.28; N, 8.97.
MIL 8.
Pale green, non-viscous liquid. Elemental analysis calculated (%) for NiC28H66N8S4F12O20: C, 26.90; H, 5.32; N, 8.96. Found: C, 27.40; H, 5.27; N, 8.75.
MIL 9.
Dark blue, non-viscous liquid. Elemental analysis calculated (%) for CoC30H78N8S4F12O20: C, 28.02; H, 6.11; N, 8.71. Found: C, 29.07; H, 5.07; N, 8.01.
MIL 10.
Light green, non-viscous liquid. Elemental analysis calculated (%) for NiC30H78N8S4F12O20: C, 28.02; H, 6.11; N, 8.71. Found: C, 28.22; H, 5.23; N, 7.53.
MIL 11.
Pink, non-viscous liquid. Elemental analysis calculated (%) for CoC76H144N6S4F12O14: C, 51.25; H, 8.15; N, 4.72. Found: C, 51.67; H, 8.47; N, 4.75.
MIL 12.
Green, non-viscous liquid. Elemental analysis calculated (%) for NiC76H144N6S4F12O14: C, 51.26; H, 8.15; N, 4.72. Found: C, 51.28; H, 8.94; N, 4.65.
2.5.
In situ formation of hydrophobic MILs in an aqueous matrix using neat reagents
To spontaneously produce MILs under aqueous conditions, 0.1 mmol of hydrated [NTf2−] Co2+ and Ni2+ salts were solubilized in 5 mL of water in 10 mL glass vials and the resulting solution was stirred at 200 rpm. Alkylimidazoles (0.6 mmol) or TODGA (0.2 mmol) were added to the glass vials to immediately yield a stable water-immiscible MIL phase that settled at the bottom of the container. Water was decanted to isolate the MIL layer for purity analysis and a pictorial representation of the in situ synthetic route is provided in Fig. 2B. All MILs were dried under vacuum for 24 h before characterizing them using CHN elemental analysis.
Characterization of MILs prepared using the in situ approach.
MIL 1.
Dark red, non-viscous liquid. Elemental analysis calculated (%) for CoC58H96N14S4F12O8: C, 45.45; H, 6.31; N, 12.79. Found: C, 45.14; H, 7.25; N, 11.71.
MIL 2.
Royal blue, non-viscous liquid. Elemental analysis calculated (%) for NiC58H96N14S4F12O8: C, 45.46; H, 6.31; N, 12.80. Found: C, 45.50; H, 7.55; N, 12.35.
MIL 3.
Dark purple, non-viscous liquid. Elemental analysis calculated (%) for CoC70H120N14S4F12O8: C, 49.43; H, 7.11; N, 11.53. Found: C, 49.83; H, 7.88; N, 10.52.
MIL 4.
Royal blue, non-viscous liquid. Elemental analysis calculated (%) for NiC70H120N14S4F12O8: C, 49.44; H, 7.11; N, 11.53. Found: C, 49.84; H, 8.02; N, 10.21.
3. Results and discussion
3.1. Designing highly tunable, sustainable, and cost-effective cationic/anionic precursors to rapidly produce pure hydrophobic MILs
To achieve drastic improvements in MIL design, it was important to incorporate chemical features in cationic/anionic precursors that can result in MIL formation upon mixing. MILs typically have cationic and anionic components synthesized as two separate species that are paired together through a counterion exchange reaction, which can generate by-products that are removed via liquid–liquid purification techniques. It was essential to simplify the MIL preparation down to two reagents: (1) a paramagnetic metal-containing salt and (2) suitable ligands for the cation that can combine to produce MILs without needing purification. The [Co(H2O)6][NTf2]2 and [Ni(H2O)6][NTf2]2 salts provide a stable cation/anion pair, are cheap and easy to prepare in bulk, eliminate the need for an anion exchange step, and can readily form coordination complexes with a wide variety of ligands. These characteristics strongly influenced the choice of paramagnetic salt employed to obtain MILs prepared in this study.
To produce a wide variety of MIL combinations, various types of cationic ligands were identified. Alkylimidazoles and 3° amides known to yield organometallic complexes with transition metal-based [NTf2−] salts were selected to form MILs and the alkyl substituents on these monodentate ligands were modulated to investigate their impact on the physico-chemical properties (i.e., viscosity and thermal stability) of these solvents. Additionally, O-donor ligands were explored to incorporate multiple coordination sites to form metal–organic complexes in an effort to investigate the effect of heteroatoms on MIL properties. To further introduce variations in MIL design, multidentate TODGA ligands were also pursued as these can form strong chelates and result in chemically/thermally stable compounds.
3.2. Optimizing the synthetic route for neat and in situ MIL preparation of water-stable solvents
To design a neat and completely solvent-free MIL synthetic route, it was essential to eliminate the use of organic solvents in every capacity. Most synthetic schemes require an organic solvent as reaction medium to solubilize the reactants for them to thoroughly react and form the desired product. However, it was determined that the ligands explored in this study could readily react with metal [NTf2−] salts, thereby enabling the reaction to proceed under neat conditions with mild heat. Although most reagents were stirred for approximately 12 h to produce MILs (Fig. 2A), the reaction time can be further shortened by employing higher heat and stir rates. Given the use of hydrated transition metal-based [NTf2−] salts, an additional drying step was necessary, which can be eliminated by substituting with anhydrous reagents.
Despite simplifying the synthesis of MILs, the heat/stir method lacked the ability to rapidly produce MILs as and when needed. To further reduce preparation time, the differences in solubilities of reagents and MIL in water were exploited to develop an in situ approach. It was observed that most starting materials readily dissolved in water while the MIL formed a separate immiscible phase. As shown in Fig. 2B, vigorously mixing hydrophobic cationic ligands with aqueous solutions of metal [NTf2−] salts in the desired stoichiometry rapidly yielded MILs in <30 s. To provide evidence that the MILs formed using both methods were chemically identical, batches obtained using the in situ approach were characterized using CHN elemental analysis (Section 2.5) and benchmarked against those produced through heating and stirring (Section 2.4); the obtained data agreed using both reaction routes. Given the ability to withstand bulk water and maintain their chemical integrity, the long-term stability of MILs stored under aqueous condition was investigated. The [Co(HIm)6][NTf2]2 MIL was found to remain stable in water for over 6 months and produced no visible degradation, as observed from Fig. S3 (ESI†).
3.3. Effect of ligands and metal centers on the viscosity of [NTf2−]2 MILs
To investigate their ability to flow, the viscosity of 12 MILs was thoroughly screened using viscometry at 22.8 °C and all measurements are provided in Table 1. MILs exhibited a wide range of viscosities with the lowest values ranging from 198.3 to 308.2 cP obtained for combinations formed using the N,N-DMAc and N,N-DMP ligands. Among MILs comprised of imidazoles, those containing octyl substituents yielded slightly more viscous solvents (3317 to 4726 cP) compared to their hexyl-based homologs (2231 to 3068 cP) due to an increase in dispersion forces. However, a dramatic increase in viscosity was observed with the incorporation of the amino functional group in the [Co(AmIm)6][NTf2]2 and [Ni(AmIm)6][NTf2]2 MILs, which can be attributed to additional intermolecular interactions such as H-bonding. Although MILs formed using TODGA produced moderately high viscosities (7306 to 9284 cP), these solvents were only one-third as viscous as the [Co(TODGA)2][Co(hfacac)3]2 and [Ni(TODGA)2][Ni(hfacac)3]2 MILs made using the same multidentate cationic ligand. In addition, all Co2+ MILs exhibited lower viscosities than their Ni2+-based counterparts, irrespective of the type of ligand used.
Table 1 Viscosity measurements obtained at 22.8 °C and magnetic properties analyzed by SQUID and MSB for MILs formed using transition metal-based [NTf2−] salts
MIL No. |
MIL/salt abbreviation |
Viscosity (cP) |
μ
theor
(μB) |
μ
eff
(μB) |
Ref. |
Theoretical effective magnetic moment for high-spin complexes of transition metals.
Effective magnetic moment calculated using magnetic susceptibility measurements performed on a magnetic susceptibility balance.
MIL turns into a slurry when left to stand overnight.
MIL turns into an amorphous solid when left to stand overnight.
MILs that oscillate between high and low-spin complexes were reported.
Effective magnetic moment calculated using magnetic susceptibility measurements performed on a SQUID magnetometer.
Viscosity measurement reported at 23.7 °C.
Viscosity measurement reported at 19.9 °C.
|
1 |
[Co(HIm)6][NTf2]2 |
2231 |
4.3–5.2 |
4.54 |
Present work |
2 |
[Ni(HIm)6][NTf2]2 |
3068 |
2.9–3.3 |
2.81 |
Present work |
3 |
[Co(OIm)6][NTf2]2 |
3317c |
4.3–5.2 |
4.47 |
Present work |
4 |
[Ni(OIm)6][NTf2]2 |
4726c |
2.9–3.3 |
2.78 |
Present work |
5 |
[Co(AmIm)6][NTf2]2 |
52 920 |
4.3–5.2 |
4.67 |
Present work |
6 |
[Ni(AmIm)6][NTf2]2 |
68 742 |
2.9–3.3 |
2.80 |
Present work |
7 |
[Co(N,N-DMAc)6][NTf2]2 |
236.9 |
4.3–5.2 |
5.16 |
Present work |
8 |
[Ni(N,N-DMAc)6][NTf2]2 |
308.2 |
2.9–3.3 |
3.12 |
Present work |
9 |
[Co(N,N-DMP)6][NTf2]2 |
198.3 |
4.3–5.2 |
4.29 |
Present work |
10 |
[Ni(N,N-DMP)6][NTf2]2 |
256.8 |
2.9–3.3 |
3.01 |
Present work |
11 |
[Co(TODGA)2][NTf2]2 |
7306 |
4.3–5.2 |
4.65 |
Present work |
12 |
[Ni(TODGA)2][NTf2]2 |
9284 |
2.9–3.3 |
3.11 |
Present work |
— |
[Co(H2O)6][NTf2]2 salt |
— |
4.3–5.2 |
4.73 |
Present work |
— |
[Ni(H2O)6][NTf2]2 salt |
— |
2.9–3.3 |
3.19 |
Present work |
— |
[Co(BIm)4][Cl][NTf2] |
1256d |
4.3–5.2 |
2.47ef |
22
|
— |
[Ni(BIm)4][Cl][NTf2] |
2501d |
2.9–3.3 |
1.59ef |
22
|
— |
[Co(OIm)4][Cl][NTf2] |
152 |
4.3–5.2 |
3.24ef |
22
|
— |
[Ni(OIm)4][Cl][NTf2] |
680 |
2.9–3.3 |
1.47ef |
22
|
— |
[P66614][Co(hfacac)3] |
575.8g |
4.3–5.2 |
4.30f |
23
|
— |
[P66614][Ni(hfacac)3] |
927.9g |
2.9–3.3 |
2.80f |
23
|
— |
[Co(TODGA)2][Co(hfacac)3]2 |
28 310h |
8.30 |
8.03f |
24
|
— |
[Ni(TODGA)2][Ni(hfacac)3]2 |
33 830h |
5.37 |
4.71f |
24
|
3.4. Magnetic properties of MILs comprised of transition metals and [NTf2−]2 salts
As opposed to ILs, DESs, and organic solvents, MILs possess a paramagnetic component and respond to the presence of a hand-held magnet, allowing the user to manipulate and influence their motion in other non-miscible solvents. When an external magnetic field is brought near a paramagnetic rare-earth or transition metal, the unpaired electrons in its 3d or 4f orbitals undergo spin ordering that result in temporary magnetization of the metal center. The Curie–Weiss law defines a linear relationship between the inverse of magnetic susceptibility of magnetoactive materials and temperature from which the effective paramagnetic moment of a substance can be calculated.
A total of 12 MILs and 2 paramagnetic salts incorporating transition metals were investigated using a magnetic susceptibility balance (MSB) and a detailed description of equations/parameters used to analyze data are provided in the ESI.†Table 1 shows the theoretical and calculated μeff values (in μB/formula unit) for all solvents/reagents examined using MSB. The μeff values determined for MILs ranged between 2.78 and 5.16μB per formula unit and a statistical match was observed with theoretical values calculated for high-spin complexes of Co2+ and Ni2+ metals. This is in strong contrast with previous transition metal-based [NTf2−] MILs that oscillated between high and low spin states and exhibited limited magnetic susceptibility (see Table 1). Compared to a value of 2.78μB for the [Ni(OIm)6][NTf2]2 MIL, the Co2+-based solvent comprised of 1-octylimidazole ligand offered stronger magnetic susceptibility (4.47μB). The strength of magnetization was observed to be primarily dependent on the type of metal center as modulating the cationic ligand resulted in comparable μeff values of 2.81, 2.78, and 2.80μB for the [Ni(HIm)6][NTf2]2, [Ni(OIm)6][NTf2]2, and [Ni(AmIm)6][NTf2]2 MILs. Additionally, MILs comprised of mono and multidentate chelating ligands exhibited similar magnetic susceptibility, demonstrating that metal-to-ligand stoichiometry and coordination geometry have little influence on magnetic properties.
To develop a deeper understanding of their magnetic properties and investigate any possibility of magnetic transitions, MILs were further studied using a SQUID magnetometer. Fig. 3 demonstrates the magnetization of the [Co(OIm)6][NTf2]2 and [Ni(OIm)6][NTf2]2 MILs versus temperature. Both solvents produced a linear trendline passing through the origin for the reciprocal susceptibility (1/χ) plotted against temperature, indicating paramagnetic characteristics between T = 5–300 K. Similar results were observed for the [Co(HIm)6][NTf2]2 MIL, as shown in Fig. S4 (ESI†). In addition, the μeff values calculated from SQUID were comparable to those obtained using MSB, demonstrating the data from both techniques to be in strong agreement.
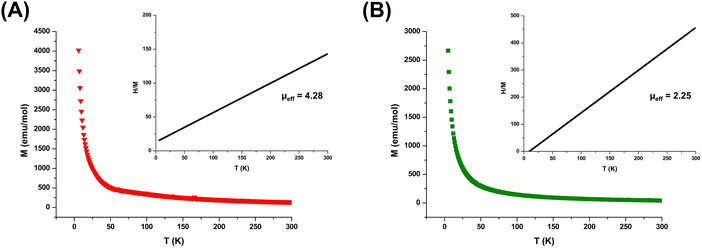 |
| Fig. 3 Magnetization of Co2+ and Ni2+-based MILs studied as a function of temperature under an applied magnetic field of H = 20 kOe: (A) [Co(OIm)6][NTf2]2 and (B) [Ni(OIm)6][NTf2]2. The inset shows the Curie–Weiss fit of the linear portion of the reciprocal susceptibility. | |
3.5. Miscibility and solvent compatibility of MILs with organic solvents
The solubility of MILs was investigated in volatile organic solvents commonly employed in laboratories. A 10 mg sample of each MIL was placed in a 1 mL glass vial containing 100 μL of a specific solvent and the contents were thoroughly stirred to prepare solutions at a concentration of 10% (w/v) MIL-to-solvent ratio. Additional concentration levels were also explored using the same preparation method and the solubility data for each MIL is provided in Table 2. MILs comprised of alkylimidazoles and TODGA ligands were insoluble in water at a concentration as low as 0.01% (w/v), indicating their hydrophobic properties. In contrast, O-donor ligands generally yielded MILs exhibiting hydrophilic characteristics with poor solubilities in non-polar solvents such as benzene and toluene. While MILs formed using imidazoles generally dissolved in most non-polar solvents, only TODGA-based MILs were soluble in hexane/heptane at concentrations as high as 50% (w/v) MIL-to-solvent ratio. In addition, all MILs were found to be soluble in polar solvents such as methanol and acetonitrile.
Table 2 Decomposition/degradation temperatures measured using TGA and solubility of [NTf2−]-based MILs investigated in 14 different polar and non-polar solvents at 3 different concentrations
MIL no. |
MIL/salt abbreviation |
Solubility |
Onset of MIL volatilizationa (°C) |
Peak decomposition temperatureb (°C) |
Ref. |
MIL degradation temperature measured using TGA at 5% weight loss.
MIL degradation temperature measured using TGA at highest rate of thermal decomposition monitored via DTG (derivative of TG).
MIL degradation temperature not reported using DTG.
MIL degradation temperature measured using a gas chromatograph (GC) equipped with flame ionization detector (FID).Sa = soluble in methanol, ethanol, acetone, acetonitrile, and isopropanol at 10% (w/v). Sb = soluble in benzene, toluene, diethyl ether, ethyl acetate, dichloromethane, and chloroform at 10% (w/v). Sc = soluble in methanol, ethanol, acetone, acetonitrile, isopropanol, benzene, toluene, diethyl ether, ethyl acetate, dichloromethane, and chloroform at 50% (w/v). Sd = insoluble in hexane and heptane at 10% (w/v). Se = insoluble in water at 0.01% (w/v). Sf = soluble in methanol, ethanol, acetone, acetonitrile, and isopropanol at 50% (w/v). Sg = insoluble in benzene, toluene, diethyl ether, and ethyl acetate at 10% (w/v). Sh = insoluble in dichloromethane and chloroform at 10% (w/v). Si = soluble in water at 50% (w/v). Sj = soluble hexane and heptane at 50% (w/v). |
1 |
[Co(HIm)6][NTf2]2 |
SaSbScSdSe |
186 |
403 |
Present work |
2 |
[Ni(HIm)6][NTf2]2 |
SaSbScSdSe |
194 |
353 |
Present work |
3 |
[Co(OIm)6][NTf2]2 |
SaSbScSdSe |
202 |
407 |
Present work |
4 |
[Ni(OIm)6][NTf2]2 |
SaSbScSdSe |
206 |
356 |
Present work |
5 |
[Co(AmIm)6][NTf2]2 |
SdSeSfSgSh |
202 |
379 |
Present work |
6 |
[Ni(AmIm)6][NTf2]2 |
SdSeSfSgSh |
214 |
295 |
Present work |
7 |
[Co(N,N-DMAc)6][NTf2]2 |
SdSfSgSi |
134 |
364 |
Present work |
8 |
[Ni(N,N-DMAc)6][NTf2]2 |
SdSfSgSi |
122 |
347 |
Present work |
9 |
[Co(N,N-DMP)6][NTf2]2 |
SdSfSi |
123 |
357 |
Present work |
10 |
[Ni(N,N-DMP)6][NTf2]2 |
SdSfSi |
132 |
335 |
Present work |
11 |
[Co(TODGA)2][NTf2]2 |
SaSbScSeSj |
345 |
388 |
Present work |
12 |
[Ni(TODGA)2][NTf2]2 |
SaSbScSeSj |
309 |
353 |
Present work |
— |
[Co(H2O)6][NTf2]2 salt |
SdSfSgShSi |
123 |
373 |
Present work |
— |
[Ni(H2O)6][NTf2]2 salt |
SdSfSgShSi |
86 |
400 |
Present work |
— |
[Co(BIm)4][Cl][NTf2] |
SdSe |
159 |
—c |
22
|
— |
[Ni(BIm)4][Cl][NTf2] |
SdSe |
164 |
—c |
22
|
— |
[Co(OIm)4][Cl][NTf2] |
SdSe |
168 |
—c |
22
|
— |
[Ni(OIm)4][Cl][NTf2] |
SdSe |
178 |
—c |
22
|
— |
[P66614][Co(hfacac)3] |
SaSbSe |
130d |
—c |
23
|
— |
[P66614][Ni(hfacac)3] |
SaSbSe |
215d |
—c |
23
|
— |
[Co(TODGA)2][Co(hfacac)3]2 |
SaSbSeSj |
231d |
—c |
24
|
— |
[Ni(TODGA)2][Ni(hfacac)3]2 |
SaSbSeSj |
235d |
—c |
24
|
3.6. Thermal stability of MILs comprising TODGA, imidazoles, and O-donor ligands
To examine the stability of MILs by studying their decomposition/degradation as a function of temperature, all solvents/reagents were investigated using thermogravimetric analysis (TGA). A detailed description of temperature program and thermograms (Fig. S5–S18, ESI†) are provided in the ESI.† The onset temperature of MIL degradation/volatilization was studied at 5% weight loss from TGA measurements, as shown in Table 2. MIL stability varied in the range of 122 and 345 °C with TODGA ligands offering the highest resilience, possibly due to their ability to form strong chelates. Amongst the monodentate ligands, the O-donor compounds exhibited the lowest ability to withstand elevated temperatures by undergoing degradation between 122 and 134 °C. MILs comprised of imidazoles generally volatilized around 200 °C while longer alkyl substituents and amine functional groups slightly enhanced solvent thermal stability. Additionally, no significant difference was observed in the onset temperature of MIL degradation when the metal center was varied between Co2+ and Ni2+.
While ligands gradually begin to dissociate from metal centers at temperatures close to the onset of MIL degradation, peak decomposition temperature describes stronger volatilization at a more mature stage of MIL disintegration. The derivative of TG (DTG) allows the determination of the maximum rate of weight loss that occurs at point of inflection on the trendline. All thermograms in Fig. S5–S18 (ESI†) are equipped with DTG plots and the peak decomposition temperatures for MILs and salts are summarized in Table 2. Irrespective of the type of ligand, Co2+-based MILs generally exhibited stronger degradation at temperatures 20–50 °C higher than those obtained for Ni2+ MILs. Despite undergoing gradual volatilization at much lower temperatures, MILs composed of N,N-DMAc and N,N-DMP degraded faster at much higher temperatures of over 335 °C. A similar trend was observed for the reagents where stronger decomposition was observed at 373 °C for Co2+ and 400 °C for Ni2+ hydrated salts, despite showing some reduction in weight around 100 °C possibly due to loss of water of crystallization. In addition, all MILs and salts were further studied using DSC and their melting points/glass transition temperatures (Tg) a (Table S1, ESI†), instrument parameters, and thermograms (Fig. S19–S32, ESI†) are provided in the ESI.† In the temperature range of −40 and 200 °C, only the [Co(OIm)6][NTf2]2 and [Ni(OIm)6][NTf2]2 MILs exhibited strong melt peaks at 20.89 and 26.06 °C, respectively. While no glass transition peaks were observed for the MILs, distinct thermal transitions were obtained for the [Co(H2O)6][NTf2]2 (31.93 and 59.93 °C) and [Ni(H2O)6][NTf2]2 (11.38 °C) salts. Additionally, melting points were determined to be (65.78 and 95.04 °C) for the hydrated Co2+ salt and 74.46 °C for the Ni2+ variant.
4. Conclusions
As a sustainable alternative to traditional organic solvents, MILs have established a strong footprint in high-throughput laboratories and industrial settings. To design and develop green, automated separation systems and analytical testing methodologies, MILs are prioritized as extractants over other solvents due to their magnetic properties that allow ease of handling. However, these solvents are acquired through complex synthetic routes which are tedious, labor-intensive, and time-consuming. Food/beverage and pharmaceutical industries, for example, operate under strict guidelines that require the use of standardized and readily available materials in manufacturing and quality control processes. Extensive preparation/purification steps required to obtain MILs under the established synthetic schemes prevent researchers from thoroughly harnessing their potential in fast-paced, eco-friendly environments. In the interest of minimizing costs and moving towards greener methods of MIL synthesis, this study is the first to utilize desirable HMDES preparation methods to produce chemically robust hydrophobic MILs.
In this work, mono and multidentate ligands comprised of alkylimidazoles, TODGA, and 3° amides were paired with transition metal-based [NTf2−] salts to form stable hydrophobic metal–organic complexes that formed an independent phase in aqueous matrices. Two different approaches were developed to obtain MILs: (1) a solvent-free heat/stir method that can form neat compounds and (2) an in situ setup that could instantly combine reagents to produce the same MIL under aqueous conditions. MILs designed in this study possess viscosities (as low as 198.3 cP) and magnetic susceptibilities (μeff of up to 5.16μB) that are comparable to previous generations of MILs. However, their chemical and thermal stabilities are unrivalled as they maintained their chemical composition and structural integrity in water for over 6 months and at temperatures as high as 345 °C. MILs demonstrated excellent solubilities in a spectrum of organic solvents by solubilizing in non-polar solvents such as benzene at high concentrations of 50% (w/v) MIL-to-solvent ratio while being insoluble in water even at 0.01% (w/v). This study pioneers the most sustainable class of MILs that is easy to prepare, requires zero synthetic skills, and provides the option to modulate their chemical structures with cheap and commercially available reagents to expand the scope of these magnetoactive solvents in resource-limited settings where researchers often compromise by employing HMDESs that clearly demonstrate poor chemical and thermal stabilities.
Conflicts of interest
There are no conflicts to declare.
Acknowledgements
The authors acknowledge funding from the Chemical Measurement and Imaging Program at the National Science Foundation (Grant number CHE-2203891). The study of magnetic properties of the samples is supported by the Division of Materials Science and Engineering of the Office of Basic Energy Sciences, Office of Science of the U. S. Department of Energy (DOE) through the Ames National Laboratory. Ames National Laboratory is operated for the U.S. Department of Energy by Iowa State University under contract DE-AC02-07CH11358. Special thanks to Dr Y. Mudryk for the useful discussion.
References
- A. A. Quintana, A. M. Sztapka, V. Santos Ebinuma, C. de and C. Agatemor, Enabling Sustainable Chemistry with Ionic Liquids and Deep Eutectic Solvents: A Fad or the Future?, Angew. Chemie, 2022, 61(37) DOI:10.1002/ange.202205609.
- J. P. Hallett and T. Welton, Room-Temperature Ionic Liquids: Solvents for Synthesis and Catalysis. 2, Chem. Rev., 2011, 111(5), 3508–3576, DOI:10.1021/cr1003248.
- Z. Lei, B. Chen, Y. M. Koo and D. R. Macfarlane, Introduction: Ionic Liquids, Chem. Rev., 2017, 117(10), 6633–6635, DOI:10.1021/acs.chemrev.7b00246.
- A. Brzeczek-szafran, K. Erfurt, A. Blacha-grzechnik, M. Krzywiecki, S. Boncel and A. Chrobok, Carbohydrate Ionic Liquids and Salts as All-in-One Precursors for N-Doped, Carbon, 2019, 7(24), 19880–19888, DOI:10.1021/acssuschemeng.9b05297.
- S. Raiguel, W. Dehaen and K. Binnemans, Stability of Ionic Liquids in Brønsted-Basic Media, Green Chem., 2020, 5225–5252, 10.1039/d0gc01832e.
- R. E. Del Sesto, C. Corley, A. Robertson and J. S. Wilkes, Tetraalkylphosphonium-Based Ionic Liquids, J. Organomet. Chem., 2005, 690(10), 2536–2542, DOI:10.1016/j.jorganchem.2004.09.060.
- D. Fang, J. Yang and C. Jiao, Dicationic Ionic Liquids as Environmentally Benign Catalysts for Biodiesel Synthesis, ACS Catal., 2011, 1(1), 42–47, DOI:10.1021/cs100026q.
- S. T. Handy and X. Zhang, Organic Synthesis in Ionic Liquids: The Stille Coupling, Org. Lett., 2001, 3(2), 233–236, DOI:10.1021/ol0068849.
- J. H. Davis, Task-Specific Ionic Liquids, Chem. Lett., 2004, 33(9), 1072–1077, DOI:10.1246/cl.2004.1072.
- R. González-Martín, E. Lodoso-Ruiz, M. J. Trujillo-Rodríguez and V. Pino, Magnetic Ionic Liquids in Analytical Microextraction: A Tutorial Review, J. Chromatogr. A, 2022, 1685, 463577, DOI:10.1016/j.chroma.2022.463577.
- H. Chen, J. L. Anderson and R. K. Anand, Electropolymerization of Pyrrole-Based Ionic Liquids on Selected Wireless Bipolar Electrodes, ACS Appl. Mater. Interfaces, 2022, 14(16), 18087–18096, DOI:10.1021/acsami.1c25230.
- P. Nockemann, B. Thijs, N. Postelmans, K. Van Hecke, L. Van Meervelt and K. Binnemans, Anionic Rare-Earth Thiocyanate Complexes as Building Blocks for Low-Melting Metal-Containing Ionic Liquids, J. Am. Chem. Soc., 2006, 128(42), 13658–13659, DOI:10.1021/ja0640391.
- O. Nacham, K. D. Clark, H. Yu and J. L. Anderson, Synthetic Strategies for Tailoring the Physicochemical and Magnetic Properties of Hydrophobic Magnetic Ionic Liquids, Chem. Mater., 2015, 27(3), 923–931, DOI:10.1021/cm504202v.
- M. Sajid, Magnetic Ionic Liquids in Analytical Sample Preparation: A Literature Review, TrAC, Trends Anal. Chem., 2019, 113, 210–223, DOI:10.1016/j.trac.2019.02.007.
- E. Santos, J. Albo, A. Rosatella, C. A. M. Afonso and Á. Irabien, Synthesis and Characterization of Magnetic Ionic Liquids (MILs) for CO2 Separation, J. Chem. Technol. Biotechnol., 2014, 89(6), 866–871, DOI:10.1002/jctb.4323.
- Y. Yoshida and G. Saito, Influence of Structural Variations in 1-Alkyl-3-Methylimidazolium Cation and Tetrahalogenoferrate(III) Anion on the Physical Properties of the Paramagnetic Ionic Liquids, J. Mater. Chem., 2006, 16(13), 1254–1262, 10.1039/b515391c.
- S. Hayashi and H. O. Hamaguchi, Discovery of a Magnetic Ionic Liquid [Bmim]FeCl4, Chem. Lett., 2004, 33(12), 1590–1591, DOI:10.1246/cl.2004.1590.
- D. K. Bwambok, M. M. Thuo, M. B. J. Atkinson, K. A. Mirica, N. D. Shapiro and G. M. Whitesides, Paramagnetic Ionic Liquids for Measurements of Density Using Magnetic Levitation, Anal. Chem., 2013, 85(17), 8442–8447, DOI:10.1021/ac401899u.
- Z. L. Xie and A. Taubert, Thermomorphic Behavior of the Ionic Liquids [C4mim][FeCl4] and [C12mim][FeCl4], ChemPhysChem, 2011, 12(2), 364–368, DOI:10.1002/cphc.201000808.
- P. Brown, C. P. Butts, J. Eastoe, E. Padrón Hernández, F. L. De Araujo Machadob and R. J. De Oliveirac, Dication Magnetic Ionic Liquids with Tuneable Heteroanions, Chem. Commun., 2013, 49(27), 2765–2767, 10.1039/c3cc00103b.
- M. Q. Farooq, D. Chand, G. A. Odugbesi, M. Varona, Y. Mudryk and J. L. Anderson, Investigating the Effect of Ligand and Cation on the Properties of Metal Fluorinated Acetylacetonate Based Magnetic Ionic Liquids, New J. Chem., 2019, 43(28), 11334–11341, 10.1039/c9nj02595b.
- D. Chand, M. Q. Farooq, A. K. Pathak, J. Li, E. A. Smith and J. L. Anderson, Magnetic Ionic Liquids Based on Transition Metal Complexes with N-Alkylimidazole Ligands, New J. Chem., 2019, 43(1), 20–23, 10.1039/c8nj05176c.
- S. A. Pierson, O. Nacham, K. D. Clark, H. Nan, Y. Mudryk and J. L. Anderson, Synthesis and Characterization of Low Viscosity Hexafluoroacetylacetonate-Based Hydrophobic Magnetic Ionic Liquids, New J. Chem., 2017, 41(13), 5498–5505, 10.1039/c7nj00206h.
- N. M. Abbasi, V. R. Zeger, A. Biswas and J. L. Anderson, Synthesis and Characterization of Magnetic Ionic Liquids Containing Multiple Paramagnetic Lanthanide and Transition Metal Centers and Functionalized Diglycolamide Ligands, J. Mol. Liq., 2022, 361, 119530, DOI:10.1016/j.molliq.2022.119530.
- K. Wu and X. Shen, Designing a New Type of Magnetic Ionic Liquid: A Strategy to Improve the Magnetic Susceptibility, New J. Chem., 2019, 43(40), 15857–15860, 10.1039/c9nj03464a.
- Y. Funasako, T. Mochida, T. Inagaki, T. Sakurai, H. Ohta, K. Furukawa and T. Nakamura, Magnetic Memory Based on Magnetic Alignment of a Paramagnetic Ionic Liquid near Room Temperature, Chem. Commun., 2011, 47(15), 4475–4477, 10.1039/c0cc05820c.
- N. M. Abbasi, S. De Silva, A. Biswas and J. L. Anderson, Ultra-Low Viscosity and High Magnetic Susceptibility Magnetic Ionic Liquids Featuring Functionalized Diglycolic Acid Ester Rare-Earth and Transition Metal Chelates, ACS Omega, 2023, 8(30), 27751–27760, DOI:10.1021/acsomega.3c03938.
- E. L. Smith, A. P. Abbott and K. S. Ryder, Deep Eutectic Solvents (DESs) and Their Applications, Chem. Rev., 2014, 114(21), 11060–11082, DOI:10.1021/cr300162p.
- M. Q. Farooq, N. M. Abbasi and J. L. Anderson, Deep Eutectic Solvents in Separations: Methods of Preparation, Polarity, and Applications in Extractions and Capillary Electrochromatography, J. Chromatogr. A, 2020, 1633, 461613, DOI:10.1016/j.chroma.2020.461613.
- N. Yadav, K. Bhakuni, M. Bisht, I. Bahadur and P. Venkatesu, Expanding the Potential Role of Deep Eutectic Solvents toward Facilitating the Structural and Thermal Stability of α-Chymotrypsin, ACS Sustainable Chem. Eng., 2020, 8(27), 10151–10160, DOI:10.1021/acssuschemeng.0c02213.
- C. Vidal, L. Merz and J. García-Álvarez, Deep Eutectic Solvents: Biorenewable Reaction Media for Au(I)-Catalysed Cycloisomerisations and One-Pot Tandem Cycloisomerisation/Diels-Alder Reactions, Green Chem., 2015, 17(7), 3870–3878, 10.1039/c5gc00656b.
- C. Florindo, F. Lima, L. C. Branco and I. M. Marrucho, Hydrophobic Deep Eutectic Solvents: A Circular Approach to Purify Water Contaminated with Ciprofloxacin, ACS Sustainable Chem. Eng., 2019, 7(17), 14739–14746, DOI:10.1021/acssuschemeng.9b02658.
- C. Florindo, F. S. Oliveira, L. P. N. Rebelo, A. M. Fernandes and I. M. Marrucho, Insights into the Synthesis and Properties of Deep Eutectic Solvents Based on Cholinium Chloride and Carboxylic Acids, ACS Sustainable Chem. Eng., 2014, 2(10), 2416–2425, DOI:10.1021/sc500439w.
- M. Á. Aguirre and A. Canals, Magnetic Deep Eutectic Solvents in Microextraction Techniques, TrAC, Trends Anal. Chem., 2022, 146, DOI:10.1016/j.trac.2021.116500.
- M. Q. Farooq, N. Tryon-Tasson, A. Biswas and J. L. Anderson, Preparation of Ternary Hydrophobic Magnetic Deep Eutectic Solvents and an Investigation into Their Physicochemical Properties, J. Mol. Liq., 2022, 365, 120000, DOI:10.1016/j.molliq.2022.120000.
- E. Massolo, S. Palmieri, M. Benaglia, V. Capriati and F. M. Perna, Stereoselective Organocatalysed Reactions in Deep Eutectic Solvents: Highly Tunable and Biorenewable Reaction Media for Sustainable Organic Synthesis, Green Chem., 2016, 18(3), 792–797, 10.1039/c5gc01855b.
- X. Yang, Q. Zou, T. Zhao, P. Chen, Z. Liu, F. Liu and Q. Lin, Deep Eutectic Solvents as Efficient Catalysts for Fixation of CO2 to Cyclic Carbonates at Ambient Temperature and Pressure through Synergetic Catalysis, ACS Sustainable Chem. Eng., 2021, 9(31), 10437–10443, DOI:10.1021/acssuschemeng.1c03187.
- M. Zuo, K. Le, Z. Li, Y. Jiang, X. Zeng, X. Tang, Y. Sun and L. Lin, Green Process for Production of 5-Hydroxymethylfurfural from Carbohydrates with High Purity in Deep Eutectic Solvents, Ind. Crops Prod., 2017, 99, 1–6, DOI:10.1016/j.indcrop.2017.01.027.
- B. Jiang, H. Dou, B. Wang, Y. Sun, Z. Huang, H. Bi, L. Zhang and H. Yang, Silver-Based Deep Eutectic Solvents as Separation Media: Supported Liquid Membranes for Facilitated Olefin Transport, ACS Sustainable Chem. Eng., 2017, 5(8), 6873–6882, DOI:10.1021/acssuschemeng.7b01092.
- S. Liu, D. Yu, Y. Chen, R. Shi, F. Zhou and T. Mu, High-Resolution Thermogravimetric Analysis Is Required for Evaluating the Thermal Stability of Deep Eutectic Solvents, Ind. Eng. Chem. Res., 2022, 61(38), 14347–14354, DOI:10.1021/acs.iecr.2c02240.
- Y. Dai, J. van Spronsen, G. J. Witkamp, R. Verpoorte and Y. H. Choi, Natural Deep Eutectic Solvents as New Potential Media for Green Technology, Anal. Chim. Acta, 2013, 766, 61–68, DOI:10.1016/j.aca.2012.12.019.
- Y. Dai, G. J. Witkamp, R. Verpoorte and Y. H. Choi, Tailoring Properties of Natural Deep Eutectic Solvents with Water to Facilitate Their Applications, Food Chem., 2015, 187, 14–19, DOI:10.1016/j.foodchem.2015.03.123.
- D. J. G. P. Van Osch, C. H. J. T. Dietz, J. Van Spronsen, M. C. Kroon, F. Gallucci, M. Van Sint Annaland and R. Tuinier, A Search for Natural Hydrophobic Deep Eutectic Solvents Based on Natural Components, ACS Sustainable Chem. Eng., 2019, 7(3), 2933–2942, DOI:10.1021/acssuschemeng.8b03520.
- J. Sniekers, P. Geysens, J. C. Malaquías, T. Vander Hoogerstraete, L. Van Meervelt, J. Fransaer and K. Binnemans, Cobalt(II) Containing Liquid Metal Salts for Electrodeposition of Cobalt and Electrochemical Nanoparticle Formation, Dalton Trans., 2017, 46(38), 12845–12855, 10.1039/c7dt02604h.
- J. Sniekers, P. Geysens, T. Vander Hoogerstraete, L. Van Meervelt, J. Fransaer and K. Binnemans, Cobalt(II) Liquid Metal Salts for High Current Density Electrodeposition of Cobalt, Dalton Trans., 2018, 47(14), 4975–4986, 10.1039/c8dt00283e.
Footnote |
† Electronic supplementary information (ESI) available: List of chemicals and reagents, equations used in magnetic susceptibility calculations, instrument parameters to conduct TGA and DSC measurements, melting point/glass transition temperatures of MILs/salts, pictorial representation of long-term MIL stability stored under water, XRD patterns of [NTf2−] salts and relate discussion, Curie–Weiss plots for select MILs, thermograms for MILs/reagents obtained using TGA and DSC, and 1H and 13C NMR spectra for TODGA ligand synthesized in the study. See DOI: https://doi.org/10.1039/d4nj00624k |
|
This journal is © The Royal Society of Chemistry and the Centre National de la Recherche Scientifique 2024 |
Click here to see how this site uses Cookies. View our privacy policy here.