DOI:
10.1039/D2VA00236A
(Critical Review)
Environ. Sci.: Adv., 2023,
2, 409-423
Prospects of carbon capture, utilization and storage for mitigating climate change
Received
1st October 2022
, Accepted 19th December 2022
First published on 12th January 2023
Abstract
Carbon capture (i.e., CO2 capture) and storage (CCS) and carbon capture and utilization (CCU) are recognized as potential pathways to combat global climate change. Numerous efforts are underway, such as CCS (e.g., biochar used for soil amendment; captured carbon injected into onshore or offshore reservoirs) and CCU (captured CO2 used for crop/algae production), due to enormous societal, economic and environmental concerns on climate change and for complying with emission regulations as well as meeting the committed emission reduction targets. This study compiled information on greenhouse gas (GHG) emission reduction initiatives, including CCS and CCU on the earth, recent technological advances in CCS and CCU, and their economic, environmental and societal implications. The prospect of CCS and CCU technologies has also been discussed. CCS processes require less energy compared with CCU and thus tend to be more cost-effective than CCU. The global warming potential (GWP) and the cost of energy systems that deployed CCS/CCU varied from 0.007–0.225 kg CO2 eq per MJ and $0.017–0.070 MJ−1, respectively. Both CCS and CCU have challenges, and public perception of these initiatives seems to be complex or not satisfactory for deploying large-scale projects, which hinders meeting the GHG emission reduction targets of different jurisdictions. The acceptance of CCU seems to be higher compared to that of CCS because of the concerns about land-use complexities. For a carbon-neutral economy, a single transition pathway appears to be inadequate. Stringent policies, financial incentives/benefits, stakeholder participation and technological advances would be crucial for the transition; however, technological advances and policy initiatives must be justified by a broader sustainability check to avoid the risk of investment and climate change.
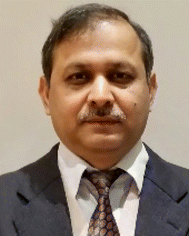 Poritosh Roy | Dr Poritosh Roy is an Agricultural Engineer (P. Eng). His areas of research interest are value-added product development from biomass (thermochemical and biochemical conversion, and co-processing), bio-based economy, bioprocessing & bioreactor design, food processing, industrial symbiosis, life cycle assessment and life cycle costing of agri-food industries. He has authored/co-authored 60 peer-reviewed research articles and six book chapters. He served as a Guest Editor for special issues of Sustainability (MDPI) and AIMS Energy (AIMS Press). He is an Editorial Board Member of AIMS Energy and the Journal of Food Science and Nutrition Therapy. Presently, Dr Roy is working as a Sessional Lecturer and Special Graduate Faculty at the School of Engineering, University of Guelph, Ontario, Canada. |
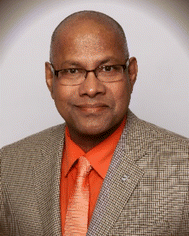 Amar K. Mohanty | Prof. Amar Mohanty is a Distinguished Research Chair in Sustainable Biomaterials at the Ontario Agriculture College and the Director of the Bioproducts Discovery and Development Centre. He is a Professor in the Department of Plant Agriculture and School of Engineering at the University of Guelph, Ontario, Canada. Dr Mohanty is the Editor-in-Chief of Sustainable Composites @ Composites Part C – Open Access. He is one of the most cited researchers worldwide with more than 800 publications to his credit, including 450 peer-reviewed journal papers, 70 patents (awarded/applied), 5 edited books, 25 book chapters, 350+ conference papers and over 200 plenary and keynote presentations. His Google-Scholar citation count is 48115 and the h-index is 98 (January 29, 2023). He has received many awards, the most recent one being the prestigious Miroslaw Romanowski Medal of 2021 for his significant scientific contributions to the resolution of environmental problems from the Royal Society of Canada. He has also received the Synergy Award for Innovation from the Natural Sciences and Engineering Research Council of Canada (NSERC), the Andrew Chase Forest Products Division Award from the American Institute of Chemical Engineers (AIChE) and the Lifetime Achievement Award from the BioEnvironmental Polymer Society (BEPS). |
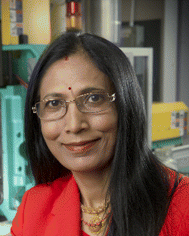 Manjusri Misra | Dr Manjusri Misra is a Professor and Tier 1 Canada Research Chair (CRC) in Sustainable Biocomposites in the School of Engineering and holds a joint appointment in the Department of Plant Agriculture at the University of Guelph. She is also the Research Program Director of the Bioeconomy Panel for the Ontario Agri-Food Innovation Alliance, a program between the Ontario Ministry of Agriculture and Rural Affairs (OMAFRA) and the University of Guelph. Dr Misra completed her Bachelor's, Master's, MPhil and PhD from Ravenshaw College at Utkal University in India focusing on Chemistry with a specialization in Polymer Chemistry and Natural Fibers during her graduate program. Dr Misra's current research focuses primarily on novel bio-based composites and nanocomposites from agricultural, forestry and recycled resources for the sustainable bioeconomy moving towards the Circular Economy. She has authored more than 750 publications, including 445 peer-reviewed journal papers, 25 book chapters, and 55 patents. She is the editor or co-editor of 5 books in the area of biocomposites and nano-composites. In 2020, she was selected as one of Canada's Most Powerful Women: Top 100 Award Winner in the Manulife Science and Technology category from the Women Executive Network. She is a Fellow of the Royal Society of Chemistry (UK), the American Institute of Chemical Engineers (AIChE), and the Society of Plastic Engineers (SPE)). |
Environmental significance
Environmental, economic, and societal concerns over the effect of climate change led countries to deploy CCU and CCS technologies in an attempt to combat global climate change. Although enormous efforts have been made on deploying CCU and CCS technologies, neither recent technological advances in CCS and CCU technologies nor their environmental, economic and societal implications have been thoroughly examined. We have compiled information on GHG emission reduction initiatives, including CCS and CCU emphasizing the recent technological advances and economic, environmental and societal implications. The prospects of CCS and CCU for mitigating global climate changes have also been discussed. We sincerely believe that this work fits the scope of Environmental Science: Advances.
|
Introduction
Greenhouse gas (GHG) emissions continue to increase with industrial growth to meet the rising demand of the increasing population on the earth. Fig. 1 depicts the emissions of CO2 from different sectors on the earth.1 Most of the climate change in the last 50 years resulted mainly from human activities, and population growth is noted to be the main driver of GHG emissions.2 It is noted that about 87% of CO2 emissions produced by human activities are from burning of fossil fuels.3 The growing concerns about the global warming led different jurisdictions to initiate CCS initiatives in an attempt to combat climate change. Despite various climate change mitigation initiatives, CO2 emissions from energy sectors increased by 1.7% in 2018 compared to the previous year and reached 33.1 billion tonnes.4 Annual global anthropogenic CO2 emissions are 32
000 million tonnes, while utilization is noted to be only 200 million tonnes.5 The global emissions need to be reduced at a rate of 3% (37 Gt CO2 per year) to net-zero by 20506 to limit global warming to 2 °C. Fossil fuel-powered plants (industries/power plants) require CCS for low carbon energy technologies and to mitigate rising emissions;7,8 however, low carbon technologies are yet to be ready for commercial deployment.9 The International Energy Agency (IEA) urged for annual CCS of 6000 MtCO2 by 205010 to combat rising global warming. However, by 2040 more than 2500 large-scale CCS/CCU facilities are required to limit climate change to 2 °C.11–13 The methods of post-combustion carbon capture include adsorption by solid materials and aqueous solutions14 along with afforestation and in situ injection and storage of carbon in the form of treated biomass (biocarbon/biochar). The adsorption methods are less energy-intensive as well as avoid the production of contaminated sorbents and adverse byproducts.14,15 Consequently, carbon balance not only in the terrestrial ecosystem but also in the entire ecosystem by 2050 would be the key to mitigating global climate changes.
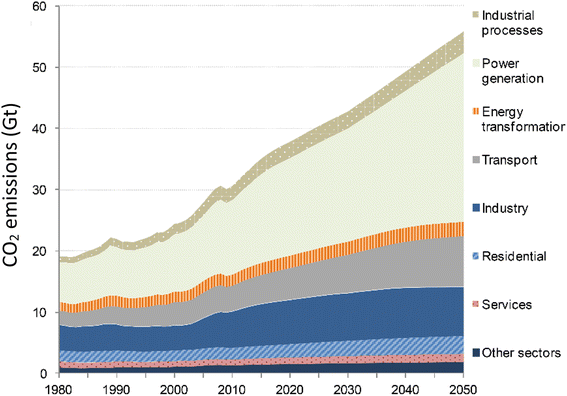 |
| Fig. 1 Projected global CO2 emissions from different sectors.1 [The power generation sector is the main contributor, followed by the industry and transportation sectors. Deployment of carbon capture, utilization and storage initiatives in power generation and industrial sectors can play a key role in mitigating the increasing GHG emissions]. | |
The commonly used CCS/CCU routes are the bioroute (biomass is grown and processed for producing chemicals) and the current fossil fuel and chemical processes, which are equipped with a carbon capture infrastructure and the captured carbon is used for green hydrogen production in a chemical process.16 Several authors have noted that pyrolytic CCS (PyCCS) is a potential way to mitigate global climate changes.17,18 In a PyCCS process, biochar/biocarbon and bio-oil, as well as CO2 captured from pyrogas, are stored for a prolonged period, which may evolve as a key tool in developing sustainable development goals and mitigating climate change.17
The Intergovernmental Panel on Climate Change (IPCC) identified four potential pathways to limit the global temperature rise to 1.5 °C and foresee the significant contribution of CCS and bioenergy with CCS (BECCS),11,19 which could be the key to limiting the climate change to 1.5–2 °C;11,20 however, the process is yet to be implemented on the industrial scale.6 In the long-term, the CCS approach tends to be a cost-efficient power supply; however, the energy efficiency is hindered by the energy requirement in operating the CCS process.19 The International Energy Agency (IEA) also projected the required sectoral changes for achieving the CO2 emission mitigation target. CO2 emission reduction through CCS, renewables, nuclear generation efficiency, power generation efficiency and fuel switching, end-use fuel switching, and end-use fuel and electricity efficiency need to be 19, 17, 6, 5, 15 and 38%, respectively, to meet the climate change goal by 2050.21,22Fig. 2 represents the required emission reduction through different technological changes from 2010–2050.22 Although numerous efforts are underway to deploy carbon capture, utilization and storage initiatives, their economic, environmental and societal prospects are yet to be fully developed. This study compiled information on greenhouse gas (GHG) emission reduction initiatives, including CCS and CCU, and recent technological advances in CCS and CCU, and their economic, environmental and societal implications have been discussed.
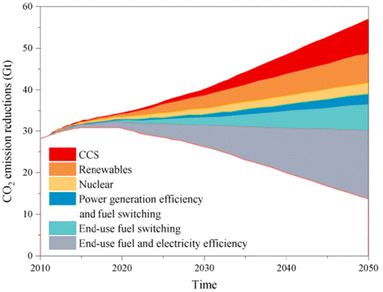 |
| Fig. 2 Projected technologies to be used to mitigate climate change.22 [Although enormous efforts are underway in every sector of human activities for mitigating rising GHG emissions and meeting the climate change targets, a wide range of technologies will be necessary to reduce energy-related CO2 emissions substantially. Among the projected technologies, the end-use fuel and electricity efficiency leads the potential emission reduction, followed by CCS, renewables and end-use fuel switching]. Copyright 2022, Elsevier (Earth-Science Reviews; Open access). | |
Carbon capture, utilization and storage (CCUS) initiatives
CCUS encompasses carbon capture, separation and compression, transport, storage, and utilization and is a recognized promising emission mitigation technology.23 Various methods are being used or proposed in the CCS or CCU initiatives (Fig. 3): afforestation, application of biomass for bioenergy/biomaterial production, direct carbon capture from air, direct injection of industrial carbon into the ocean/subsurface reservoirs or utilization of captured carbon from various industries, and produced different end-products such as chemicals or fuels or plastic products or algae.24–27 A study that introduced industrial CCS in a global multi-region multi-sector energy-economic model also confirmed the CO2 mitigation opportunity in industrial sectors, especially in the chemicals, steel and cement industries.12
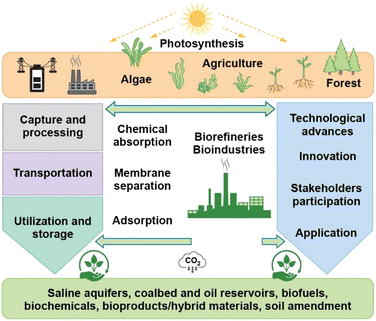 |
| Fig. 3 An overview of carbon capture, utilization, and storage technologies. [Carbon can be captured from the power generation or industry sector or from the atmosphere through chemical absorption, membrane separation or adsorption, concentrated at high pressure and temperature, transported to the onshore or offshore reservoir or saline aquifers and then injected for storage. Alternatively, atmospheric carbon can be captured through photosynthesis and converted into various bioproducts (e.g., biocarbon, bio-oil, graphene, etc.). For a successful implementation of CCS and CCU, technological advances and stakeholder participation are required]. | |
BECCS and afforestation with land use management are recognized as the main negative emission technologies.28,29 However, CCU was noted to be a better option compared to CCS,25 and CCU has higher acceptance.30 In contrast, the CCS approach is noted to be more practical31,32 and can be adapted to address global climate change challenges.31 CCU is also recognized as a valid alternative to geological storage and producing value-added products.33 For example, emissions from steel mills and gasified waste biomass were converted directly into monoethylene glycol (MEG) through fermentation by using an engineered bacterium as the building block for various plastic products such as resins, fibres, polyethylene terephthalate (PET) and bottles;34 however, the demand of CO2 as a feedstock for various end-products is noted to be less than global CO2 emissions.30 However, CCU is identified to be energy-intensive (10–25 times higher than the CCS process if hydrogen is produced), mainly because of greater electricity consumption in hydrogen production.16 On the other hand, the bio-route (biomass is grown and processed for producing chemicals) requires 40 and 400 times more land area compared with CCU and CCS processes, respectively.
The present technologies and infrastructures can be used for CCS instead of reshaping the chemical industries for CCU, i.e., hydrogen production from captured carbon; however, the authors noted that each process has its pros and cons; thus, there is no clear winner.16 Although various technologies are available for decarbonization of industries, CCS is noted to be the cheap option for the cement and steel industries.35,36 New power plants operating on fossil commodities require about 25–30% energy per net kW h output for carbon capturing.31 In an advanced chemical process, anthropogenic carbon is captured and converted into inorganic solid carbonates to reduce the carbon intensity of energy systems.37 In addition, carbonates can be produced by deploying accelerated carbonation in solid waste management stages, which have the reuse potential.38
Geological storage
CCS usually consists of four stages: capturing, compression, transportation and injection.39,40 The depleted oil reservoirs, coal beds or deep saline aquifers,41–43 and in situ mineralization in basalts44,45 are used for geological CO2 storage. It is estimated that oil and gas reservoirs as well as saline aquifers have adequate capacity to store anthropogenic CO2 for two centuries.24 The captured carbon is concentrated at high pressure and temperature (at a supercritical state; 31 °C at 73.77 bar)3,46 and then transported (usually through pipelines)47 to onshore or offshore reservoirs or into the saline aquifers for storage. The injected carbon reacts with other minerals in the host rocks and produces solid minerals, considered a permanent form of sequestration (known as mineral carbonation).48 On the other hand, carbon mineralization also has the potential where CO2 from waste can react with mineral/metal oxides, silicates or hydroxides to produce carbonates.38
For in situ mineralization in basaltic rocks, CO2 is separated from other non-condensable emissions in the exhaust gas of a thermal power plant through water dissolution and then transported to the well-site and injected along with geothermal brine from the power plant.44 Koukouzas et al. noted that the physicochemical properties of basaltic rocks provide significant advantages for CO2 mineral carbonation and thus for CCS.45 A laboratory-based study on mineral carbonation in fluid-rocks, i.e., representative mantle rocks also confirmed that carbon can be stored in mantle rocks.49
Afforestation and reforestation
Afforestation and reforestation is another potential pathway of carbon capture from air through photosynthesis and produces oxygen, thus reducing CO2 concentration in air,50,51 which is also known to be a nature-based solution.52 A hybrid model (computable general equilibrium model) for China predicted that annual carbon removals through afforestation will reach 617 MtCO2 in 2060.53 The model also projected that most biomass would consist of 43–47% cellulosic crops and 49–52% residues in 2060; thus, the cropland in China would reduce by 6.9–8.3% due to land competition.53 The Chinese study also indicates that not only in China, but this trend would also prevail in most other jurisdictions. Ocean afforestation (with Sargassum) was also reported to be effective for CCS; however, carbon removal efficiency of Sargassum reduced by 20–100% because of nutrient reallocation and calcification by marine life.54
The afforestation of unproductive or abandoned farmland or non-forest land increases carbon land sinks and thus can mitigate climate change.55 The authors also noted that the choice of species and the forest management strategy have a significant impact on carbon stocks.55 Although afforestation is a potential pathway for CCS, emission mitigation of afforestation depends on the forest growth rate irrespective of tree harvesting.56 The soil organic carbon also has valuable implications in afforestation or reforestation and their management.57 Reforestation also shows promise for carbon capture; however, there are concerns about water use for CO2 fixation.58 In another study, it is also noted that afforestation may be limited by water availability and about half of the suitable tree restoration on the earth are experiencing water scarcity for at least 7 months of the year.59 The higher temperature due to the global warming can accelerate decomposition of the soil organic matter, which affects nutrient availability and thus plant productivity.60 In addition, the rising concerns about land-use change can also restrict afforestation activities for carbon capture. Consequently, plant species and land need to be selected carefully for any afforestation and reforestation activity.
Seaweed/algae
The photosynthesis rate of algae was noted to be forty times greater than those of terrestrial plants,61 and thus can play a crucial role in carbon capture and mitigating GWP. Then algae can be used for diversified biomaterials and biofuels and thus can also be a potential BECCS pathway. For example, microalgae are identified as one of the potential feedstocks for third-generation bioenergy/biofuels for transferring atmospheric CO2 into biomass through photosynthesis and algae are accounted for 50% of global organic CO2 fixation;62 however, it is not yet commercially viable and is currently operating at a pilot scale only.63 Technological advances in the cultivation and conversion process of microalgae to bioenergy would be effective in CO2 removal. In addition, algae contain health-beneficial components such as iodine and tyrosine as well as antioxidants,64 and thus can be used as vegetable/food ingredients in the food industry or the medical industry and can help in mitigating CO2 in the environment. Although seaweed farming is likely useful in achieving some of the sustainable development goals (zero hunger, sustainable production, and consumption, economic growth, etc.) of the United Nations but it may affect marine life.65 In addition, BECCS deployed in algal bioenergy (bicarbonate-based integrated carbon capture and algae production) can also be a low-carbon algal bioeconomy.66
Microalgae use CO2 as their building block and can be used for various value-added products such as biofuels, carbohydrates and lipids, biomaterials, and extracellular polymeric substances, and electricity.67 In the USA, a model study confirmed that pure CO2 from the corn ethanol production process could be used for algae cultivation in an open pond, thus reducing GHG emission and water stress and can avoid the energy-intensive carbon capturing process for CCU.68 A wide range of value-added products for industrial applications can also be produced from algae-based biomass, such as food, feed and cosmetics.69 It seems that algae not only have a promising future for removing atmospheric CO2 but also can play an important role in producing food, feed and biofuels.
Application of biocarbon for carbon capture and storage
Biocarbon/biochar used for soil amendment is one of the promising pathways to capture and store carbon as well as improve soil health;17 however, the accumulation capacity of organic carbon is limited.70 The carbon efficiency of the thermal conversion of biomass into biochar is noted to be only 30–50%; however, it can be more than 70% in the case of pyrolytic CCS.17 It is also argued that carbon in bio-oil can be sequestered for more than 1000 years if pumped into the depleted fossil deposits/reservoirs.71 Simultaneous activation and graphitization at high temperatures in the presence of iron acetate and potassium acetate produced porous graphitized carbon facilitating a smooth transition from micro to mesopores which is suitable for both low- and high-pressure carbon capture.72 The carbon capture efficiency (35–74 mg g−1) was noted to be dependent on the treatment conditions of biochar/biocarbon.73 However, microporous carbon had a higher adsorption capacity, i.e., it seems adsorption depends on the narrow space (<1 nm) rather than on the surface area or the pore space of activated carbon.74
Activated carbons produced from biomass usually have a high surface area and porous structure,75 thus having great application in many sectors such as carbon capture, contaminant removal, etc.76 In addition, the surface functionality and microporosity of activated carbon can be controlled by changing sodium hydroxide concentration.77 The CO2 adsorption capacity of graphene-type materials (0.07 mol g−1) is noted to be ten times higher than that of the activated carbon.5 The regeneration of the CO2 sorbent is energy intensive; however, the recent advances in sorbent technology reduce the energy consumption in the regeneration process. For example, regeneration energy consumption for the modulated amine blend reached 2.17 GJ per tonne of CO2; however, it is 3.5 GJ in the case of monoethanolamine.5
Various adsorption methods are used in pre-conversion and post-conversion stages, such as amine-based, alkaline, activated carbon, etc.; however, the amine-based solvent (monoethanolamine) is noted to be the most commonly used solvent.78 The specific surface area increases several times if biocarbon is activated.74 The adsorption capacity of porous biocarbon produced from the undervalued biomass led to increased applications for purification in both gaseous and aqueous phases.79 Porous biocarbon is recognized as an excellent adsorbent to remove CO2 from biogas/syngas to produce bio-CH4.79 Pressure swing adsorption (PSA) is one of the most used gas purification processes using a carbon molecular sieve,80 which uses pressure modulation to capture undesired gases such as CO2 in biogas.81
Policy efforts for carbon capture, storage, and utilization
Many policy efforts are underway to commercialize the CCS initiatives, such as cash and tax incentives, preferential financing, etc.7,82 Turning carbon sources into carbon sink initiatives are in place to combat the rising GHG emissions, especially in developing countries, through the World Bank BioCarbon Fund or the voluntary market.83 The United States of America (USA) established the Regional Carbon Sequestration Partnership Initiative comprising more than 400 organizations in 43 states to support research for storing carbon through field projects. Canada has conducted 19 small-scale field research studies in four provinces and developed the required framework to validate geological carbon storage.82 The capacity of underground/geological (oil and gas; coal bed and saline aquifers) storage is projected to be 10
329–200
492 GtCO2;24 however, site development would be costly and time-consuming.11 The storage capacity of various reservoirs in the USA and Canada is 2618–21
978 GtCO2, which can store anthropogenic CO2 for two centuries.24 On the other hand, a hybrid computable general equilibrium (CGE) model for China predicted that in 2060 the annual carbon removal through bioelectricity with CCS, biofuels with CCS and afforestation will reach 2118, 170 and 617 MtCO2, respectively.53
CO2 source–sink mapping analysis noted that almost all stationary CO2 (annual emission from power plants, refineries, iron and steel mills and cement factories is 1.7 Gt) in China for 367 years could be stored in offshore gas and oil reservoirs and saline aquifers within a 350 km distance from the coast.84 The increasing policy support resulted in the development of 23 large-scale projects, mainly in natural gas processing and chemical processing plants in the world, with an annual CO2 capture capacity of 40 million tonnes.11 However, in 2021, 27 plants were in operation, and the total CCS capacity of those plants was 36.6 million tonnes.85 Although various activities are underway, technological readiness, the capital cost, and the credibility of revenues and incentives are noted to be the important attributes.7 The technological advances as well as policy initiatives may dictate the viability of CCS and CCU.11
Norway is the first country to adopt the CCUS initiative and draft an action plan, ‘Climate Settlement 2007’ for becoming a net carbon-neutral country86,87 followed by Japan which enacted a law in 2007 to legalize the injection of CO2 into underground saline aquifers.87,88 In 2018, the incentive for CCUS was enacted in the United States of America (USA) to enable it to be economically viable. It is noted that 50% of government financing in infrastructure development would enable to profitably capture and transport 19 million tonnes of CO2 each year, while 100% of financing would lead to enhancing the oil recovery industry by 50%.89 The progress of CCUS is falling short of what is required to achieve net-zero emissions. For example, the CCS technology is deployed in only 3% of electricity generation plants in some jurisdictions such as China, Japan, the European Union, and the USA.18 The UK's committee on climate change recommends the deployment of various technologies for electricity generation to achieve the emission reduction targets of 50–100 g CO2 per kW h.18Fig. 4 depicts various combinations of electricity technology deployment to achieve the emission reduction target of the UK.18 The two surfaces (ACDB and cdb) of this figure represent the technological mix to meet the reduction targets of 50 g CO2 per kW h and 100 g CO2 per kW h, respectively. For example, point A is pure nuclear (31 GW), and C is the mix of wind (56 GW) and nuclear (18 GW) in the absence of gas-CCS. This model would be useful for other jurisdictions to outline their strategies for meeting climate change goals.
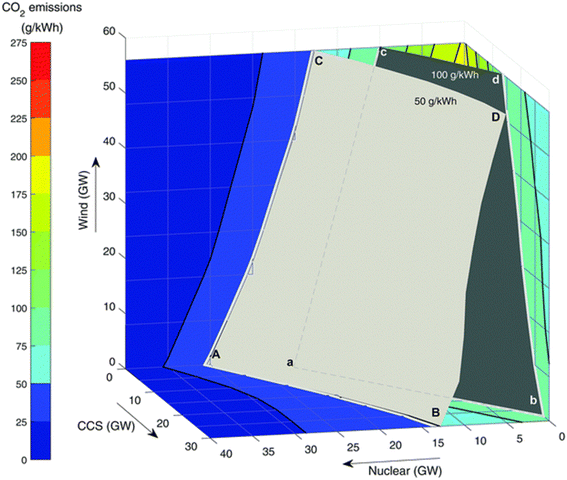 |
| Fig. 4 Technology deployment requirements in order to meet the UK's CCC target.18 [The surfaces show the requirement of technology deployment for meeting the UK's decarbonization targets of the power generation sector for CO2 emissions of 100 g kW−1 h−1 and 50 g kW−1 h−1. For a weaker emission target (100 g kW−1 h−1 target) a pure CCS solution is possible at just 27 GW of CCS. On the other hand, for a maximum wind scenario (56 GW), a combination of low carbon capacity power systems is required (either 11 GW new nuclear or 19 GW of CCS)]. Copyright 2018, Royal Society of Chemistry (Energy & Environmental Science; Open access). | |
Wang et al. noted that the risk in the implementation of CCS projects increases with the plant capacity, and larger plants face a greater risk. For example, the risk of failure increases by 50% if the annual plant capacity is raised by 1 million tonnes of CO2.90 However, increased policy support in building its market as well as gradual upscaling would balance the risk and return.90 The authors also argued that the existing support is inadequate to mitigate the risk allied with CCS project upscaling. The carbon taxes are noted to be a better option for the deployment of CCS technologies compared to emission trading.90 The recent introduction of the 45Q tax credit in the USA stimulated private sector industries for the deployment of CCS, which would be the foundation for future CCS projects.82 Although CCS/CCUS is useful for decarbonizing coal-based power plants, technological advances, markets, and policy design would determine the viability of CCU/CCUS in coal-based power plants.82
The carbon finance initiatives can be a potential way not only in developing countries but also in developed countries. For example, a study in Alberta, Canada, confirmed that carbon incentives for afforestation and agricultural farming for new cash crops are beneficial.83 China has adopted CCUS for future strategies for carbon mitigation initiatives which have exhibited a strong influence on progress; however, the Chinese CCUS policy was noted to be insufficient for further development.87 It is also noted that CCUS has the potential to meet the energy demand and CO2 emission reduction limit in developing countries87 and is crucial for decarbonizing the energy system.91 The existing energy system can be decarbonized through CCUS, and it can be achieved at a low cost through hydrogen storage and renewables.13,92 However, the construction of new power plants without CCS receive higher public support compared with the plants with CCS because of greater taxes to support the CCS approach; thus, a specific policy is required to shape the public support for the deployment of large-scale CCS projects.93 In addition, the public support for CCS seems to be low,94,95 which needs to increase for the successful deployment of CCS projects.
Advances in carbon capture and storage
CCS and CCU are widely recognized decarbonizing processes having the potential to meet climate change challenges.18,96 The potential CCS technologies applied in power plants are post-combustion (solvent is used to capture carbon and it is passed through a regenerator for stripping the CO2 from the solution), oxy-combustion (fossil fuels burn in oxygen) and pre-combustion (fossil fuels are converted into synthetic gas, especially into hydrogen and CO2).97 Becattini et al. revealed that the CCUS initiative where CO2 was captured directly from air or from the point source such as the biogenic carbon capture from waste to energy plants (in both cases, CO2 was used as a feedstock to produce jet fuel) was feasible for the net-zero emission aviation industry. Although CCUS exhibits the potential of capturing anthropogenic carbon,38 there are pros and cons to the processes as well as challenges to achieving net-zero emission aviation.96 Leakage has been identified to be one of the major constraints in geological carbon storage; however, various sealant materials and mechanisms have also been developed for CO2 leakage remediation (Portland cement, resins, geopolymer cement, foams, biofilms barriers, gel systems, and nanoparticles).41 Lime production through the calcium-looping process integrated with the steel industry is identified as a potential pathway for decarbonizing the industrial sector by 2030 and meeting the long-term decarbonization target, which also emerged as economically viable.98
Fuel switching, improvement of energy efficiency and increasing use of renewables are noted to be useful in the decarbonization of energy systems.99 There are concerns about the environment and biodiversity in the case of the deployment of large-scale bioenergy systems with CCS because of its land and water demand20 as well as competition with food crops for productive land. However, the impacts of BECCS on agricultural commodity prices are projected to be limited (5–15%), and the deployment of large-scale BECCS projects is not detrimental to agricultural commodity prices.28 Consequently, careful consideration must be placed on the deployment of a large-scale bioenergy system coupled with CCUS to avoid competition for land with food crops to avoid any unwanted rebound effects on the food supply chains for the rising population on the earth; thus, a regulatory framework is a must for land use for the jurisdictions adopting large-scale bioenergy systems.
Environmental, economic, and societal impacts
Although CCS plays an important role in mitigating global climate change,100,101 its economic and environmental costs are not well known,101 or economic viability and technological feasibility are challenging,100 or the potential economic and environmental implications of decarbonization pathways have not been fully evaluated.53 A study that simulated hydrogen production (i.e., energy production) from either natural gas or biomethane with CCS provided economic and environmental benefits; however, the use of biomethane was reported to be a better option.102 The CCU initiatives in hydrogen production reduced 23% of global warming potential (GWP) compared to the CCS initiatives, where captured carbon was used for polyol production.103 On the other hand, GWP reduction from the power plants was 63–82% in the case of CCS; however, it was 4–48% in the case of mineral carbonization.78 Schakel et al. noted that dimethyl ether (DME) produced from captured carbon through dry reforming reduced GWP by 8% compared to that without CO2 capture; however, it was 37% higher than the conventionally produced DME, while carbon is stored.104
The addition of CCS with the steam reforming of natural gas into hydrogen reduced GWP by 45–85%, while other impacts slightly increased.8,102 The authors noted that at a 20–60% increased production cost of hydrogen, 55–90% of CO2 of the plant can be captured.102 For example, although 90% reduction of direct CO2 emission was observed in the case of CCS-adopted conventional power plants (combustion-based electricity generation), the actual reduction reached 40–80% for the climate change impact; however, other environmental impacts (AP, EP, HTP, and ETP) increased to a varying extent,8,105 which resulted in 15–44% energy penalty.8 The environmental impacts of CCS-adopted coal-based power plants also depend on CCS technologies.78
A trial experiment for offshore carbon storage confirmed the potentiality of offshore carbon storage even though a small leakage was observed where each day 43–143 kg CO2 was injected.106 A model also projected that with a yearly <0.01% leakage, 90% of injected carbon would sequester after 1000 years;107 however, if the leakage can be controlled to <0.001%, the climate change mitigation strategy becomes efficient.108 The pH of seawater changes in the case of leakage in offshore storage.109 Industrial sustainability can also be improved by deploying CCS.12,110 For example, the environmental burden of the steel industry was reduced from −0.5 to 0.1 t CO2 per t steel by introducing bioenergy and CCS, whereas it was 1.3–2.4 t CO2 per t steel without bioenergy and CCS; however, CCS provided greater mitigation than bioenergy while the combination of bioenergy and CCS had greater decarbonization potential in the steel industry.110 The environmental impacts were found to be widely varied among the reviewed articles (Table 1) because of the variation in the system boundary, assumption, feedstock, technology, plant capacity and the scenarios or deployment of CCS in the systems.78,111
Table 1 Carbon capture technologies and their environmental impactsa
CCS/CCU method |
Feedstock |
Purpose |
System boundary |
Plant capacity |
GWP, kg CO2 eq per MJ |
AP, kg SO2 eq per MJ |
References |
CCS: Carbon capture and storage; SMR: steam methane reforming; ATR: autothermal reforming; GWP: global warming potential; AP: acidification potential; kt: thousand tonne.
|
Vacuum pressure swing adsorption (VPSA) and amine-based (SMR and ATR) |
Syngas; natural gas |
Hydrogen production |
Cradle-to-gate |
75 kt |
0.022–0.038 |
— |
102
|
Oxyfuel combustion |
Natural gas |
Electricity production |
Cradle to grave |
— |
0.033 |
0.0001 |
118
|
Oxyfuel combustion |
Coal |
Electricity production |
Cradle to grave |
— |
0.055 |
0.0004 |
118
|
Monoethanolamine (MEA) |
Natural gas |
Electricity production |
Cradle-to-grave |
— |
0.046 |
0.0002 |
119
|
Integrated gasification combined cycle (IGCC) |
Coal |
Electricity production |
Cradle-to-gate |
440–610 MWe |
0.094–0.255 |
0.00014–0.00049 |
105
|
Integrated gasification combined cycle (IGCC) |
Coal |
Electricity production |
Cradle-to-gate |
600 MW |
0.226 |
0.00011 |
120
|
Integrated gasification combined cycle (IGCC) (solvents) |
Coal |
Electricity production |
Cradle-to-gate |
— |
0.031–0.047 |
0.0001 |
121
|
Integrated gasification combined cycle (IGCC) (MEA) |
Natural gas |
Electricity production |
Cradle-to-gate |
— |
0.021–0.068 |
0.00002–0.0002 |
121
|
Pulverized coal (MEA) |
Coal |
Electricity production |
Cradle-to-gate |
— |
0.022–0.076 |
0.00009–0.00058 |
121
|
Oxyfuel combustion |
Coal |
Electricity production |
Cradle-to-gate |
— |
0.0069–0.0489 |
0.000036–0.00033 |
121
|
The cost is one of the main impediments to CCS and CCU in the energy sector.11 Carbon capture is the main cost component in a power plant that integrates carbon capturing systems.112 Energy consumption in the CCU process was 20 times higher than that in CCS; thus, the CCS process emerged as the most cost-effective pathway for decarbonizing the aviation industry as well as it can be cost-competitive with the conventional jet fuels while a carbon tax is harnessed.96 On the other hand, value-added products produced in CCU approaches are noted to have improved the carbon capture process.33 Direct carbon capture from the air pathway was more expensive than point source carbon capture.96 On the other hand, the CCUS approach is noted to be both economically and environmentally favourable, where shale and flue gas from a coal-based power plant were co-converted into value-added chemicals (methanol, urea and sulphur).113
The MIT Economic Projection and Policy Analysis model revealed that the BECCS could be a backstop technology at a price of $240 per tonne CO2.28 A simulated study revealed that the carbon capture cost for a bioenergy system (sugarcane biorefineries) varied from $17.2–262.0 per tonne CO2 depending on the seasonal variability, operating time and plant capacity.114 However, various jurisdictions have set up the goal of reducing the cost of CCS/CCU. For example, the US Department of Energy's (DOE) goal is to reduce to $30 per tonne by 2030 through innovation pathways (equipment, processes and materials).11 However, the projected decarbonizing cost will reach $82 per tonne of CO2 by 2050 for the ambitious decarburization initiative for creating a carbon-neutral economy.35 On the other hand, for an optimal CCUS supply chain network (mixed integer linear programming model), the emission mitigation cost was noted to be about $23.53 per tonne of CO2.115 Climate change mitigation was noted to be 12% and 71% higher in 2075 and 2100 without adopting CCS compared with the adoption of CCS in the industrial sector.12 In addition, the CCU-adopted energy system becomes profitable at a price range of £72–102 MW−1 h−1 while the current market price is £52 MW−1 h−1; however, the scope of CCU for decarbonization is small.92
The cost of CO2 emission abatement from coal-fired power plants by deploying CCS depends on the CO2 removal efficiency, plant location and capacity, variable and fixed cost, and CCS technologies (Table 2);116,117 however, the cost increased by 58–108% compared to that without CCS.117 In the USA, the 45Q tax credit was $20 and $10 per tonne of CO2 geological storage and CO2-EOR, respectively, in 2018, and it will be raised to $50 and $35, respectively, in 2026.82 BECCS was noted to be the most cost-effective route to achieving negative emissions when combined with photosynthesis and carbon capture.51
Table 2 Cost of carbon capture and storage (CCS), and carbon capture and utilization (CCU).a
CCS/CCU method |
Feedstock |
Product |
System boundary |
Plant capacity |
Cost, $ per MJ |
References |
FT: Fischer–Tropsch.
|
Gasification and fermentation |
Woody biomass |
Hydrogen production |
Cradle-to-gate |
10.5 MW |
0.033 |
122
|
Gasification (FT) |
Eucalyptus and pine residue |
Biodiesel |
— |
— |
0.040 |
123
|
Gasification (IGCC) |
Coal |
Electricity |
— |
4.24 PW−1 h−1 |
0.017 |
124
|
BECCS |
Biomass (cofiring) |
Electricity |
— |
0.55 PW−1 h−1 |
0.021 |
124
|
BECCS |
Biomass (cofiring) |
Electricity |
— |
1.74 PW−1 h−1 |
0.036 |
124
|
BECCS |
Biomass (cofiring) |
Electricity |
— |
3.34 PW−1 h−1 |
0.070 |
124
|
The low oil price, public acceptance, inconsistent policy, lack of incentives and high investment for geological storage hinder the profitability of CO2-enhanced oil recovery and implementation of CCS projects.24 The authors perceived that public–private partnership, stakeholder engagement, carbon pricing, and the development of regional CCS corridors would accelerate the implementation of CCS projects. However, the present incentive and investment packages are identified to be inadequate for the deployment of CCUS projects, which can be improved by improving carbon trading.125 It is also noted that the geological storage of carbon affects the quality of coal as the pore structure of the coal changes due to different pressure and temperature (usually at the supercritical phase), which also affects the carbon storage capacity.126 Similarly, the porosity of sandstone is also affected due to carbon geological storage in sandstone reservoirs.127 Stored carbon tends to move vertically towards the surface due to the density difference with brine.127 The geological formation contains a minute amount of organic materials, which influence CO2 containment security and fluid dynamics.22 In addition, the time-dependent deformation of the rock affects its properties which influence the injectivity and security of geological storage;128 thus, the rebound effects of geological storage of carbon, especially in coal beds, need to be carefully considered.
The integrity of CCS and the comprehended risk of CO2 leakage as well as social acceptance are major concerns which may inhibit the implementation of CCS projects.11 The over-pressurization during the injection process is noted to be one of the main reasons for CO2 leakage in the case of geological storage, which also affects the surface water pH, enhances organic compounds in water and metal and metalloid mobilization, and the alteration depends on the amount of leakage.129 The sealing capacity also depends on the surface wettability, and thus the carbon storage potential and integrity of geological storage.22,130
Despite the large-scale BECCS reducing pressure on the planetary boundary, BECCS through afforestation may exacerbate water scarcity,131 especially in dryland regions such as Africa and Oceania.59 In addition, in the case of ocean afforestation, the carbon removal efficiency of Sargassum reduces because of nutrient reallocation and calcification by marine animals;54 thus, its potential rebound impacts on ocean animals such as fish need to be explored.
Although enormous efforts are underway to reduce GHG emissions and climate change, only 24 countries were able to record a reduction in their annual GHG emissions, where energy sectors, especially electricity and heat generation, played the main role in reducing emissions.132 Consequently, it seems that CCUS coupled in/with energy systems and industry would lead to sustaining GHG emission reduction targets that are set by different countries. For example, the Global CCS Institute is an international think tank whose mission is to accelerate the deployment of carbon capture and storage (CCS), a vital technology to tackle climate change and deliver climate neutrality.133 It seems that the sustainability of CCUS is highly dependent on the scenarios (CCS or CCU deployment rate, CO2 storage capacity, land use, use of biomass resources, etc.) and technologies adopted for the study, carbon trading and stakeholder participation. In addition, CCS and CCU are recognized as linear and circular approaches;33 thus, the improvement of the CCU approach in reducing energy consumption is desirable and can grow in the near future. However, concerns about the economic and social impacts of BECCS, such as the competition for productive land and the rising food prices, have to be addressed for the deployment of large-scale BECCS projects.
Sun et al. revealed that energy stakeholders are favourable toward CCS while public attitudes vary as they are concerned about the risk of the CCS approach.134 Large-scale removal of atmospheric carbon dioxide (BECCS or afforestation) to combat climate change may lead to public disengagement because of a lack of widespread involvement as well as land-use complexities.135 Consequently, it is important to ensure the participation of all stakeholders, increase public awareness about CCS for the successful deployment of CCS projects and mitigate the risk of investment. In addition, interdisciplinary assessments such as techno-economic and life cycle assessments can be integrated to develop a standardized assessment framework for a broader sustainability check.
Conclusions
Understanding and identifying the CO2 utilization and storage technologies and their performance are complex as well as both CCS and CCU have challenges. However, acceptance of CCU seems to be higher compared to that of CCS. It is also important to note that multiple transition pathways may need to be adopted for a carbon-neutral economy, as a single transition pathway seems to be inadequate. The global warming potential (GWP) and the cost of energy systems that deployed CCS/CCU varied from 0.007–0.225 kg CO2 eq per MJ and $0.017–0.070 MJ−1, respectively. CCUS of fossil fuel-based CO2, as well as adequate use of biomass resources, stringent policy, financial benefits and stakeholder participation are crucial for the transition to address global climate change challenges. The technological advances, as well as policy initiatives may dictate the viability of CCS and CCU. Any technological advances and policy initiatives must be justified by broader sustainability checks to avoid risks to investment and climate change. The following measures can be adopted for a carbon-neutral economy.
• Multiple transition pathways (end-use fuel switching, bioenergy systems with CCUS, and end-use fuel and electricity efficiency).
• Innovation and technological advances in CCUS.
• Economic and tax incentives to CCUS initiatives.
• Policy initiatives to avoid land use complexities and avoid competition for land with food crops.
• Awareness on the beneficial impacts of CCUS initiatives to improve public acceptance.
• Broader sustainability assessment to avoid any rebound effects of CCUS initiatives.
Author Contributions
Methodology, investigation, data analysis, and writing—original draft preparation, P. R.; project conceptualization, methodology, administration, funding acquisition and supervision, A. K. M. and M. M.; writing—review and editing, A. K. M., M. M. and P. R. All authors contributed to the discussion, reviews and approval of the manuscript for publication.
Conflicts of interest
The authors declare that they have no known conflict of interest that could have appeared to influence the work reported in this paper.
Acknowledgements
This study was financially supported by the Ontario Ministry of Agriculture, Food and Rural Affairs (OMAFRA) – University of Guelph, the Bioeconomy Industrial Uses Research Program Theme (Project No. 030486, 030578 and 030648); OMAFRA-University of Guelph Gryphon's Leading to the Accelerated Adoption of Innovative Research (LAAIR) Program (Project No. 030736); the Ontario Ministry of Economic Development, Job Creation and Trade ORF-RE09-078 (Project No. 053970 and 054345); the Natural Sciences and Engineering Research Council of Canada (NSERC), Canada Research Chair (CRC) program Project No. 460788; and the Agriculture and Agri-Food Canada (AAFC), Maple Leaf Foods, Canada and the Bank of Montreal (BMO), Canada through Bioindustrial Innovation Canada (BIC) Bioproducts AgSci Cluster Program (Project No. 054015, 054449 and 800148).
References
-
OECD, OECD Environmental Outlook to 2050, in OECD Environment Directorate (ENV) and the PBL Netherlands Environmental Assessment Agency (PBL), 2011. Available from: https://www.oecd.org/env/cc/49082173.pdf Search PubMed.
-
CBD. Population Pressure and the Climate Crisis, The Center for Biological Diversity, 2021, [cited 2022 Jun 16]. Available from: https://www.biologicaldiversity.org/programs/population_and_sustainability/climate/ Search PubMed.
- T. Wilberforce, A. G. Olabi, E. T. Sayed, K. Elsaid and M. A. Abdelkareem, Progress in carbon capture technologies, Sci. Total Environ., 2021, 761, 143203 CrossRef CAS PubMed.
-
IEA. Global Energy & CO2 Status Report 2019, International Energy Agency, 2019, [cited 2022 Jun 8]. Available from: https://www.iea.org/reports/global-energy-co2-status-report-2019 Search PubMed.
- A. I. Osman, M. Hefny, M. I. A. Abdel Maksoud, A. M. Elgarahy and D. W. Rooney, Recent advances in carbon capture storage and utilisation technologies: a review, Environ. Chem. Lett., 2021, 19(2), 797–849 CrossRef CAS.
- R. S. Haszeldine, S. Flude, G. Johnson and V. Scott, Negative emissions technologies and carbon capture and storage to achieve the Paris Agreement commitments, Philos. Trans. R. Soc., A, 2018, 376(2119), 20160447 CrossRef PubMed.
- A. Abdulla, R. Hanna, K. R. Schell, O. Babacan and D. G. Victor, Explaining successful and failed investments in US carbon capture and storage using empirical and expert assessments, Environ. Res. Lett., 2020, 16(1), 14036 CrossRef.
- Y. Wang, Z. Pan, W. Zhang, T. N. Borhani, R. Li and Z. Zhang, Life cycle assessment of combustion-based electricity generation technologies integrated with carbon capture and storage: A review, Environ. Res., 2022, 207, 112219 CrossRef CAS PubMed.
-
IEA, Energy Technology Perspectives 2020: Special Report on Carbon Capture Utilisation and Storage CCUS in Clean Energy Transitions, 2020. Available from: https://iea.blob.core.windows.net/assets/181b48b4-323f-454d-96fb-0bb1889d96a9/CCUS_in_clean_energy_transitions.pdf Search PubMed.
-
GHG IEA, CCS Industry Build-Out Rates–Comparison with Industry Analogues, IEA GHG, 2017, vol. 30 Search PubMed.
-
S. Cornot-Gandolphe, Carbon Capture, Storage and Utilization to the Rescue of Coal. Glob Perspect with Focus China SAD, 2019 Search PubMed.
- S. Paltsev, J. Morris, H. Kheshgi and H. Herzog, Hard-to-Abate Sectors: The role of industrial carbon capture and storage (CCS) in emission mitigation, Appl. Energy, 2021, 300, 117322 CrossRef CAS.
- N. E. Vaughan, C. Gough, S. Mander, E. W. Littleton, A. Welfle and D. E. H. J. Gernaat,
et al., Evaluating the use of biomass energy with carbon capture and storage in low emission scenarios, Environ. Res. Lett., 2018, 13(4), 44014 CrossRef.
- I. Majchrzak-Kucęba and M. Sołtysik, The potential of biocarbon as CO2 adsorbent in VPSA unit, J. Therm. Anal. Calorim., 2020, 142(1), 267–273 CrossRef.
- N. Querejeta, F. Rubiera and C. Pevida, Enhanced capacity to CO2 sorption in humid conditions with a K-doped biocarbon, J. Energy Chem., 2019, 34, 208–219 CrossRef.
- P. Gabrielli, M. Gazzani and M. Mazzotti, The role of carbon capture and utilization, carbon capture and storage, and biomass to enable a net-zero-CO2 emissions chemical industry, Ind. Eng. Chem. Res., 2020, 59(15), 7033–7045 CrossRef CAS.
- H. Schmidt, A. Anca-Couce, N. Hagemann, C. Werner, D. Gerten and W. Lucht,
et al., Pyrogenic carbon capture and storage, GCB Bioenergy, 2019, 11(4), 573–591 CrossRef CAS.
- M. Bui, C. S. Adjiman, A. Bardow, E. J. Anthony, A. Boston and S. Brown,
et al., Carbon capture and storage (CCS): the way forward, Energy Environ. Sci., 2018, 11(5), 1062–1176 RSC.
- T. Mikunda, L. Brunner, E. Skylogianni, J. Monteiro, L. Rycroft and J. Kemper, Carbon capture and storage and the sustainable development goals, Int. J. Greenhouse Gas Control, 2021, 108, 103318 CrossRef CAS.
- S. V. Hanssen, V. Daioglou, Z. J. N. Steinmann, J. C. Doelman, D. P. Van Vuuren and M. A. J. Huijbregts, The climate change mitigation potential of bioenergy with carbon capture and storage, Nat. Clim. Change, 2020, 10(11), 1023–1029 CrossRef CAS.
-
IEA, Energy Technology Perspectives 2010: Scenarios and Strategies to 2050, International Energy Agency, 2010, [cited 2022 Jul 7]. Available from: https://iea.blob.core.windows.net/assets/04776631-ea93-4fea-b56d-2db821bdad10/etp2010.pdf Search PubMed.
- M. Ali, N. K. Jha, N. Pal, A. Keshavarz, H. Hoteit and M. Sarmadivaleh, Recent advances in carbon dioxide geological storage, experimental procedures, influencing parameters, and future outlook, Earth-Sci. Rev., 2022, 225, 103895 CrossRef CAS.
- S. Zhang, Y. Zhuang, R. Tao, L. Liu, L. Zhang and J. Du, Multi-objective optimization for the deployment of carbon capture utilization and storage supply chain considering economic and environmental performance, J. Clean Prod., 2020, 270, 122481 CrossRef.
- H. C. Lau, S. Ramakrishna, K. Zhang and A. V. Radhamani, The role of carbon capture and storage in the energy transition, Energy Fuels, 2021, 35(9), 7364–7386 CrossRef CAS.
- K. Arning, J. Offermann-van Heek, A. Linzenich, A. Kätelhön, A. Sternberg and A. Bardow,
et al., Same or different? Insights on public perception and acceptance of carbon capture and storage or utilization in Germany, Energy Policy, 2019, 125, 235–249 CrossRef.
-
C2ES, Carbon Utilization: A Vital and Effective Pathway for Decarbonization, Centerefor Climate and Energy Solutions, 2019, [cited 2022 Jun 15]. Available from: https://www.c2es.org/document/carbon-utilization-a-vital-and-effective-pathway-for-decarbonization/ Search PubMed.
- L. Tomić, V. Karović-Maričić, D. Danilović and M. Crnogorac, Criteria for CO2 storage in geological formations, Podzemni Radovi, 2018,(32), 61–74 CrossRef.
- M. Fajardy, J. Morris, A. Gurgel, H. Herzog, N. Mac Dowell and S. Paltsev, The economics of bioenergy with carbon capture and storage (BECCS) deployment in a 1.5 C or 2 C world, Glob. Environ. Change, 2021, 68, 102262 CrossRef.
- D. Huppmann, J. Rogelj, E. Kriegler, V. Krey and K. Riahi, A new scenario resource for integrated 1.5 C research, Nat. Clim. Change, 2018, 8(12), 1027–1030 CrossRef.
- A. Linzenich, K. Arning, J. Offermann-van Heek and M. Ziefle, Uncovering attitudes towards carbon capture storage and utilization technologies in Germany: Insights into affective-cognitive evaluations of benefits and risks, Energy Res. Soc. Sci., 2019, 48, 205–218 CrossRef.
- T. Wilberforce, A. Baroutaji, B. Soudan, A. H. Al-Alami and A. G. Olabi, Outlook of carbon capture technology and challenges, Sci. Total Environ., 2019, 657, 56–72 CrossRef CAS PubMed.
- Z. Zhang, S.-Y. Pan, H. Li, J. Cai, A. G. Olabi and E. J. Anthony,
et al., Recent advances in carbon dioxide utilization, Renew. Sustain. Energy Rev., 2020, 125, 109799 CrossRef CAS.
- F. Nocito and A. Dibenedetto, Atmospheric CO2 mitigation technologies: carbon capture utilization and storage, Curr. Opin. Green Sustainable Chem., 2020, 21, 34–43 CrossRef.
-
Bioplastics, Proof of Concept: Captured Carbon to Monoethylene Glycol (MEG) Bioplastics MAGAZINECOM, 2022, May [cited 2022 Jun 1]. Available from: https://www.bioplasticsmagazine.com/en/news/meldungen/20223105-LanzaTech.php?sn=sn814f66fed8fb948d8a7b03ad06eb3c Search PubMed.
- G. Safonov, V. Potashnikov, O. Lugovoy, M. Safonov, A. Dorina and A. Bolotov, The low carbon development options for Russia, Clim. Change, 2020, 162(4), 1929–1945 CrossRef CAS.
-
A. Favier, K. Scrivener and G. Habert, Decarbonizing the cement and concrete sector: integration of the full value chain to reach net zero emissions in Europe, in IOP Conference Series: Earth and Environmental Science, IOP Publishing, 2019, p. 12009 Search PubMed.
- G. Gadikota, Carbon mineralization pathways for carbon capture, storage and utilization, Commun. Chem., 2021, 4(1), 1–5 CrossRef PubMed.
- C. D. Hills, N. Tripathi and P. J. Carey, Mineralization technology for carbon capture, utilization, and storage, Front Energy Res., 2020, 8, 142 CrossRef.
-
R. Porrostami, A. Q. Zahedi and V. Etemad, Spatial Variability of Carbon Storage and Sequestration in Leaf Litter and Layers of Soil in the Forest Area of Jahannama Park, 2020 Search PubMed.
- J. Gusca and D. Blumberga, Simplified dynamic life cycle assessment model of CO2 compression, transportation and injection phase within carbon capture and storage, Energy Procedia, 2011, 4, 2526–2532 CrossRef CAS.
- D. Zhu, S. Peng, S. Zhao, M. Wei and B. Bai, Comprehensive review of sealant materials for leakage remediation technology in geological CO2 capture and storage process, Energy Fuels, 2021, 35(6), 4711–4742 CrossRef CAS.
- N. Koukouzas, P. Tyrologou, D. Karapanos, J. Carneiro, P. Pereira and F. de Mesquita Lobo Veloso,
et al., Carbon Capture, Utilisation and Storage as a Defense Tool against Climate Change: Current Developments in West Macedonia (Greece), Energies, 2021, 14(11), 3321 CrossRef CAS.
- M. Safaei-Farouji, H. V. Thanh, Z. Dai, A. Mehbodniya, M. Rahimi and U. Ashraf,
et al., Exploring the power of machine learning to predict carbon dioxide trapping efficiency in saline aquifers for carbon geological storage project, J. Clean Prod., 2022, 372, 133778 CrossRef CAS.
- T. M. P. Ratouis, S. Ó. Snæbjörnsdóttir, M. J. Voigt, B. Sigfússon, G. Gunnarsson and E. S. Aradóttir,
et al., Carbfix 2: A transport model of long-term CO2 and H2S injection into basaltic rocks at Hellisheidi, SW-Iceland, Int. J. Greenhouse Gas Control, 2022, 114, 103586 CrossRef CAS.
- N. Koukouzas, P. Koutsovitis, P. Tyrologou, C. Karkalis and A. Arvanitis, Potential for mineral carbonation of CO2 in Pleistocene basaltic rocks in Volos region (central Greece), Minerals, 2019, 9(10), 627 CrossRef CAS.
- C. M. White, D. H. Smith, K. L. Jones, A. L. Goodman, S. A. Jikich and R. B. LaCount,
et al., Sequestration of carbon dioxide in coal with enhanced coalbed methane recovery a review, Energy Fuels, 2005, 19(3), 659–724 CrossRef CAS.
- T. Ajayi, J. S. Gomes and A. Bera, A review of CO2 storage in geological formations emphasizing modeling, monitoring and capacity estimation approaches, Pet. Sci., 2019, 16(5), 1028–1063 CrossRef CAS.
-
A. Bui, The Feasibility of Implementing Carbon Capture and Storage at Yale, Department of Geology and Geophysics, Yale University, 2017 Search PubMed.
- A. M. Lacinska, M. T. Styles, K. Bateman, M. Hall and P. D. Brown, An experimental study of the carbonation of serpentinite and partially serpentinised peridotites, Front Earth Sci., 2017, 5, 37 CrossRef.
- A. Gambhir and M. Tavoni, Direct air carbon capture and sequestration: how it works and how it could contribute to climate-change mitigation, One Earth, 2019, 1(4), 405–409 CrossRef.
- A. Babin, C. Vaneeckhaute and M. C. Iliuta, Potential and challenges of bioenergy with carbon capture and storage as a carbon-negative energy source: A review, Biomass Bioenergy, 2021, 146, 105968 CrossRef CAS.
- W. R. Turner, Looking to nature for solutions, Nat. Clim. Change, 2018, 8(1), 18–19 CrossRef.
- Y. Weng, W. Cai and C. Wang, Evaluating the use of BECCS and afforestation under China's carbon-neutral target for 2060, Appl. Energy, 2021, 299, 117263 CrossRef.
- L. T. Bach, V. Tamsitt, J. Gower, C. L. Hurd, J. A. Raven and P. W. Boyd, Testing the climate intervention potential of ocean afforestation using the Great Atlantic Sargassum Belt, Nat. Commun., 2021, 12(1), 1–10 CrossRef PubMed.
- I. Ménard, E. Thiffault, Y. Boulanger and J.-F. Boucher, Multi-model approach to integrate climate change impact on carbon sequestration potential of afforestation scenarios in Quebec, Canada, Ecol. Modell., 2022, 473, 110144 CrossRef.
- E. J. Forster, J. R. Healey, C. Dymond and D. Styles, Commercial afforestation can deliver effective climate change mitigation under multiple decarbonisation pathways, Nat. Commun., 2021, 12(1), 1–12 CrossRef PubMed.
- Y. Xiang, Y. Li, X. Luo, Y. Liu, P. Huang and B. Yao,
et al., Mixed plantations enhance more soil organic carbon stocks than monocultures across China: Implication for optimizing afforestation/reforestation strategies, Sci. Total Environ., 2022, 821, 153449 CrossRef CAS PubMed.
- C. Chen, J. F. K. Kotyk and S. W. Sheehan, Progress toward commercial application of electrochemical carbon dioxide reduction, Chem, 2018, 4(11), 2571–2586 CAS.
- L. Ricciardi, P. D'Odorico, N. Galli, D. D. Chiarelli and M. C. Rulli, Hydrological implications of large-scale afforestation in tropical biomes for climate change mitigation, Philos. Trans. R. Soc., B, 2022, 377(1857), 20210391 CrossRef PubMed.
- D. Yumashev, V. Janes-Bassett, J. W. Redhead, E. Rowe and J. A. C. Davies, Terrestrial carbon sequestration under future climate, nutrient and land use change and management scenarios: a national-scale UK case study, Environ. Res. Lett., 2022, 17(11), 114054 CrossRef.
- S. Paul, S. Bera, R. Dasgupta, S. Mondal and S. Roy, Review on the recent structural advances in open and closed systems for carbon capture through algae, Energy Nexus, 2021, 4, 100032 CrossRef CAS.
- C. B. Field, M. J. Behrenfeld, J. T. Randerson and P. Falkowski, Primary production of the biosphere: integrating terrestrial and oceanic components, Science, 1998, 281(5374), 237–240 CrossRef CAS PubMed.
- Y. Y. Choi, A. K. Patel, M. E. Hong, W. S. Chang and S. J. Sim, Microalgae Bioenergy with Carbon Capture and Storage (BECCS): An emerging sustainable bioprocess for reduced CO2 emission and biofuel production, Bioresour. Technol. Rep., 2019, 7, 100270 CrossRef.
-
S. O'Brien, 7 Surprising Health Benefits of Eating Eeaweed, 2018 [cited 2022 Jul 6]. Available from: https://www.healthline.com/nutrition/benefits-of-seaweed Search PubMed.
- S. Spillias, R. S. Cottrell, R. Kelly, K. R. O'Brien, J. Adams and A. Bellgrove,
et al., Expert perceptions of seaweed farming for sustainable development, J. Clean Prod., 2022, 133052 CrossRef.
- Y. K. Leong, K. W. Chew, W.-H. Chen, J.-S. Chang and P. L. Show, Reuniting the biogeochemistry of algae for a low-carbon circular bioeconomy, Trends Plant Sci., 2021, 26(7), 729–740 CrossRef CAS PubMed.
- M. Bertolini and F. Conti, Capture, Storage and Utilization of Carbon Dioxide by Microalgae and Production of Biomaterials, Rigas Teh Univ Zinat Raksti, 2021, 25(1), 574–586 CAS.
- L. Ou, S. Banerjee, H. Xu, A. M. Coleman, H. Cai and U. Lee,
et al., Utilizing high-purity carbon dioxide sources for algae cultivation and biofuel production in the United States: Opportunities and challenges, J. Clean Prod., 2021, 321, 128779 CrossRef CAS.
- A. Sarwer, S. M. Hamed, A. I. Osman, F. Jamil, A. H. Al-Muhtaseb and N. S. Alhajeri,
et al., Algal biomass valorization for biofuel production and carbon sequestration: a review, Environ. Chem. Lett., 2022, 1–55 Search PubMed.
- B. Minasny, B. P. Malone, A. B. McBratney, D. A. Angers, D. Arrouays and A. Chambers,
et al., Soil carbon 4 per mille, Geoderma, 2017, 292, 59–86 CrossRef.
- C. Werner, H.-P. Schmidt, D. Gerten, W. Lucht and C. Kammann, Biogeochemical potential of biomass pyrolysis systems for limiting global warming to 1.5 C, Environ. Res. Lett., 2018, 13(4), 44036 CrossRef.
- G. Singh, J. Lee, R. Bahadur, A. Karakoti, J. Yi and A. Vinu, Highly Graphitized Porous Biocarbon Nanosheets with Tunable Micro-Meso Interfaces and Enhanced Layer Spacing for CO2 Capture and LIBs, Chem. Eng. J., 2022, 134464 CrossRef CAS.
- A. E. Creamer, B. Gao and M. Zhang, Carbon dioxide capture using biochar produced from sugarcane bagasse and hickory wood, Chem. Eng. J., 2014, 249, 174–179 CrossRef CAS.
- K. K. Kishibayev, J. Serafin, R. R. Tokpayev, T. N. Khavaza, A. A. Atchabarova and D. A. Abduakhytova,
et al., Physical and chemical properties of activated carbon synthesized from plant wastes and shungite for CO2 capture, J. Environ. Chem. Eng., 2021, 9(6), 106798 CrossRef CAS.
- M. Danish and T. Ahmad, A review on utilization of wood biomass as a sustainable precursor for activated carbon production and application, Renew. Sustain. Energy Rev., 2018, 87, 1–21 CrossRef CAS.
- C. Quan, X. Jia and N. Gao, Nitrogen-doping activated biomass carbon from tea seed shell for CO2 capture and supercapacitor, Int. J. Energy Res., 2020, 44(2), 1218–1232 CrossRef CAS.
- G. Singh, R. Bahadur, J. M. Lee, I. Y. Kim, A. M. Ruban and J. M. Davidraj,
et al., Nanoporous activated biocarbons with high surface areas from alligator weed and their excellent performance for CO2 capture at both low and high pressures, Chem. Eng. J., 2021, 406, 126787 CrossRef CAS.
- R. M. Cuéllar-Franca and A. Azapagic, Carbon capture, storage and utilisation technologies: A critical analysis and comparison of their life cycle environmental impacts, J. CO2 Util., 2015, 9, 82–102 CrossRef.
- M. Bernardo, N. Lapa, I. M. Fonseca and I. A. A. C. Esteves, Biomass Valorization to Produce Porous Carbons: Applications in CO2 Capture and Biogas Upgrading to Biomethane—A Mini-Review, Front Energy Res., 2021, 9, 625188 CrossRef.
- L. Riboldi and O. Bolland, Overview on pressure swing adsorption (PSA) as CO2 capture technology: state-of-the-art, limits and potentials, Energy Procedia, 2017, 114, 2390–2400 CrossRef CAS.
- R. L. S. Canevesi, K. A. Andreassen, E. A. da Silva, C. E. Borba and C. A. Grande, Pressure swing adsorption for biogas upgrading with carbon molecular sieve, Ind. Eng. Chem. Res., 2018, 57(23), 8057–8067 CrossRef CAS.
-
D. Rassool, C. Consoli, A. Townsend and H. Liu, Overview of Organisations and Policies Supporting the Deployment of Large-Scale CCS Facilities, Glob CCS Inst Washington, DC, USA, 2020 Search PubMed.
- B. M. Swallow and T. W. Goddard, Value chains for bio-carbon sequestration services: Lessons from contrasting cases in Canada, Kenya and Mozambique, Land use policy, 2013, 31, 81–89 CrossRef.
- K. Zhang, H. C. Lau, S. Liu and H. Li, Carbon capture and storage in the coastal region of China between Shanghai and Hainan, Energy, 2022, 247, 123470 CrossRef CAS.
-
Global-CCS-Institute, Global Status Report 2021: Policy Fact Sheet, 2022 [cited 2022 Jun 8]. Available from: https://www.globalccsinstitute.com/wp-content/uploads/2021/10/GSR2021_Policy-Factsheet_2021_EN.pdf Search PubMed.
- J.-K. S. Roettereng, How the global and national levels interrelate in climate policymaking: Foreign Policy Analysis and the case of Carbon Capture Storage in Norway's foreign policy, Energy Policy, 2016, 97, 475–484 CrossRef.
- K. Jiang, P. Ashworth, S. Zhang, X. Liang, Y. Sun and D. Angus, China's carbon capture, utilization and storage (CCUS) policy: A critical review, Renew. Sustain. Energy Rev., 2020, 119, 109601 CrossRef.
- A. Ishii and O. Langhelle, Toward policy integration: Assessing carbon capture and storage policies in Japan and Norway, Glob. Environ. Change, 2011, 21(2), 358–367 CrossRef.
- R. W. J. Edwards and M. A. Celia, Infrastructure to enable deployment of carbon capture, utilization, and storage in the United States, Proc. Natl. Acad. Sci., 2018, 115(38), E8815–E8824 CrossRef CAS PubMed.
- N. Wang, K. Akimoto and G. F. Nemet, What went wrong? Learning from three decades of carbon capture, utilization and sequestration (CCUS) pilot and demonstration projects, Energy Policy, 2021, 158, 112546 CrossRef CAS.
- N. Zhang, Z. Pan, Z. Zhang, W. Zhang, L. Zhang and F. M. Baena-Moreno,
et al., CO2 capture from coalbed methane using membranes: a review, Environ. Chem. Lett., 2020, 18(1), 79–96 CrossRef CAS.
- C. J. Quarton and S. Samsatli, The value of hydrogen and carbon capture, storage and utilisation in decarbonising energy: Insights from integrated value chain optimisation, Appl. Energy, 2020, 257, 113936 CrossRef CAS.
- S. Pianta, A. Rinscheid and E. U. Weber, Carbon Capture and Storage in the United States: Perceptions, preferences, and lessons for policy, Energy Policy, 2021, 151, 112149 CrossRef CAS.
- P. Tcvetkov, A. Cherepovitsyn and S. Fedoseev, Public perception of carbon capture and storage: A state-of-the-art overview, Heliyon, 2019, 5(12), e02845 CrossRef PubMed.
- L. Whitmarsh, D. Xenias and C. R. Jones, Framing effects on public support for carbon capture and storage, Palgrave Commun., 2019, 5(1), 1–10 CrossRef.
- V. Becattini, P. Gabrielli and M. Mazzotti, Role of carbon capture, storage, and utilization to enable a net-zero-CO2-emissions aviation sector, Ind. Eng. Chem. Res., 2021, 60(18), 6848–6862 CrossRef CAS.
-
K. Dubin, Petra Nova Is One of Two Carbon Capture and Sequestration Power Plants in the World, 2017 [cited 2022 Jun 8]. Available from: https://www.eia.gov/todayinenergy/detail.php?id=33552 Search PubMed.
- S. Tian, J. Jiang, Z. Zhang and V. Manovic, Inherent potential of steelmaking to contribute to decarbonisation targets via industrial carbon capture and storage, Nat. Commun., 2018, 9(1), 1–8 CrossRef PubMed.
- W. F. Lamb, T. Wiedmann, J. Pongratz, R. Andrew, M. Crippa and J. G. J. Olivier,
et al., A review of trends and drivers of greenhouse gas emissions by sector from 1990 to 2018, Environ. Res. Lett., 2021, 16(7), 73005 CrossRef CAS.
- A. W. Zimmermann, J. Wunderlich, L. Müller, G. A. Buchner, A. Marxen and S. Michailos,
et al. Techno-economic assessment guidelines for CO2 utilization, Front Energy Res., 2020, 5 CrossRef.
- D. Sawyer, R. Harding, C. Pozlott, P. Dickey, R. Harding and C. Pozlott, Carbon capture and storage—the environmental and economic case and challenges, Int Inst Sustain Dev Univ Calgary Inst Sustain Energy, Environ Econ Pembin Inst., 2008, 1–26 Search PubMed.
- C. Antonini, K. Treyer, A. Streb, M. van der Spek, C. Bauer and M. Mazzotti, Hydrogen production from natural gas and biomethane with carbon capture and storage–A techno-environmental analysis, Sustain. Energy Fuels, 2020, 4(6), 2967–2986 RSC.
- C. Fernández-Dacosta, M. Van Der Spek, C. R. Hung, G. D. Oregionni, R. Skagestad and P. Parihar,
et al., Prospective techno-economic and environmental assessment of carbon capture at a refinery and CO2 utilisation in polyol synthesis, J. CO2 Util., 2017, 21, 405–422 CrossRef.
- W. Schakel, G. Oreggioni, B. Singh, A. Strømman and A. Ramírez, Assessing the techno-environmental performance of CO2 utilization via dry reforming of methane for the production of dimethyl ether, J. CO2 Util., 2016, 16, 138–149 CrossRef CAS.
- L. Petrescu and C.-C. Cormos, Environmental assessment of IGCC power plants with pre-combustion CO2 capture by chemical & calcium looping methods, J. Clean Prod., 2017, 158, 233–244 CrossRef CAS.
- A. Lichtschlag, C. R. Pearce, M. Suominen, J. Blackford, S. M. Borisov and J. M. Bull,
et al., Suitability analysis and revised strategies for marine environmental carbon capture and storage (CCS) monitoring, Int. J. Greenhouse Gas Control, 2021, 112, 103510 CrossRef CAS.
- R. P. Hepple and S. M. Benson, Geologic storage of carbon dioxide as a climate change mitigation strategy: performance requirements and the implications of surface seepage, Environ. Geol., 2005, 47(4), 576–585 CrossRef CAS.
- P. M. Haugan and F. Joos, Metrics to assess the mitigation of global warming by carbon capture and storage in the ocean and in geological reservoirs, Geophys.
Res. Lett., 2004, 31(18), L18202 CrossRef.
- S. A. Monk, A. Schaap, R. Hanz, S. M. Borisov, S. Loucaides and M. Arundell,
et al., Detecting and mapping a CO2 plume with novel autonomous pH sensors on an underwater vehicle, Int. J. Greenhouse Gas Control, 2021, 112, 103477 CrossRef CAS.
- S. E. Tanzer, K. Blok and A. Ramírez, Can bioenergy with carbon capture and storage result in carbon negative steel?, Int. J. Greenhouse Gas Control, 2020, 100, 103104 CrossRef CAS.
- P. Withey, C. Johnston and J. Guo, Quantifying the global warming potential of carbon dioxide emissions from bioenergy with carbon capture and storage, Renew. Sustain. Energy Rev., 2019, 115, 109408 CrossRef CAS.
-
H. Herzog, K. Smekens, P. Dadhich, J. Dooley, Y. Fujii, O. Hohmeyer, et al., Cost and Economic Potential, 2005 Search PubMed.
- Y. Yang, Q. Zhang, H. Yu and X. Feng, Tech-economic and environmental analysis of energy-efficient shale gas and flue gas coupling system for chemicals manufacture and carbon capture storage and utilization, Energy, 2021, 217, 119348 CrossRef CAS.
- I. L. Wiesberg, J. L. de Medeiros, R. V. P. de Mello, J. G. S. S. Maia, J. B. V. Bastos and F. A. Ofélia de Queiroz, Bioenergy production from sugarcane bagasse with carbon capture and storage: Surrogate models for techno-economic decisions, Renew. Sustain. Energy Rev., 2021, 150, 111486 CrossRef CAS.
- S. Zhang, L. Liu, L. Zhang, Y. Zhuang and J. Du, An optimization model for carbon capture utilization and storage supply chain: A case study in Northeastern China, Appl. Energy, 2018, 231, 194–206 CrossRef CAS.
- E. Smith, J. Morris, H. Kheshgi, G. Teletzke, H. Herzog and S. Paltsev, The cost of CO2 transport and storage in global integrated assessment modeling, Int. J. Greenhouse Gas Control, 2021, 109, 103367 CrossRef.
- B. Hu and H. Zhai, The cost of carbon capture and storage for coal-fired power plants in China, Int. J. Greenhouse Gas Control, 2017, 65, 23–31 CrossRef.
- B. Singh, A. H. Strømman and E. G. Hertwich, Comparative life cycle environmental assessment of CCS technologies, Int. J. Greenhouse Gas Control, 2011, 5(4), 911–921 CrossRef CAS.
- B. Singh, A. H. Strømman and E. Hertwich, Life cycle assessment of natural gas combined cycle power plant with post-combustion carbon capture, transport and storage, Int. J. Greenhouse Gas Control, 2011, 5(3), 457–466 CrossRef CAS.
- X. Liang, Z. Wang, Z. Zhou, Z. Huang, J. Zhou and K. Cen, Up-to-date life cycle assessment and comparison study of clean coal power generation technologies in China, J. Clean Prod., 2013, 39, 24–31 CrossRef CAS.
- M. Corsten, A. Ramírez, L. Shen, J. Koornneef and A. Faaij, Environmental impact assessment of CCS chains–lessons learned and limitations from LCA literature, Int. J. Greenhouse Gas Control, 2013, 13, 59–71 CrossRef CAS.
- S. Michailos, O. Emenike, D. Ingham, K. J. Hughes and M. Pourkashanian, Methane production via syngas fermentation within the bio-CCS concept: A techno-economic assessment, Biochem. Eng. J., 2019, 150, 107290 CrossRef CAS.
- I. S. Tagomori, P. R. R. Rochedo and A. Szklo, Techno-economic and georeferenced analysis of forestry residues-based Fischer-Tropsch diesel with carbon capture in Brazil, Biomass Bioenergy, 2019, 123, 134–148 CrossRef CAS.
- X. Xing, R. Wang, N. Bauer, P. Ciais, J. Cao and J. Chen,
et al., Spatially explicit analysis identifies significant potential for bioenergy with carbon capture and storage in China, Nat. Commun., 2021, 12(1), 1–12 CrossRef PubMed.
- B. Lin and Z. Tan, How much impact will low oil price and carbon trading mechanism have on the value of carbon capture utilization and storage (CCUS) project? Analysis based on real option method, J. Clean Prod., 2021, 298, 126768 CrossRef CAS.
- C. Liu, S. Sang, X. Fan, K. Zhang, F. Song and X. Cui,
et al., Influences of pressures and temperatures on pore structures of different rank coals during CO2 geological storage process, Fuel, 2020, 259, 116273 CrossRef CAS.
-
E. A. Al-Khdheeawi, D. S. Mahdi, M. Ali, S. Iglauer and A. Barifcani, Reservoir scale porosity-permeability evolution in sandstone due to CO2 geological storage, in Proceedings of the 15th Greenhouse Gas Control Technologies Conference, 2021, pp. 15–8 Search PubMed.
- H. Zheng, S. Cao, W. Yuan, Q. Jiang, S. Li and G. Feng, A Time-Dependent Hydro-mechanical Coupling Model of Reservoir Sandstone During CO2 Geological Storage, Rock Mech. Rock Eng., 2022, 1–17 Search PubMed.
- P. K. Gupta and B. Yadav, Leakage of CO2 from geological storage and its impacts on fresh soil–water systems: a review, Environ. Sci. Pollut. Res., 2020, 27(12), 12995–13018 CrossRef CAS PubMed.
- A. Fatah, Z. Bennour, H. B. Mahmud, R. Gholami and M. Hossain, Surface wettability alteration of shales exposed to CO2: Implication for long-term integrity of geological storage sites, Int. J. Greenhouse Gas Control, 2021, 110, 103426 CrossRef CAS.
- V. Heck, D. Gerten, W. Lucht and A. Popp, Biomass-based negative emissions difficult to reconcile with planetary boundaries, Nat. Clim. Change, 2018, 8(2), 151–155 CrossRef CAS.
- W. F. Lamb, M. Grubb, F. Diluiso and J. C. Minx, Countries with sustained greenhouse gas emissions reductions: an analysis of trends and progress by sector, Clim. Policy, 2022, 22(1), 1–17 CrossRef.
-
D. Rassool, C. Consoli, A. Townsend and H. Liu, Overview of Organizations and Policies Supporting the Deployment of Large-Scale CCS Facilities, Global CCS Institute, Washington, DC, USA, 2020 Search PubMed.
- Y. Sun, Y. Li, B. Cai and Q. Li, Comparing the explicit and implicit attitudes of energy stakeholders and the public towards carbon capture and storage, J. Clean Prod., 2020, 254, 120051 CrossRef CAS.
- L. Waller, T. Rayner and J. Chilvers, Searching for a public in controversies over carbon dioxide removal: An Issue Mapping Study on BECCS and afforestation, Sci. Technol. Hum. Values, 2021, 01622439211043568 Search PubMed.
|
This journal is © The Royal Society of Chemistry 2023 |
Click here to see how this site uses Cookies. View our privacy policy here.