DOI:
10.1039/D3YA00306J
(Perspective)
Energy Adv., 2023,
2, 1591-1603
Amorphous MOFs for next generation supercapacitors and batteries
Received
28th June 2023
, Accepted 7th August 2023
First published on 8th August 2023
Abstract
Metal–organic frameworks (MOFs) are porous materials comprised of metal nodes and organic linkers, which feature highly tunable structures and compositions that can be tailored for energy storage applications. While MOFs research in this area has predominantly focused on the crystalline domain, amorphous MOFs have also attracted increasing interests. Amorphpus MOFs, which lack any long-range periodic order in the framework, exhibit several properties that are beneficial for energy storage such as isotropic conduction, higher ionic and electrical conductivity, increased defect sites and enhanced electrochemical stability. This perspective aims to highlight the recent developments in amorphous MOFs including amorphous discrete powder, glassy and composite forms for batteries and supercapacitors. Additionally, we will also provide prospective areas for the future development of amorphous MOFs in this field.
Introduction
With the remarkable advancements in technology witnessed in the past century, there has been a substantial surge in the demand for energy and its consumption. Consequently, there has been a notable shift away from fossil fuels as society strives towards a cleaner energy future, driven by stringent environmental policies. Presently, various battery technologies, including supercapacitors, lithium-ion batteries (LIB), and lithium–sulphur batteries, have garnered considerable attention due to their exceptional energy storage capacities and conversion efficiencies. However, it is important to address the significant capacity and stability challenges faced by both the electrodes and electrolytes in these batteries, as they can result in performance degradation during operation. Therefore, there is an urgent need for further advancements and improvements in battery technology development.19,20
Metal–organic frameworks (MOFs) or porous coordination polymers belong to a class of hybrid compounds that combine metal ions or clusters with organic ligands. This unique combination imparts them with several advantageous properties such as high surface area, tuneable pore sizes, and ease of modification.21 As a result, MOFs have found extensive utility in diverse fields, ranging from catalysis22,23 to gas separation,24–26 gas adsorption26,27 and recently electrochemical applications.28–31
MOFs have emerged as promising materials in the field of energy storage, leveraging their high tunability to achieve enhanced electrical performance. For example, MOFs tuning allows for improved electron access to active centres and, consequently, enhances the utilization of these active sites on MOFs, leading to superior performance in many fields such as electrocatalysis.32,33 Furthermore, tuning of the metals, ligands and pore structures of MOFs can also enable fast and unimpeded ion transport leading to improvements in the rate performance, cycling stability and power density of energy storage devices.34,35
Although MOFs research in the energy field have predominantly focused on the crystalline domain, amorphous MOFs have also garnered increasing attention. This is because amorphous MOFs exhibit a relatively high energy state, possess abundant defect sites, and can offer isotropic ion conduction pathways.13,36,37 For example, Zhang et al. found that amorphous MIL-88B (Fe3O [C6H4(CO2)2]3OH·nH2O) has better rate and cycle performance in lithium–sulphur battery compared with its crystalline counterpart.38
Hitherto, there has been limited reviews focusing on amorphous MOFs for energy storage, despite their significant potentials in this area.20,39,40 Given the recent developments on amorphous MOFs in energy storage (Fig. 1), we aim to highlight and provide our perspectives on these materials, which encompasses discrete amorphous MOF powders, melt-quenched MOF glasses and amorphous MOF composites. We will focus on two specific energy storage fields, including supercapacitors and batteries (lithium-ion batteries (LIB), lithium–sulphur batteries etc.). Furthermore, we will provide future prospects of amorphous MOFs in this field. It is envisioned that this perspective will provide useful insights into the development of amorphous MOFs and their composites for energy storage.
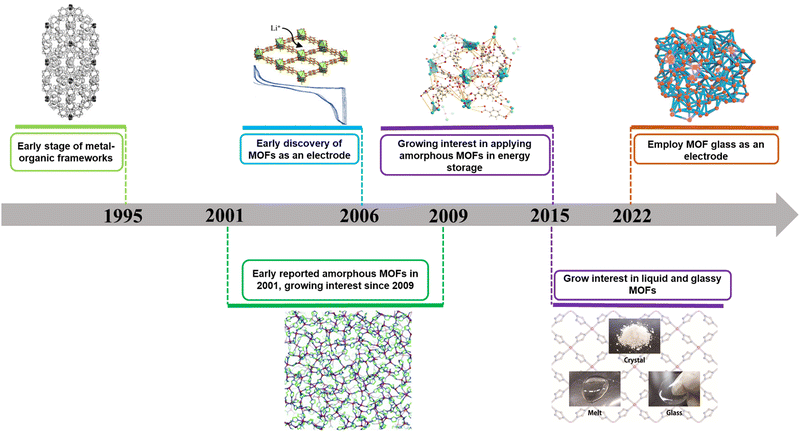 |
| Fig. 1 Roadmap of development of amorphous MOFs and its application in batteries and supercapacitors.1–11 | |
Amorphous MOFs for energy storage
What are amorphous MOFs?
Majority of MOFs research including the energy storage area has focused on the crystalline domain. Crystalline MOFs have well defined and ordered structures. In other words, metal ions and organic ligands are arranged in repeated continuous patterns, featuring both long-range and short-range orders. Therefore, crystalline MOFs structures can be characterised by X-ray diffraction analysis and differentiated by the positions of diffraction peaks. Normally, crystalline MOFs are fabricated via solvothermal or hydrothermal approaches. Mechanochemical milling can also fabricate crystalline MOFs, but it is likely to introduce amorphous phases.41,42
In contrast to crystalline MOFs, non-crystalline MOFs, also called amorphous MOFs, feature a random distribution of metal ions and ligands within the framework, lacking any long-range order (Fig. 2). Consequently, amorphous MOFs display only diffuse scattering in X-ray or neutron diffraction patterns.12
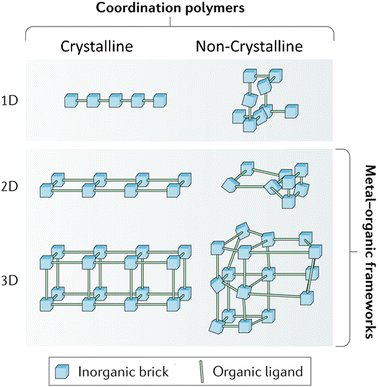 |
| Fig. 2 Crystalline vs. non-crystalline. Reproduced from ref. 12 with permission from Springer Nature, copyright 2018. | |
How to obtain amorphous MOFs
Amorphous MOFs can be obtained through amorphization of crystalline MOFs, which is an entropy driven process. The amorphization process can be described as the following three steps (Fig. 3): Firstly, stress is applied to the crystalline MOFs. This causes the long-range order to be gradually lost due to accumulation of defects within the stressed MOFs’ lattice. Meanwhile, entropy and enthalpy also increased due to the stress applied (State 1). When the increase in enthalpy caused by stress (ΔHstress) is equal to the enthalpy of transition from perfect crystalline to amorphous phase (ΔHCa), the long-range configuration of the atoms is no longer constrained. All atoms rotate and rearrange locally to the amorphous configuration where only short-range order exist. It is important to note that the crystal to amorphous transformation might be isenthalpic in some cases where there is a sudden and dramatic increase in entropy (State 2). In the final stage, no significant change in entropy or enthalpy is seen (Stage 3).13
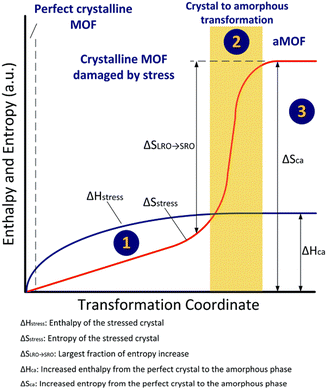 |
| Fig. 3 Schematic representation of amorphization. Reproduced from ref.13 with permission from Royal Society of Chemistry, copyright 2021. | |
Amorphization can be achieved through two routes, namely the top-down synthesis route and bottom-up synthesis route. Top-down synthesis involves the application of an external stimulus such as pressure, heat or radiation on pre-synthesized crystalline MOFs. This results in a change, loss or damage in the MOFs’ lattice, porosity, channels, geometry, and connection.5,43–45 Long-range order cannot be maintained and the MOFs gradually lose their crystallinity. The outcome is a disordered, amorphous MOFs with an abundance of defect sites. On the other hand, bottom-up synthesis represents the direct synthesis of amorphous MOFs from metals and ligands precursors, which is regulated by the choices of precursors and synthesis conditions.46 There are three methods to control the direct synthesis of amorphous MOFs. The first method is to use flexible and/or asymmetric ligands that have strong connection with metal ions, such as amino acids and nucleotides, to directly fabricate amorphous MOFs.47,48 Apart from ligands, templates and seeding agents can also be used to increase the local concentration of metal ions or ligands, which can help to form amorphous MOFs.49–51 Lastly, synthesis conditions are also an important parameter that regulates MOFs’ crystallinity. Solvent, reaction time, pH, type of metal salt precursors, metal to ligand ratios and concentration can all impact the crystallinity of MOFs.52
A synthesis route that is only applicable to a subgroup of amorphous MOFs is melt-quenching. This process requires the MOFs to have an accessible melting temperature that is below its decomposition temperature. This means that the covalent bonds within the ligands have to be stronger than the coordinative bonding between metal nodes and ligands to avoid decomposition.44,53 Upon heat treatment, a transition from solid to liquid phase can be observed at the melting temperature (Tm). Subsequent rapid cooling of the melt, otherwise known as quenching, can avoid re-crystallisation and form an amorphous solid called melt-quenched MOF glass (MQG). The presence of a glass transition temperature (Tg) in this amorphous solid is required to classify it as a glass.44 The ability of a glass-forming liquid to resist crystallisation during cooling to form glass is called the glass forming ability.54,55 Generally, low GFA melting liquid will require faster quenching rate to overcome crystallisation and vice versa. The Kauzmann “2/3” law denotes that the Tg of a glass is empirically about 2/3 of its melting point (Tm), and thus a melt with a Tg/Tm ratio higher than 2/3 is considered to have a high GFA. It is commonly accepted that GFA is also associated with liquid fragility, crystal density, glass density and viscosity at Tm.7
Compared to crystalline MOFs, amorphous MOFs and MOF glasses tend to have lower porosity and surface area, but they feature higher ionic and electrical conductivity and more defect sites.12,56,57 The use of amorphous MOFs in the MOF composites can provide new order–disorder structural configurations, thereby expanding the variety of composite structures and properties in the energy storage field. Moreover, amorphous MOFs have also been demonstrated to act as a suitable host matrix for a variety of functional guest materials.58–60 This paper will mainly discuss amorphous MOFs in discrete powders form including its sub-family of melt-quenched MOF glass (referred as MOF glass in the following content). In addition, we will also discuss composites based on amorphous MOFs.
Discrete amorphous MOFs powders
The most common form factor of amorphous MOFs is discrete powders. Normally, MOF materials exhibit lower electrical conductivity with the loss of crystallinity due to the loss of porosity, lower surface area as well as the size imperfections.61,62 However, amorphous MOFs can improve conductivity through other aspects, such as providing additional structural defect sites and unsaturated coordination sites that increase the active conductive surface area. This enables amorphous MOFs to be a promising candidate for energy storage purposes. For example, Yang et al. found that amorphous UiO-66 (Zr6O4(OH)4(BDC)6; BDC = 1,4-benzodicarboxylate) as illustrated in Fig. 4 has significantly higher specific capacitance (910 F g−1) compared to crystalline UiO-66 (452 F g−1). After 5000 cycles, the capacitance (610 F g−1) of the amorphous phase remained high, which was over 1.5 times that of crystalline UiO-66. The improvement in performance was attributed to the increase in exposure of redox-active ligands resulting from the MOFs’ metastable state and disorder within frameworks. Additionally, amorphous UiO-66 also exhibited higher thermal stability as indicated by the higher decomposition temperature compared to the crystalline counterpart.6
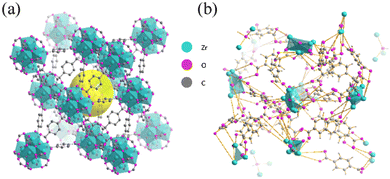 |
| Fig. 4 Schematic representation of UiO-66 structures in the (a) crystal and (b) amorphous forms gained from reverse Monte Carlo (RMC) modelling. Amorphous UiO-66 has promising potential as a supercapacitor electrode material. Reproduced from ref. 6 with permission from Elsevier, copyright 2018. | |
Layered materials are also quite common in the energy storage field.63 The application of amorphous MOFs with layered structures and large inter-sheet spacings can result in low diffusive resistance and high ion transport kinetics. Cui et al. fabricated amorphous Ni-pPD MOFs nanosheets (a-Ni-pPD, pPD = p-phenylenediamine) with large inter-sheet spacing of 1.6 nm induced by polarization. When coupled with an active carbon cathode, a-Ni-pPD showed a high gravimetric capacitance (259 F g−1) and high areal capacitance (2.9 F cm−2) at 2 A g−1 with a wide potential window of 2.85 V. High cycling stability was also observed where around 80% capacity was retained over 12
000 cycles.64
Amorphization can induce structural changes and bond rearrangement, so that new chemical bonding is likely to be introduced. New chemical bonding can generate new electron transport pathways and hence improve the electrical performance. Xiu et al. found that electrically bistable MOFs CuI[CuIII(pdt)2] (pdt = 2,3-pyrazinedithiolate) can undergo reversible crystal-amorphous transformation upon external stimulation, and both forms (crystal and amorphous) can stably exist at ambient condition. After examination, they found that the reversible transformation was closely related to the adsorption and removal of acetonitrile (CAN). Interestingly, amorphous CuI[CuIII(pdt)2] exhibited 130% higher electrical conductivity compared to the crystalline form. The enhancement arose from conservation of functional groups in the structure and additional electron pathway (new Cu–S bonding) created during amorphization process.65 This work opened the door for the application of amorphous MOFs as a switchable electrical conductor. Similar observations have also been made by Tomoinaka and co-workers, where amorphization created Cu–S–Cu bonding (CuSx network) that improved electron transfer (Fig. 5). This turned crystalline CuICl(ttcH3) (ttcH3 = trithiocyanuric acid) that was originally an insulator into an amorphous CuI1.8(ttc)0.6(ttcH3)0.4 semiconductor.15
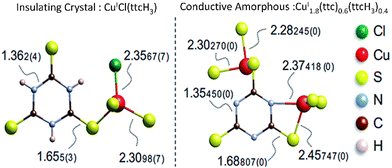 |
| Fig. 5 Local structure and bond lengths of CuICl(ttcH3) and CuI1.8(ttc)0.6(ttcH3)0.4. Reproduced from ref. 15 with permission from Royal Society of Chemistry, copyright 2015. | |
Melt-quenched MOF glasses (MQG)
Glassy materials are known since the early days to be excellent ion conductors, such as glassy ceramics,66 alkali silicate glasses67 and oxide glasses.68 Due to the enhanced ion dynamics and the presence of isotropous conduction pathways, the glassy form of MOFs often exhibits superior ion conductivity over their crystalline phases, thereby improving the energy storage performances.44,69,70 The heat treatment applied during the synthesis of MQG also serves as an activation step for solvent removal, which provides more active surface area and less potential solvent pollution with better processability than crystal. (Fig. 6)
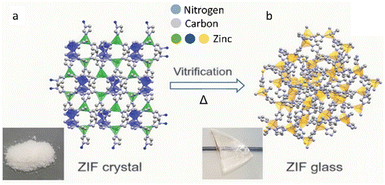 |
| Fig. 6 Schematic representation of ZIF-62 structures in the (a) crystal and (b) glass forms. Reproduced from ref. 14 with permission from The American Association for the Advancement of Science, copyright 2020. | |
A disordered phase has a relatively higher potential energy than an ordered crystalline phase. As MOF glass is in a metastable state with high potential energy, the structure can be easily affected by the insertion/extraction of Li+ during the charge/discharge cycles, which can increase the size and number of conduction pathways. The formation of these additional and larger channels can benefit lithium-ion diffusion and storage (Fig. 7).9 Furthermore, the presence of structural defect sites in MOF glass such as broken bonds and unsaturated coordination sites, coupled with the MOFs glass’ higher active surface area for electrochemical reaction, can also enhance the performance. In contrast, the energy storage performance of crystalline MOFs might be restricted by their anisotropic properties, low degree of depolymerisation and low concentration of structural defects.9
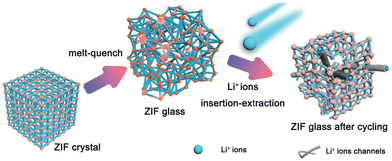 |
| Fig. 7 Schematic indication of how ZIF-62 (Co) glass enhance the performance. Reproduced from ref. 9 with permission from John Wiley and Sons, copyright 2022. | |
Compared with other amorphous MOFs, MOF glasses have higher degree of disorder, even in short range. Increasing disorder can effectively shorten the diffusion distance of ions, which can help to promote ion transport kinetics and ion intercalation within the material.9,14,71 Gao et al. investigated the use of crystalline, amorphous and glassy states of cobalt-ZIF-62 (Co(Im)1.75(bIm)0.25, where Im = imidazole, bIm = benzimidazole) as anodes in a lithium-ion battery. It was observed that the lithium storage performances such as capacity, rate capability and cycling stability followed the order of glassy state > amorphous state > crystal state.9 The enhancement in performance was attributed to the increase in distortion and local breakage of Co–N bonding which made Li-ion intercalation more accessible. Generated transfer channels exhibit enhanced stability, allowing a capacity increase of up to 1500 cycles. Furthermore, these channels also facilitate Li+ ion diffusion and storage.9
Amorphous MOFs in MOF composites
Advantage of MOF composites in energy storage field.
Researchers have investigated the incorporation of electronically conductive materials into MOFs to create MOF composites. This raises the question of whether the energy storage performance is enhanced in these composites compared to the original materials. The majority of MOFs are generally regarded as poor electrical conductors due to several factors, such as the presence of a wide bandgap, redox-inactive organic ligands, and limited pathways for low-energy electron transfer. These intrinsic barriers pose challenges for the practical utilization of MOFs as conductive media and therefore compositing can be a potential solution.72
Researchers have discovered that certain Fe-based MOFs possess favourable redox activity and lithium-ion mobility, leading them to be used as electrodes as early as 2007. However, the electrical performance of these MOFs was found to be relatively modest.11 Subsequently, numerous researchers have endeavoured to employ MOFs as electrodes. Unfortunately, these attempts have often resulted in significant performance degradation, low capacity, and inherent mechanical brittleness.73–75
A prevalent observation with crystalline MOFs during the lithiation/delithiation process is their tendency to undergo structural collapse and irreversible amorphization due to their limited structural stability.18,76 As a consequence, it may no longer be suitable for use as an electrode. However, in contrast, MOF composites may benefit from the lithiation/delithiation process. For example, Gao and his co-workers fabricated graphene/Al(OH)[O2C–C6H4–CO2] composite (also called AMG in Fig. 8) and found that the lithiation/delithiation process resulted in more open channels in MOFs for Li+ ions migration and storage, and hence result in enhanced conductivity and lithium ion storage capacity.17 Meanwhile, the introduction of graphene exhibit additional advantages arising from the synergistic effects. Graphene sheets can help to facilitate the interconnection of MOFs particle in composite and promote the Li+ migration on the solid-electolyte interface (SEI) as well as charger transfer reaction during operation.17
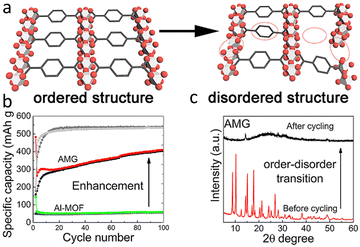 |
| Fig. 8 (a) Structural change of AMG from crystal state to amorphous state during operation of lithium-ion battery. (b) Performance enhancement of AMG after amorphization (c) XRD result of AMG before and after cycling. Reproduced from ref. 17 with permission from Elsevier, copyright 2019. | |
Although MOF composites are actively being used for energy storage purposes, the diversity of MOF composites remains rather limited. Secondary materials incorporated into MOFs usually need to have desirable properties such as high electrical conductivity, good chemical compatibility as well as high stability and excellent mechanical strength. Only a limited number of guest materials have been used so far, with carbon nanotubes (CNTs),77 graphene (G),17,78,79 graphene oxide (GO),80 reduced graphene oxide (rGO)81–83 and conductive polymers84,85 being the main guest materials of choice. For MOFs, the metal ions are usually transition metals such as Fe, Mn and Ni, while organic ligands are normally based on carboxylates, N-donor groups, sulfonates, phosphonates, or heterocyclic compounds.76,86,87 Further exploration of MOF composites beyond the crystalline form towards amorphous MOF based composites can offer a potential solution to expand the diversity and performance of these materials in energy storage applications.
Amorphous MOF composites vs. Crystalline MOF composites
Amorphous MOFs have several advantages over their crystalline counterparts, making them highly desirable for composite applications. Firstly, amorphization can increase and expose active metal sites of MOFs to secondary materials, which can promote better delivery of electrons and interfacial contact with conductive medium.13,36 Furthermore, the higher energy state and disorderly structure of amorphous MOFs make them more amenable to functionalization, providing increased opportunities for chemical modification.53(Fig. 3) This flexibility allows for tailoring of the material properties to meet specific application requirements. Additionally, amorphous MOFs tend to exhibit superior mechanical and thermal stability compared to their crystalline counterparts,36 which can be useful in composite applications where these properties are important. Lastly, some amorphous MOF layers can exhibit oxygen storage capability. The stored oxygen in MOFs could help to mitigate issues caused by oxygen loss in electrodes such as Li-rich layered oxide, which can provide enhanced performance and stable operation.88
In a composite, enhancements in performance can arise from improved interfacial contact and interactions between MOF and secondary material. The amorphization process can help to increase and expose the active metal sites of MOFs to secondary materials for improved delivery of electrons. Lin et al. composited amorphous Ni-MOFs nanowires with conductive Ni carbon cloth (Ni/CC) to form a fisher-net superstructure. (Fig. 9) The composite featured abundant active sites and significantly increased pore volume, specific surface area and conductive surface area, which synergistically promoted electrical conduction and ion transportation within composite.18 Another similar example was shown by Zhao et al., where silver nanowire was composited into a 2-D amorphous MOF to form a conductive nanocomposite (a-CoL/Ag NC). The fabricated composite exhibited a higher specific capacitance, rate capacity, and long-term cycling stability compared to the parental materials when used as electrode. This was due to the enhanced interfacial interactions between composite and electrolyte, which facilitated ion and electron transport.89 Zhao and co-workers also suggested that amorphous MOFs can achieve better interfacial interaction with secondary materials compared to the crystalline counterpart due to the higher energy state of amorphous materials.
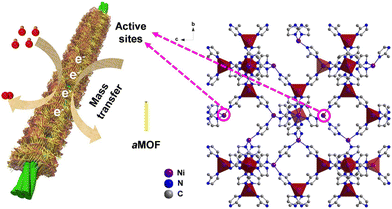 |
| Fig. 9 Schematic of fishnet-like composites of amorphous Ni-MOFs nanowires grown on conductive Ni carbon cloth (Ni/CC). Reproduced from ref. 18 with permission from Elsevier, copyright 2020. | |
In addition, some amorphous MOFs also showed unique properties which can be beneficial in a composite material for energy storage purposes. In a pioneering work by Qiao and co-workers, an amorphous Mn-based MOF layer (2–3 nm thickness) was coated on a crystalline Li-rich layered oxide. The composite exhibited an increased discharge capacity (323.8 mA h g−1 at 0.1C rate), high initial Coulombic efficiency (91.1%), and good thermal stability without impacting the cycling performance and high-rate capability. The observed enhancement was attributed to the oxygen storage ability of the amorphous MOF layer. This opens a new avenue of research by using amorphous MOFs for surface modification to achieve higher discharge capacity in lithium-ion battery.88
Furthermore, MOF as a host matrix can also be used to protect guest materials from external stimuli and limit internal alterations via confinement.90 However, structural compatibility is always an issue during compositing. Poor compatibility is likely to lead to problems such as poor stability and pore blocking.91 The use of amorphous MOFs, particularly MOF glass, is a good option for compositing as the high chemical reactivity of the liquid state upon melting can promote better binding with the secondary materials. In addition, structural disorder can facilitate higher ion conductivity and enhanced ion dynamics.58,92,93 Nozari et al. composited sodium-containing ionic liquid (sodium bis(trifluoromethylsulfonyl)imide) into ZIF-8. Although the fabricated composite showed good ionic conductivity and low activation energy, it had relatively poor stability at ambient condition. Further partial amorphization of the composite by ball milling resulted in significant improvements in stability compared to the crystalline structure. (Fig. 10) The induced structural disorder reduced the pore size, which hindered ionic liquid exudation and prevented them from contact with the atmosphere. There was only a 6% decrease in performance after 20 days compared to 20% for the crystalline ZIF-8 composite. In terms of the activation energy, it remained almost unchanged for the partially amorphized composite with only a slight increase to 0.28 eV after 6 days of exposure, while there was an approximately 58% increase for the crystalline composite from 0.26 eV to 0.4 eV. Although amorphization also disrupted the interconnected conduction channels in MOF, the composite still maintained superionic behaviour.16 Similar observations were also made by Qiao and co-workers.88
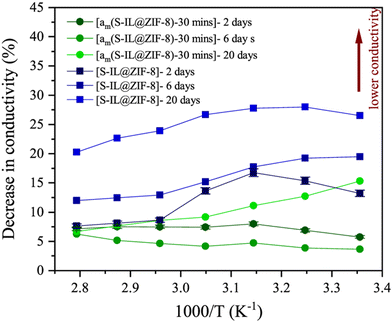 |
| Fig. 10 Change in the ionic conductivity of crystalline (square) and amorphous composite (circle). Reproduced from open access journal ref. 16. | |
Conclusion and perspectives
As reviewed, amorphous MOFs have great potential applications in energy storage fields. However, due to limited understanding and relatively short-term investigations of disordered amorphous MOFs, its potentials are still uncovered. We hope the following perspectives can give researchers ideas and direction about the future development of amorphous MOFs in energy storage fields.
Development of MOF liquid/glasses
An interesting theoretical study that has been reported is that a large fraction of void space (95%) seems to be retained in ZIF-4 liquid at 1500 K, which is higher than its crystal counterpart (74%) at 300 K. This finding suggests the retention of porosity in MOF liquid even at high temperature. The larger pore volume of MOF liquid compared to the corresponding glassy state makes it particularly intriguing for energy storage under extreme conditions.94 Nevertheless, there remains some bottlenecks for the development of MOF liquids. Firstly, majority of MOFs undergo decomposition (Td) before reaching the melting temperature (Tm), which limits the diversity of this group of MOFs. In addition, the high melting temperature of meltable MOFs makes it difficult to stabilise them or perform experiments under this condition. For example, liquid ZIF-76 (Zn(Im)1.62(cPhIm)0.38) can only be obtained above its melting temperature of 451 °C and many characterisations, such as nuclear magnetic resonance (NMR), cannot proceed at such high temperature. In order to employ MOF liquids in the energy storage field, there is an urgent need to stabilise MOF liquids at ambient conditions for it to be practically feasible. Several efforts have already been made to lower Tm, such as ionic liquid incorporation and introduction of external pressure.95–97 Efforts in these directions are vital to unlock the full potential of MOF liquids for energy storage applications.
MOF composites
Further efforts should be dedicated to investigating the synergistic effects between the parent materials in MOF composites to enhance their overall performance. Compatibility is the first thing to be considered, MOF glass emerges as a favourable candidate as a host matrix due to its high processability and chemical reactivity with functional materials during composite formation and melting. Moreover, by subjecting MOF glass composites to heat treatment, it is possible to regulate and eliminate interfacial defects, further improving their performance.60 The utilization of MOF glass composites for energy storage purposes is currently in its nascent stages, with only a limited number of published papers focusing on anode applications.9,98 Given the high moldability and excellent compatibility of glassy MOFs with guest materials, it has huge potential to be used as other energy storage components. Recent research has revealed that MOF glasses exhibit an even higher level of disorder compared to other amorphous MOF materials. This increased disorder can effectively shorten the diffusion distance of ions, thereby enhancing ion transport kinetics within the material.9,14,71 As a result, we anticipate that in the future, we will witness a growing body of research on glassy MOF composites, exploring their application across a wider range of energy storage systems.
Additionally, it is crucial to acknowledge that the formation of MOF composites often leads to the disruption of the intrinsic organic–inorganic hybrid structure of MOFs. To ensure stable operation over extended periods, it becomes necessary to prioritize the preservation of the structural integrity of MOFs. This involves maintaining the active surface area and promoting good conductivity throughout the composite material. By preserving the original hybrid structure of MOFs, one can maximize their inherent properties and facilitate efficient charge transfer, leading to enhanced performance and long-term stability.9,99,100 Strategies that promote the structural integrity of MOFs within composites should be explored to ensure their successful application in energy storage and other fields. This includes, but is not limited to, careful selection of guest materials, optimization of synthesis conditions, and the development of appropriate fabrication techniques that minimize structural damage while achieving desired composite properties.
Benefiting from structural disorder
Structural disorder is the main difference between crystalline MOFs and amorphous MOFs, and there are still promising potentials to be uncovered.101 The abundance of unsaturated sites within amorphous MOFs offers opportunities for diverse applications alongside energy storage purposes, including their use as catalysts, which endows amorphous MOFs with multi-functionality. For instance, Zhang et al. employed amorphous MIL-88B (Fe3O [C6H4(CO2)2]3OH·nH2O) separator that can convert polysulfide to the non-soluble form in lithium sulphur battery.38 By harnessing the abundant unsaturated coordination sites in amorphous MOFs, scientists can exploit their potential to achieve enhanced performance across a range of applications.
Meanwhile, the incorporation of structural disorder in MOF composites holds the potential to enhance the stability of energy storage devices. With the wide range of MOF composites already reported, there are ample opportunities to further enhance their performance stability by exploring amorphization. Establishing a clear relationship between the degree of amorphization and the resulting stability of the composite materials would be highly valuable, offering significant implications for advancements in the field of energy storage. By understanding and optimizing the extent of amorphization, researchers can unlock new strategies to improve the long-term stability and reliability of MOF composites in energy storage applications. However, this requires careful materials structure investigation to understand the gradual loss of the long-range order.
In the context of energy storage, such as lithium-ion batteries, 2D materials are considered favourable options due to their ability to undergo intercalation, driven by covalent interactions within layers and van der Waals interactions between layers.102 When it comes to 2D amorphous materials, the weakened interaction between layers facilitates diffusion, making them particularly promising for energy storage applications.103 Additionally, 2D amorphous MOFs exhibit increased intersheet spacing under significant polarization, further enhancing their potential for energy storage purposes.64 In the future, there is ample room for exploration and research on 2D amorphous MOFs, and even the development of MOF glass sheets, to unlock their full potential as versatile materials for energy storage applications.
Apart from varying dimensionality, scientists could also try to add electron withdrawing group. Hou et al. found that use of electron withdrawing group can weaken the organic–inorganic bonding, and the smaller van der Waals volume of the functional group can reduce steric hindrance around the metal node to facilitate its dissociation. The incorporation of electron withdrawing group into MOFs could be another possible strategy to improve conductivity. This is because easier breakage of organic–inorganic bonding and higher degree structural disorder caused by the introduction of electron withdrawing group could help to create new channels for ion diffusion and storage during the cycling process in batteries.104 However, it is also necessary to investigate the stability of generated channels to fulfil long-term operation.
Notably, structural changes in amorphous MOFs are inevitable during operation and hence short-range order might be hard to maintain. However, there is currently no comprehensive comparison between short-range order and disorder in energy storage fields for MOFs. Even though MOF glasses, featuring higher degree of disorder, have shown better result in lithium battery application compared with other counterparts,9,14 further investigations are still needed to gain deeper understanding of this phenomenon.
Fabrication of amorphous MOFs
In addition to traditional melting methods, a recently developed approach for melting MOFs involves the use of high-energy ultrafast femtosecond (fs) infrared (IR) laser pulses. Kulachenkov et al. successfully fabricated well-organized spheres comprised of a metal oxide dendrite core and an amorphous organic shell from MOFs, which have the potential for producing a new generation of materials.94,105 Moreover, the ultrafast melting method can find application in fabricating melt-quenched MOF glasses. This could result in intriguing morphologies and the formation of larger glassy particles, further expanding the range of materials that can be explored for energy storage purposes.94,105
Direct synthesis of amorphous MOFs is still in its early stages and further development is needed. Direct synthesis generally does not require extreme condition, such as high pressure and temperature as is the case in top-down synthesis. Its mild and ambient synthesis condition can help to reduce the energy consumption and tends to be more environmentally friendly. Additionally, mild condition is especially beneficial for fragile materials, such as biomedical materials and drugs. Meanwhile, direct synthesis allows for relatively more control in coordination kinetics and amorphousness during fabrication, which could be helpful for scientists to investigate the optimum degree of amorphousness in energy storage applications.46
Understanding the properties and structure
Another important consideration prior to the application of amorphous MOFs is to obtain a fundamental and mechanistic understanding of these materials through proper characterisation. X-Ray or neutron total scattering experiments can be performed to yield the total scattering patterns, which feature information on both Bragg and diffuse scattering. Pair distribution function (PDF) can be calculated from the X-ray diffusion pattern which describes the degree of local and intermediate ordering.36 In addition, solid-state nuclear magnetic resonance (SSNMR) can also provide information on the local structure and chemical bonding within amorphous MOFs. Furthermore, Fourier-transform infrared spectroscopy (FTIR) can provide additional information about the chemical bonding within MOFs, while Terahertz and Far-IR Spectroscopy (THz-FIR) can be used to further probe the metal-linker vibrations in the Thz region.60,104 Another approach is to push characterisation to near or at atomic resolution. High-resolution transmission electron microscopy (TEM) and scanning TEM (STEM) imaging can be used to probe the interface, metallic centres and organic linkers. In addition, scanning electron diffraction (SED) can also be a powerful tool to characterise the regions of crystallinity/non-crystallinity in a MOF composite sample.58,60,106
At the moment, predicting the band structure of amorphous MOFs is a challenging task due to the disordered nature and large unit cell of these materials. The introduction of amorphous MOF composites further complicates computational calculations. As a result, there are practical limitations in terms of available computational methods and resources for accurately predicting the band structures of these materials.33 Despite these challenges, ongoing research and advancements in computational methods hold the room for addressing these limitations and expanding our ability to predict the band structures of MOFs. Further development and refinement of computational algorithms, as well as improvements in computing power, will likely contribute to overcoming the current obstacles and enable more accurate predictions of the band structures of amorphous MOF composites.
Scalability and industrialization
Scalability is a crucial factor to consider in materials research, particularly when aiming for industrial-scale applications. The utilization of crystalline MOFs in such applications is often hindered by their relatively high cost and negative environmental impact resulting from the use of organic solvents during synthesis.107 Even though mechanochemical synthesis shows promise in addressing these issues, the lack of comprehensive understanding in mechanochemistry often leads to partial amorphization of the samples. In contrast, the growing interest in amorphous MOFs offers a viable solution that completely circumvents the aforementioned challenges associated with mechanochemical milling. The shift in interest towards amorphous MOFs can effectively avoid these issues in mechanochemical milling. It is expected that the scale up of amorphous MOF materials is more easily achievable.
In order to practically use MOFs in the industry, scientists need to find a way of transferring MOF microcrystals into macroscopically shaped bodies which can fit the applications.108 However, processibility is a major issue of crystalline MOFs that becomes a bottleneck towards industrialization.109 In order to circumvent the issue of processability, one emerging group of amorphous MOFs called MOF glasses might be the solution. MOF glasses with an accessible liquid state upon heat treatment have much higher processability compared to their crystalline counterparts, which enables them to be easily shaped into different device configurations (Fig. 6).
Conflicts of interest
The authors declare no conflict of interest.
Acknowledgements
W. W. would like to thank Australian government and the University of Queensland for the Graduation School Scholarship support. J. H. acknowledges the financial support from the Australian Research Council (FT210100589) and the University of Queensland (2021002771). V. C. acknowledges funding by the Australian Government through the Australian Research Council through its Discovery Grant Program (DP180103874).
Notes and references
- O. M. Yaghi and H. Li, Hydrothermal Synthesis of a Metal–Organic Framework Containing Large Rectangular Channels, J. Am. Chem. Soc., 1995, 117(41), 10401–10402 CrossRef CAS
.
- N. Masciocchi, S. Bruni, E. Cariati, F. Cariati, S. Galli and A. Sironi, Extended Polymorphism in Copper(II) Imidazolate Polymers:
A Spectroscopic and XRPD Structural Study, Inorg. Chem., 2001, 40(23), 5897–5905 CrossRef CAS PubMed
.
- K. W. Chapman, G. J. Halder and P. J. Chupas, Pressure-Induced Amorphization and Porosity Modification in a Metal−Organic Framework, J. Am. Chem. Soc., 2009, 131(48), 17546–17547 CrossRef CAS PubMed
.
- T. D. Bennett, S. Cao, J. C. Tan, D. A. Keen, E. G. Bithell, P. J. Beldon, T. Friscic and A. K. Cheetham, Facile Mechanosynthesis of Amorphous Zeolitic Imidazolate Frameworks, J. Am. Chem. Soc., 2011, 133(37), 14546–14549 CrossRef CAS PubMed
.
- T. D. Bennett, D. A. Keen, J.-C. Tan, E. R. Barney, A. L. Goodwin and A. K. Cheetham, Thermal Amorphization of Zeolitic Imidazolate Frameworks, Angew. Chem., Int. Ed., 2011, 50(13), 3067–3071 CrossRef CAS PubMed
.
- F. Yang, W. Li and B. Tang, Facile synthesis of amorphous UiO-66 (Zr-MOF) for supercapacitor application, J. Alloys Compd., 2018, 733, 8–14 CrossRef CAS
.
- T. D. Bennett, J.-C. Tan, Y. Yue, E. Baxter, C. Ducati, N. J. Terrill, H. H. M. Yeung, Z. Zhou, W. Chen, S. Henke, A. K. Cheetham and G. N. Greaves, Hybrid glasses from strong and fragile metal-organic framework liquids, Nat. Commun., 2015, 6(1), 8079 CrossRef CAS PubMed
.
- D. Umeyama, S. Horike, M. Inukai, T. Itakura and S. Kitagawa, Reversible Solid-to-Liquid Phase Transition of Coordination Polymer Crystals, J. Am. Chem. Soc., 2015, 137(2), 864–870 CrossRef CAS PubMed
.
- C. Gao, Z. Jiang, S. Qi, P. Wang, L. R. Jensen, M. Johansen, C. K. Christensen, Y. Zhang, D. B. Ravnsbæk and Y. Yue, Metal-Organic Framework Glass Anode with an Exceptional Cycling-Induced Capacity Enhancement for Lithium-Ion Batteries, Adv. Mater., 2022, 34(10), 2110048 CrossRef CAS PubMed
.
- X. Li, F. Cheng, S. Zhang and J. Chen, Shape-controlled synthesis and lithium-storage study of metal-organic frameworks Zn4O(1,3,5-benzenetribenzoate)2, J. Power Sources, 2006, 160(1), 542–547 CrossRef CAS
.
- G. Férey, F. Millange, M. Morcrette, C. Serre, M.-L. Doublet, J.-M. Grenèche and J.-M. Tarascon, Mixed-Valence Li/Fe-Based Metal–Organic Frameworks with Both Reversible Redox and Sorption Properties, Angew. Chem., Int. Ed., 2007, 46(18), 3259–3263 CrossRef PubMed
.
- T. D. Bennett and S. Horike, Liquid, glass and amorphous solid states of coordination polymers and metal–organic frameworks. Nature Reviews, Materials, 2018, 3(11), 431–440 Search PubMed
.
- J. Fonseca, T. Gong, L. Jiao and H.-L. Jiang, Metal–organic frameworks (MOFs) beyond crystallinity: amorphous MOFs, MOF liquids and MOF glasses, J. Mater. Chem. A, 2021, 9(17), 10562–10611 RSC
.
- R. S. K. Madsen, A. Qiao, J. Sen, I. Hung, K. Chen, Z. Gan, S. Sen and Y. Yue, Ultrahigh-field 67Zn NMR reveals short-range disorder in zeolitic imidazolate framework glasses, Science, 2020, 367(6485), 1473–1476 CrossRef CAS PubMed
.
- S. Tominaka, H. Hamoudi, T. Suga, T. D. Bennett, A. B. Cairns and A. K. Cheetham, Topochemical conversion of a dense metal–organic framework from a crystalline insulator to an amorphous semiconductor, Chem. Sci., 2015, 6(2), 1465–1473 RSC
.
- V. Nozari, C. Calahoo, J. M. Tuffnell, P. Adelhelm, K. Wondraczek, S. E. Dutton, T. D. Bennett and L. Wondraczek, Sodium Ion Conductivity in Superionic IL-Impregnated Metal-Organic Frameworks: Enhancing Stability Through Structural Disorder, Sci. Rep., 2020, 10(1), 3532 CrossRef CAS PubMed
.
- C. Gao, P. Wang, Z. Wang, S. K. Kær, Y. Zhang and Y. Yue, The disordering-enhanced performances of the Al-MOF/graphene composite anodes for lithium ion batteries, Nano Energy, 2019, 65, 104032 CrossRef CAS
.
- C. Lin, W. Zhao, X. Yan, B. Liu, X. Fang, J. Wang, N. Xia and J. Tian, Fishnet-like superstructures constructed from ultrafine and ultralong Ni-MOF nanowire arrays directionally grown on highly rough and conductive scaffolds: synergistic activating effect for efficient and robust alkaline water oxidation activity, Appl. Surf. Sci., 2020, 529, 147030 CrossRef CAS
.
- T. Li, Y. Bai, Y. Wang, H. Xu and H. Jin, Advances in transition-metal (Zn, Mn, Cu)-based MOFs and their derivatives for anode of lithium-ion batteries, Coord. Chem. Rev., 2020, 410, 213221 CrossRef CAS
.
- G. Xu, C. Zhu and G. Gao, Recent Progress of Advanced Conductive Metal–Organic Frameworks: Precise Synthesis, Electrochemical Energy Storage Applications, and Future Challenges, Small, 2022, 18(44), 2203140 CrossRef CAS PubMed
.
- G. Khandelwal, N. P. Maria Joseph Raj and S.-J. Kim, ZIF-62: a mixed linker metal–organic framework for triboelectric nanogenerators, J. Mater. Chem. A, 2020, 8(34), 17817–17825 RSC
.
- S. C. Moore, M. R. Smith, J. L. Trettin, R. A. Yang and M. L. Sarazen, Kinetic Impacts of Defect Sites in Metal–Organic Framework Catalysts under Varied Driving Forces, ACS Energy Lett., 2023, 8(3), 1397–1407 CrossRef CAS
.
- S. Gulati, S. Vijayan, Mansi, S. Kumar, B. Harikumar, M. Trivedi and R. S. Varma, Recent advances in the application of metal-organic frameworks (MOFs)-based nanocatalysts for direct conversion of carbon dioxide (CO2) to value-added chemicals, Coord. Chem. Rev., 2023, 474, 214853 CrossRef CAS
.
- R.-B. Lin, S. Xiang, H. Xing, W. Zhou and B. Chen, Exploration of porous metal–organic frameworks for gas separation and purification, Coord. Chem. Rev., 2019, 378, 87–103 CrossRef CAS
.
- A. Ebadi Amooghin, H. Sanaeepur, R. Luque, H. Garcia and B. Chen, Fluorinated metal–organic frameworks for gas separation, Chem. Soc. Rev., 2022, 51(17), 7427–7508 RSC
.
- W. Gong, Y. Xie, T. D. Pham, S. Shetty, F. A. Son, K. B. Idrees, Z. Chen, H. Xie, Y. Liu, R. Q. Snurr, B. Chen, B. Alameddine, Y. Cui and O. K. Farha, Creating Optimal Pockets in a Clathrochelate-Based Metal–Organic Framework for Gas Adsorption and Separation: Experimental and Computational Studies, J. Am. Chem. Soc., 2022, 144(8), 3737–3745 CrossRef CAS PubMed
.
- C. Jiang, X. Wang, Y. Ouyang, K. Lu, W. Jiang, H. Xu, X. Wei, Z. Wang, F. Dai and D. Sun, Recent advances in metal–organic frameworks for gas adsorption/separation, Nanoscale Adv., 2022, 4(9), 2077–2089 RSC
.
- N. Ogihara, M. Hasegawa, H. Kumagai, R. Mikita and N. Nagasako, Heterogeneous intercalated metal-organic framework active materials for fast-charging non-aqueous Li-ion capacitors, Nat. Commun., 2023, 14(1), 1472 CrossRef CAS PubMed
.
- B. He, Q. Zhang, Z. Pan, L. Li, C. Li, Y. Ling, Z. Wang, M. Chen, Z. Wang, Y. Yao, Q. Li, L. Sun, J. Wang and L. Wei, Freestanding Metal–Organic Frameworks and Their Derivatives: An Emerging Platform for Electrochemical Energy Storage and Conversion, Chem. Rev., 2022, 122(11), 10087–10125 CrossRef CAS PubMed
.
- R. K. Tripathy, A. K. Samantara and J. N. Behera, Metal–organic framework (MOF)-derived amorphous nickel boride: an electroactive material for electrochemical energy conversion and storage application. Sustainable, Energy Fuels, 2021, 5(4), 1184–1193 CAS
.
- M. Ko, A. Aykanat, M. K. Smith and K. A. Mirica, Drawing Sensors with Ball-Milled Blends of Metal-Organic Frameworks and Graphite, Sensors, 2017, 17(10), 2192 CrossRef PubMed
.
- C. Li, L. Zhang, J. Chen, X. Li, J. Sun, J. Zhu, X. Wang and Y. Fu, Recent development and applications of electrical conductive MOFs, Nanoscale, 2021, 13(2), 485–509 RSC
.
- L. S. Xie, G. Skorupskii and M. Dincă, Electrically Conductive Metal–Organic Frameworks, Chem. Rev., 2020, 120(16), 8536–8580 CrossRef CAS PubMed
.
- Y. Park, H. Choi, M.-C. Kim, N. A. T. Tran, Y. Cho, J. I. Sohn, J. Hong and Y.-W. Lee, Effect of ionic conductivity in polymer-gel electrolytes containing iodine-based redox mediators for efficient, flexible energy storage systems, J. Ind. Eng. Chem., 2021, 94, 384–389 CrossRef CAS
.
- H. Tan, Y. Zhou, S.-Z. Qiao and H. J. Fan, Metal organic framework (MOF) in aqueous energy devices, Mater. Today, 2021, 48, 270–284 CrossRef CAS
.
- T. D. Bennett and A. K. Cheetham, Amorphous Metal–Organic Frameworks, Acc. Chem. Res., 2014, 47(5), 1555–1562 CrossRef CAS PubMed
.
- J. Zhang, Y. Li, Z. Chen, Q. Liu, Q. Chen and M. Chen, Amorphous Electrode: From Synthesis to Electrochemical Energy Storage, Energy Environ. Mater., 2023, e12573 CrossRef
.
- X. Zhang, G. Li, Y. Zhang, D. Luo, A. Yu, X. Wang and Z. Chen, Amorphizing metal-organic framework towards multifunctional polysulfide barrier for high-performance lithium-sulfur batteries, Nano Energy, 2021, 86, 106094 CrossRef CAS
.
- Z. Liang, C. Qu, W. Guo, R. Zou and Q. Xu, Pristine Metal–Organic Frameworks and their Composites for Energy Storage and Conversion, Adv. Mater., 2018, 30(37), 1702891 CrossRef PubMed
.
- H. Wang, Q. Yang, N. Zheng, X. Zhai, T. Xu, Z. Sun, L. Wu and M. Zhou, Roadmap of amorphous metal-organic framework for electrochemical energy conversion and storage, Nano Res., 2023, 16(3), 4107–4118 CrossRef CAS
.
-
S. Tanaka, Mechanochemical synthesis of MOFs, in Metal–Organic Frameworks for Biomedical Applications, ed. M. Mozafari, Woodhead Publishing, 2020, ch. 10, pp. 197–222 Search PubMed
.
- W. Wang, M. Chai, M. Y. Bin Zulkifli, K. Xu, Y. Chen, L. Wang, V. Chen and J. Hou, Metal–organic framework composites from a mechanochemical process, Mol. Syst. Des. Eng., 2023, 5, 560–579 RSC
.
- Z. Su, Y.-R. Miao, S.-M. Mao, G.-H. Zhang, S. Dillon, J. T. Miller and K. S. Suslick, Compression-Induced Deformation of Individual Metal–Organic Framework Microcrystals, J. Am. Chem. Soc., 2015, 137(5), 1750–1753 CrossRef CAS PubMed
.
- N. Ma and S. Horike, Metal–Organic Network-Forming Glasses, Chem. Rev., 2022, 122(3), 4163–4203 CrossRef CAS PubMed
.
- S. Conrad, P. Kumar, F. Xue, L. Ren, S. Henning, C. Xiao, K. A. Mkhoyan and M. Tsapatsis, Controlling Dissolution and Transformation of Zeolitic Imidazolate Frameworks by using Electron-Beam-Induced Amorphization, Angew. Chem., Int. Ed., 2018, 57(41), 13592–13597 CrossRef CAS PubMed
.
- Z. Lin, J. J. Richardson, J. Zhou and F. Caruso, Direct synthesis of amorphous coordination polymers and metal–organic frameworks, Nat. Rev. Chem., 2023, 7(4), 273–286 CrossRef CAS PubMed
.
- C. Wang, Z. Di, Z. Xiang, J. Zhao and L. Li, Coordination-driven assembly of proteins and nucleic acids in a single architecture for carrier-free intracellular co-delivery, Nano Today, 2021, 38, 101140 CrossRef CAS
.
- A. Lopez and J. Liu, Self-Assembly of Nucleobase, Nucleoside and Nucleotide Coordination Polymers: From Synthesis to Applications, ChemNanoMat, 2017, 3(10), 670–684 CrossRef CAS
.
- M. A. Rahim, K. Kempe, M. Müllner, H. Ejima, Y. Ju, M. P. van Koeverden, T. Suma, J. A. Braunger, M. G. Leeming, B. F. Abrahams and F. Caruso, Surface-Confined Amorphous Films from Metal-Coordinated Simple Phenolic Ligands, Chem. Mater., 2015, 27(16), 5825–5832 CrossRef CAS
.
- Z. Lin, J. Zhou, C. Cortez-Jugo, Y. Han, Y. Ma, S. Pan, E. Hanssen, J. J. Richardson and F. Caruso, Ordered Mesoporous Metal–Phenolic Network Particles, J. Am. Chem. Soc., 2020, 142(1), 335–341 CrossRef CAS PubMed
.
- B. Li and H. C. Zeng, Synthetic Chemistry and Multifunctionality of an Amorphous Ni-MOF-74 Shell on a Ni/SiO2 Hollow Catalyst for Efficient Tandem Reactions, Chem. Mater., 2019, 31(14), 5320–5330 CrossRef CAS
.
- X. Gong, K. Gnanasekaran, K. Ma, C. J. Forman, X. Wang, S. Su, O. K. Farha and N. C. Gianneschi, Rapid Generation of Metal–Organic Framework Phase Diagrams by High-Throughput Transmission Electron Microscopy, J. Am. Chem. Soc., 2022, 144(15), 6674–6680 CrossRef CAS PubMed
.
- Q. Zheng, Y. Zhang, M. Montazerian, O. Gulbiten, J. C. Mauro, E. D. Zanotto and Y. Yue, Understanding Glass through Differential Scanning Calorimetry, Chem. Rev., 2019, 119(13), 7848–7939 CrossRef CAS PubMed
.
- Y. Zhao, Y. Song, Z. Hu, W. Wang, Z. Chang, Y. Zhang, Q. Lu, H. Wu, J. Liao, W. Zou, X. Gao, K. Jia, L. Zhuo, J. Hu, Q. Xie, R. Zhang, X. Wang, L. Sun, F. Li, L. Zheng, M. Wang, J. Yang, B. Mao, T. Fang, F. Wang, H. Zhong, W. Liu, R. Yan, J. Yin, Y. Zhang, Y. Wei, H. Peng, L. Lin and Z. Liu, Large-area transfer of two-dimensional materials free of cracks, contamination and wrinkles via controllable conformal contact, Nat. Commun., 2022, 13(1), 4409 CrossRef CAS PubMed
.
- A. Qiao, T. D. Bennett, H. Tao, A. Krajnc, G. Mali, C. M. Doherty, A. W. Thornton, J. C. Mauro, G. N. Greaves and Y. Yue, A metal-organic framework with ultrahigh glass-forming ability, Sci. Adv., 2018, 4(3), eaao6827 CrossRef PubMed
.
- T. D. Bennett, T. K. Todorova, E. F. Baxter, D. G. Reid, C. Gervais, B. Bueken, B. Van de Voorde, D. De Vos, D. A. Keen and C. Mellot-Draznieks, Connecting defects and amorphization in UiO-66 and MIL-140 metal–organic frameworks: a combined experimental and computational study, Phys. Chem. Chem. Phys., 2016, 18(3), 2192–2201 RSC
.
- C. Liu, J. Wang, J. Wan, Y. Cheng, R. Huang, C. Zhang, W. Hu, G. Wei and C. Yu, Amorphous Metal–Organic Framework-Dominated Nanocomposites with Both Compositional and Structural Heterogeneity for Oxygen Evolution, Angew. Chem., Int. Ed., 2020, 59(9), 3630–3637 CrossRef CAS PubMed
.
- J. Hou, C. W. Ashling, S. M. Collins, A. Krajnc, C. Zhou, L. Longley, D. N. Johnstone, P. A. Chater, S. Li, M.-V. Coulet, P. L. Llewellyn, F.-X. Coudert, D. A. Keen, P. A. Midgley, G. Mali, V. Chen and T. D. Bennett, Metal-organic framework crystal-glass composites, Nat. Commun., 2019, 10(1), 2580 CrossRef PubMed
.
- R. Lin, X. Li, A. Krajnc, Z. Li, M. Li, W. Wang, L. Zhuang, S. Smart, Z. Zhu, D. Appadoo, J. R. Harmer, Z. Wang, A. G. Buzanich, S. Beyer, L. Wang, G. Mali, T. D. Bennett, V. Chen and J. Hou, Mechanochemically Synthesised Flexible Electrodes Based on Bimetallic Metal–Organic Framework Glasses for the Oxygen Evolution Reaction, Angew. Chem., Int. Ed., 2022, 61(4), e202112880 CrossRef CAS PubMed
.
- J. Hou, P. Chen, A. Shukla, A. Krajnc, T. Wang, X. Li, R. Doasa, L. H. G. Tizei, B. Chan, D. N. Johnstone, R. Lin, T. U. Schülli, I. Martens, D. Appadoo, M. S. Ari, Z. Wang, T. Wei, S.-C. Lo, M. Lu, S. Li, E. B. Namdas, G. Mali, A. K. Cheetham, S. M. Collins, V. Chen, L. Wang and T. D. Bennett, Liquid-phase sintering of lead halide perovskites and metal-organic framework glasses, Science, 2021, 374(6567), 621–625 CrossRef CAS PubMed
.
- B. Weidenfeller, H. Rode, L. Weidenfeller and K. Weidenfeller, Crystallinity, thermal diffusivity, and electrical conductivity of carbon black filled polyamide 46, J. Appl. Polym. Sci., 2020, 137(29), 48882 CrossRef CAS
.
- M. Flygare and K. Svensson, Influence of crystallinity on the electrical conductivity of individual carbon nanotubes, Carbon Trends, 2021, 5, 100125 CrossRef CAS
.
- Y. Guo, Y. Wei, H. Li and T. Zhai, Layer Structured Materials for Advanced Energy Storage and Conversion, Small, 2017, 13(45), 1701649 CrossRef PubMed
.
- X. Cui, L. Zhang, J. Zhang, L. Gong, M. Gao, P. Zheng, L. Xiang, W. Wang, W. Hu, Q. Xu, W. Wei and H. Zeng, A novel metal-organic layered material with superior supercapacitive performance through ultrafast and reversible tetraethylammonium intercalation, Nano Energy, 2019, 59, 102–109 CrossRef CAS
.
- J.-W. Xiu, G.-E. Wang, M.-S. Yao, C.-C. Yang, C.-H. Lin and G. Xu, Electrical bistability in a metal–organic framework modulated by reversible crystalline-to-amorphous transformations, Chem. Commun., 2017, 53(16), 2479–2482 RSC
.
- M. Tatsumisago, Glassy materials based on Li2S for all-solid-state lithium secondary batteries, Solid State Ionics, 2004, 175(1), 13–18 CrossRef CAS
.
- A. Chandra, A. Bhatt and A. Chandra, Ion Conduction in Superionic Glassy Electrolytes: An Overview, J. Mater. Sci. Technol., 2013, 29(3), 193–208 CrossRef CAS
.
- T. Minami, Fast ion conducting glasses, J. Non-Cryst. Solids, 1985, 73(1), 273–284 CrossRef CAS
.
- D. Umeyama, S. Horike, M. Inukai and S. Kitagawa, Integration of Intrinsic Proton Conduction and Guest-Accessible Nanospace into a Coordination Polymer, J. Am. Chem. Soc., 2013, 135(30), 11345–11350 CrossRef CAS PubMed
.
- M. Wang, H. Zhao, B. Du, X. Lu, S. Ding and X. Hu, Functions and applications of emerging metal–organic-framework liquids and glasses, Chem. Commun., 2023, 47, 7126–7140 RSC
.
- M.-H. Zeng, Short-range disorder in MOF glasses, Natl. Sci. Rev., 2020, 8(5), nwaa207 CrossRef PubMed
.
- L. Majidi, A. Ahmadiparidari, N. Shan, S. Kumar Singh, C. Zhang, Z. Huang, S. Rastegar, K. Kumar, Z. Hemmat, A. T. Ngo, P. Zapol, J. Cabana, A. Subramanian, L. A. Curtiss and A. Salehi-Khojin, Nanostructured Conductive Metal Organic Frameworks for Sustainable Low Charge Overpotentials in Li–Air Batteries, Small, 2022, 18(4), 2102902 CrossRef CAS PubMed
.
- M. Rana, H. A. Al-Fayaad, B. Luo, T. Lin, L. Ran, J. K. Clegg, I. Gentle and R. Knibbe, Oriented nanoporous MOFs to mitigate polysulfides migration in lithium-sulfur batteries, Nano Energy, 2020, 75, 105009 CrossRef CAS
.
- Z. Ye, Y. Jiang, L. Li, F. Wu and R. Chen, Rational Design of MOF-Based Materials for Next-Generation Rechargeable Batteries, Nano-Micro Lett., 2021, 13(1), 203 CrossRef CAS PubMed
.
- H. Zhang, W. Zhao, M. Zou, Y. Wang, Y. Chen, L. Xu, H. Wu and A. Cao, 3D, Mutually Embedded MOF@Carbon Nanotube Hybrid Networks for High-Performance Lithium-Sulfur Batteries. Advanced Energy, Materials, 2018, 8(19), 1800013 Search PubMed
.
- S. Zheng, X. Li, B. Yan, Q. Hu, Y. Xu, X. Xiao, H. Xue and H. Pang, Transition-Metal (Fe, Co, Ni) Based Metal-Organic Frameworks for Electrochemical Energy Storage. Advanced Energy, Materials, 2017, 7(18), 1602733 Search PubMed
.
- C. Xu, L. Liu, C. Wu and K. Wu, Unique 3D heterostructures assembled by quasi-2D Ni-MOF and CNTs for ultrasensitive electrochemical sensing of bisphenol A, Sens. Actuators, B, 2020, 310, 127885 CrossRef CAS
.
- A. E. Baumann, J. R. Downing, D. A. Burns, M. C. Hersam and V. S. Thoi, Graphene–Metal–Organic Framework Composite Sulfur Electrodes for Li–S Batteries with High Volumetric Capacity, ACS Appl. Mater. Interfaces, 2020, 12(33), 37173–37181 CrossRef CAS PubMed
.
- T. T. Tung, M. T. Tran, J.-F. Feller, M. Castro, T. Van Ngo, K. Hassan, M. J. Nine and D. Losic, Graphene and metal organic frameworks (MOFs) hybridization for tunable chemoresistive sensors for detection of volatile organic compounds (VOCs) biomarkers, Carbon, 2020, 159, 333–344 CrossRef CAS
.
- S. Li, C. Shi, Y. Pan and Y. Wang, 2D/2D NiCo-MOFs/GO hybrid nanosheets for high-performance asymmetrical supercapacitor, Diamond Relat. Mater., 2021, 115, 108358 CrossRef CAS
.
- Y. Han, Z. Liu, F. Zheng, Y. Bai, Z. Zhang, X. Li, W. Xiong, J. Zhang and A. Yuan, Two-dimensional flower-like cobalt-porphyrin MOF/rGO composite anodes for high-performance Li-ion batteries, J. Alloys Compd., 2021, 881, 160531 CrossRef CAS
.
- F. Tang, J. Gao, Q. Ruan, X. Wu, X. Wu, T. Zhang, Z. Liu, Y. Xiang, Z. He and X. Wu, Graphene-Wrapped MnO/C Composites by MOFs-Derived as Cathode Material for Aqueous Zinc ion Batteries, Electrochim. Acta, 2020, 353, 136570 CrossRef CAS
.
- C. Meng, P. Hu, H. Chen, Y. Cai, H. Zhou, Z. Jiang, X. Zhu, Z. Liu, C. Wang and A. Yuan, 2D conductive MOFs with sufficient redox sites: reduced graphene oxide/Cu-benzenehexathiolate composites as high capacity anode materials for lithium-ion batteries, Nanoscale, 2021, 13(16), 7751–7760 RSC
.
- N. Patterson, B. Xiao and A. Ignaszak, Polypyrrole decorated metal–organic frameworks for supercapacitor devices, RSC Adv., 2020, 10(34), 20162–20172 RSC
.
- Z. Xin, J. Liu, X. Wang, K. Shen, Z. Yuan, Y. Chen and Y.-Q. Lan, Implanting Polypyrrole in Metal-Porphyrin MOFs: Enhanced Electrocatalytic Performance for CO2RR, ACS Appl. Mater. Interfaces, 2021, 13(46), 54959–54966 CrossRef CAS PubMed
.
- F. Sun, Q. Li, H. Xue and H. Pang, Pristine Transition-Metal-Based Metal-Organic Frameworks for Electrocatalysis, ChemElectroChem, 2019, 6(5), 1273–1299 CrossRef CAS
.
- J. Meng, X. Liu, C. Niu, Q. Pang, J. Li, F. Liu, Z. Liu and L. Mai, Advances in metal–organic framework coatings: versatile synthesis and broad applications, Chem. Soc. Rev., 2020, 49(10), 3142–3186 RSC
.
- Q.-Q. Qiao, G.-R. Li, Y.-L. Wang and X.-P. Gao, To enhance the capacity of Li-rich layered oxides by surface modification with metal–organic frameworks (MOFs) as cathodes for advanced lithium-ion batteries, J. Mater. Chem. A, 2016, 4(12), 4440–4447 RSC
.
- M. Zhao, Q. Zhao, J. Qiu, X. Lu, G. Zhang, H. Xue and H. Pang, Amorphous Cobalt Coordination Nanolayers Incorporated with Silver Nanowires: A New Electrode Material for Supercapacitors, Part. Part. Syst. Charact., 2017, 34(11), 1600412 CrossRef
.
- Q.-L. Zhu and Q. Xu, Metal–organic framework composites, Chem. Soc. Rev., 2014, 43(16), 5468–5512 RSC
.
- R. Semino, J. C. Moreton, N. A. Ramsahye, S. M. Cohen and G. Maurin, Understanding the origins of metal–organic framework/polymer compatibility, Chem. Sci., 2018, 9(2), 315–324 RSC
.
- Y. García, L. Porcarelli, H. Zhu, M. Forsyth, D. Mecerreyes and L. A. O'Dell, Probing disorder and dynamics in composite electrolytes of an organic ionic plastic crystal and lithium functionalised acrylic polymer nanoparticles, J. Magn. Reson. Open, 2023, 14–15, 100095 CrossRef
.
- L. Longley, C. Calahoo, R. Limbach, Y. Xia, J. M. Tuffnell, A. F. Sapnik, M. F. Thorne, D. S. Keeble, D. A. Keen, L. Wondraczek and T. D. Bennett, Metal-organic framework and inorganic glass composites, Nat. Commun., 2020, 11(1), 5800 CrossRef CAS PubMed
.
- R. Gaillac, P. Pullumbi, K. A. Beyer, K. W. Chapman, D. A. Keen, T. D. Bennett and F.-X. Coudert, Liquid metal–organic frameworks, Nat. Mater., 2017, 16(11), 1149–1154 CrossRef CAS PubMed
.
- V. Nozari, C. Calahoo, J. M. Tuffnell, D. A. Keen, T. D. Bennett and L. Wondraczek, Ionic liquid facilitated melting of the metal-organic framework ZIF-8, Nat. Commun., 2021, 12(1), 5703 CrossRef CAS PubMed
.
- V. Nozari, O. Smirnova, J. M. Tuffnell, A. Knebel, T. D. Bennett and L. Wondraczek, Low-Temperature Melting and Glass Formation of the Zeolitic Imidazolate Frameworks ZIF-62 and ZIF-76 through Ionic Liquid Incorporation, Adv. Mater. Technol., 2022, 7(11), 2200343 CrossRef CAS
.
- R. N. Widmer, G. I. Lampronti, S. Anzellini, R. Gaillac, S. Farsang, C. Zhou, A. M. Belenguer, C. W. Wilson, H. Palmer, A. K. Kleppe, M. T. Wharmby, X. Yu, S. M. Cohen, S. G. Telfer, S. A. T. Redfern, F.-X. Coudert, S. G. MacLeod and T. D. Bennett, Pressure promoted low-temperature melting of metal–organic frameworks, Nat. Mater., 2019, 18(4), 370–376 CrossRef CAS PubMed
.
- J. Yan, C. Gao, S. Qi, Z. Jiang, L. R. Jensen, H. Zhan, Y. Zhang and Y. Yue, Encapsulation of nano-Si into MOF glass to enhance lithium-ion battery anode performances, Nano Energy, 2022, 103, 107779 CrossRef CAS
.
- J. Joseph, S. Iftekhar, V. Srivastava, Z. Fallah, E. N. Zare and M. Sillanpää, Iron-based metal-organic framework: Synthesis, structure and current technologies for water reclamation with deep insight into framework integrity, Chemosphere, 2021, 284, 131171 CrossRef CAS PubMed
.
- V. Nozari, C. Calahoo, L. Longley, T. D. Bennett and L. Wondraczek, Structural integrity, meltability, and variability of thermal properties in the mixed-linker zeolitic imidazolate framework ZIF-62. The, J. Chem. Phys., 2020, 153(20), 204501 CrossRef CAS PubMed
.
- I. Bechis, A. F. Sapnik, A. Tarzia, E. H. Wolpert, M. A. Addicoat, D. A. Keen, T. D. Bennett and K. E. Jelfs, Modeling the Effect of Defects and Disorder in Amorphous Metal–Organic Frameworks, Chem. Mater., 2022, 34(20), 9042–9054 CrossRef CAS PubMed
.
- Y. Wu, D. Li, C.-L. Wu, H. Y. Hwang and Y. Cui, Electrostatic gating and intercalation in 2D materials. Nature Reviews, Materials, 2023, 8(1), 41–53 Search PubMed
.
- P. Yan, L. Ji, X. Liu, Q. Guan, J. Guo, Y. Shen, H. Zhang, W. Wei, X. Cui and Q. Xu, 2D amorphous-MoO3−x@Ti3C2-MXene non-van der Waals heterostructures as anode materials for lithium-ion batteries, Nano Energy, 2021, 86, 106139 CrossRef CAS
.
- J. Hou, M. L. Ríos Gómez, A. Krajnc, A. McCaul, S. Li, A. M. Bumstead, A. F. Sapnik, Z. Deng, R. Lin, P. A. Chater, D. S. Keeble, D. A. Keen, D. Appadoo, B. Chan, V. Chen, G. Mali and T. D. Bennett, Halogenated Metal–Organic Framework Glasses and Liquids, J. Am. Chem. Soc., 2020, 142(8), 3880–3890 CrossRef CAS PubMed
.
- N. K. Kulachenkov, S. Bruyere, S. A. Sapchenko, Y. A. Mezenov, D. Sun, A. A. Krasilin, A. Nominé, J. Ghanbaja, T. Belmonte, V. P. Fedin, E. A. Pidko and V. A. Milichko, Ultrafast Melting of Metal–Organic Frameworks for Advanced Nanophotonics, Adv. Funct. Mater., 2020, 30(7), 1908292 CrossRef CAS
.
- A. F. Sapnik, C. Sun, J. E. M. Laulainen, D. N. Johnstone, R. Brydson, T. Johnson, P. A. Midgley, T. D. Bennett and S. M. Collins, Mapping nanocrystalline disorder within an amorphous metal–organic framework, Commun. Chem., 2023, 6(1), 92 CrossRef CAS PubMed
.
-
D. Ozer, Mechanochemistry: A Power Tool for Green Synthesis, in Advances in Green Synthesis: Avenues and Sustainability, Inamuddin, ed. R. Boddula, M. I. Ahamed, A. Khan, Springer International Publishing, Cham, 2021, pp. 23–39 Search PubMed
.
- Q. Ma, T. Zhang and B. Wang, Shaping of metal-organic frameworks, a critical step toward industrial applications, Matter, 2022, 5(4), 1070–1091 CrossRef CAS
.
- P. Cheng, C. Wang, Y. V. Kaneti, M. Eguchi, J. Lin, Y. Yamauchi and J. Na, Practical MOF Nanoarchitectonics: New Strategies for Enhancing the Processability of MOFs for Practical Applications, Langmuir, 2020, 36(16), 4231–4249 CrossRef CAS PubMed
.
|
This journal is © The Royal Society of Chemistry 2023 |
Click here to see how this site uses Cookies. View our privacy policy here.