DOI:
10.1039/D4BM00043A
(Review Article)
Biomater. Sci., 2024,
12, 1346-1356
Blood–brain barrier-crossing dendrimers for glioma theranostics
Received
10th January 2024
, Accepted 12th February 2024
First published on 13th February 2024
Abstract
Glioma, as a disease of the central nervous system, is difficult to be treated due to the presence of the blood–brain barrier (BBB) that can severely hamper the efficacy of most therapeutic agents. Hence, drug delivery to glioma in an efficient, safe, and specifically targeted manner is the key to effective treatment of glioma. With the advances in nanotechnology, targeted drug delivery systems have been extensively explored to deliver chemotherapeutic agents, nucleic acids, and contrast agents. Among these nanocarriers, dendrimers have played a significant role since they possess highly branched structures, and are easy to be decorated, thus offering numerous binding sites for various drugs and ligands. Dendrimers can be designed to cross the BBB for glioma targeting, therapy or theranostics. In this review, we provide a concise overview of dendrimer-based carrier designs including dendrimer surface modification with hydroxyl termini, peptides, and transferrin etc. for glioma imaging diagnostics, chemotherapy, gene therapy, or imaging-guided therapy. Finally, the future perspectives of dendrimer-based glioma theraputics are also briefly discussed.
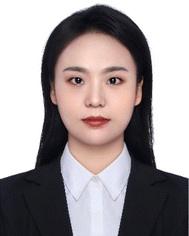 Jinxia Wang | Jinxia Wang received her B.E. degree and M.S. degree both in Chemical Engineering and Technology from Shanghai University of Engineering Science in 2020 and 2023, respectively. Currently, she is a Ph.D. candidate in the College of Biological Science and Medical Engineering, Donghua University, supervised by Prof. Mingwu Shen. Her current research project is focused on the preparation of dendrimer nanogel-based platforms for biomedical applications. |
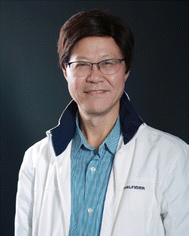 Guixiang Zhang | Guixiang Zhang, MD, Chief Physician, professor and doctoral supervisor at Shanghai Fourth People's Hospital, School of Medicine, Tongji University, obtained his PhD in clinical medical imaging in 1993, received a diploma in foreign attending physician from the School of Medicine at the University of Lyon in France in 1993 and worked as an employed physician at the Department of Radiology of Nancy Neurological Hospital in France in 1995. Dr Zhang has rich experience in clinical medical imaging diagnosis, and has published over 100 SCI-indexed peer-reviewed journal articles. |
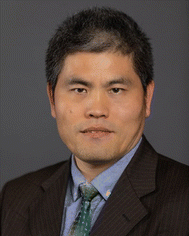 Xiangyang Shi | Xiangyang Shi obtained his PhD degree in 1998 from the Chinese Academy of Sciences. From 2002 to 2008, he was appointed as a research fellow, research associate II, research investigator, and research assistant professor in the Medical School of the University of Michigan, Ann Arbor. In September 2008, he joined Donghua University as a full professor. He has published 503 peer-reviewed SCI-indexed journal articles with an h-index of 90. His current research interests are focused on the development of organic/inorganic hybrid nanoplatforms and dendrimeric nanoparticles for sensing, imaging, and theranostic applications, in particular for precision theranostics of cancer and inflammatory diseases. |
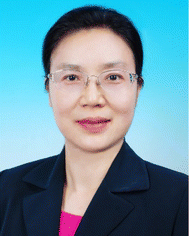 Mingwu Shen | Mingwu Shen received her PhD degree in 2001 from Tsinghua University. Afterwards, she went to the University of Michigan, Ann Arbor, as a visiting scholar and a research area specialist intermediate. She joined Donghua University as an associate professor in 2008, and since 2017, she has been appointed as a full professor of Biomedical Engineering at Donghua University. She has published more than 210 SCI-indexed peer-reviewed journal articles with an h-index of 60. Prof. Shen's current research interests include organic/inorganic hybrid nanoparticle-based platforms for medical imaging and therapy applications. |
Introduction
As a primary brain tumor, glioma is difficult to treat and characterized by high aggressiveness, easy recurrence, and high mortality, seriously endangering the life and health of human beings.1,2 Traditional surgical treatment, chemotherapy, and radiotherapy cannot completely eradicate glioma and drug toxicity and may harm surrounding normal brain tissues.3,4 The combination therapy has become a primary clinical treatment approach. However, the blood–brain barrier (BBB), comprising of brain endothelial cells, astrocytes, pericytes, and basement membranes, exhibits exceptional selective permeability to maintain cerebral homeostasis.5 This barrier strictly regulates the passage of substances, making it challenging for most drugs to effectively accumulate at the tumor site and significantly limits the efficiency of drug delivery. Therefore, the development of drug delivery systems capable of crossing the BBB is of paramount importance for enhanced glioma therapy.
The recent progress in nanotechnology and nanomedicine holds great promise for the efficient treatment of glioma.6,7 A range of nanoscale drug delivery systems including but not limited to inorganic nanomaterials,8 dendrimers,9 polymer nanoparticles (NPs),10 liposomes,11 micelles,12 and nanogels13,14 have been developed. These carriers can be used to encapsulate various kinds of therapeutic molecules and contrast agents such as small molecular drugs, proteins, peptides, genes, gold (Au) NPs, and so on, through physical or chemical interactions.15 The accumulation of drugs at tumor sites can be enhanced by targeting ligand surface modification, and the intelligent nanoplatforms also enable responsive release of drugs specifically at the lesion site, offering significant advantages in glioma treatment. Among these nanocarriers, dendrimers with highly branched three-dimensional structures possess monodispersity, easy surface functionalization ability, good biocompatibility, and low immunogenicity, thus serving as excellent carriers for therapeutic drugs and imaging agents.16,17 In recent years, dendrimer-based delivery systems have been widely used for glioma treatment.18 Dendrimers including poly(amidoamine) (PAMAM) dendrimers,19 polyester dendrimers20 and amphiphilic dendrimers21 can be surface modified to cross the BBB since the multiple terminal units of dendrimers can be easily conjugated with various bioactive substances. Some proteins/cytokines are specifically expressed on the BBB or glioma, such as neuropilin-1 (NRP-1) receptor, low-density lipoprotein receptor (LRP), transferrin receptor (TfR) or others. Hence, modifications of the suitable targeting agents on the surface of dendrimers can facilitate their effective penetration of the BBB, enhance glioma targeting, and reduce cytotoxicity to normal tissue for the designed nanomedicines.
In this review, we discuss the recent advances in the design of BBB-crossing dendrimer-based nanocarrier design, including dendrimer surface modification with hydroxyl groups, peptides, transferrin (Tf), and others. The effective treatments of glioma are summarized as follows: diagnostic imaging, chemotherapy, gene therapy, and imaging-guided therapy (Fig. 1). Some representative modification methods and their therapeutic and diagnostic applications are summarized in Table 1. This review begins with a short introduction, discusses the recent advances in the dendrimer-based BBB-crossing nanocarrier design for different therapeutic and diagnostic applications of glioma, and lastly briefly addresses the challenges and perspectives of dendrimer nanocarriers for glioma treatment. It should be noted that this is not a comprehensive review, but rather focuses on the recent key developments in the area of dendrimer-based nanomedicines for glioma theranostics.
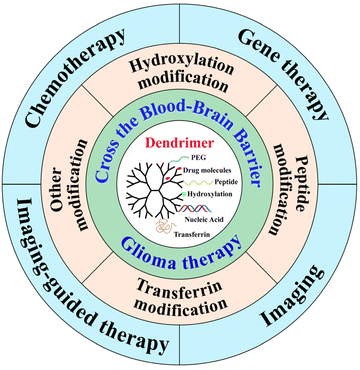 |
| Fig. 1 Schematic illustration of functionalized dendrimers to cross the BBB for glioma imaging, therapy or theranostics. | |
Table 1 Summary of various functionalized dendrimers for diagnostics and therapeutics of glioma
Modification |
Dendrimers |
Targeting strategies |
Glioma targets |
Diagnostics and therapeutics |
Ref. |
Hydroxylation |
G4 PAMAM |
G4-OH |
TAMs |
Crossing BBB |
27
|
G6 PAMAM |
G6-OH |
TAMs |
Crossing BBB |
25
|
G4 PAMAM |
G4-OH |
TAMs |
Rapa delivery for immunotherapy |
28
|
G6 PAMAM |
G6-OH |
TAMs |
siRNA delivery for gene therapy |
47
|
|
Peptide |
G2 PAMAM |
RGD |
αvβ3 integrin for BBB crossing and glioma |
MR/CT imaging |
48
|
G4 PAMAM |
PEG and iRGD (CRGDKGPDC) |
αvβ3 integrin and NRP-1 for BBB crossing and glioma |
DOX delivery for chemotherapy |
34
|
G5 PAMAM |
PEG and Cys-Arg-Glu-Lys-Ala peptide |
Fibrin for glioma |
DOX delivery for chemotherapy |
49
|
G5 PAMAM |
PEG and Pep-1 |
Interleukin 13 receptor α2 for glioma |
Glioma targeting |
50
|
G3 and G5 PAMAM |
PEG and RGD |
αvβ3 integrin for BBB and glioma |
MR imaging-guided chemodynamic therapy |
51
|
|
Tf |
G4 PAMAM |
Tf and tamoxifen |
TfR and the drug efflux proteins for BBB and glioma cells |
DOX delivery for chemotherapy |
40
|
G4 PAMAM |
Tf and WGA |
TfR and N-acetylglucosamine for BBB and glioma cells |
DOX delivery for chemotherapy |
39
|
|
Others |
G4 PAMAM |
G4-OH and α-D-glucose, α-D-mannose, or α-D-galactose |
BBB and sugar transporters on glioma cells |
BBB crossing and glioma targeting |
43
|
G4 PAMAM |
Angiopep-2 and EP-1 |
LRP1 for BBB and EGFR for glioma cells |
DOX delivery for chemotherapy |
37
|
G3 PAMAM |
chlorotoxin |
Membrane-bound matrix metalloproteinase-2 endopeptidase for glioma cells |
pORF-TRAIL plasmid DNA delivery for gene therapy |
52
|
G3 PAMAM |
PMPC |
Acetylcholine transporters for BBB |
BBB crossing for DOX-mediated chemotherapy |
44
|
|
|
G5 PAMAM |
— |
— |
MR/fluorescence imaging |
53
|
Design of BBB-crossing dendrimer carriers for brain glioma targeting
The presence of the BBB brings a great challenge for effective drug delivery to glioma. As is known, the intravenously administered dendrimers with a size less than 12 nm are able to cross the BBB to reach malignant glioma.22 Dendrimers have been often pre-modified or post-modified with polyethylene glycol (PEG) to reduce their toxicity and prolong their blood circulation time by neutralizing their surface positive charges.23,24 For effective BBB crossing, dendrimers have been surface functionalized with various functional groups or ligands (Table 1). In this section, we detail the dendrimer surface modification strategies for BBB crossing and glioma targeting in recent years.
Hydroxylation modification of dendrimers
Recent advances in nanomedicine based on dendrimers have revealed that terminal hydroxylated PAMAM dendrimers can cross the BBB and target brain tumors without additional modification of targeting ligands.25,26 A study conducted by Zhang et al. demonstrated that hydroxylated generation 4 (G4) PAMAM dendrimers (G4-OH) possess the capability to cross the damaged BBB and accumulate in tumor-associated macrophages (TAMs).27 The excellent physicochemical properties of G4-OH PAMAM dendrimers, such as nearly neutral surface charge (ζ-potential = 4.5 ± 0.1 mV) and small size (∼4 nm), allow them to cross the BBB. The research team investigated the pharmacokinetics and biodistribution of dendrimers using Fischer 344 rats to establish an intracranial brain tumor model, and showed the uniform distribution of dendrimers in the entire solid tumor (∼6 mm) and the peritumor region. Despite their ability to penetrate the BBB and accumulate in glioma, they can also be cleared through the kidneys within 24 h due to their much smaller size than the renal filter.22 Liaw et al. further demonstrated that G6-OH PAMAM dendrimers could also have significantly promoted tumor accumulation, tumor specificity, and tumor retention compared to G4-OH PAMAM dendrimers (Fig. 2A and B).25 Importantly, the increase in dendrimer generations did not seem to affect their uniform tumor distribution and intrinsic targeting.
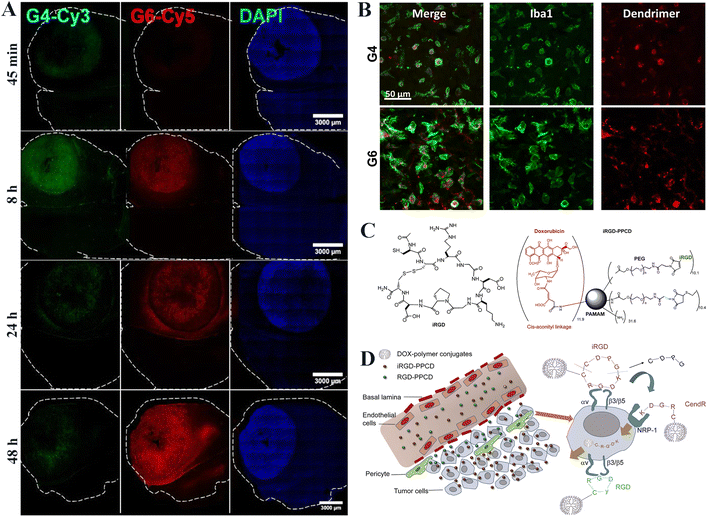 |
| Fig. 2 (A) Fluorescence imaging of G4 (green) and G6 (red) dendrimer distributions in the tumor-bearing brain at different time points. The tumor mass is indicated by nuclear DAPI staining (blue). (B) Both G4 and G6 dendrimers are localized specifically within TAMs (green) at 24 h post intravenous administration. Scale bar = 50 μm for each panel. Reproduced with permission from ref. 25 Copyright 2020, John Wiley and Sons. (C) The structure of iRGD and iRGD–PPCD conjugates. (D) Illustration of in vivo delivery and penetrating behavior of iRGD–PPCD and RGD–PPCD. Reproduced with permission from ref. 34 Copyright 2014, Elsevier. | |
Moreover, Sharma et al. utilized a G4-OH dendrimer–rapamycin conjugate (D-Rapa) to specifically target TAMs in glioma after systemic administration to improve the treatment efficacy.28 It was shown that D-Rapa could suppress the mammalian target of the Rapa (mTOR) pathway of activated TAMs and improve both the reprogramming of TAMs toward an antitumor phenotype and antiproliferative efficacy in glioma cells in vitro, thus improving the systemic treatment of glioma in vivo. In another work, Liaw et al. presented a G4-OH dendrimer conjugated with triptolide for TAM-specific delivery and pH-responsive release of triptolide.29 The treatment in vitro exhibited signal transducer and activator of transcription 3 (STAT3) inhibition and anti-tumor immune signalling upregulation. Systemic dendrimer delivery of triptolide significantly improved the antitumor efficacy when compared to the administered free triptolide in vivo. These results demonstrate that hydroxylated dendrimers can serve as a promising BBB-crossing nanodrug carrier for glioma treatment.
Peptide modification of dendrimers
Peptide-mediated drug delivery is also an effective BBB crossing and glioma targeting strategy.30 Some peptide molecules exhibit remarkable affinity and specificity for receptors expressed on the BBB or glioma, such as NRP-1 receptor, LRP or others.31–33 The peptide modification can ensure the specific delivery of therapeutic cargoes to glioma site through receptor-mediated endocytosis. For instance, the Arg-Gly-Asp (RGD) peptide has been identified to have high affinity with integrin αvβ3, and the internalizing RGD (iRGD) can combine with both integrins αvβ3 and NRP-1, respectively overexpressed on endothelial cells and tumor cells. As shown in Fig. 2C and D, Wang et al.34 prepared an iRGD-PEG-PAMAM-cis-aconityl-doxorubicin (DOX) (for short, iRGD–PPCD) conjugate by covalently linking PEGylated and iRGD-modified G4 PAMAM dendrimer with cis-aconityl-DOX similar to the protocols used to synthesize RGD–PPCD conjugate. Glioma penetration studies have shown that the iRGD–PPCD possessed much better BBB penetration and glioma targeting properties than the RGD–PPCD delivery system, although both are able to cross the BBB. In vivo studies also confirmed the significantly enhanced intratumoral drug accumulation and improved therapeutic efficacy of gliomas after administration of iRGD–PPCD.
The low-density lipoprotein receptor-associated protein (LRP) is overexpressed at the BBB and on the surface of glioma cells, and can readily bind to Angiopep-2 ligand with a high affinity. Hence, as a targeting peptide for LRP, the modification of angiopep-2 renders nanomedicines with high BBB penetration ability in vitro and in vivo.35 In addition, epidermal growth factor receptor (EGFR) is also known to have overexpression on the surface of glioma cells.36 Based on this, Liu et al.37 concurrently conjugated both Angiopep-2 and EGFR-targeting peptide (EP-1) onto the surface of G4 PAMAM dendrimers and loaded DOX within the dendrimer internal cavity. In vitro and in vivo experiments showed that the dual-targeting modification of dendrimers significantly improved the BBB permeability and glioma targeting effect of the nanocarriers and the glioma therapeutic efficacy of DOX.
Transferrin modification of dendrimers
Besides the dendrimer surface hydroxylation and peptide modification, transferrin (Tf), a protein with an Mw of 76 kDa is also recognized to target transferrin receptor (TfR) overexpressing in brain microvascular endothelial cells and glioma cells, and has been modified for brain-targeted drug delivery systems.38
In a recent study, He et al.39 prepared a drug delivery carrier for glioma therapy based on Tf- and wheat germ agglutinin (WGA)-modified G4 PAMAM dendrimers (Fig. 3A). Similar to Tf, the attached WGA also shows a strong affinity to cerebral capillary endothelium and malignant glioma cells due to the N-acetylglucosamine receptor on the cell surface. In vitro BBB transport experiment showed that the dual targeting modification of dendrimers led to much greater BBB penetration and glioma targeting of the PAMAM-PEG-WGA-Tf-DOX NPs than that of the single targeting-modified counterpart NPs of PAMAM-PEG-WGA-DOX or PAMAM-PEG-Tf-DOX (Fig. 3B). The growth of the avascular C6 glioma spheroids was efficiently inhibited due to the effective DOX accumulation (Fig. 3C). In another study, Li et al.40 prepared a pH-sensitive Tf and tamoxifen dual-targeted drug carrier (G4-DOX-PEG-Tf-tamoxifen) to enhance the BBB transport capacity and drug accumulation within glioma cells. The modified tamoxifen can inhibit the drug efflux proteins on both BBB and glioma cells, facilitating drug accumulation in tumor cells. In the non-vascular C6 glioma spheroid model assay, the developed G4-DOX-PEG-Tf-tamoxifen could induce C6 glioma cell death, leading to a substantial reduction in the spheroid volume. However, this study lacked animal studies to prove that the dual-targeting ligand modification can promote BBB crossing and glioma targeting in an orthotopic glioma model.
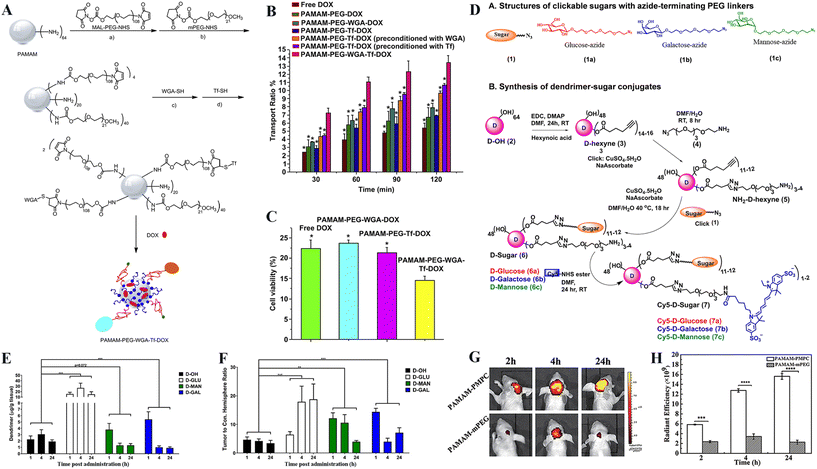 |
| Fig. 3 (A) The synthetic route of dual-targeting drug carrier of PAMAM-PEG-WGA-Tf-DOX. (B) The transport ratio of DOX across the in vitro BBB model during 30–120 min of incubation with different nanomedicines. (C) The viability of the C6 cells after drug transporting across the BBB. Data were presented as the mean ± standard deviation (n = 4). *p < 0.001, as compared with the PAMAM-PEG-WGA-Tf-DOX. Reproduced with permission from ref. 39 Copyright 2010, Elsevier. (D) Structural representation of clickable sugars (glucose-azide, galactose-azide and mannose-azide with PEG linkers) and synthetic protocol for dendrimer–sugar conjugates. The synthesis of fluorescently labeled Cy5-D-glucose, Cy5-D-mannose, and Cy5-D-galactose is presented using CuAAC click chemistry approach. (E) Quantification of tumor accumulation by sugar–dendrimer conjugates after glioma-bearing mice were injected with glucose-, mannose-, or galactose-conjugated dendrimers (D-GLU, D-MAN or D-GAL). *** p < 0.001. (F) Sugar-conjugated dendrimers exhibit significantly greater glioma specificity in the contralateral hemisphere than the unmodified G4-OH dendrimers. ** p < 0.01 and *** p < 0.001. Reproduced with permission from ref. 43 Copyright 2021, Elsevier. (G) In vivo noninvasive near-infrared time-dependent whole-body fluorescence imaging of brain glioma tumor-bearing nude mice and (H) corresponding quantitative fluorescence intensity analysis after intravenous injection of Cy5.5-labeled PAMAM-PMPC or PAMAM-mPEG NPs for 2, 4, and 24 h, respectively. Reproduced with permission from ref. 44 Copyright 2021, Wiley-VCH. | |
Other modifications of dendrimers
In addition to those above dendrimer surface modifications, there are also other modifications to render dendrimer-based nanomedicines with the ability of BBB crossing and glioma targeting. Glycosylation has garnered significant attention in the advancement of targeted drug delivery systems owing to the sugar-mediated targeting specificity.41,42 For instance, Sharma et al.43 conjugated sugar molecules to hydroxyl-terminated PAMAM dendrimers for glioma targeting (Fig. 3D). The commercial G4-OH PAMAM dendrimers were partially modified with α-D-glucose, α-D-mannose, or α-D-galactose, respectively to target TAMs with upregulated glucose transporters and mannose receptors (CD206), or glioma cells overexpressing galectins. At 24 h post intravenous injection of unmodified or sugar-modified G4-OH dendrimers, the G4-OH dendrimers partially modified with α-D-glucose exhibited ∼8-fold higher tumor accumulation than the G4-OH dendrimers without glycosylation as revealed by fluorescence imaging. The G4-OH dendrimers partially modified with α-D-mannose or α-D-galactose exhibited respective ∼2- and ∼2.5-fold greater tumor accumulation at 1 h postinjection than the sugar-free G4-OH dendrimers, but were cleared faster from the tumor and exhibited ∼50% lower tumor accumulation at 24 h postinjection than the G4-OH dendrimers (Fig. 3E). All sugar-modified dendrimers had significantly improved specificity to the orthotopic glioma (Fig. 3F), and the α-D-glucose modification was the most significant one among all groups.
Besides, it has been reported that acetylcholine transporters and choline transporters are highly expressed in luminal brain capillary endothelial cells,45 hence can be developed for brain targeting for nanomedicines. In a recent work, zwitterionic poly(2-methacryloyloxyethyl phosphorylcholine) (PMPC) having a similar structure to acetylcholine, has been modified onto the surface of NPs to render them with BBB permeability and brain tumor targeting specificity.46 Similarly, G3 PAMAM dendrimers can also be modified with PMPC to not only significantly reduce the cytotoxicity of dendrimers, but also efficiently cross BBB and target the brain glioma (Fig. 3G and H). There is a much stronger fluorescence signal of Cy5.5-labelled dendrimers still present in the brain tumor area even at 24 h postinjection.44
Diagnostics and therapeutics of glioma using BBB-crossing dendrimers
Based on the above strategies used to prepare BBB-crossing dendrimers, different nanomedicine formulations have been developed for glioma imaging, therapy or theranostics. In this section, we review some key developments of dendrimer-based nanomedicines in imaging diagnostics, chemotherapy, gene therapy, or imaging-guided therapy of brain glioma, although some of the application aspects have already been illustrated in the last section.
Imaging
Precise imaging can greatly increase the success rate of glioma treatment. To achieve high sensitivity, high specificity, and deep penetration into the brain tissue, various non-invasive imaging modalities such as ultrasonography, computed tomography (CT), and magnetic resonance (MR) imaging are currently utilized to diagnose glioma. Nevertheless, conventional contrast agents have many problems such as short retention time, renal toxicity, and lack of specificity.9 Functionalized dendrimers can be applied as targeted nanocarriers of imaging contrast agents to facilitate precision glioma diagnosis.54,55 For example, Karki et al.53 prepared an MR and fluorescence imaging agent utilizing G5 PAMAM dendrimers to load clinically relevant Gd-DOTA and the near-infrared fluorescence dye DL680. In vitro and in vivo MR/fluorescence imaging results revealed that the contrast agent showed much more accumulation in orthotopic U251 glioma through the passive enhanced permeability and retention effect and excreted through liver possibly as a result of small particle size, relatively neutral surface charge and hydrophilicity in the blood. Apparently, due to the lack of the associated surface modifications for BBB crossing and glioma targeting, the imaging sensitivity and specificity are quite low. In another study, Xu et al.48 developed a multifunctional low-generation dendrimer nanoprobe (G2-RGD-Au-Mn) for targeted dual-mode MR/computed tomography (CT) imaging of orthotopic glioma. They first modified G2 PAMAM dendrimers with 1,4,7-triacyclononane-1,4,7-triacetic acid (NOTA) and the targeting ligand of cyclic RGD peptide via a PEG spacer. Au NPs were then synthesized using the functionalized G2 dendrimers as templates, followed by chelation with Mn(II). The G2-RGD-Au-Mn nanoprobe exhibited good colloidal stability, higher r1 relaxivity (9.88 mM−1 s−1) than the commercial Gd-based Magnevist® (4.54 mM−1 s−1), and greater X-ray attenuation intensity than iodine-based CT contrast agents such as Omnipaque (Fig. 4A–C). Through the RGD surface modification, the nanoprobe can cross BBB and target glioma for precise MR/CT dual-mode imaging (Fig. 4D–G).
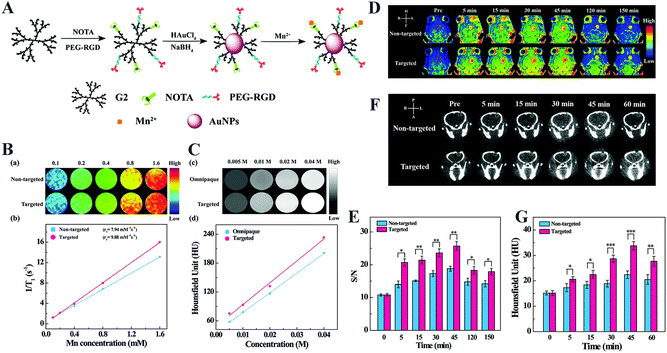 |
| Fig. 4 (A) Synthesis of RGD-Au-Mn DENPs. (B) The T1-weighted MR images of the RGD-free Au-Mn and RGD-Au-Mn DENPs at different Mn concentrations (0.1, 0.2, 0.4, 0.8, and 1.6 mM, respectively) and linearly fitted 1/T1 of the corresponding particles versus Mn concentration. (C) The CT images and the quantitative CT values of the RGD-Au-Mn DENPs and Omnipaque at the Au or I concentration of 0.005, 0.01, 0.02 and 0.04 M, respectively. (D) The T1-weighted MR images and (E) the MR signal-to-noise (S/N) ratio of the C6 orthotopic glioma tumor (the red circle indicates the tumor region), and (F) the CT images and (G) quantitative CT values of the C6 orthotopic glioma (the green circle denotes the tumor area) before and after the non-targeted Au–Mn or targeted RGD-Au-Mn DENPs were intravenously injected (Mn mass = 400 mg, [Au] = 0.05 M, in 150 mL PBS for each mouse). The star symbols are denoted as follows: (*) for p < 0.05, (**) for p < 0.01, and (***) for p < 0.001, respectively. Reproduced with permission from ref. 48 Copyright 2019, Royal Society of Chemistry. | |
Chemotherapy
Some small molecular chemotherapeutic drugs such as DOX,56 arsenic trioxide (ATO),32 and temozolomide,57 possess low bioavailability and high side effects and are difficult to cross the BBB. To overcome these limitations, BBB-crossing dendrimers have been utilized as drug carriers to load drugs through physical adsorption or covalent surface modification for specific glioma targeting, thereby significantly enhancing drug bioavailability and mitigating the non-desired systemic side effects. For instance, Xu et al.58 constructed a glutathione (GSH)-sensitive G4-DOX-Angiopep-PEG nanoplatform, which exhibited a better in vitro BBB penetration ability than the Angiopep-free G4-DOX-PEG counterpart, resulting in a significant reduction of avascular C6 glioma spheroid volume in vitro. However, the effective chemotherapy effect of the G4-DOX-Angiopep-PEG nanoplatform has not been proofed using an in vitro orthotopic glioma animal model. In another study, Shi et al.59 established a dual-targeting drug delivery system for ATO delivery by modifying the G5 PAMAM dendrimers with a 12-amino-acid TGN peptide (TGNYKALHPHNG) and iRGD (Fig. 5A). The attached TGN peptide is known to have an efficient cerebral transport ability.59 ATO was complexed with the dendrimers by electrostatic interaction and the ATO encapsulation efficiency of this dual-targeting PAMAM dendrimers reached approximately 71.92%. The loaded drug could be fast released under a slightly acidic pH. Combined with attached iRGD ligand, the G5 PAMAM dendrimers are able to cross the BBB and target glioma to facilitate ATO delivery in glioma. In vitro experiments revealed a more significantly improved survival rate in the iRGD/TGN co-modified group than in the other groups (Fig. 5B and C).
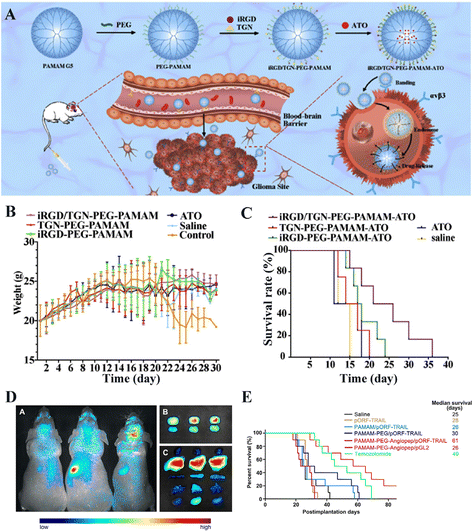 |
| Fig. 5 (A) Illustration of TGN/iRGD-comodified G5 PAMAM dendrimers for drug delivery. (B) Body weight of U87 tumor-bearing mice (n = 6). (C) Kaplan–Meier survival curves for each group (n = 6). Reproduced with permission from ref. 59 Copyright 2020, Elsevier. (D) In vivo and in vitro imaging (brain, glioma and five viscera) of mice administrated with PAMAM/pORF-TRAIL (left), PAMAM-PEG/pORF-TRAIL (middle), or PAMAM-PEG-Angiopep/pORF-TRAIL (right). Images were taken at 120 min post administration. (E) Survival curve of glioma-bearing mice in different groups. Reproduced with permission from ref. 60 Copyright 2011, Elsevier. | |
Gene therapy
As an emerging therapeutic approach, gene therapy holds promise in selectively targeting cancer cells with minimal side effects.54 However, the presence of BBB hinders gene delivery to glioma efficiently. In addition to chemotherapeutic drugs, dendrimers can be loaded with nucleic acids for gene therapy of brain tumors.55 For example, the tumor necrosis factor-related apoptosis inducing ligand (TRAIL) is often used in gene therapy to induce apoptosis in cancer cells. In a recent study, Huang et al.60 conducted a gene delivery system by utilizing G5 PAMAM dendrimers modified with PEG and the targeting ligand Angiopep-2 as carriers, and then complexed pORF-TRAIL, which is a plasmid DNA expressing TRAIL (PAMAM-PEG-Angiopep/pORF-TRAIL). In vitro transfection experiment revealed that the PAMAM-PEG-Angiopep/pORF-TRAIL polyplexes could be more significantly taken up by C6 cells than the free pORF-TRAIL. Further analysis of the glioma sections through terminal deoxynucleotidyl transferase dUTP nick end labeling (TUNEL) staining revealed that the PAMAM-PEG-Angiopep/pORF-TRAIL led to the most significant tumor cell apoptosis. The biodistribution in vivo showed that the uptake of PAMAM-PEG-Angiopep/pORF-TRAIL polyplexes in the glioma site was obvious (Fig. 5D). As shown in Fig. 5E, in vitro experiments revealed that glioma-bearing mice treated with the PAMAM-PEG-Angiopep/pORF-TRAIL polyplexes had a more significantly prolonged survival time than other groups.
Small interfering RNAs (siRNAs) as potent tools for gene silencing have many applications in central nervous system diseases by directly inhibiting disease-causing gene expression with high targeting specificity and low effective doses. To realize the effective delivery of siRNA, Liyanage et al.47 covalently conjugated green fluorescent protein siRNA (siGFP) to G6-OH dendrimers via a GSH-sensitive linker to form G6-OH dendrimer–siRNA conjugates. The conjugates allowed precise siRNA loading, protected siRNA from premature degradation and efficiently delivered siRNA into glioma cells both in vitro and in vivo. When administered intratumorally to an orthotopic glioma mouse model, the conjugates could cross the BBB and be localized in the TAMs to effectively silence GFP expression.
Imaging-guided therapy
For precision cancer theranostics, it is vital to develop a dendrimer-based nanomedicine platform to cross BBB for simultaneous glioma imaging and therapy. In a recent example,51 our group developed a multifunctional acetylated core–shell tecto dendrimer (CSTD) platform (M-CSTD·NHAc) based on the supramolecular self-assembly of β-cyclodextrin (CD)-modified G5 PAMAM dendrimers as cores and adamantane (Ad)-functionalized G3 PAMAM dendrimers (G3·NH2-Ad) as shells, where the G3·NH2-Ad dendrimers acted as standalone modules to be modified with pyridine for copper (Cu(II)) complexation, dermorphin for BBB crossing and RGD peptide for glioma targeting, respectively (Fig. 6A) for orthotopic glioma MR imaging and chemodynamic therapy (CDT). In vitro experiments utilizing 3D C6 multicellular tumor spheroids (MCTS) revealed that the M-CSTD·NHAc-FITC had a better penetration ability than the single-generation M-G5·NHAc-FITC (Fig. 6B–D). Furthermore, the complexes could effectively deplete GSH within glioma cells, initiating a Cu(II)-mediated Fenton-like reaction to lead to increased levels of toxic reactive oxygen species and cellular lipid peroxidation, hence significantly inhibiting the growth of MCTS during the seven-day treatment period (Fig. 6E and F). The T1-weighted MR phantom studies showed that M-CSTD·NHAc/Cu(II) complexes had an r1 relaxivity of 0.7331 mM−1 s−1 due to the presence of Cu(II) (Fig. 6G), thus enabling effective T1-weighted MR imaging of an orthotopic glioma mouse model. The glioma MR signal intensity increased with the time post-injection and reached the peak at 45 min, demonstrating the ability of the M-CSTD·NHAc/Cu(II) complexes to cross the BBB for glioma targeting (Fig. 6H). After treatment, the orthotopic glioma growth was inhibited, and the survival rate of glioma-bearing mice in the M-CSTD·NHAc/Cu(II) group substantially increased (Fig. 6I).
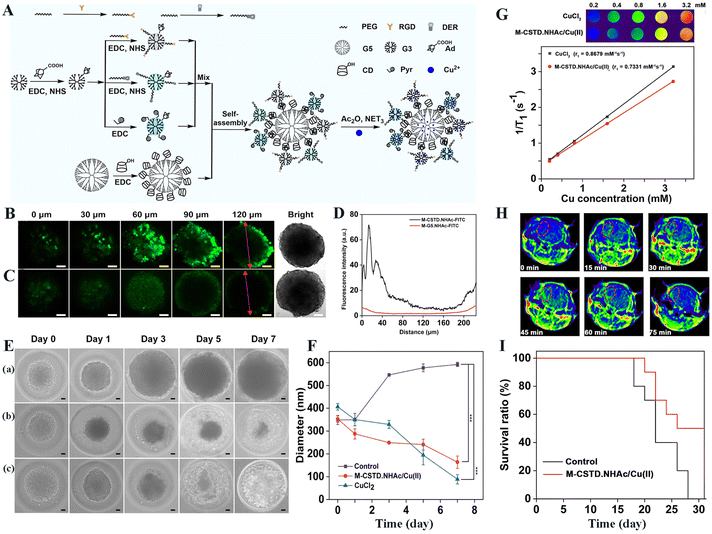 |
| Fig. 6 (A) Schematic illustration of the modular design of multifunctional M-CSTD·NHAc/Cu(II) complexes. CLSM images of 3D C6 MCTS incubated with M-CSTD·NHAc-FITC (B) or M-G5·NHAc-FITC (C) for 6 h ([G5] = 5 μM for both materials). (D)The fluorescence intensity profile of 3D C6 MCTS (red line with two arrows in (B and C). (E) Representative optical micrographs of C6 MCTS incubated with (a) normal saline (NS), (b) M-CSTD·NHAc/Cu(II) complexes and (c) CuCl2, respectively. (F) The MCTS diameter changes in each group at various time points (*** for p < 0.001, n = 3). The scale bar in each panel for B, C and E, F represents 50 μm. (G) T1-weighted MR pseudocolor images and linear fitting of 1/T1 of the M-CSTD·NHAc/Cu(II) complexes or CuCl2 at different Cu concentrations. (H) T1-weighted MR imaging of glioma at different time points post intravenous injection of the complexes. (I) The survival rate of mice treated with the complexes or NS as a control ([Cu] = 8 mM, in 100 μL NS for each mouse, n = 10). Reproduced with permission from ref. 51 Copyright 2021, Elsevier. | |
Conclusions and outlook
In summary, this review surveys the recent advances in the design of BBB-crossing dendrimers for glioma targeting, imaging, therapy or theranostics. For effective BBB crossing and glioma targeting, dendrimers can be surface modified with hydroxyl groups, peptides, transferrin, and other ligands. Meanwhile, due to the unique structural features, dendrimers can be effectively modified or complexed with anticancer drugs, therapeutic genes, or imaging agents to provide a wide variety of feasibilities for glioma imaging diagnostics, chemotherapy, gene therapy, or imaging-guided therapy.
Although there are many advantages of PAMAM dendrimer carriers in glioma therapy, their design and biomedical applications still remain challenging. For example, due to the limited internal cavity, the loading capacity of single dendrimers is quite limited. It is desired to design dendrimer carrier systems with a clustered or nanogel structure to significantly improve the payload of the therapeutic or imaging agents, thus enhancing the efficacy of glioma imaging or therapy. In addition, the BBB crossing and glioma targeting strategy may be further expanded beyond the existing dendrimer surface modifications. The dendrimer carriers could be camouflaged with cell membranes or coated with exosomes to render them with the same BBB crossing and glioma targeting purposes. Lastly, to reduce the recurrence of glioma, more therapeutic modes such as immunotherapy or immunotherapy-integrated combination therapy should be developed based on the versatile dendrimer nanotechnology. In general, the strategic design of nanocarriers utilizing dendrimers offers a highly specific and targeted therapeutic approach for efficient glioma treatment, having a broad application prospect.
Author contributions
Jinxia Wang: formal analysis, investigation, writing – original draft. Zhiqiang Wang: writing – review & editing. Guixiang Zhang: formal analysis, resources, funding acquisition. João Rodrigues: formal analysis, resources. Helena Tomás: formal analysis, resources. Xiangyang Shi: conceptualization, resources, funding acquisition, project administration, supervision, writing – review & editing. Mingwu Shen: formal analysis, resources, funding acquisition, project administration, supervision, writing – review & editing.
Conflicts of interest
The authors declare no competing financial interest.
Acknowledgements
This research has been financially supported by the National Natural Science Foundation of China (52350710203 and U23A2096), the National Key R&D Program (2022YFE0196900), the Science and Technology Commission of Shanghai Municipality (23520712500, 21490711500, 23WZ2503300 and 20DZ2254900), and the Shanghai Education Commission through the Leading Talent Program. This work was supported by the Fundação para a Ciência e a Tecnologia (FCT) with Portuguese Government funds through the CQM Base Fund - UIDB/00674/2020 (DOI: 10.54499/UIDB/00674/2020) and Programmatic Fund - UIDP/00674/2020 (DOI: 10.54499/UIDP/00674/2020).
References
- S. Padmakumar and M. M. Amiji, Adv. Drug Delivery Rev., 2023, 197, 114853 CrossRef CAS PubMed
.
- W. Wu, J. L. Klockow, M. Zhang, F. Lafortune, E. Chang, L. Jin, Y. Wu and H. E. Daldrup-Link, Pharmacol. Res., 2021, 171, 105780 CrossRef CAS PubMed
.
- Q. Qiu, X. Ding, J. Chen, S. Chen and J. Wang, J. Controlled Release, 2023, 358, 681–705 CrossRef CAS PubMed
.
- B. Sesé, M. Ensenyat-Mendez, S. Iñiguez, P. Llinàs-Arias and D. M. Marzese, Clin. Epigenet., 2021, 13, 150 CrossRef PubMed
.
- T. Xiao, M. He, F. Xu, Y. Fan, B. Jia, M. Shen, H. Wang and X. Shi, ACS Nano, 2021, 15, 20377–20390 CrossRef CAS PubMed
.
- L. Qiao, H. Yang, X. Shao, Q. Yin, X. Fu and Q. Wei, Mol. Pharmaceutics, 2022, 19, 1927–1951 CrossRef CAS PubMed
.
- L. Shabani, M. Abbasi, M. Amini, A. M. Amani and A. Vaez, J. Neurol. Sci., 2022, 440, 120316 CrossRef PubMed
.
- D. Hu, M. Xia, L. Wu, H. Liu, Z. Chen, H. Xu, C. He, J. Wen and X. Xu, Mater. Today Bio, 2023, 20, 100673 CrossRef CAS PubMed
.
- H. Gaitsch, A. M. Hersh, S. Alomari and B. M. Tyler, Cancers, 2023, 15, 1075 CrossRef CAS PubMed
.
- B. Rabha, K. K. Bharadwaj, S. Pati, B. K. Choudhury, T. Sarkar, Z. A. Kari, H. A. Edinur, D. Baishya and L. I. Atanase, Polymers, 2021, 13, 4114 CrossRef CAS PubMed
.
- H. Zhang, C. Shi, F. Han, M. Li, H. Ma, R. Sui, S. Long, W. Sun, J. Du, J. Fan, H. Piao and X. Peng, Biomaterials, 2022, 289, 121770 CrossRef CAS PubMed
.
- Y. Bi, L. Liu, Y. Lu, T. Sun, C. Shen, X. Chen, Q. Chen, S. An, X. He, C. Ruan, Y. Wu, Y. Zhang, Q. Guo, Z. Zheng, Y. Liu, M. Lou, S. Zhao and C. Jiang, ACS Appl. Mater. Interfaces, 2016, 8, 27465–27473 CrossRef CAS PubMed
.
- S. L. Mekuria, Z. Ouyang, C. Song, J. Rodrigues, M. Shen and X. Shi, Bioconjugate Chem., 2021, 33, 87–96 CrossRef PubMed
.
- M. He, T. Xiao, Y. Wang, H. Yu, Z. Wang, X. Shi and H. Wang, Chem. Eng. J., 2023, 453, 139634 CrossRef CAS
.
- Y. H. Tsou, X. Q. Zhang, H. Zhu, S. Syed and X. Xu, Small, 2017, 13, 1701921 CrossRef PubMed
.
- C. Song, M. Shen, J. Rodrigues, S. Mignani, J.-P. Majoral and X. Shi, Coord. Chem. Rev., 2020, 421, 213463 CrossRef CAS
.
- Z. Xiong, M. Shen and X. Shi, Sci. China Mater., 2018, 61, 1387–1403 CrossRef CAS
.
- M. Fana, J. Gallien, B. Srinageshwar, G. L. Dunbar and J. Rossignol, Int. J. Nanomed., 2020, 15, 2789–2808 CrossRef CAS PubMed
.
- X. Li, W. Ta, R. Hua, J. Song and W. Lu, Biomedicines, 2022, 10, 2455 CrossRef CAS PubMed
.
- P. Stenström, D. Manzanares, Y. Zhang, V. Ceña and M. Malkoch, Molecules, 2018, 23, 2028 CrossRef PubMed
.
- A. Ellert-Miklaszewska, N. Ochocka, M. Maleszewska, L. Ding, E. Laurini, Y. Jiang, A.-J. Roura, S. Giorgio, B. Gielniewski, S. Pricl, L. Peng and B. Kaminska, Nanomedicine, 2019, 14, 2441–2459 CrossRef CAS PubMed
.
- H. Sarin, A. S. Kanevsky, H. Wu, K. R. Brimacombe, S. H. Fung, A. A. Sousa, S. Auh, C. M. Wilson, K. Sharma, M. A. Aronova, R. D. Leapman, G. L. Griffiths and M. D. Hall, J. Transl. Med., 2008, 6, 80 CrossRef PubMed
.
- K. H. Wong, Z. Guo, M.-K. Law and M. Chen, Biomater. Sci., 2023, 11, 1589–1606 RSC
.
- A. Janaszewska, J. Lazniewska, P. Trzepiński, M. Marcinkowska and B. Klajnert-Maculewicz, Biomolecules, 2019, 9, 330 CrossRef CAS PubMed
.
- K. Liaw, F. Zhang, A. Mangraviti, S. Kannan, B. Tyler and R. M. Kannan, Bioeng. Transl. Med., 2020, 5, e10160 CrossRef CAS PubMed
.
- M. K. Mishra, C. A. Beaty, W. G. Lesniak, S. P. Kambhampati, F. Zhang, M. A. Wilson, M. E. Blue, W. A. Baumgartner and R. M. Kannan, ACS Nano, 2014, 8, 2134–2147 CrossRef CAS PubMed
.
- F. Zhang, P. Mastorakos, M. K. Mishra, A. Mangraviti, L. Hwang, J. Zhou, J. Hanes, H. Brem, A. Olivi, B. Tyler and R. M. Kannan, Biomaterials, 2015, 52, 507–516 CrossRef CAS PubMed
.
- A. Sharma, K. Liaw, R. Sharma, T. Spriggs, S. Appiani La Rosa, S. Kannan and R. M. Kannan, Biomacromolecules, 2020, 21, 5148–5161 CrossRef CAS PubMed
.
- K. Liaw, R. Sharma, A. Sharma, S. Salazar, S. Appiani La Rosa and R. M. Kannan, J. Controlled Release, 2021, 329, 434–444 CrossRef CAS PubMed
.
- B. Jafari, M. M. Pourseif, J. Barar, M. A. Rafi and Y. Omidi, Expert Opin. Drug Delivery, 2019, 16, 583–605 CrossRef CAS PubMed
.
- Z. Jin, L. Piao, G. Sun, C. Lv, Y. Jing and R. Jin, J. Drug Targeting, 2020, 29, 323–335 CrossRef PubMed
.
- Y. Lu, S. Han, H. Zheng, R. Ma, Y. Ping, J. Zou, H. Tang, Y. Zhang, X. Xu and F. Li, Int. J. Nanomed., 2018, 13, 5937–5952 CrossRef CAS PubMed
.
- L. Zhang, S. Zhu, L. Qian, Y. Pei, Y. Qiu and Y. Jiang, Eur. J. Pharm. Biopharm., 2011, 79, 232–240 CrossRef CAS PubMed
.
- K. Wang, X. Zhang, Y. Liu, C. Liu, B. Jiang and Y. Jiang, Biomaterials, 2014, 35, 8735–8747 CrossRef CAS PubMed
.
- Y. Bertrand, J. C. Currie, J. Poirier, M. Demeule, A. Abulrob, D. Fatehi, D. Stanimirovic, H. Sartelet, J. P. Castaigne and R. Béliveau, Br. J. Cancer, 2011, 105, 1697–1707 CrossRef CAS PubMed
.
- Y. Chen, R. Huo, W. Kang, Y. Liu, Z. Zhao, W. Fu, R. Ma, X. Zhang, J. Tang, Z. Zhu, Q. Lyu, Y. Huang, M. Yan, B. Jiang, R. Chai, Z. Bao, Z. Hu, W. Wang, T. Jiang, Y. Cao and J. Wang, Cell Rep. Med., 2023, 4, 101177 CrossRef CAS PubMed
.
- C. Liu, Z. Zhao, H. Gao, I. Rostami, Q. You, X. Jia, C. Wang, L. Zhu and Y. Yang, Nanotheranostics, 2019, 3, 311–330 CrossRef PubMed
.
- H. Li and Z. M. Qian, Med. Res. Rev., 2002, 22, 225–250 CrossRef CAS PubMed
.
- H. He, Y. Li, X.-R. Jia, J. Du, X. Ying, W.-L. Lu, J.-N. Lou and Y. Wei, Biomaterials, 2011, 32, 478–487 CrossRef CAS PubMed
.
- Y. Li, H. He, X. Jia, W.-L. Lu, J. Lou and Y. Wei, Biomaterials, 2012, 33, 3899–3908 CrossRef CAS PubMed
.
- T. S. Patil and A. S. Deshpande, J. Controlled Release, 2020, 320, 239–252 CrossRef CAS PubMed
.
- E. C. Calvaresi and P. J. Hergenrother, Chem. Sci., 2013, 4, 2319 RSC
.
- R. Sharma, K. Liaw, A. Sharma, A. Jimenez, M. Chang, S. Salazar, I. Amlani, S. Kannan and R. M. Kannan, J. Controlled Release, 2021, 337, 179–192 CrossRef CAS PubMed
.
- J. Ban, S. Li, Q. Zhan, X. Li, H. Xing, N. Chen, L. Long, X. Hou, J. Zhao and X. Yuan, Macromol. Biosci., 2021, 21, 2000392 CrossRef CAS PubMed
.
- D. Wu, M. Qin, D. Xu, L. Wang, C. Liu, J. Ren, G. Zhou, C. Chen, F. Yang, Y. Li, Y. Zhao, R. Huang, S. Pourtaheri, C. Kang, M. Kamata, I. S. Y. Chen, Z. He, J. Wen, W. Chen and Y. Lu, Adv. Mater., 2019, 31, 1807557 CrossRef PubMed
.
- T. Goda, K. Ishihara and Y. Miyahara, J. Appl. Polym. Sci., 2015, 132, 41766 CrossRef
.
- W. Liyanage, T. Wu, S. Kannan and R. M. Kannan, ACS Appl. Mater. Interfaces, 2022, 14, 46290–46303 CrossRef CAS PubMed
.
- X. Xu, K. Liu, Y. Wang, C. Zhang, M. Shi, P. Wang, L. Shen, J. Xia, L. Ye, X. Shi and M. Shen, J. Mater. Chem. B, 2019, 7, 3639–3643 RSC
.
- J. Zhao, B. Zhang, S. Shen, J. Chen, Q. Zhang, X. Jiang and Z. Pang, J. Colloid Interface Sci., 2015, 450, 396–403 CrossRef CAS PubMed
.
- Y. Jiang, L. Lv, H. Shi, Y. Hua, W. Lv, X. Wang, H. Xin and Q. Xu, Colloids Surf., B, 2016, 147, 242–249 CrossRef CAS PubMed
.
- C. Song, Z. Ouyang, Y. Gao, H. Guo, S. Wang, D. Wang, J. Xia, M. Shen and X. Shi, Nano Today, 2021, 41, 101325 CrossRef CAS
.
- R. Huang, W. Ke, L. Han, J. Li, S. Liu and C. Jiang, Biomaterials, 2011, 32, 2399–2406 CrossRef CAS PubMed
.
- K. Karki and J. R. Ewing, J. Nanomed. Nanotechnol., 2016, 7, 2157–7439 Search PubMed
.
- Y. Bae, J. Lee, C. Kho, J. S. Choi and J. Han, Korean J. Physiol. Pharmacol., 2021, 25, 467–478 CrossRef CAS PubMed
.
- C. Z. Bai, S. Choi, K. Nam, S. An and J.-S. Park, Int. J. Pharm., 2013, 445, 79–87 CrossRef CAS PubMed
.
- X. Xu, J. Li, S. Han, C. Tao, L. Fang, Y. Sun, J. Zhu, Z. Liang and F. Li, Eur. J. Pharm. Sci., 2016, 88, 178–190 CrossRef CAS PubMed
.
- A. K. Sharma, L. Gupta, H. Sahu, A. Qayum, S. K. Singh, K. T. Nakhate, Ajazuddin and U. Gupta, Pharm. Res., 2018, 35, 9 CrossRef PubMed
.
- Z. Xu, Y. Wang, Z. Ma, Z. Wang, Y. Wei and X. Jia, Polym. Chem., 2016, 7, 715–721 RSC
.
- X. Shi, R. Ma, Y. Lu, Y. Cheng, X. Fan, J. Zou, H. Zheng, F. Li and J.-G. Piao, Biochem. Biophys. Res. Commun., 2020, 527, 117–123 CrossRef CAS PubMed
.
- S. Huang, J. Li, L. Han, S. Liu, H. Ma, R. Huang and C. Jiang, Biomaterials, 2011, 32, 6832–6838 CrossRef CAS PubMed
.
|
This journal is © The Royal Society of Chemistry 2024 |
Click here to see how this site uses Cookies. View our privacy policy here.