Environmental safety of nanocellulose: an acute in vivo study with marine mussels Mytilus galloprovincialis†
Received
28th February 2023
, Accepted 5th November 2023
First published on 14th November 2023
Abstract
The growing application of nanocellulose in various industrial sectors with potential release into the natural environment demands a safety assessment and thus ecotoxicity. Herein, we tested two types of cellulose nanofibers, non-oxidized (CNF) and TEMPO-oxidized (TOCNF), by performing acute in vivo studies with the marine bivalve Mytilus galloprovincialis at 1 μg L−1 and 1 mg L−1 as resembling realistic and acute exposure scenarios, respectively. Uptake and sub-lethal biological responses (lysosomal membrane stability, neurotoxicity, oxidative stress and biotransformation) were investigated along with changes in fibers with water salinity. TOCNF resulted in more dispersion than CNF in natural sea water (NSW) probably owing to higher repulsion among fibers driven by their negative surface charges and colloidal organic material. Both CNF and TOCNF were found in mussel tissues (gills and hemolymph) using labelled stocks. The destabilization of lysosomal membranes of hemocytes was observed; similarly, the inhibition of P-gp efflux activities in the gills was stronger for CNF at the highest concentration (1 mg L−1). Cholinergic enzymes (ASCh–ChE activities) were inhibited in hemocytes, gills and digestive glands regardless of CNF oxidation and concentration tested. In contrast, neither oxidative stress nor biotransformation was affected in the digestive glands and gills of the mussels. Overall, the findings showed CNF uptake by marine mussels and the disruption of gill functionality and immune cells by mechanical interaction even in environmentally realistic exposure scenarios. The paucity of data on the hazards posed by CNF for aquatic species demands that safety aspects be considered in future risk assessment scenarios.
Environmental significance
Nanocellulose (NC) is a biodegradable and renewable material that steadily occupies a larger portion of the market with an increasingly higher environmental load that requires a proper safety evaluation. Through sewages and wastewaters, NC is likely to end up in an aquatic environment, for which limited information in terms of ecotoxicity is currently available. Recent evidence on the presence of textile fibers, mostly made of cellulose, in invertebrate species populating marine coastal areas worldwide, raises questions on the potential hazards posed by NC and its consequences on marine biodiversity.
|
Introduction
Cellulose (C6H10O5) is a naturally abundant biopolymer; it is primarily obtained from plants and other biomass sources and has gained significant attention in the production market with an estimate to reach 300 million $ by 2026.1,2 Nanocellulose (NC) with at least one dimension below 100 nm, either as cellulose nanocrystals (CNCs) or cellulose nanofibers (CNFs), possesses particular and tunable physico-chemical properties, which include size, crystallinity, low density and high biodegradability.3–5 Owing to these properties, NC plays an increasingly significant role in various sectors ranging from biomedical, food industry, and construction to environmental remediation.6–10 Nanocellulose can be also obtained from renewable and abundant biomasses (i.e., biowaste), thereby competing, in this case, with synthetic fibers in terms of biodegradability, cost-effectiveness and environmental and human safety.11–14 CNFs are the most widely used NC materials in a wide range of applications, both as additives and as building blocks for the design of nanostructured materials. They comprise both amorphous and crystalline cellulose domains, with a high surface-to-volume ratio and a mechanical strength greater than synthetic petroleum-derived fibers (plastic polymers).15–18 There are two main top-down procedures to produce CNF from biomass sources, both consisting of the cleaving of the hierarchical structure of cellulose fibers, but providing products characterized by different chemical compositions and physical properties. A first non-oxidized formulation, representing standard CNF, derives from an enzymatic-mediated pre-hydrolysis of cellulose fiber pulp.19 A second one is obtained by partially converting the C6 alcoholic groups of the cellulose glucopyranosyl units into the corresponding carboxylic moieties using 2,2,6,6-tetramethyl-1-piperidinyloxy (TEMPO)-mediated oxidation, leading to the formation of TEMPO-oxidized CNF (TOCNF).20 Thus, TOCNF differs from CNF because of the presence of certain amounts of carboxylic groups on the nanofiber backbone.
Owing to the wide and increasing number of NC applications, production volumes are expected to increase in subsequent years, which will inevitably lead to their significant release into the environment, demanding a timely and accurate assessment of human and environmental safety.21,22 Being considered environmentally friendly and non-toxic, NC has barely been targeted for behavior and fate in the environment (i.e., water), and the limited investigations available on its ecotoxicity raise uncertainties in terms of environmental safety.23 A recently applied dynamic and probabilistic modelling approach has defined a low environmental risk for NC material on surface water based on risk characterization ratio (RCR) for the European market, but the use and production of NC are accelerating worldwide, calling for an updated risk assessment for aquatic systems.24 To date, controversial findings have been observed in most toxicity studies on NC mainly concentrated on human health through acute short-term in vitro and in vivo exposures either by inhalation and/or ingestion.25–29 Thus, CNFs were not cytotoxic to human gastrointestinal epithelial cells (Caco-2), while in human lung epithelial cells (A549), the higher toxicity of CNF over CNC rebounds to their specific properties; CNF forms long chains of fibrous structures, while CNC is crystalline long needle-like rods.30,31 The length but above all the diameter is a key parameter that can dictate toxicity because of the potential biological interaction with cells and/or tissues, which dictate toxicological outcomes.21,32–35
Few studies have focused so far on aquatic organisms, confirming NC uptake in zebrafish embryos (Danio rerio) with low or absence of toxicity of up to 1 g L−1 and uptake upon dermal and oral exposure (1 mg mL−1).36–38 No toxicity was reported for the freshwater green algae Pseudokirchneriella subcapitata (up to 2.8 g L−1 of CNC and 1.2 g L−1 of CNF) or the microcrustacean Daphnia sp. (up to 14 g L−1 CNC and 6 g L−1 for CNF, respectively) even in prolonged exposure (21-day up to 2.06 g L−1); however, at low regime and lower concentration (20.6 mg L−1), growth and reproduction of daphnids were impaired.35,39 Increased ROS levels but no mortality were reported in green algae Scenedesmus obliquus, D. magna and zebrafish embryos upon exposure to wood-based CNC, cotton-based CNC and CNF at environmentally relevant concentrations (0.01–10 mg L−1) by Wang et al.40 No acute toxicity to CNF extracted from kenaf (Hibiscus cannabinus) was observed in D. magna and adult zebrafish (100 mg L−1), but altered swimming behavior was documented in daphnids probably due to CNF gut retention. Conversely, high clearance of CNF (99%) was shown after 60 min of active feeding with CNF in daphnids upon acute exposure.39
Larger gaps of knowledge are recognized for marine species in terms of NC behavior in seawater, uptake and ultimate ecotoxicity. Surprisingly, textile microfibers mostly made of cellulose, cotton, wool and silk have recently been found in marine species of coastal waters far higher than those of synthetic origin (>74%, polyester and polyamide).41–43 Adherence to ingestion in the accumulation of synthetic microfibers (polyester) in various organs of the marine mussel Mytilus edulis was reported (2000 microfibers per L in 48 h).44 Time-dependent accumulation (24 h up to 7 days) of polyamide microfibres (10 × 30 μm, 500 ng mL−1) has been reported in mussel's digestive glands although immune responses and cytotoxicity were found only for nanoscopic material due to easy permeation into circulatory fluid and tissues.45 Neurotoxic effects and reproductive failure along with other sub-lethal effects have been documented upon long-term exposure (32 days) of Mytilus galloprovincialis to polyethylene terephthalate (PET) microfibers (100 μm) up to 100 mg L−1.46 Fiber lengths (50–100 μm) also affect toxicological outcomes under short-term exposure conditions.47
In farmed mussels from the Mediterranean Sea M. galloprovincialis, synthetic and natural textile microfibers (an average of 14.5720 microfibres per individual) have been documented with cotton and wool as the majority (54% and 985.14 μm in length), raising concerns owing to recent reports on neurotoxicity, oxidative stress and metabolic and developmental disorders.48,49
To date, no information is available on the effects of nanoscale natural fibers although fragmentation below 100 nm could naturally occur and is more likely in areas receiving higher amounts, such as along marine coasts. To fill such a knowledge gap, herein, we investigate the uptake and biological effects of NC fibers in the marine mussel M. galloprovincialis through an acute in vivo exposure (96 h uptake; 48 h effects) using synthesized CNF and TOCNF in two concentrations: a) 1 μg L−1 in the range of Predicted Environmental Concentrations (PEC, 0.23–2.37 μg L−1 in 2025 by Stoudmann et al.24) and b) 1 mg L−1 as representative of acute exposure scenarios. A battery of biological responses was evaluated: cytotoxicity (lysosomal membrane stability) in circulating immune cells (hemocytes), neurotoxicity (cholinergic enzyme activity) in hemolymph, gills and digestive glands, oxidative stress and cellular biotransformation (efflux pumps, phase I and II enzymes) in gills and digestive glands.
Materials and methods
All the reagents used were purchased from Sigma-Aldrich (Milano, Italy). For NC synthesis, endoglucanase FiberCare R was provided by Novozymes (Bagsværd, Denmark). Both CNF and TOCNF were obtained from cotton linter sheets provided by the Bartoli Paper Mill factory in Capannori (Lucca, Italy). Deionized water was produced using a Millipore Elix® Deionizer with Progard® S2 ion exchange resins and other pieces of equipment used in synthetic procedures include a Branson Sonifier 250 equipped with a 6.5 mm probe tip, a SP Scientific BenchTop Pro Lyophilizer, a GEA Niro Soavi NS3006Land high-pressure homogenizer and a PFI refiner compliant to the UNI EN ISO 5264-2:2011 normative. For ecotoxicological studies, the following materials were used: Petri dishes (22–10 cm), 1 mL syringe, vial 15 mL, cotton-blend gauze, polylysine slides, coverslips (22 mm × 22 mm), transparent Greiner® UV plates and transparent NUNC plates by Thermo Fisher and the following reagents (Sigma-Aldrich): neutral red, HEPES, NaCl, MgSO4, KCl, CaCl, rhodamine B, DCFH-DA, DMSO, 1-methyl-2-phenylindole, acetonitrile, HCl 37%, 1,1,3,3-tetramethoxypropane, Tris–HCl, BioRad proteins, KH2PO4, EDTA (ethylenediaminetetraacetic), DTT, DTNB, ASCh, p-NPA, ethanol-96, p-NPB, purified proteins (CE1CAT, GST, GR, AChE), CDNB, GSH, GSGG, NADPH, CHP, H2O2, NADPH, and NaN3.
Synthesis of cellulose nanofibers (CNFs) and TEMPO-oxidized cellulose nanofibers (TOCNFs)
CNFs were synthesized according to an optimized procedure previously reported.13 Briefly, in the first step, a pre-refined, 2% w/w cellulose water suspension was subjected to enzymatic treatment using Endoglucanase FiberCare R (0.1 mg g−1) to maintain the pulp under magnetic stirring for 1 h at 50 °C. At the end of the reaction, the suspension was filtered and resuspended in deionized water at a 10% w/w concentration for a second refining phase. Finally, cotton linters with a 2% w/w suspension were homogenized using a high-pressure homogenizer. After this passage, the obtained CNF suspension was freeze-dried to completely remove water residues, resulting in a white powder.
TOCNFs were synthesized according to a previously reported standardized protocol.13,50 The first step consisted of the TEMPO-mediated oxidation of cellulose.51,52 Cotton linters pulp was suspended in a keg containing TEMPO (0.22 g, 1.38 mmol) and KBr (1.54 g, 12.9 mmol) at a final cellulose concentration of 2% w/w (final volume: 570 mL). The oxidation process was promoted by adding dropwise an aqueous solution of NaClO (12.5% w/w aqueous solution, 43.7 mL) to the cellulose suspension under vigorous magnetic stirring. The pH was monitored and maintained above 10.5–11 by adding small amounts of 4 M NaOH aqueous solution. The suspension was left to react overnight, after which it was acidified by the addition of 5 mL of 12 M HCl aqueous solution. TEMPO-oxidized cellulose fibers were then filtered, resuspended in deionized water at a 2% w/w concentration and sonicated with an immersion ultra-sonifier for five minutes, obtaining a 2% w/w suspension of TOCNF. This suspension was freeze-dried, providing a pale yellowish powder. To estimate carboxyl groups on the TOCNF cellulose backbone, titration was performed with NaOH using phenolphthalein as a colorimetric indicator.
Synthesis of rhodamine B-labelled CNF (CNF–RhB) and TOCNF (TOCNF–RhB)
CNF and TOCNF batched labelled with Rhodamine B (RhB) were also provided to track their uptake upon in vivo exposure to marine bivalves. The synthesis of CNF–RhB is represented in Scheme 1.
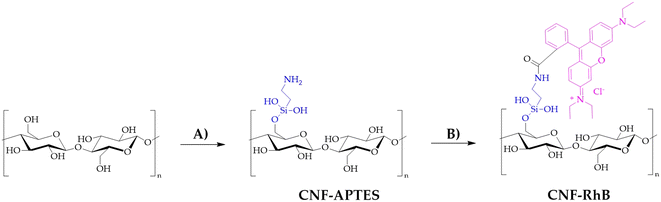 |
| Scheme 1 Synthesis of CNF–RhB. A) Toluene, 120 °C, 12 h, APTES; B) Na2CO3, rhodamine B, EDC, NHS, water, room temperature, 48 h. | |
TOCNF (1000 mg) was placed in a two-necked flask equipped with a condenser under an N2 atmosphere and then dispersed in toluene (60 mL). To this suspension, 3-amino-propil-triethoxysilane (APTES, 440 mg, 2 mM) was added. The reaction mixture was heated to reflux for 12 hours at 120 °C under magnetic stirring. The product obtained was then filtered using a Büchner funnel and washed with toluene (3 × 10 mL), water (3 × 10 mL) and acetone (3 × 10 mL) and allowed to air dry, obtaining a yellowish CNF–APTES powder. CNF–RhB was synthesized starting with CNF–APTES. In a 100 mL beaker, CNF–APTES (1.00 g) and Na2CO3·H2O (380 mg) were mixed in 50 mL of deionized water (solution A). In another 100 mL beaker, rhodamine B (60 mg), 1-ethyl-3-(3-dimethylaminopropyl)carbodiimide (EDC, 500 mg) and N-hydroxysuccinimide (NHS, 125 mg) were dissolved in 50 mL of deionized water, maintaining the solution under magnetic stirring for 30 minutes while cooling with the aid of an ice-bath (solution B). Solution B was then added to solution A; stirring the mixture at room temperature for 48 hours, a pale pink solid was filtered on a Büchner funnel and washed with deionized water (3 × 10 mL), 0.1 N NaOH (3 × 10 mL), 0.1 N HCl (3 × 10 mL), and finally with acetone (3 × 10 mL). The procedure for the synthesis of TOCNF–RhB (Scheme 2) is the same as that described for CNF–RhB. TOCNF–APTES recovered a pale beige solid (step C in Scheme 2), while TOCNF–RhB (step D in Scheme 2) resulted in a pale pink solid.
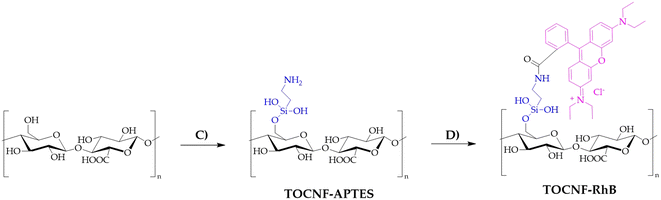 |
| Scheme 2 Synthesis of TOCNF–RhB. C) Toluene, 120 °C, 12 h, APTES; D) Na2CO3, rhodamine B, EDC, NHS, water, room temperature, 48 h. | |
CNF and TOCNF characterization
The solid-phase FTIR spectra of CNF and TOCNF were obtained using a Varian 640-IR spectrometer equipped with a single bounce ZnSe ATR accessory. All the samples were treated with HCl 0.1 N before acquisition. The number of acquisitions for each sample was 64. The point of zero charge (pHPZC) was measured using the pH drift method described by Lopez-Ramon et al.53 For this purpose, a 0.01 M NaCl solution (20 mL) was placed in a Falcon vial and degassed by bubbling N2 to stabilise the pH by preventing the dissolution of CO2 in the solution. The pH was then adjusted to the starting values between 2 and 12 by dripping HCl 0.01 N or NaOH 0.01 N. The material (0.06 g) was then added to the solution, reaching a final stable pH value after 4 hours. These final pH values were plotted against the initial ones to determine the pHPZC; this is the pH at which the curve crosses the bisector pHfinal = pHinitial. Powder XRD patterns were recorded using a Bruker D2 Phaser X-ray powder diffractometer with CuKα radiation. The data were measured in the 2θ range of 4–30° with a step size of 0.02° and a counting time of 2 s per step. The data were recorded at room temperature using a Bragg–Brentano reflection geometry. The crystallinity index (CrI) was determined by applying Segal method,5 as shown in eqn (1): | 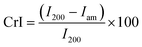 | (1) |
where CrI is the crystallinity index (%), I200 is the maximum intensity of the (200) reflection, at 2θ of 22–23°, and Iam is the lowest intensity of diffraction at 2θ of 18–19° for the amorphous part.54,55
The morphology of CNF and TOCNF in deionized water, natural sea water (NSW) and artificial sea water (ASW) was examined by TEM (transmission electron microscopy). To prepare each sample, a drop of suspension was placed on a copper screen (200 mesh) covered with carbon film using a glass Pasteur pipette. The drop deposited on the screen was left to evaporate overnight at room temperature. The screen was then observed under a Philips CM200 FEG TEM at 200 kV.
The surface charge (ζ-potential) of the CNF and TOCNF dispersions (1 mg mL−1) in MilliQ and 0.22 μm-filtered NSW was measured by dynamic light scattering (DLS) using a Zetasizer Nano ZS90, Malvern, combined with Zetasizer Nano Series software, version 7.02, Particular Sciences. Measurements were performed at time 0 and after 24 h of incubation of CNF and TOCNF with the two water media. ζ potential values were generated by triplicate measurements performed using an instrumentally determined number of runs.
Ecotoxicity assessment: experimental design
The experimental design of the ecotoxicological study summarized in Scheme 3 can be divided into three steps: 1) characterisation and behaviour of CNF and TOCNF in MilliQ water, artificial sea water (ASW) and natural seawater (NSW) by investigating changes in salinity, pH and fiber morphology (TEM); 2) uptake of CNF and TOCNF in marine mussels M. galloprovincialis through in vivo exposure (96 h) using fluorescent RhB labelled NC fibers; and 3) ecotoxicological assessment through an in vivo acute exposure of mussels to CNF and TOCNF (48 h) at realistic (1 μg L−1) and severe environmental concentrations (1 mg L−1) (Scheme 3).
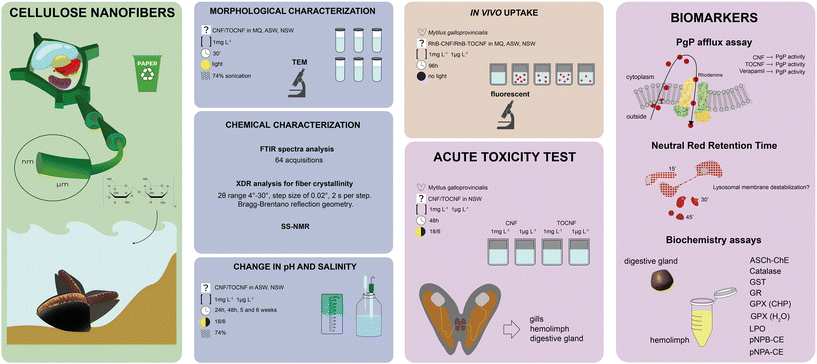 |
| Scheme 3 Graphical description of the experimental design of the ecotoxicity assessment of CNF and TOCNF. | |
Behaviour of CNF and TOCNF in seawater media and TEM analysis
MilliQ water was obtained from a Millipore Milli-Q® Integral Water Purification System; ASW was obtained by dissolving Coral Pro Salt-Red Sea in distilled water (35 g L−1; 35‰) and filtered at 0.22 μm; and NSW was collected from Marina di Grosseto (GR, Tuscany Italy), an area with a low level of contamination (35‰) and filtered at 0.22 μm. CNF and TOCNF behaviours in MilliQ, ASW and NSW were investigated by incubating 1 mg L−1 of CNF and TOCNF in the three media, and changes in salinity and pH were measured at 24 h (t1), 5 (t2) and 6 (t3) weeks using a bench refractometer and a pH-Meter Basic20+ (Crison). All water samples were kept at room temperature (22 °C) and a natural photoperiod (18
:
6). In parallel, suspensions were gently bath sonicated (30 minutes at 20 °C at 74% power, ultrasonic cleaner CP316) to mimic potential natural breakdown processes (i.e., waves). CNF and TOCNF were recovered and observed by light microscopy using an Olympus optical microscope with 20× magnification. The stability of the RhB labelled on CNF and TOCNF (CNF–RhB and TOCNF–RhB) was verified upon incubation in NSW, which was used for the uptake mussel study. Approximately 1 mg L−1 of each stock was incubated in NSW for 24 h, and the fibers were recovered by centrifugation and observed using a fluorescence microscope at Ex 522–Em 586 (Olympus) at 20× magnification with an RhB filter (Ex 522–Em 586). The conditioned NSW was also checked for RhB content using a Victor 3 spectrophotofluorometer (Perkin Elmer) with Ex 522 and Em 585 filters.
In vivo acute exposure to CNF and TOCNF in mussels
Uptake of CNF and TOCNF by mussels.
Mussel uptake of CNF and TOCNF was investigated using rhodamine B (RhB) labelled stocks (CNF–RhB and TOCNF–RhB) (see sections of methods for details of preparation) under 96 h in vivo exposure conditions at two different concentrations of 1 μg L−1 and 1 mg L−1 following the methods of Patel et al.38 and Robin and O′Reilly.56 Mussels (7.16 ± 0.05 cm shell length) were obtained from a commercial farm in Alborea (OR, Italy) in autumn 2021 and acclimatized (1 organism per L) for 24 h in polyethylene and glass resin tanks filled with NSW (40 psu, pH 8 ± 0.1, density 1.025 ± 0.001 g cm−3, conductivity 49 ± 1 mS cm−1 and temperature 18 ± 1 °C). Three mussels per tank were exposed to CNF–RhB and TOCNF–RhB (at 1 μg L−1 and 1 mg L−1 in NSW) in triplicate for each concentration plus a control only in NSW and kept at 20 °C within a natural photoperiod (18
:
6) and constant aeration. After 96 h, hemolymph was removed from adductor muscle with a 1 mL sterile syringe, previously rinsed with physiological solution (HEPES 20 mM, NaCl 436 mM, MgSO4 53 mM, KCl 12 mM and CaCl2 12 mM, pH 7.6) and placed on microscope slides. Gills and mantle were also removed, and punch biopsies (1 mm × 1 mm) were placed on slides. The presence of CNF–RhB and TOCNF–RhB was confirmed using an Olympus optical fluorescence microscope using RhB Ex 522–Em 586 and 20× magnification.
Ecotoxicological effects of CNF and TOCNF in mussels.
After 48 h of acclimation, specimens of mussels were divided into five experimental groups as follows: 1) CTRL exposed to only NSW (40 psu, pH 8 ± 0.1, density 1.025 ± 0.001 g cm−3, conductivity 49 ± 1 mS cm−1 and temperature 18 ± 1 °C); 2) CNF at 1 μg L−1; 3) CNF at 1 mg L−1; 4) TOCNF at 1 μg L−1 and 5) TOCNF at 1 mg L−1 all in NSW. A natural photoperiod was maintained (18
:
6); water was renewed every 24 h, and organisms were not fed during the exposure time according to the protocol by Liberatori et al.57 Three independent experiments were carried out with a total of three replicates for each exposure concentration. After 48 h, specimens were removed from water, the hemolymph was removed from the adductor muscle, and the gills and digestive glands were dissected according to the protocols used for the following assays: neutral red retention time assay (NRRT) in mussel's hemocytes to assess lysosomal membrane stability; P-glycoprotein (P-gp) efflux assay in gill biopsies to assess potential impairment of ATP binding cassette (ABC) efflux activity; enzymatic activities, such as cholinesterase (ASCh–ChE), carboxylesterase (CbE), glutathione-S-transferase (GST), glutathione peroxidase (GPx), catalase (CAT), and glutathione reductase (GR). Lipid peroxidation (LPO), total protein content in the gills and digestive glands and ASCh–ChE in the hemolymph were also considered.
Neutral red retention time (NRRT) assay in mussel hemocytes.
NRRT assay was conducted on mussel's hemocytes according to the method of Lowe et al.58 Mussel's hemolymph was removed from the adductor muscle using a sterile syringe (1 mL) pre-loaded with a buffer solution aiming to prevent hemocyte clotting (20 mM 4-(2-hydroxyethyl)-1-piperazineethanesulfonic acid (HEPES), 436 mM NaCl, 53 mM MgSO4, 12 mM KCl, and 10 mM CaCl2). 200 mL of the obtained hemocyte suspension was placed in a coverslip (22 × 22 mm) in the dark at 18 °C and replaced with 200 mL of neutral red dye solution (0.1 mg mL−1 NR in dimethyl sulfoxide, DMSO). The slides were then rinsed twice with buffer solution to remove excess NR and observed under an Olympus microscope at 80× magnification. The percentage of hemocytes showing loss of NR dye from the lysosomes was scored every 15 min. Three replicates of each slide were run in parallel, each combining hemolymph from at least five individuals for each experimental group. The end point was set as the time when 50% of the hemocytes showed signs of NR lysosomal leakage and the data are shown as% of lysosomal damage (LMS) (% of control).
P-glycoprotein efflux assay in mussel gill's biopsies.
The P-gp efflux assay was performed on gill biopsies according to the method of Neyfakh et al.59 modified by Luckenbach et al.60 Incubations of 10 gill biopses (AcuPunch, 6 mm) per experimental group obtained from 3 pools of 10 individuals were performed using Petri dishes in 40 mL of NSW plus RhB (1 μM) in the dark with gentle shaking by applying Sunflower Mini-Shaker PS-3D. A control group was further exposed to the P-gp efflux known inhibitor verapamil (1 μM) as a positive control. After 90 min of incubation at room temperature, each biopsy was rinsed twice in NSW and then quickly transferred to Eppendorf tubes containing 300 μL of hypotonic lysis buffer (10 mM KCl, 1.5 mM MgCl2, 10 mM Tris pH 7.4) and sonicated using the tip Bandelin (30 seconds each, 70% of power). Sonicates were centrifuged at 13
000 rpm for 10 minutes, and 200 μL of the supernatant was transferred to 96-well black microplates. RhB fluorescence was measured using Victor 3 1420 Multilabel Counter (Wallac software) at Ex 522–Em 586. The data are expressed as arbitrary units of fluorescence (AUF).
Oxidative stress biomarkers and cholinergic enzymes.
A portion of gill tissue (approx. 400 mg) was homogenised (1
:
4, w/v) in 50 mM ice-cold phosphate buffer pH 7.4 containing 1 mM EDTA, while a similar amount of digestive gland tissue was homogenised (1
:
5, w/v) in 100 mM potassium phosphate buffer pH 7.4 containing 150 mM KCl, 1 mM EDTA, and 1 mM dithiothreitol (DTT), in both cases using a Polytron blender. The tissues were independently processed (n = 6 each), and the corresponding homogenates were centrifuged at 10
000 rpm for 30 min at 4 °C. The resulting supernatant (S10) was used for biochemical determinations. A detailed description of the adopted protocols for the biochemical determination of oxidative stress markers (CAT, GR, GST, GPX and LPO) and xenobiotic phase I carboxylesterase activity (CbE) using two commercial substrates (p-nitrophenyl acetate (p-NPA) and p-nitrophenyl butyrate (p-NPB)) can be found in Dallarés et al.61 for mussels, as shown in ESI† material. All the activities were determined at 25 °C in 96-well plates using the spectrophotometer infinite M200 by TECAN, with Magellan software (V6.2 or V1.6). Enzymatic activities are expressed as nmol min−1 mg per protein, except CAT, in μmol min−1 mg per protein and the LPO content in nmol/MDAg w/w. Acetylcholinesterase activity (ASCh–ChE) was measured in whole hemolymph, gills and digestive glands using the method introduced by Ellman et al.62 with modifications adapted according to the tissue. For hemolymph, 50 μL were mixed with 5,5′-dithio-bis-2-nitrobenzoate (DTNB) and acetylthiocholine (ASCh) (both at 1 mM final well concentration), while 25 μL of undiluted S10 gill and digestive gland extracts was mixed with DTNB (180 μM) and ASCh (1 mM). Purified eel AChE was used as a positive control (CAS 900-81-1) for methodological purposes. Kinetics were read at 405 nm for 5 min (ε = 13.6 mM−1 cm−1). The total protein content of the tissue homogenates was measured following the method introduced by Bradford et al.63
Statistical analysis
Statistical analysis was performed using STATISTICA™ software with one-way ANOVA and Bonferroni multiple comparison tests to assess differences between experimental groups with controls and between the two tested concentrations of CNF and TOCNF. For all data analysed, the statistical significance level was set at p < 0.05.
Results
Synthesis and characterization of CNF and TOCNF
In this eco-toxicological study on marine mussels, the uptake and sub-lethal responses of two types of CNF were investigated. These nanofibers are characterized by different chemical and physical properties derived from several combinations of enzymatic and oxidative pre-treatments and mechanical treatments in the synthetic process. TOCNF are TEMPO-oxidized CNF characterized by the presence of carboxylic moieties on the C6 glucopyranosic unit of the chain, showing an oxidation degree of 1.55 mmolCOOH gTOCNF−1, determined by the procedure reported in the Materials and methods section. CNF is characterized by the absence of the carboxyl group on the fiber backbone, with only the presence of hydroxyl groups on the polymer chain. This difference is clearly visible by recording the FT-IR spectra of the two materials, as shown in Fig. 1. TOCNF exhibits a signal at 1722 cm−1, characteristic of the carbonyl of the carboxyl group (–COOH), which is absent in the CNF spectrum.
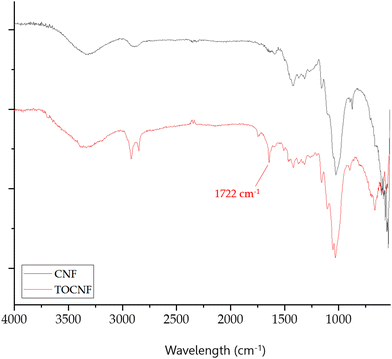 |
| Fig. 1 FTIR spectra of CNF (black line) and TOCNF (red line) with the distinctive spectra of TOCNF at 1722 cm−1. | |
Another difference between the two types of CNFs can be found in the pHPZC, namely the pH above, in which the total surface of the material is negatively charged. The presence of carboxyl groups on the surface of TOCNF leads to the measurement of a pHPZC of 4.01, while a value of 7.10 is measured for non-oxidized CNF. Therefore, it can be concluded that the –COOH moieties on TOCNF give a negatively charged material at neutral pH, while CNFs have a pHPZC much closer to neutrality. Moreover, XRD analysis showed different degrees of crystallinity between CNF and TOCNF. Specifically, the diffractogram showed that CNF had a crystallinity index of 75.3%, which is ten percentage points higher than the crystallinity index of TOCNF (65.3%) (see diffractograms in ESI† materials Fig. S1). This difference could be associated with the different pretreatments used to produce CNF and TOCNF.
CNF and TOCNF characterization in water suspensions (MilliQ, ASW and NSW)
Morphological characterization of CNF and TOCNF by TEM analysis confirmed a nanometric diameter and a micrometric length size of either CNF or TOCNF upon dispersion in each of the three water media under investigation: MilliQ water (Fig. 2A and D), ASW (Fig. 2B and E) and NSW (Fig. 2C and F). No substantial differences were observed in either CNF or TOCNF morphology upon water dispersion regardless of the absence/presence of ionic species and colloidal particles (inorganic and organic as natural organic matter, NOM), which are known to play a significant role in the transformations encountered by the nanoscale material in water media.64,65
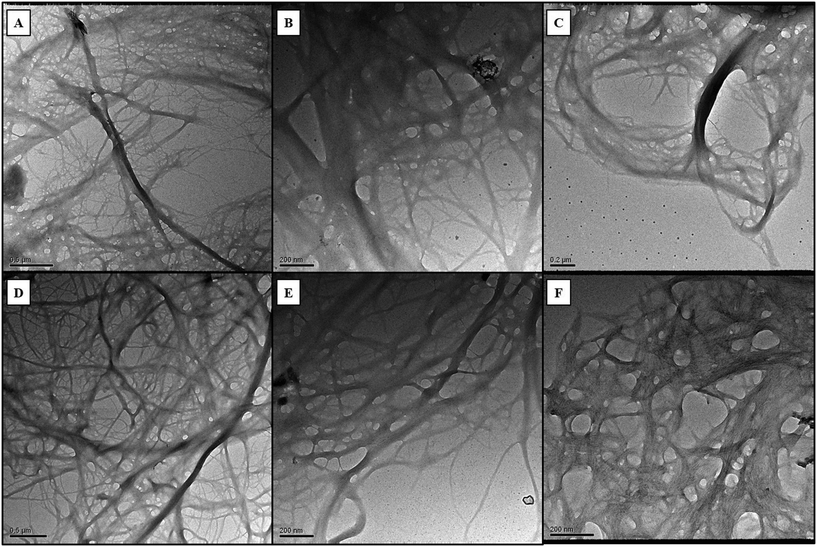 |
| Fig. 2 TEM images of CNF and TOCNF suspensions in MilliQ water (A - CNF; D - TOCNF), ASW (B - CNF; E - TOCNF) and NSW (C - CNF; F - TOCNF). | |
Similarly, no significant changes over time in the pH and salinity of the three water media were observed upon incubation of CNF and TOCNF at 1 mg L−1; values constantly resembled those of unspiked waters (controls) even after 6 weeks of incubation (see Table S1 in ESI† material). However, upon short sonication (15 min at 70 mV), the fiber morphology changed, appearing not entirely aggregated, as observed before sonication (Fig. 3). In general, CNF was more affected by sonication with the formation of smaller and less condensed aggregates of fibers (as shown in Fig. 3b, d and f compared to Fig. 3a, c and e) regardless of the three water media used (MilliQ, ASW and NSW). Conversely, TOCNF resulted in fewer aggregates formed after sonication only in the case of NSW media with fibers appearing more dispersed compared to the non-sonicated ones (Fig. 3l and k). In contrast, sonication did not modify the TOCNF aggregates in the ASW and MilliQ suspensions (Fig. 3h and j); in fact, no significant change in the shape of the fibers was observed compared to those not sonicated (Fig. 3g and i). Therefore, TOCNFs appeared to be more affected by sonication only in NSW, and as the fibers were more dispersed, they could potentially be more easily uptaken by mussels.
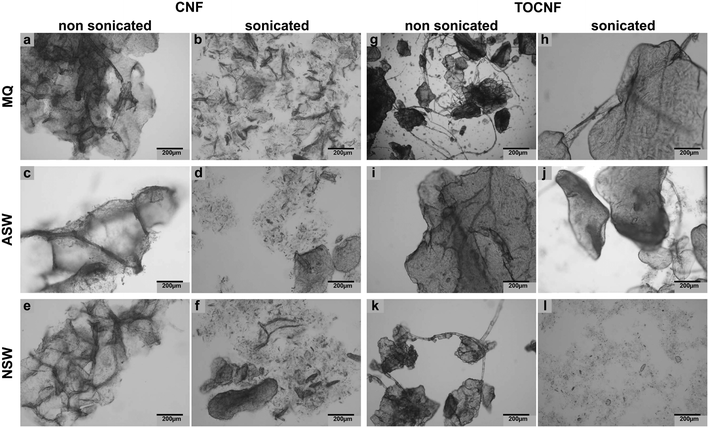 |
| Fig. 3 Light microscopy of CNF and TOCNF suspension in three water media (80×). CNF not sonicated and sonicated in MilliQ (a and b), ASW (c and d) and NSW (e and f). TOCNF not sonicated and sonicated in MilliQ (g and h), ASW (i and j) and NSW (k and l). | |
ζ potential values measured by DLS confirmed the higher negative surface charges of TOCNF compared to CNF in MilliQ or NSW at time 0 and after 24 h of incubation (Table 1). Similarly, labelled stocks showed similar values to TOCNF–RhB, with significantly higher negative ζ potential values compared to CNF–RhB except for 24 h incubation in NSW as for unlabelled ones.
Table 1
ζ potential values of CNF, TOCNF, RhB–CNF and RhB–TOCNF suspensions in MQ and NSW (1 mg mL−1). Measurements were performed at time 0 and after 24 h of incubation. Data are shown as mean values ± standard deviation
|
t0 |
24 h |
mQ |
NSW |
mQ |
NSW |
CNF |
−3.69 ± 1.90 |
−2.84 ± 5.44 |
−1.58 ± 0.19 |
−12.46 ± 5.91 |
TOCNF |
−30.0 ± 4.23 |
−9.05 ± 8.85 |
−19.23 ± 2.46 |
−9.19 ± 3.96 |
CNF–RhB |
−7.55 ± 2.81 |
−5.88 ± 2.31 |
−1.64 ± 0.38 |
−14.02 ± 3.47 |
TOCNF–RhB |
−5.27 ± 0.63 |
−11.64 ± 0.82 |
−3.59 ± 0.13 |
−6.47 ± 2.80 |
Ecotoxicity assessment of CNF and TOCNF in marine mussels
CNF and TOCNF uptake in mussels.
Evidence of CNF and TOCNF uptake and distribution in mussel's hemolymph upon 24 h waterborne exposure to 1 mg L−1 was provided by light microscopy with neutral red staining hemocytes attached to them, as shown in Fig. 4. Conversely, only RhB–CNF was found in all tissues investigated and at tested concentrations (1 μg L−1 and 1 mg L−1); any TOCNF was found neither as individual fibers nor fragments probably because of the nano-defibrillation in NSW previously described upon sonication (Fig. 3l).
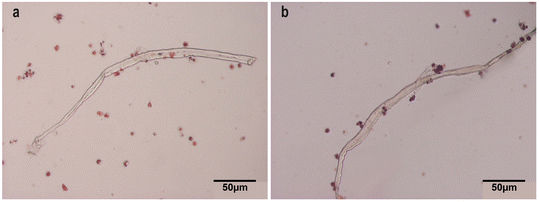 |
| Fig. 4 Light microscopy of CNF and TOCNF in hemolymph. Hemocytes stained with neutral red (20×). (a) CNF (1 mg L−1); (b) TOCNF (1 mg L−1). Note that hemocytes surround the fibers in both cases. | |
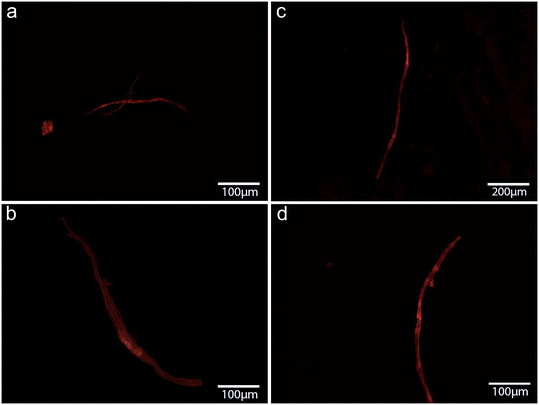 |
| Fig. 5 Fluorescence microscopy images of CNF stained with RhB found in mussels in vivo exposed to CNF at 1 μg L−1 in (a) gills and (b) hemolymph and at 1 mg L−1 in (c) gills (d) and hemolymph (80×). | |
As a confirmation that RhB was not leaching from CNF and TOCNF upon suspension in NSW, fluorescence microscopy showed the stability of the dye for both CNF and TOCNF at 1 mg mL−1 for 24 h incubation, as shown in Fig. S2 and S3.†
Biological effects upon acute waterborne exposure
Lysosomal membrane stability.
The confirmation of CNF and TOCNF interactions with the lysosomal membranes of mussel hemocytes was provided by a neutral red retention time (NRRT) assay. The results showed an increasing trend in the destabilization of the lysosomal membrane over time from 15 to 45 min in all experimental groups, including the control (Fig. 6 and Table S2†). Overall, a higher percentage of destabilized hemocytes was reached at the lowest exposure concentration of 1 μg L−1 (about 90%), while at the acute dose, it was circa 70% and only significant for CNF-treated mussels. For the group exposed to 1 μg L−1, statistically significant differences are observed between the CNF group and the control at 15 min (p = 0.001) (Fig. 6A) and after 30 min (6B) for both the CNF and TOCNF groups (p = 0.0001 and p = 0.0001, respectively) lasting even after 45 minutes (6C).
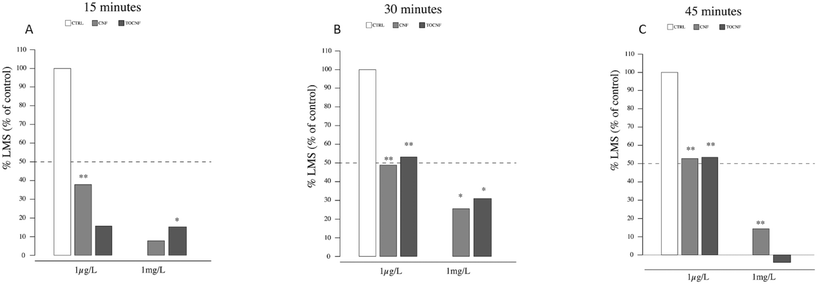 |
| Fig. 6 Neutral red retention time (NRRT) assay in hemocytes of mussels exposed to 1 μg L−1 and 1 mg L−1 of CNF and TOCNF after 15 min (A), 30 (B) and 45 min (C). Data shown as % LMS vs. control (% control). The asterisks on the top of the bars show significant differences with the control groups at each time point (*p < 0.05; **p < 0.01). | |
In the case of mussel hemocytes exposed to 1 mg L−1 of TOCNF, a significant number of destabilized lysosomes compared to the control were observed at 15 min (p = 0.039) (Fig. 6A). After 30 min (6B), mussels exposed to CNF or TOCNF showed a similar degree of lysosomal destabilization compared to the controls (p = 0.032 and p = 0.033, respectively). After 45 min minutes (Fig. 6B), only mussels exposed to CNF showed enhanced destabilized lysosomes compared to the control and TOCNF groups (p = 0.0004). Hemocytes from mussels exposed to both NC fibers (1 μg L−1 and 1 mg L−1) experienced the same 20% increase in destabilized lysosomes during the 45 min assay (Fig. 6C).
P-glycoprotein efflux activity
CNF and TOCNF were able to affect mussels' gill P-glycoprotein efflux pump activity with an almost 2-fold significant increase in RhB content even at the lowest exposure concentration of 1 μg L−1(CNF, p = 0.0005 and TOCNF, p = 0.004) compared to controls (in NSW only) and the known P-gp inhibitor verapamil (p = 0.001) (Fig. 7 and Table S3†). A higher inhibitory effect on P-gp efflux activity was observed for 1 mg L−1 with over a 3-fold increase in RhB content and equally significant for CNF and TOCNF compared to controls and even higher than verapamil (p = 0.0001). The inhibitory effect of verapamil was similar to that of CNF and TOCNF only at the lowest concentration tested (1 μg L−1) (Fig. 7).
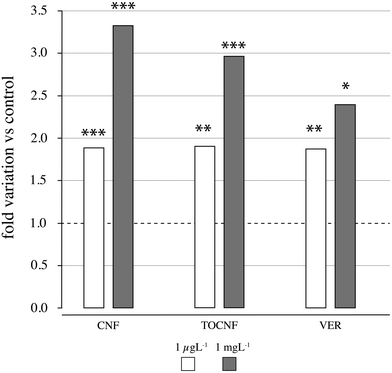 |
| Fig. 7 P-gp efflux activity in the gills of CNF and TOCNF-exposed mussels (1 μg L−1 and 1 mg L−1, respectively) and to verapamil (VER, 1 μM) as a positive control. Data shown as fold variation in RhB content compared to controls. The asterisks on the top of the bars show significant differences with the respective control groups (*p < 0.05; **p < 0.01 and ***p < 0.001). | |
Enzymatic activities
Out of the enzymatic measures related to neurotoxicity (ASCh–ChE), oxidative stress (antioxidant responses and LPO) and detoxification metabolism (CbEs and GCT), only the disruption of ASCh–ChE activities was consistent with the former toxicological effects described on haemocytes and gills. As shown in Fig. 8A, lower ASCh–ChE activities than controls were observed in mussels exposed to TOCNF at 1 μg L−1 (p = 0.001), while a similar trend was also observed in the gills and digestive glands, and no significant differences were found (p > 0.05). CNF exposure was also responsible for reduced ASCh–ChE activities in the three tested tissues but without significant differences compared to controls (p > 0.05). In an acute exposure scenario (Fig. 8B), ASCh–ChE activities significantly reduced hemolymph (p = 0.0001) but also gills (p = 0.032) and digestive glands (p = 0.044) of mussels exposed to TOCNF. Exposure to CNF was responsible only for decreased activity in the gills (p = 0.003) compared to the control group (Table S4†).
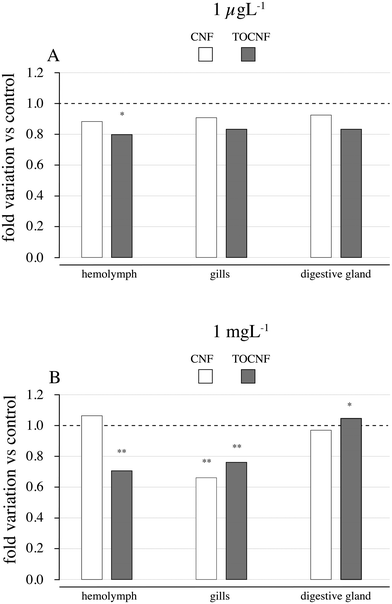 |
| Fig. 8 ASCh–ChE activity in the hemolymph, gills and digestive glands of mussels exposed to 1 μg L−1 (A) and 1 mg L−1 (B) of CNF and TOCNF. Data shown as the fold variation of ASCh–ChE activity vs. controls. The asterisks on the top of the bars show significant differences among control groups (*p < 0.05; **p < 0.01). | |
The biomarker responses in the gills and digestive glands (catalase, GST, GR, GPX(CHP), GPXH2O2, LPO, pNPA-CE, and pNPB-CE) in the gills and digestive glands are shown in Table 1. CAT activity was significantly reduced in the gills by both TOCNF (1 mg L−1) (p = 0.009) and CNF (p < 0.001) vs. controls. GR increased in the gills of TOCNF-exposed mussels vs. CNF (p = 0.00001) and controls (p = 0.003). Similarly, GPX measured as total (CHP) or the Se-dependent (H2O2) form increased in gills upon TOCNF exposure but only at the highest concentrations vs. control (p = 0.014 – p = 0.0005) and CNF (p = 0.02 – p = 0.0003). Lipid peroxidation (LPO) seemed not to be affected by either CNF or TOCNF at both concentrations. An enhancement of LPO levels is usually the result of oxidative damage to lipid membranes. They are expected to increase during ROS formation when antioxidant defenses are unable to counteract these oxy radicals. However, a decrease in LPO can also result from decreased lipid content or a modification of its composition (with lipids that are not so prone to suffer peroxidation).
Regarding CbE activities, a significant increase was observed in the gills of mussels exposed to CNF (1 μg L−1) (p = 0.013) vs. control, while a significant reduction was observed in those exposed to TOCNF (1 mg L−1) (p = 0.0001) compared to control and CNF (p = 0.00003). Hydrolysis rates with pNPB, potentially indicating other CbE isoforms, confirm such a trend but only at the highest concentration for CNF (p = 0.003). No effects were observed in the digestive glands although higher activities were recorded in line with the detoxification role of this enzyme (Table 2).
Table 2 Activity of catalase, GST, GR, GPXCHP, GPCH2O2, LPO, pNPA-CbE, and pNPB-CbE in mussel gills and digestive glands. The asterisks on the top of the bars show significant differences versus the control groups (*p < 0.05, **p < 0.01 and ***p < 0.001). Dollar symbols show significant differences between the two exposed groups ($p < 0.05, $$p < 0.01, $$$p < 0.001). CAT activity was expressed as μmol min−1 mg per protein, LPO was expressed as nmol/MDAg w/w, and the activity of the other enzymes was expressed as nmol min−1 mg per protein. The number of biological replicates was n = 6 for the gills and n = 4 for the digestive gland
Concentration tested |
1 μg L−1 |
1 mg L−1 |
Tissues |
Gill |
Digestive gland |
Gill |
Digestive gland |
Catalase |
Control |
6.57 ± 0.80 |
38.4 ± 3.02 |
8.67 ± 0.95 |
37.6 ± 4.32 |
CNF |
7.89 ± 0.72 |
45.6 ± 4.07 |
8.98 ± 0.41 |
42.5 ± 6.97 |
TOCNF |
7.78 ± 0.88 |
34.1 ± 1.67 |
5.45 ± 0.36**$$$ |
45.5 ± 8.18 |
GST |
Control |
46.1 ± 1.78 |
37.4 ± 2.46 |
49.8 ± 2.58 |
43.5 ± 3.63 |
CNF |
52.9 ± 4.58 |
43.5 ± 2.05 |
42.4 ± 2.22 |
40.4 ± 9.04 |
TOCNF |
45.1 ± 3.00 |
36.3 ± 6.34 |
43.6 ± 1.45 |
41.4 ± 6.27 |
GR |
Control |
12.4 ± 1.68 |
11.5 ± 0.96 |
16.9 ± 0.55 |
11.7 ± 1.22 |
CNF |
8.3 ± 0.74 |
12.5 ± 0.78 |
19.2 ± 0.93 |
12.6 ± 1.78 |
TOCNF |
19.5 ± 0.83**$$$ |
11.8 ± 1.02 |
21.9 ± 3.58** |
13.8 ± 1.59 |
GPX (CHP) |
Control |
7.18 ± 0.47 |
1.10 ± 0.11 |
5.52 ± 0.24 |
1.52 ± 0.45 |
CNF |
7.51 ± 0.55 |
1.24 ± 0.09 |
5.42 ± 0.26 |
1.32 ± 0.02 |
TOCNF |
7.98 ± 0.6 |
1.24 ± 0.17 |
7.27 ± 0.55*$ |
1.46 ± 0.16 |
GPX (H2O2) |
Control |
32.1 ± 2.11 |
6.77 ± 0.40 |
33.2 ± 1.07 |
8.01 ± 1.13 |
CNF |
33.7 ± 2.95 |
8.05 ± 0.72 |
30.9 ± 1.57 |
9.09 ± 0.66 |
TOCNF |
30.5 ± 2.32 |
7.91 ± 0.30 |
45.4 ± 2.22***$$$ |
10.4 ± 0.43 |
LPO |
Control |
46.1 ± 3.18 |
224.7 ± 2.66 |
58.5 ± 1.26 |
300.3 ± 11.94 |
CNF |
57.7 ± 1.13 |
206.2 ± 2.92 |
57.7 ± 1.13 |
209.0 ± 15.63 |
TOCNF |
39.4 ± 2.23 |
185.6 ± 2.92 |
39.3 ± 2.45 |
264.00 ± 13.29 |
pNPA-CbE |
Control |
9.4 ± 0.33 |
26.8 ± 0.96 |
12.7 ± 0.42 |
31.1 ± 3.13 |
CNF |
12.0 ± 0.79* |
26.9 ± 1.17 |
12.7 ± 0.49 |
30.4 ± 4.34 |
TOCNF |
10.2 ± 0.28 |
30.3 ± 1.8 |
9.7 ± 0.09 ***$$ |
35.7 ± 6.8 |
pNPB-CbE |
Control |
25.5 ± 0.85 |
60.6 ± 2.13 |
33.8 ± 1.07 |
73.8 ± 3.2 |
CNF |
39.5 ± 2.88 |
61.5 ± 8.52 |
37.1 ± 0.73 ** |
63.5 ± 10.9 |
TOCNF |
33.1 ± 1.24 |
65.4 ± 7.07 |
30.3 ± 1.06 *$$ |
82.2 ± 18.60 |
Discussion
Nanocellulose is becoming an emerging material, and estimates of surface water concentrations require a safety assessment for aquatic biota.13,24 Herein, we assess for the first time the environmental safety of non-oxidized (CNF) and TEMPO-oxidized CNF (TOCNF) for a marine filter-feeder M. galloprovincialis along with fiber stability in NSW, mussel's uptake and sub-lethal effects.
Behaviour of CNF and TOCNF in water media
No changes in pH values and salinity of CNF and TOCNF suspensions in MilliQ, ASW and NSW media were observed, which supports their environmental safety with regards to in situ applications, such as seawater treatment.66 Slight decreases in seawater pH (even by −0.1 pH units from natural 8.1 average values) affect the calcification of marine gastropods with reduced shell thickness and mantle formation at the mid-trochophore/ D-veligers stage of M. galloprovincialis.67,68 The accumulation, clearance and toxicity of emerging marine pollutants (i.e., heavy metals, flame retardants and PFCs) in marine mussels have been documented to be affected by slight decreases in seawater pH with implications for human health.69 Changes in salinity can also significantly affect marine life and even little shifts as those affecting marine coastal waters have been recently reported to have serious effects on metabolism, oxidative status and immune responses of mussels.70 Therefore, the absence of effects on the salinity and pH of seawater supports safe in situ applications of CNF and TOCNF.
Effect of sonication on CNF and TOCNF stability in water suspensions
The sonication protocol was designed to resemble the process of the natural breakdown of NC fibers owing to the wave motion that naturally occurs in marine coastal areas. By mimicking such a natural process, TOCNF ended up with fewer aggregations compared to CNF with fibers more dispersed in NSW probably owing to a process of nano-defibrillation (Fig. 3). Thus, TOCNF could be more bioavailable to mussels and more easily translocated inside the body. TOCNF–RhB was found in the hemolymph but not in the gills and digestive glands; cilia movement during the natural filter-feeding process may have reduced TOCNF retention in the gills and favor their transfer through the circulatory system to the hemolymph. In addition, the higher negative surface charges of TOCNF compared to CNF as shown by ζ potential values and pHPZC at neutral pH could have played a repulsive role and reduced fiber aggregation favoured by the presence of NOM and other organic colloids in NSW.71,72 Similarly, the presence of water inside the mussel's mantle cavity and open circulation could also explain the presence of both TOCNF and CNF in the hemolymph. The use of stable RhB-labelled NC fibers (Fig. S2 and S3†) facilitates the understanding of waterborne uptake and tissue distribution in marine mussels in agreement with previous studies.38
Ecotoxicological effects
It has been reported that exposure to microfibers can interfere with biological functions in mussels as a consequence of their bioaccumulation and internal translocation.72 Surface adherence, rather than ingestion, has been shown to cause microplastic accumulation in mussel's organs not involved in detoxification, such as the gills and mantle.44 Our previous findings revealed that the uptake of cellulose nanosponges by mussels through water with higher retention in mantle ridges.57 Although the presence of NC fibers was unobserved in the digestive glands of mussels in this study, it is also unlikely that the translocation of both types of NC fibers from the gills and the hemolymph may have reached the organ devoted to metabolism owing to the short-term exposure (48 h). In agreement with this, biological responses are more evident in the hemolymph and gills than in the digestive gland (Table 1). As shown in Fig. 4, haemocyte adhesion on both NC fibers could explain the observed destabilization of lysosomal membranes (% LMS) even at the lowest concentrations (1 μg L−1). The use of NR dye is a very sound technique for evaluating the hemocyte viability of mussels as the integrity of the lysosomal membrane is responsible for digestive processes and the sequestration of xenobiotics from the cytoplasm.58,73 Considering the key role of hemocytes in the immunity of mussels, an immune-related pathway alteration by CNF and TOCNF exposure is hypothesized. Similarly, P-glycoprotein efflux activities were inhibited by CNFs in the gills, and CNF and TOCNF were visually tracked (Fig. 5). However, in this case, the acute dose (1 mg L−1) was responsible for a higher accumulation of RhB even to a greater extent than the inhibitory effect of the model pump blocker verapamil (Fig. 5).74 A concentration-dependent effect on both CNF fibers on pump efflux can be hypothesized compared to the inhibition caused by verapamil at realistic environmental concentrations (1 μg L−1). Being responsible for a multi-xenobiotic resistance mechanism, P-gp efflux activity is considered the first barrier towards the uptake of toxic compounds by the gills and hemocytes of mussels.75 Inhibition of P-gp efflux activity could affect the disposition of other toxic chemicals within the cell and, in worst-case scenarios, increase their accumulation with an off-set of cytotoxicity.60
Regardless of the specific organ investigated, CN fibers were also able to inhibit ASCh–ChE activity with stronger inhibitory effects in mussels exposed to TOCNF than in those exposed to CNF (Fig. 7). These results agree with previous observations of greater fiber dispersion in NSW and stronger surface adhesion to the cell membrane, which can override electrostatic repulsion with TOCNF negative charges.52,76 In addition, mussel gill cells are covered by a mucus made of acid and neutral polysaccharides with an active role in particle ingestion via cilia-transported mucus masses.77 Although no concentration-dependent effects were observed in ASCh–ChE activity, both CN fibers affected the cholinergic function via an indirect mechanism (i.e., cell membrane interaction) rather than direct binding to the AChE enzyme. Similarly, P-gp efflux inhibition of CNF could be observed because of membrane interaction rather than directly within the efflux protein binding sites. Both AChE and P-gp are complex transmembrane proteins bearing, respectively, highly selective and unselective binding sites, both of which require membrane integrity to perform their role. Any disruption/alteration on the cell membrane caused, for instance, by NC fiber adhesion, could have consequences for their function. Cytoskeleton disruption and impaired lysosomal function have been reported in human lung adenocarcinoma (A549) cells exposed to graphite carbon nanotube fibers (48 h) via the induction of oxidative stress and destabilization of lysosomes.78 Moreover, the same authors observed that GCNT fibers damage mitochondria with a significant reduction in ATP levels and, in general, an energetic impairment of exposed cells. A significant decrease in ATP levels has been reported upon acute short-term exposure to cellulose nanofibers (1–500 mg mL−1) of the freshwater microalgae Chlorella vulgaris, indicating a loss of mitochondrial function.79 The pump P-gp is actively transporting substrate outside of the cells through the use of ATP. Therefore, the observed alteration in the mussel's gills could have involved the depletion of ATP by CNFs. In addition, impairment in the energetic metabolism of the cells could have more severe consequences, such as compromised cell survival. Previous observations in hemocytes and gills support the close relationship between the immune and neuroendocrine systems in mussels and the likelihood that the cholinergic system could also be regarded as a defense mechanism.80 Similarly, there is a demonstrated link between inflammatory and oxidative stress processes as well as between oxidative stress responses and xenobiotic metabolism in bivalves.81,82 However, our results in oxidative stress-related parameters and xenobiotic transformation reactions did not seem to be affected by NC exposures. Oxidative stress caused by the induction of reactive oxygen species has been recognized as the most common pathway affected by nanomaterial exposure and has recently been evoked in NC.29,40 However, to the best of our knowledge, very little has been achieved due to the limited number of studies on NC fibers in either human models or aquatic species. A significant increase in intracellular ROS levels has been reported by Wang et al.40 in freshwater algae Scenedesmus obliquus, Daphnia magna and Danio rerio upon short-term exposure to wood-based and cotton-based cellulose nanocrystals (4–10 nm and length 100–500 nm), cotton-based CNFs (4–10 nm and length 1000–3000 nm) and cotton-based CNC in the range of 1–10 mg L−1.
In agreement with the short-term time-frame exposure of our study, together with the lack of consistent and significant alterations in digestive gland biomarker responses, further prolonged exposure studies are needed to unravel the mechanistic actions of NF in more realistic scenarios. In fact, in the gills, some alterations could be justified by the fact that it is the first contact tissue with NSW and a natural selective barrier to particle uptake. The observed lesser responsiveness of the digestive gland is supported by short-term exposure (48 h), which could have prevented translocation from gills and hemolymph. Despite the little biomarker (oxidative stress and detoxification processes) modulation observed by CNF and TOCNF, some significant traits can be pointed out: 1) gills experienced more biomarker modifications than the digestive gland upon CNF/TOCNF exposures, 2) more alterations were revealed at higher doses (1 mg L−1), 3) no damage due to oxidative stress was revealed because LPO levels were not significantly altered and 4) detoxification by CbE was enhanced upon CNF exposure, while it was inhibited by TOCNF exposure depending on the substrate used and tissue targeted. Longer-term exposure and a larger sample size are likely to help to support the present results and include chronic exposures. However, the interaction of the NC fibers, likely of a physical/mechanical nature rather than biochemical nature over the hemocytes and gills at already environmentally realistic concentrations, supports the need to increase the regulation of these smaller-sized materials so far questioned because of their bio-polymer nature. Long-term exposure scenarios could also lead to translocation phenomena in the digestive glands according to previous findings conducted on ingested microscopic plastics translocated to the circulatory system of Mytilus sp.83
The limited contributions to the acute toxicity of NC to aquatic species showed significant effects only at very high concentrations (>100 mg L−1), which is unrealistic for natural exposure scenarios.36,37,84 Even if pro-inflammatory pathways can trigger ROS production, this, in turn, is a signal of enhanced antioxidant defenses, and this is a timely process that requires adequate receptor activation, gene transduction and translation; in mussels, 48 h may be insufficient.82 Xenobiotic metabolism can also enhance ROS formation, but the nature of this stressor is not likely to induce these mechanistic defenses nor was any interference observed in the metabolic capacity as a consequence of NC exposures at the catalytic level.48 Based on the results presented above, it can thus be stated that the fibers were likely unable to enter the cells because neither changes in oxidative stress nor enzymes involved in biotransformation were observed.
Conclusions
Our findings showed that cellulose NFs, CNF and TOCNF can affect hemocyte lysosomal stability, P-gp efflux activity and cholinergic function in the gills of the mussel M. galloprovincialis at environmentally realistic concentrations (1 μg L−1). This is of ecological relevance because hemocytes are also involved in bivalve immune regulation and gills in filtration and energy uptake metabolism and growth. The nature of the disruption is likely rather physical/mechanical owing to the CNF interaction with the cell membranes. No significant and consistent alterations were observed in terms of oxidative stress markers or detoxification metabolism in the mussel's digestive gland probably because of short-term exposure conditions (48 h). Furthermore, no significant acute toxicity by CNF and TOCNF was observed in marine mussels, but the onset of potential negative effects derived from the mechanical interaction of CNF with mussel's immune cells and gill functionality deserves future attention.
Associated content
ESI† detailed description of methods for enzymatic assays are provided; additional images of CNF and TOCNF characterization together with results of enzymatic assays are provided as well.
Conflicts of interest
There are no conflicts to declare.
Acknowledgements
This study was carried out within the following projects: National Biodiversity Future Center – NBFC, received funding from the National Recovery and Resilience Plan (NRRP), Mission 4 Component 2 Investment 1.4 – Call for tender No. 3138 of 16 December 2021, rectified by Decree No. 3175 of 18 December 2021 of Italian Ministry of University and Research funded by the European Union – NextGenerationEU; Award Number: Project code CN_00000033, Concession Decree No. 1034 of 17 June 2022 adopted by the Italian Ministry of University and Research, CUP B63C22000650007; the RETURN Extended Partnership and received funding from the European Union Next-GenerationEU (National Recovery and Resilience Plan – NRRP, Mission 4, Component 2, Investment 1.3 – D.D. 1243 2/8/2022, PE0000005). To the institutional support of the ‘Severo Ochoa Centre of Excellence’ accreditation (CEX2019-000928-S). PID2021–122592NB-100 (Ministerio de Ciencia e Innovacion, Agencia Estatal de Investigacion and Fondo Europeo de Desarrollo Regional (FEDER).
References
- J. A. Shatkin, T. H. Wegner and E. M. (TED) Bilek,
et al., Market projections of cellulose nanomaterial-enabled products −Part 1: Applications, Tappi J., 2014, 13(5), 9–16 CAS.
- A. Barhoum, J. Jeevanandam and A. Rastogi,
et al., Plant celluloses, hemicelluloses, lignins, and volatile oils for the synthesis of nanoparticles and nanostructured materials, Nanoscale, 2020, 12(45), 22845–22890 RSC.
- A. Dufresne, Nanocellulose: a new ageless bionanomaterial, Mater. Today, 2013, 16(6), 220–227 CrossRef CAS.
- O. Nechyporchuk, M. N. Belgacem and J. Bras, Production of cellulose nanofibrils: A review of recent advances, Ind. Crops Prod., 2016, 93, 2–25 CrossRef CAS.
- D. Trache, M. H. Hussin, M. K. M. Haafiz and V. K. Thakur, Recent progress in cellulose nanocrystals: Sources and production, Nanoscale, 2017, 9(5), 1763–1786 RSC.
- M. Gouda, A. Hebeish and A. I. Aljafari, Synthesis and characterization of novel drug delivery systems based on cellulose acetate electrospun nanofiber mats, J. Ind. Text., 2014, 43(3), 319–329 CrossRef CAS.
- T. Abitbol, A. Rivkin and Y. Cao,
et al., Nanocellulose, a tiny fiber with huge applications, Curr. Opin. Biotechnol., 2019, 39, 76–88 CrossRef PubMed.
- O. Jeong-A, M. Aakyiir and Y. Liu,
et al., Durable cement/cellulose nanofiber composites prepared by a facile approach, Cem. Concr. Compos., 2021, 125, 104321 Search PubMed.
- T. Shahnaz, D. Bedadeep and S. Narayanasamy, Investigation of the adsorptive removal of methylene blue using modified nanocellulose, Int. J. Biol. Macromol., 2022, 200, 162–171 CrossRef CAS PubMed.
- B. Thomas, M. C. Raj and K. B. Athira,
et al., Nanocellulose, a Versatile Green Platform: From Biosources to Materials and Their Applications, Chem. Rev., 2018, 118(24), 11575–11625 CrossRef CAS PubMed.
-
P. U. Agarwal, H. Rajai Atalla and A. Isogai, Nanocelluloses: Their Preparation, Properties, and Applications, American Chemical Society, 2017, vol. 1251(9), pp. 171–189 Search PubMed.
- J. M. Silva, H. S. Barud and A. B. Meneguin,
et al., Inorganic-organic bio-nanocomposite films based on Laponite and Cellulose Nanofibers (CNF), Appl. Clay Sci., 2019, 168, 428–435 CrossRef CAS.
- P. G. Stampino, L. Riva and C. Punta,
et al., Comparative Life Cycle Assessment of Cellulose Nanofibres Production Routes from Virgin and Recycled Raw Materials, Molecules, 2021, 26, 2558 CrossRef CAS PubMed.
- K. Akatan, S. Kabdrakhmanova and T. Kuanyshbekov,
et al. Highly-efficient isolation of microcrystalline cellulose and nanocellulose from sunflower seed waste via environmentally benign method, Cellulose, 2022, 29, 3787–3802 CrossRef CAS.
- M. El Bakkari, V. Bindiganavile and J. Goncalves,
et al., Preparation of cellulose nanofibers by TEMPO-oxidation of bleached chemi-thermomechanical pulp for cement applications, Carbohydr. Polym., 2019, 203, 238–245 CrossRef PubMed.
-
K. Zhang, A. Barhoum and C. Xiaoqing, et al., Cellulose Nanofibers: Fabrication and Surface Functionalization Techniques, in Handbook of Nanofibers, ed. A. Barhoum, M. Bechelany and A. Makhlouf, Springer, Cham, 2019 Search PubMed.
- A. Meftahi, P. Samyn, S. A. Geravand, R. Khajavi, S. Alibkhshi, M. Bechelany and A. Barhoum, Nanocelluloses as skin biocompatible materials for skincare, cosmetics, and healthcare: Formulations, regulations, and emerging applications, Carbohydr. Polym., 2022, 278, 118956 CrossRef CAS PubMed.
- R. Arun, R. Shruthy, R. Preetha and V. Sreejit, Biodegradable nano composite reinforced with cellulose nano fiber from coconut industry waste for replacing synthetic plastic food packaging, Chemosphere, 2022, 291, 132786 CrossRef CAS PubMed.
- M. Pääkkö, M. Ankerfors and H. Kosonen,
et al., Enzymatic Hydrolysis Combined with Mechanical Shearing and High-Pressure Homogenization for Nanoscale Cellulose Fibrils and Strong Gels, Biomacromolecules, 2007, 8(6), 1934–1941 CrossRef PubMed.
- P. Guillaume, C. Punta and C. Delattre,
et al., TEMPO-mediated oxidation of polysaccharides: An ongoing story, Carbohydr. Polym., 2017, 165, 71–85 CrossRef PubMed.
- C. Endes, S. Camarero-Espinosa and S. Mueller,
et al., A critical review of the current knowledge regarding the biological impact of nanocellulose, J. Nanobiotechnol., 2016, 14, 78 CrossRef CAS PubMed.
-
M. Kumari, Risk assessment of nanocellulose exposure, Nano-Biosorbents for Decontamination of Water, Air, and Soil Pollution, Micro and Nano Technologies, 2022, ch. 11, pp. 243–250 Search PubMed.
- J. A. Shatkin and B. Kim, Cellulose nanomaterials: life cycle risk assessment, and environmental health and safety roadmap, Environ. Sci.: Nano, 2015, 2, 477–499 RSC.
-
N. Stoudmann, N. Nowack and C. Som, Prospective environmental risk assessment of nanocellulose for Europe, 2019, vol. 6, pp. 2520–2531 Search PubMed.
- C. Endes, S. Camarero-Espinosa and S. Mueller,
et al., A critical review of the current knowledge regarding the biological impact of nanocellulose, J. Nanobiotechnol., 2016, 14, 78 CrossRef CAS PubMed.
- M. Roman, Toxicity of Cellulose Nanocrystals: A Review, Ind. Biotechnol., 2015, 11(1), 25–33 CrossRef CAS.
- G. Pyrgiotakis, W. Luu and Z. Zhang,
et al., Development of high throughput, high precision synthesis platforms and characterization methodologies for toxicological studies of nanocellulose, Cellulose, 2018, 25, 2303–2319 CrossRef CAS PubMed.
- A. B. Seabra, J. S. Bernardes and W. J. Fávaro,
et al., Cellulose nanocrystals as carriers in medicine and their toxicities: A review, Carbohydr. Polym., 2018, 181, 514–527 CrossRef CAS PubMed.
- W. Brand, C. Petra and E. van Kesteren,
et al., Overview of potential adverse health effects of oral exposure to nanocellulose, Nanotoxicology, 2022, 16(2), 217–246 CrossRef CAS PubMed.
- V. R. Lopes, M. Strømme and N. Ferraz, In Vitro Biological Impact of Nanocellulose Fibers on Human Gut Bacteria and Gastrointestinal Cells, Nanomaterials, 2020, 10, 1159 CrossRef CAS PubMed.
- A. L. Menas, N. Yanamala and M. T. Farcas,
et al., Fibrillar vs. crystalline nanocellulose pulmonary epithelial cell responses: Cytotoxicity or inflammation?, Chemosphere, 2017, 171, 671–680 CrossRef CAS PubMed.
- J. M. G. Davis, The need for standardised testing procedures for all products capable of liberating respirable fibres: the example of materials based on cellulose, Br. J. Ind. Med., 1993, 50(2), 187–190 CAS.
- M. T. Farkas, E. R. Kisin and A. L. Menas,
et al., Pulmonary exposure to cellulose nanocrystals caused deleterious effects to reproductive system in male mice, J. Toxicol. Environ. Health, Part A, 2016, 79(21), 984–997 CrossRef PubMed.
- G. M. DeLoid, X. Cao and R. M. Molina,
et al., Toxicological effects of ingested nanocellulose in in vitro intestinal epithelium and in vivo rat models, Environ. Sci.: Nano, 2019, 6(7), 2105–2115 RSC.
- K. J. Ong, J. A. Shatkin and K. Nelson, Establishing the safety of novel bio-based cellulose nanomaterials for commercialization, Nanoimpact, 2017, 6, 19–29 CrossRef.
- T. Kovacs, V. Naish and B. O'Connor,
et al., An ecotoxicological characterization of nanocrystalline cellulose (NCC), Nanotoxicology, 2010, 4, 255–270 CrossRef CAS PubMed.
- B. J. Harper, A. Clendaniel and F. Sinche,
et al., Impacts of chemical modification on the toxicity of diverse nanocellulose materials to developing zebrafish, Cellulose, 2016, 23, 1763–1775 CrossRef CAS PubMed.
- I. Patel, J. Woodcock and R. Beams,
et al., Fluorescently Labeled Cellulose Nanofibers for Environmental Health and Safety Studies, Nanomaterials, 2021, 11(4), 1015 CrossRef CAS PubMed.
- M. Ogonowski, U. Edlund and E. Gorokhova,
et al., Multi-level toxicity assessment of engineered cellulose nanofibrils in Daphnia magna, Nanotoxicology, 2018, 12, 509–521 CrossRef CAS PubMed.
- Z. Wang, L. Song and N. Ye,
et al., Oxidative stress actuated by cellulose nanocrystals and nanofibrils in aquatic organisms of different trophic levels, NanoImpact, 2020, 2, 100211 CrossRef.
- C. G. Avio, L. Pittura and G. D'Errico,
et al., Distribution and characterization of microplastic particles and textile microfibers in Adriatic food webs: General insights for biomonitoring strategies, Environ. Pollut., 2020, 258, 113766 CrossRef CAS PubMed.
- G. Suaria, A. Achtypi and V. Perold, Microfibers in oceanic surface waters: A global characterization, Sci. Adv., 2020, 6, 8493–8501 CrossRef PubMed.
- J. Kwak, H. Liu and D. Wang,
et al., Critical review of environmental impacts of microfibers in different environmental matrices, Comp. Biochem. Physiol., Part C: Toxicol. Pharmacol., 2022, 251, 109196 CAS.
- P. Kolandhasamy, L. Su and J. Li,
et al., Adherence of microplastics to soft tissue of mussels: A novel way to uptake microplastics beyond ingestion, Sci. Total Environ., 2018, 610–611, 635–640 CrossRef CAS PubMed.
- M. Cole, C. Liddle and G. Consolandi,
et al., Microplastics, microfibres and nanoplastics cause variable sub-lethal responses in mussels (Mytilus spp.), Mar. Pollut. Bull., 2020, 160, 111552 CrossRef CAS PubMed.
- J. S. Choi, K. Kim and S. H. Hong,
et al., Impact of polyethylene terephthalate microfiber length on cellular responses in the Mediterranean mussel Mytilus galloprovincialis, Mar. Environ. Res., 2021, 168, 105320 CrossRef CAS PubMed.
- J. S. Choi, K. Kim and K. Park,
et al., Long-term exposure of the Mediterranean mussels, Mytilus galloprovincialis to polyethylene terephthalate microfibers: Implication for reproductive and neurotoxic effects, Chemosphere, 2022, 299, 134317 CrossRef CAS PubMed.
- M. Volgare, S. Santonicola and M. Cocca,
et al., A versatile approach to evaluate the occurrence of microfibers in mussels Mytilus galloprovincialis, Sci. Rep., 2022, 12, 21827 CrossRef CAS PubMed.
- L. Pittura, A. Nardi and M. Cocca,
et al., Cellular disturbance and thermal stress response in mussels exposed to synthetic and natural microfibers, Front. Mar. Sci., 2022, 9, 981365 CrossRef.
- L. Riva, N. Pastori, A. Panozzo, M. Antonelli and C. Punta, Nanostructured Cellulose-Based Sorbent Materials for Water Decontamination from Organic Dyes, Nanomaterials, 2020, 10, 1570 CrossRef CAS PubMed.
- A. Isogai, S. Tsuguyuki and H. Fukuzumi, TEMPO-oxidized cellulose nanofibers, Nanoscale, 2011, 3, 71–85 RSC.
- A. Rossetti, A. Paciaroni and B. Rossi,
et al., TEMPO-oxidized cellulose nanofibril/polyvalent cations hydrogels: a multifaceted view of network interactions and inner structure, Cellulose, 2023, 30, 2951 CrossRef CAS.
- M. V. Lopez-Ramon, F. Stoeckli and C. Moreno-Castilla,
et al., On the characterization of acidic and basic surface sites on carbons by various techniques, Carbon, 1999, 37(8), 1215–1221 CrossRef CAS.
- L. Segal, J. J. Creely and C. M. Conrad,
et al., An Empirical Method for Estimating the Degree of Crystallinity of Native Cellulose Using the X-Ray Diffractometer, Text. Res. J., 1965, 29(10), 786–794 CrossRef.
- W. Ruangudomsakul, C. Ruksakulpiwat and Y. Ruksakulpiwat, Preparation and Characterization of Cellulose Nanofibers from Cassava Pulp, Macromol. Symp., 2015, 354(1), 170–176 CrossRef CAS.
- M. P. Robin and R. O'Reilly, Strategies for preparing fluorescently labelled polymer nanoparticles, Polym. Int., 2015, 64(2), 174–182 CrossRef CAS.
- G. Liberatori, G. Grassi and P. Guidi,
et al., Effect-Based Approach to Assess Nanostructured Cellulose Sponge Removal Efficacy of Zinc Ions from Seawater to Prevent Ecological Risks, Nanomaterials, 2020, 10, 1283 CrossRef CAS PubMed.
- D. M. Lowe and V. U. Fossato,
et al., Contaminant induced lysosomal membrane damage in blood cells of mussels Mytilus galloprovincialis from the Venice Lagoon: an in vitro study, Mar. Ecol.: Prog. Ser., 1995, 129, 189–196 CrossRef.
- A. Neyfakh, Use of fluorescent dyes as molecular probes for the study of multidrug resistance, Exp. Cell Res., 1989, 174, 168–176 CrossRef PubMed.
- T. Luckenbach and D. Epel, ABCB- and ABCC-type transporters confer multixenobiotic resistance and form an environment-tissue barrier in bivalve gills, Am. J. Physiol., 2008, 294(6), 1919–1929 Search PubMed.
- S. Dallarés, N. Carrasco and D. Álvarez-Muñoz,
et al., Multibiomarker biomonitoring approach using three bivalve species in the Ebro Delta (Catalonia, Spain), Environ. Sci. Pollut. Res., 2018, 25, 36745–36758 CrossRef PubMed.
- G. L. Ellman, K. D. Courtney and V. Andres,
et al., A new and rapid colorimetric determination of acetylcholinesterase activity, Biochem. Pharmacol., 1961, 7(2), 88–95 CrossRef CAS PubMed.
- M. M. Bradford, A rapid and sensitive method for the quantitation of microgram quantities of protein utilizing the principle of protein-dye binding, Anal. Biochem., 1976, 7(7), 248–254 CrossRef.
- F. von der Kammer, P. L. Ferguson and P. A. Holden,
et al., Analysis of engineered nanomaterials in complex matrices (environment and biota): general considerations and conceptual case studies, Environ. Toxicol. Chem., 2012, 31(1), 32–49 CrossRef CAS PubMed.
- A. Praetorius, J. Labille and M. Scheringer,
et al., Heteroaggregation of Titanium Dioxide Nanoparticles with Model Natural Colloids under Environmentally Relevant Conditions, Environ. Sci. Technol., 2014, 48(18), 10690–10698 CrossRef CAS PubMed.
- I. Corsi, I. Venditti and F. Trotta, Environmental safety of nanotechnologies: The eco-design of manufactured nanomaterials for environmental remediation, Sci. Total Environ., 2023, 865, 161181 CrossRef PubMed.
- M. Bressan, A. Chinellato and M. Munari,
et al., Does seawater acidification affect survival, growth and shell integrity in bivalve juveniles?, Mar. Environ. Res., 2014, 99, 136–148 CrossRef CAS PubMed.
- L. Kapsenberg, A. Miglioli and M. C. Bitter,
et al., Ocean pH fluctuations affect mussel larvae at key developmental transitions, Proc. Biol. Sci., 2018, 285, 20182381 CAS.
- A. L. Maulvault, C. Camacho and V. Barbosa,
et al., Assessing the effects of seawater temperature and pH on the bioaccumulation of emerging chemical contaminants in marine bivalves, Environ. Res., 2018, 161, 236–247 CrossRef CAS PubMed.
- R. Freitas, S. Silvestro and F. Coppola,
et al., Combined effects of salinity changes and salicylic acid exposure in Mytilus galloprovincialis, Sci. Total Environ., 2020, 1(715), 136804 CrossRef PubMed.
- M. Baalousha, Effect of nanomaterial and media physicochemical properties on nanomaterial aggregation kinetics, NanoImpact, 2016, 6, 55–68 CrossRef.
- I. Corsi, A. Bellingeri and M. C. Eliso,
et al., Eco-interactions of engineered nanomaterials in the marine environment: Towards an eco-design framework, Nanomaterials, 2021, 11(8), 1903 CrossRef CAS PubMed.
- D. M. Lowe, M. N. Moore and B. M. Evans, Contaminant impact on interactions of molecular probes with lysosomes in living hepatocytes from dab Limanda limanda, Mar. Ecol.: Prog. Ser., 1992, 91(1–3), 135–140 CrossRef CAS.
- J. D. Wessler, L. T. Grip and J. Mendell,
et al., The P-glycoprotein transport system and cardiovascular drugs, J. Am. Coll. Cardiol., 2013, 61, 2495–2502 CrossRef CAS PubMed.
- T. Luckenbach, Fatal attraction: Synthetic musk fragrances compromise multixenobiotic defense systems in mussels, Mar. Environ. Res., 2004, 58(2–5), 215–219 CrossRef CAS PubMed.
- Y. Ma, K. Poole and J. Goyette,
et al., Introducing Membrane Charge and Membrane Potential to T Cell Signaling, Front. Immunol., 2017, 8, 1513 CrossRef PubMed.
- M. Henry, E. Boucaud-Camou and Y. Lefort, Functional micro-anatomy of the digestive gland of the scallop Pecten maximus (L.), Aquat. Living Resour., 1991, 4, 191–202 CrossRef.
- S. Mittal, P. K. Sharma and R. Tiwari,
et al., Impaired lysosomal activity mediated autophagic flux disruption by graphite carbon nanofibers induce apoptosis in human lung epithelial cells through oxidative stress and energetic impairment, Part. Fibre Toxicol., 2017, 14, 15 CrossRef PubMed.
- M. M. Pereira, L. Mouton and C. Yéprémian, Ecotoxicological effects of carbon nanotubes and cellulose nanofibers in Chlorella vulgaris, J. Nanobiotechnol., 2014, 12, 15 CrossRef PubMed.
- Z. Liu, M. Li and Q. Y, The Neuroendocrine-Immune Regulation in Response to Environmental Stress in Marine Bivalves, Front. Physiol., 2018, 13, 1456 CrossRef PubMed.
- A. Almeida, M. Solé and A. M. V. M. Soares,
et al., Anti-inflammatory drugs in the marine environment: Bioconcentration, metabolism and sub-lethal effects in marine bivalves, Environ. Pollut., 2020, 263, 114442 CrossRef CAS PubMed.
- M. Benedetti, M. E. Giuliani and M. Mezzelani,
et al., Emerging environmental stressors and oxidative pathways in marine organisms: Current knowledge on regulation mechanisms and functional effects, Biocell, 2022, 46(1), 37–49 CAS.
- M. A. Browne, D. Awantha and G. S. Tamara,
et al., Ingested Microscopic Plastic Translocates to the Circulatory System of the Mussel, Mytilus edulis (L.), Environ. Sci. Technol., 2008, 42(13), 5026–5031 CrossRef CAS PubMed.
- L. C. Felix, J. D. Ede and D. A. Snell, Physicochemical properties of functionalized carbon-based nanomaterials and their toxicity to fishes, Carbon, 2016, 104, 78–89 CrossRef CAS.
|
This journal is © The Royal Society of Chemistry 2024 |
Click here to see how this site uses Cookies. View our privacy policy here.