DOI:
10.1039/D2SM01481E
(Review Article)
Soft Matter, 2023,
19, 1282-1292
Recent progress in emulsion gels: from fundamentals to applications
Received
10th November 2022
, Accepted 23rd January 2023
First published on 23rd January 2023
Abstract
Emulsion gels, also known as gelled emulsions or emulgels, have garnered great attention both in fundamental research and practical applications due to their superior stability, tunable morphology and microstructure, and promising mechanical and functional properties. From an application perspective, attention in this area has been, historically, mainly focused on food industries, e.g., engineering emulsion gels as fat substitutes or delivery systems for bioactive food ingredients. However, a growing body of studies has, in recent years, begun to demonstrate the full potential of emulsion gels as soft templates for designing advanced functional materials widely applied in a variety of fields, spanning chemical engineering, pharmaceutics, and materials science. Herein, a concise and comprehensive overview of emulsion gels is presented, from fundamentals to applications, highlighting significant recent progress and open questions, to scout for and deepen their potential applications in more fields.
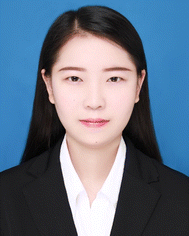
Chuchu Wan
| Chuchu Wan is currently a PhD student at the School of Chemistry and Chemical Engineering, Huazhong University of Science and Technology, China; prior to this, she received her BS degree from the School of Chemistry and Chemical Engineering, Southwest University, China. Her research focuses on building bionic liquid models via liquid–liquid interfacial assembly of nanoparticles. |
1. Introduction
Emulsions are biphasic systems of one liquid dispersed as fine droplets throughout another immiscible liquid usually by a mechanical agitation process. They have been widely utilized in foods, pharmaceuticals, cosmetics, and personal care products owing to their encapsulation ability of the desired ingredients in the dispersed phase. Nevertheless, emulsions are mostly thermodynamically unstable and tend to revert into separate bulk liquids over time via various physicochemical mechanisms such as gravitational separation, coalescence, flocculation, and Ostwald ripening, which restricts their widespread applications.1
The transformation of emulsions into emulsion gels to improve their stability is, therefore, becoming an emerging trend. Emulsion gels are soft-solid-like materials consisting of emulsified droplets trapped in a three-dimensional cross-linked polymer network (i.e., emulsion-filled gels) or a network of aggregated emulsified droplets (i.e., emulsion particulate gels).2 They combine the benefits of both emulsions and gels, not only having the ability to deliver desired ingredients but also possessing superior thermodynamic stability and tunable viscoelastic properties.3,4 Consequently, functional ingredients (e.g., polyphenols, vitamins, flavors, etc.) incorporated into emulsion gels exhibit outstanding stability against adverse environments due to the barrier effect of the dense gel network, before performing their tasks. Moreover, the microstructures and rheological properties of emulsion gels can be tailored by modulating the concentration of gelling agents, the volume fraction of the dispersed phase, and gelation triggers (e.g., heat, pH, enzyme, and salt), making them an ideal release-controlled carrier for sensitive bioactive components.2,5 In addition, emulsion gels have also been commonly used in low-fat products owing to their soft-solid-like textures, adjustable properties, and reduced rate of fat lipolysis.6 With the ever-increasing growth and enormous potential of emulsion gels in pharmaceutics and materials science, especially in recent years, it is quite necessary to systematically review this field from the fundamental aspects, from structural characteristics, preparation methods, and structure–property relationships, to applications, such as fat replacement, drug delivery, and functional materials synthesis, highlighting significant recent progress and challenges.
Many of the above subjects are covered separately in several review articles. The basic principles and practices underlying the preparation and characterization of emulsion gels have already been discussed in depth in a study by Dickinson.2 Farjami et al. described the main factors that influence the rheological properties and functionalities of emulsion gel systems.1 From an application perspective, Lu et al. provided a comprehensive overview of the studies of engineering emulsion gels as the delivery systems for functional food ingredients.6 Ren et al. focused specifically on the applications of emulsion gels as fat substitutes in meat products.7 Food 3D printing of emulsion gels has also been discussed by Li et al.8 Our aim here is to present the connections between these subjects, from fundamentals to applications. The current review is initiated with the gelation mechanisms, structures, and mechanical properties of different emulsion gel systems reported in the literature. Then, the preparation methods of different emulsion gel systems are addressed, paying particular attention to the advantages and drawbacks of each method. The effects of the preparation methods and formulations on the gel structures and then the rheological properties are also briefly covered. Afterward, the recent advances in the applications of emulsion gel systems in multiple industries are systematically summarized, including foods, pharmaceuticals, cosmetics, and materials science. Most importantly, we cover recent successes in designing advanced functional materials (e.g., thermal insulation, conductivity, cleaning, etc.) using molding and 3D printing. Finally, the prospects and challenges are also presented to predict the development tendency of emulsion gels.
2. Classification
2.1. Emulsion-filled gels
Emulsion-filled gels consist of emulsified droplets, as fillers within a continuous gel matrix. The droplets can be categorized as either active or inactive fillers, according to the interactions between the emulsified droplets and the gel matrix. Active fillers, as illustrated in Fig. 1a, whose interfacial layers interact with the continuous gel body via covalent and/or noncovalent bonds, can markedly strengthen the gel network and improve the mechanical properties of emulsion-filled gels.1 In practice, the Kerner model is often used to explain the effect of active fillers on the mechanical properties of emulsion-filled gels.8,9 When the fillers are more rigid than the gel matrix, this model expresses the increase in the shear modulus G of the emulsion-filled gel in terms of the ratio of the shear moduli of the fillers (Gf) and matrix (Gm): |  | (1) |
For an emulsion-filled gel containing monodisperse droplets of radius r with the interfacial tension γ, Gf is given by |  | (2) |
When the active fillers are evenly distributed in the emulsion-filled gel, the shear modulus of the emulsion-filled gel (G) is given by |  | (3) |
where vm is the Poisson's ratio of the gel matrix, and ϕ is the actual droplet volume fraction. The vm of the incompressible matrix is 0.5. In contrast, inactive fillers show little affinity to the gel matrix and behave like small holes in a composite material (Fig. 1b).10 Therefore, they may hinder the homogeneity of the gel network and reduce the gel rigidity, especially when small-molecule surfactants are utilized as emulsifiers.11,12
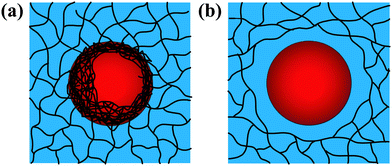 |
| Fig. 1 Schematic representation of two emulsion-filled gels. (a) Emulsified droplets act as active fillers. (b) Emulsified droplets act as inactive fillers. | |
In general, rheological analysis is often used to characterize the mechanical properties of emulsion gels, which mainly focus on the dynamic modulus (i.e., storage modulus and loss modulus), apparent viscosity, and yield stress under different stress, strain, frequency, or shear rates. The rheological properties and functional characteristics of the emulsion-filled gel systems are primarily determined by the gelling agents in the continuous phase.5 The gelling agents, therein, are usually the biopolymer molecules, such as proteins (e.g., gelatin,13 caseins,14,15 whey16–19 and soy proteins20) and polysaccharides (e.g., starch,21–23 inulin,24 alginate25 and pectin26), due to their fascinating emulsifying and gelling capacities. These biopolymers often illustrate rapid thermo-reversible and shear-thinning behavior in solution.13,17,21,27 On the one hand, they are easily dissolved in water when heated, presenting a random coil structure. During the cooling process, they undergo a conformational transition from a flexible and disordered structure to a rigid ordered state. Then, the cross-linking degree between these extensible biopolymers is greatly increased via the electrostatic interaction, hydrogen bonding, and so on. This results in the formation of a compact and firm gel structure when embedding an emulsion into this pre-gel biopolymer solution. And, the storage modulus of the resultant emulsion-filled gel is usually at least one magnitude higher than the loss modulus. On the other hand, these biopolymer solutions are highly pseudoplastic with a strong shear-thinning and rapid shear recovery behavior. Viscosity rapidly reduces when shear stress increases, but upon the removal of shear force, the initial viscosity is recovered almost immediately. This is because these natural macromolecules can form intermolecular aggregates through hydrogen bonding and polymer entanglements in solution. The highly ordered network of entangled and stiff molecules presents high viscosity at low shear stress, while the structure is disrupted rapidly when high shear stress is applied. The produced emulsion-filled gels maintain this shear-thinning characteristic as well. It is noteworthy that the mechanical properties of emulsion-filled gels are also strongly affected by the volume fraction of the dispersed phase, droplet size, matrix properties, droplet–matrix interactions, gelling methods, and interfacial aging.1,2,5 For instance, it has been reported that increasing the protein content in an emulsion-filled protein gel led to a stronger gel strength, which was attributed to the formation of a more compact gel network.5 Thus, emulsion-filled gels with the desired rheological properties, like shear-thinning behavior which is desirable during extrusion and possesses sufficient mechanical strength to withstand the layer stacking after extrusion, are suitable as soft matter systems for application in 3D printing.8,27 Furthermore, these biopolymer-based emulsion-filled gels are ideal model systems for investigating the mechanistic relationships between food structure/rheology and food sensory attributes, as well as the on-skin delivery and spreading of viscoelastic cosmetic formulations.8
2.2. Emulsion particulate gels
In emulsion particulate gels, the dispersed droplets stabilized by colloidal particles aggregate together which, potentially, may completely occupy the available volume, leading to a gel (Fig. 2). Formation of such emulsion gels involves the ‘switching on’ of cross-links and associative interactions between the adsorbed particles of adjacent emulsion droplets via some external triggers (heating, acidification, enzyme treatment, etc.).2 Their mechanical properties are mainly determined by the interaction force between the particles and the deformability of the particles.1 The aggregation of emulsion particulate gels can be classified into irreversible aggregation, reversible aggregation, and heteroaggregation, according to the nature and strength of the interactions between the droplets.1 The irreversible aggregation of particles with infinitely stiff interparticle bonds strongly enhances the strength and stiffness of the network structure and thus promotes gelation. In practice, however, emulsion droplets stick together by reversible bonding to form a weak particulate gel network in systems containing a high-volume fraction of the dispersed phase.1,9 The Kerner model modified by Lewis and Nielsen can be used to explain the effect of active fillers on the mechanical properties of emulsion particulate gels: |  | (4) |
where ψϕ is the effective volume fraction of fillers, which considers the crowding effect of fillers and can be obtained as follows: | 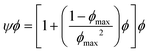 | (5) |
where ϕmax is the maximum volume fraction of the fillers. According to eqn (4), increasing ψϕ or ϕ can enhance the mechanical properties of emulsion particulate gels. In addition, the heteroaggregation of particles of different types can form emulsion particulate gels with new structural characteristics and mechanical properties.8 Most importantly, these unique microstructures can improve the viscosity and paste-like characteristics of emulsion particulate gels, thereby providing ideas for food 3D printing.8
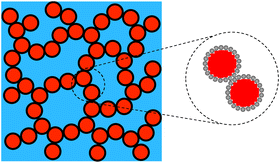 |
| Fig. 2 Schematic representation of emulsion particulate gels. | |
3. Fabrication methods
3.1. Emulsion-filled gels
Conventionally, emulsion-filled gels are formed by embedding an emulsion into either a gel matrix or a pre-gel polymer solution followed by in situ gelation.28 Methods for preparing emulsion-filled gels include heat-induced, enzyme-induced, acid-induced, salt-induced, and so on, according to the gelation process. The selection of these methods depends on the structures and properties of the gel matrix and the requirements of the resultant emulsion gels.
3.1.1. Heat-induced.
Much research has been devoted to the development of heat-induced emulsion-filled gels, as they are one of the simplest and most readily implementable gelation systems.29–31 For heat-induced gelation, the emulsion is first heated and then rapidly cooled to form a gel. The heat enables the unfolding and denaturation of the protein molecules and the exposure of more sulfhydryl groups and hydrophobic groups, which can further self-assemble into three-dimensional network structures through chemical forces (i.e., intermolecular disulfide bonds, electrostatic interactions, hydrophobic interactions, and hydrogen bonds), along with the emulsified droplets are fixed in the network.30 Liu et al. produced whey protein concentrate (WPC)-stabilized emulsion-filled gels with oil fractions ranging from 20% to 60% via a heat pretreatment of WPC dispersions at 70 °C for 30 min, followed by microfluidization (Fig. 3).17 All the emulsions obtained from the heated WPC exhibited remarkably a higher elastic modulus (G′) than loss modulus (G″) for all the frequencies tested, which is a signature of elastic gel networks. Geremias-Andrade and coworkers prepared heat-set emulsion-filled gels by mixing whey protein isolate (WPI) with xanthan gum (XG) as the gel matrix and using solid lipid particles (SLM) as the fillers, showing that the structural and rheological properties of the gels could be tailored by varying the concentration of the lipid particle dispersions.18 In the system with a higher surfactant concentration (4%), the lower oil content weakened the gel strength, while the higher content strengthened the gel network. These results indicated that in these mixed gel systems, oil droplets with lower volume acted as inactive fillers and while those with higher volume were acted as active fillers. The gel structure formed by heat-induced gelation was also closely related to ionic strength,32 pH,33 heating conditions (i.e., duration or temperature)34,35 and emulsifiers.36 While heat-induced gelation is simple, well-studied, and easy to carry out without changing the volume or composition of the emulsion system, it is inappropriate to deliver thermosensitive bioactives.
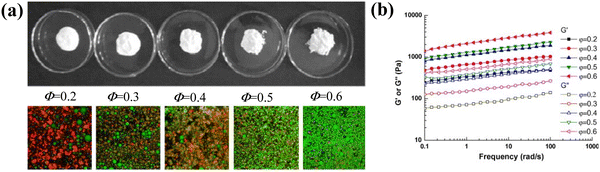 |
| Fig. 3 (a) Visual images of heat-treated WPC emulsions at various oil fractions (Φ = 0.2–0.6) after 1 day storage at room temperature. The bottom row shows the confocal laser scanning microscopy images of the emulsions. The dimension for the images is 7.5 × 7.5 µm. (b) Variations of G′ and G″ with frequency at 25 °C for the heat-treated WPC emulsions at various oil fractions (Φ = 0.2–0.6).17 Copyright 2011 Elsevier. | |
3.1.2. Enzyme-induced.
Enzyme induction, as a mild and controllable way to prepare emulsion-filled gels, gives rise to stiff and elastic gels without any by-products.37,38 Microbial transglutaminase (MTG), a class of transferase that can catalyze the cross-linking reaction between glutamine and lysine residues and promote cross-links between protein molecules to form a network structure, is commonly used in this method.39–41 Herrero's group developed emulsion-filled gels composed of a mixture of sodium caseinate and MTG, discovering that the enzymatic action of MTG induced greater protein structural changes and improved the gel strength, in comparison with the pure protein emulsifying system in aqueous solution.15 In other work, Tang et al. reported that adding the enzyme prior to the emulsification allowed a pre-incubation of the proteins with the enzyme, which dramatically improved the stiffness of the MTG-set soy protein isolate (SPI)-stabilized emulsion-filled gels.42 Additionally, emulsion concentrations,43 oil fractions,44 and protein composition45 demonstrate remarkable influences on the microstructures and properties of enzyme-induced emulsion gels. Compared with the heat-induced emulsion gels, these enzyme-induced emulsion gels can not only reduce the lipid oxidation of n-3 fatty acids during preheating but also provide extra protection for heat-sensitive substances,41,46,47 making them ideal for the processing of low-fat meat products. It should be noted, however, that the chemical composition of the system has changed with the addition of enzymes.
3.1.3. Acid-induced.
Acid-induced gelation is widely utilized in the food industry, particularly for making fermented dairy products like yogurt.48 The process usually involves two steps: (1) the unfolding and denaturation of the protein molecules by heat treatment; (2) the formation of a gel network via intermolecular cross-linking of proteins by virtue of lowering the pH below the isoelectric point of the proteins and reducing the electrostatic repulsion between proteins.49 According to Venugopal and coworkers, the glacial acetic acid (HAc)-induced gelation was a slow process at room temperature, the rate of which could be increased by mildly heating the acidified protein, while heat alone did not cause gelation.50 Likewise, Rosa et al. prepared WPI-stabilized emulsion-filled gels by adding Glucono-δ-lactone (GDL, a gluconic acid precursor) to reactive WPI aggregate solution to reach a final pH of around 4.8. Moreover, raising WPI/oil concentrations resulted in a stronger network as the dispersed oil droplets acted as active fillers.51 The effects of oil types and acidification temperature on the properties of acidified emulsion gels were also evaluated.52,53 However, there are still a few limitations on the application of this acid-induced gelation. For instance, the pre-heating procedure of this method makes it unsuitable for heat-sensitive compound encapsulation. Its inability to be applied in environments sensitive to pH, such as biological systems, is another limitation.
3.1.4. Salt-induced.
It has been proved that the addition of metallic ions (mostly calcium) was beneficial to the gelation of proteins efficiently.54,55 Three effects were hypothesized to cause calcium-induced gelation: (1) electrostatic repulsion, (2) hydrophobic or disulphide interactions and (3) calcium-bridging interactions between proteins by Lin et al.56 Furthermore, Guo et al. stated that gels prepared with lower calcium concentrations were softer and microstructurally homogeneous, whereas higher calcium concentrations led to rigid gels with dense protein aggregates.32 Moreover, types of salt ions,57 oil concentrations,58 thermal pretreatments,34,59 and the size and content of protein aggregates60 could affect the structures and mechanical properties of salt-induced emulsion gels.
3.1.5. Others.
Recently, a gelation mechanism triggered by the synergistic action of functionalized polymers onto the droplet interface,61,62 resulting in a packed three-dimensional structure with outstanding mechanical strength, has attracted increasing interest. Wu et al. created attractive Pickering emulsion gels (APEGs) by bridging neighboring carboxyl functionalized nanoparticle-stabilized water droplets through α,ω-diamino functionalized telechelic polymers in the continuous oil phase via the electrostatic interactions, as illustrated in Fig. 4.4 The resultant emulsion gel systems exhibited typical shear-thinning behaviors and the viscoelastic characteristics of which could be tailored by the pH, temperature, and molecular weight of the telechelic polymers, making them ideal for applications in various fields.
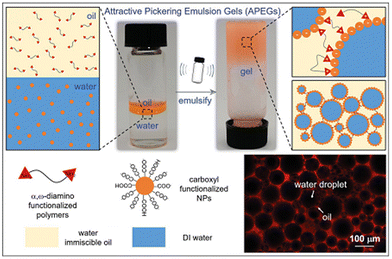 |
| Fig. 4 The formation mechanism and fluorescence confocal microscope image of APEGs.4 Copyright 2021 Wiley. | |
3.2. Emulsion particulate gels
In particle gel systems, emulsified droplets stick together by a collection of aggregated particles (i.e., emulsifiers), which jointly occupy the enclosing volume,1 ultimately forming large interconnected macroscopic networks. Emulsions with high volume fractions of the dispersed phase, such as high internal phase emulsions (HIPEs), may also form a weak and reversible particle gel network owing to the tightly connected emulsified droplets.1,63,64
In the system of the dispersed phase with low or moderate volume fraction, the liquid-like emulsion can be converted into an emulsion particulate gel via common physicochemical processes, such as heating, changing pH or ionic strength, since they can control the interparticle interactions at the oil–water interface layer to induce droplets aggregation to a high level. Wang and coworkers produced durable oil-in-water emulsion gels stabilized by insoluble chitosan/gelatin-B (CH/GB) complex particles with oil volume fraction from 0.1 to 0.4. They attributed the formation of the network structure to insoluble CH/GB complexes, which, under the oppositely charged pH (5 < pH < 6.5), strongly interacted with each other due to low electrostatic repulsion, thus resulting in droplet aggregation.65 Similarly, adding electrolytes (e.g., NaCl or CaCl2) or thermal treatment resulted in milk protein-based emulsion gels.66 It was found that the rheological properties of such particulate gel systems were significantly affected by the strength of interaction forces between the particles and the deformability of the particles. Usually, the weaker the deformation capacity of the particles, the lower the fracture strains of emulsion gels.1 In addition, excess particles in the continuous phase can further increase the elasticity of the emulsion particulate gel by forming a connected network of those excess particles themselves between dispersed droplets via van der Waals or other (e.g., hydrophobic) interactions.1,2,64,67 Chae et al. confirmed the presence of this elastic particle structure which connected the dispersed droplets well by adding salts to the continuous phase with various concentrations for controlling the attractive interaction between particles.64
Interestingly, particle bridging across two-fluid interfaces promotes a strategy for the fabrication of robust gel-like emulsions at low dispersed phase volume fractions.68,69 Lee et al. reported long-term stable emulsion gels stabilized by particles with an equilibrium three-phase contact angle of 135°, which served as a cohesive scaffold, bridging droplets together of the dispersed phase (Fig. 5). Remarkably, the rheological behavior was predominately mediated by the particle concentration and independent of the volume fraction of the dispersed phase.68 Similarly, Zhang's group generated bridged emulsion gels with partially hydrophobic layered double hydroxide (LDH) particles.70
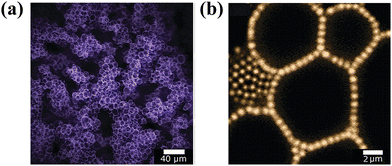 |
| Fig. 5 Confocal microscopy images of Pickering emulsion gels. (a) Low-magnification image showing tortuous clusters of droplets in the continuous fluid phase. (b) High-magnification image showing a monolayer of nearly touching particles bridging several faceted droplets in the gel interior.68 Copyright 2012 American Chemical Society. | |
High-internal-phase emulsions (HIPEs), commonly characterized by a minimum internal-phase volume fraction of 0.74,71 can also form stable gel-like networks due to the densely packed and aggregated droplets. HIPEs stabilized by solid colloidal particles, generally, exhibit fascinating advantages over those by surfactants, e.g., enhanced stabilization and lower environmental pollution risks.72,73 A variety of particles, either inorganic or organic, including silica particles,74,75 microgels,76 waterborne polyurethane,77 protein-based particles,78–80 polysaccharide-based particles,81–83 and protein–polysaccharide complexed particles84,85 have been used to stabilize the gel-like HIPEs. Li et al. developed octenylsuccinate (OS) quinoa starch granule-stabilized emulsion gels with oil volume fraction (Φ) ranging from 50% to 70% (Fig. 6).22 The results indicated that increasing Φ from 40% to 70% significantly increased the extent of compact “aggregated” oil droplets in the emulsions, and gradually enhanced the mechanical strength of the gels, as these aggregated oil droplets acted as a kind of “active fillers”. Furthermore, Masalova et al. reported that emulsion gels formulated with low polar, high viscous oils exhibited improved stability.86 Nevertheless, it is yet limited in practical applications due to the high particle concentration or volume fraction of the dispersed phase.
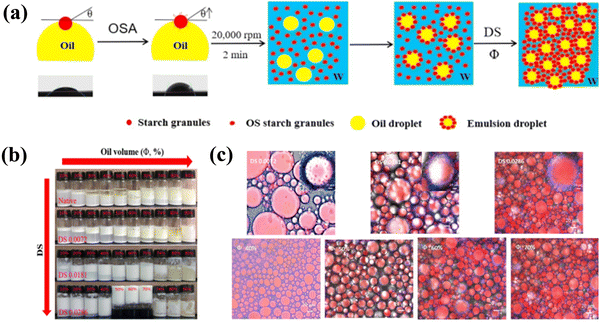 |
| Fig. 6 (a) Mechanism of formation of OS quinoa starch-based Pickering emulsion gels as a function of the degree of substitution (DS) and oil volume fraction (Φ). (b) The visual appearance of Pickering emulsions stabilized by native and OS quinoa starches as a function of DS and Φ. (c) Confocal microscopy images of Pickering emulsions and emulsion gels stabilized by OS quinoa starches as a function of DS and Φ.22 Copyright 2019 Elsevier. | |
4. Current applications
The exceptional stability and tunable rheology of emulsion gels make them a promising candidate for application in food,48,87,88 pharmaceuticals,89,90 and cosmetics.91,92 Furthermore, they can serve as soft templates for designing advanced materials with unique structural and functional properties.4,26,75 In this section, the most significant and prospective applications of emulsion gels will be discussed in detail (Fig. 7).
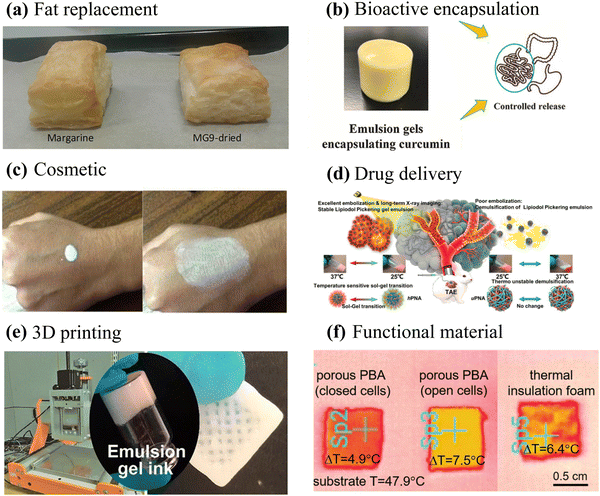 |
| Fig. 7 Application of emulsion gels in foods, pharmaceuticals, cosmetics, and materials science. (a) Image of puff pastry prepared using commercial laminating margarine and partially dried structured emulsion.101 Copyright 2021 Wiley. (b) Photograph of rhamnogalacturonan-I enriched pectin-based emulsion gel for the encapsulation and controlled release of curcumin.105 Copyright 2022 Elsevier. (c) Creams for UV protection prepared by particle-stabilized water-in-oil Pickering emulsions.91 Copyright 2016 Elsevier. (d) Pickering emulsion gel of lipiodol with favorable artery embolization and long-term X-ray imaging properties.90 Copyright 2021 Elsevier. (e) 3D-Printed scaffolds prepared using cellulose nanofibrils/alginate-based emulsion gels as inks.126 Copyright 2019 American Chemical Society. (f) Superior thermal insulation materials prepared using APEGs as templates.4 Copyright 2021 Wiley. | |
4.1. Food
4.1.1. Fat replacement.
The World Health Organization estimates that every year, trans fat intake leads to more than 500
000 deaths of people globally from cardiovascular disease.93 Thus, the consumers’ concerns about the harmful effects of fat consumption and higher requirements for food safety and nutrition have together attracted increasing attention to fat substitutes.7,87 In this regard, emulsion gels with soft-solid-like textures are considered effective means to achieve such a goal, which make liquid oils seem (mechanically) like solid fats. In terms of appearance and texture, emulsion gels resemble solid animal fats, but the oil phase of the emulsion gels consists of plant oils, such as olive oil,88,94 sunflower oil,95 corn oil,96 and coconut oil,97 which are rich in unsaturated fatty acids and are effective substitutes for animal fats. Paglarini et al. constructed the emulsion-filled gels with soy protein isolate, inulin, and soybean oil as the animal fat substitutes in Bologna sausages and obtained a reduction from 11% to 34% of fat in reformulated products.98 Likewise, Pintado et al. showed that when the fat of Frankfurters was replaced by an emulsion gel prepared with chia powder and olive oil, the fat level was reduced to 40%, resulting in a 30% decrease in energy intake, while the product displayed oxidative stability during storage.99 Similar research reported considerable reductions of fat in beef burgers100 and margarine101 using various emulsion gel systems as animal fat replacers.
In addition to employing emulsion gels to improve the functional properties of meat products (low-fat), mimicking the sensory properties of fat, such as texture, aroma, and flavor, is becoming an increasingly popular trend.102 Nishinari et al. evaluated the effects of cooling rate, shear rate, polysaccharide contents, and coexisting seasonings on the rheological properties of food emulsion gels to achieve easy swallowing, which could lower the risk of aspiration for each person who struggles with mastication and deglutition.103 Giarnetti et al. reported that shortbread cookies in which 50% of the butter was replaced by emulsion-filled gels based on inulin and olive oil displayed pleasing sensory properties close to those of full-fat ones and gained favorable consumer acceptance, accompanied by a wonderful lipid-lowering effect (19% less total fat, 39% less saturated fats) with respect to control.88
4.1.2. Encapsulation and delivery of functional food ingredients.
In emulsion gels, the incorporated functional food ingredients have limited mobility due to the compact gel network. Therefore, emulsion gels have emerged as encapsulation and delivery cargos of functional factors (e.g., polyphenols, vitamins, flavors, etc.), which can significantly improve their chemical stability, bioavailability, and range of applications in food systems.
4.1.2.1. Polyphenols.
The health benefits of polyphenols, particularly their anti-inflammatory and antioxidant effects, are well-recognized and have been thoroughly investigated.104 However, they are highly sensitive to temperature, pH, oxygen and light and it is difficult to supplement them into food due to their limited water dispersibility or solubility, which greatly hinder their widespread application in food products.105 Lv et al. fabricated chronically stable Pickering emulsion gels stabilized by food-grade WPI gel particles and the resultant emulsion gels loaded with curcumin exhibited high loading efficiency of curcumin and superior stability against light degradation.19 Likewise, Pintado et al. added two different phenolic compounds (hydroxytyrosol and flavanols) into a SPI emulsion gel system to obtain functional ingredients and employed them as fat substitutes in Frankfurters.106 The results indicated that Frankfurters containing the emulsion gel displayed high levels of hydroxytyrosol and flavanols, as well as outstanding oxidative and thermal stability without any detrimental changes in the sensory properties of the products.
4.1.2.2. Vitamins.
Vitamins, as essential micronutrients that must be ingested through diet, play key roles in human health.107 Ferreira et al. developed SPI-based heat-induced gels filled with Brazil nut oil emulsions for vehiculation of vitamin D3 (VD3-lipophilic vitamin).108 The stability and bioavailability of the incorporated VD3 were considerably improved after 30 days of storage under refrigeration, owing to the compact gelled network. Additionally, the gelled continuous phase of the emulsion can function as a carrier for hydrophilic bioactives. Roy and coworkers designed a self-assembled gel through hydrogen bonding between sulphonamide and amide groups of the amphiphiles, which effectively entrapped and released vitamin B12 at room temperature.109
4.1.2.3. Flavors.
The retention and controlled release of flavor components are critical to food quality. According to Lee et al., MTG-induced emulsion gels effectively improved the storage stability of aroma compounds.110 The result suggested that the retention of flavors (ethyl acetate and linalool) ranged from 60% to 100% in emulsion gels, whereas only 5% to 25% in emulsions when stored at 37 °C for 72 h. Moreover, the release behavior of flavors could be modified by changing the particle content,5 droplet size,111 or oil phase volume.112 For example, the increase in the WPI content conferred a pronounced reinforcing effect on the gel network, which led to reduced partition coefficients and release rates of the volatiles.5
4.1.2.4. Carotenoids.
Carotenoids consist of a series of conjugated double bonds that are extremely susceptible to oxygen, heat, and light, which largely limits their use in food formulations.113 Li et al. prepared octenylsuccinate quinoa starch (OSQS) granule-based emulsion gel as a carrier for lutein and reported that the retention index of lutein in the emulsion gel could reach 55.38% after storage for 31 days. The authors claimed that the storage stability and bioavailability of incorporated lutein were apparently increased related to the densely packed layer at the oil/water interface.23
4.1.2.5. Others.
Other functional factors included quercetin,114 minerals,58 capsaicinoids,115 terbinafine HCl,116 lactoferrin,117 anthocyanin,118 and lycopene,119 EPA and DHA.120
4.2. Pharmaceuticals
4.2.1. Drug delivery.
The novel drug-loading technique with high efficiency, sustained-releasing performance, and good bioactivity via emulsion-gel technology, exhibits great potential in bio-medicine and bio-pharmaceutics. Li et al. reported that a temperature-sensitive Pickering gel emulsion stabilized by hairy poly(N-isopropylacrylamide-co-acrylic acid) (hPNA) nanogels could efficiently improve the embolization strength and stability of Lipiodol for TAE therapy, facilitating to the enhancement of antitumor efficacy by the inhibition of the expression levels of VEGF and CD31.90 In other reports, the controlled release of metronidazole along with probiotics targeted delivery could be achieved in the emulsion gels from xanthan gum and guar gum modified sunflower oil by Pandey et al.89
4.2.2. Enzyme immobilization.
The majority of enzymes can maintain their catalytic activity and stability in microemulsion systems; however, product separation is typically difficult and in most circumstances, enzyme recovery is not practical. Rees et al. demonstrated that lipases immobilized in gelatin-containing microemulsion-based organogels (MBGs) were effective solid-phase catalysts.121 Large-scale syntheses in batch reactions were achieved in which lipases in MBGs were repeatedly used for the kinetic resolution of octan-3-ol, l-octen-3-ol, and 1-octyn-3-ol. Chiral esters with high optical purity from racemic 2-octanol and alkanoic acids were also successfully synthesized using lipases immobilized in MBGs.122 These gels for enzyme immobilization were of practical use in drug and organic synthesis due to the relatively concentrated reactant solutions, high conversions, long-term viability, and reuse of enzymes.
4.3. Cosmetics
Emulsion gel systems can also be used in the on-skin delivery and spreading of viscoelastic cosmetic formulations. Veverka et al. synthesized emulsion gels by homogenization of β-glucan (BG) hydrogel containing from 0.10 to 2.0 wt% BG with natural oils such as coconut oil and olive oil.97 The as-prepared gels could be exploited for transdermal delivery of both hydrophobic and hydrophilic substances, showing promising sufficient stability for emulsion-type cosmetics. de Lafuente et al. evaluated the impact of the incorporation of caffeine as a model active ingredient on the quality attributes of a bioadhesive emulgel formulation.92 There were no significant changes in the critical quality attributes and promising stability was observed for at least one year. Moreover, the product exhibited excellent skin adhesion properties in both in vitro/ex vivo bioadhesion experiments using human skin and better caffeine delivery performance than a commercial compounding cream. Some have also investigated the rheology of emulsion gels for cosmetic use.123
4.4. Materials science
Emulsion gels of one phase dispersed in another have, in recent years, emerged as a general platform for preparing advanced functional materials with various chemistries and morphologies. The outstanding stability and processability of emulsion gels make them ideal for moldable and porous materials.124–127 Moldable materials are prepared by placing emulsion gels in pre-made molds or 3D printing, in which the dense emulsion droplets provide the strength of the gel network and viscoelastic properties. Porous materials can be obtained by replacing the oil phase of emulsion gels with volatile substances and subsequently removing the volatile oil phase, due to the strong resistance to strain of the gel network. Zhu et al. first synthesized 3D edible structures from high internal phase Pickering emulsions (HIPPEs) stabilized by naturally derived chitin nanofibrils (NCh), and the resultant gels were demonstrated as the emulgel inks which were suitable for 3D printing (millimeter definition) via direct ink writing. Moreover, lightweight NCh-based porous materials were prepared by changing the internal phase to a volatile mineral oil.128 Wu et al. designed and prepared APEGs by bridging neighboring particle-stabilized droplets via telechelic polymers.4 The APEG systems showed typical shear-thinning behaviors and their viscoelastic properties were tunable by temperature, pH, and molecular weight of the telechelic polymers, making them ideal for 3D printing inks both in air and underwater. APEG-templated porous materials, further, were photopolymerized under UV irradiation, which exhibited better thermal insulation performance than commercial thermal insulation foam. Besides, the establishment of these emulsion-gel-templated highly porous materials opened the door for constructing ultra-sensitive and ultra-stretchable strain sensors and adsorption foams, etc.129–131
Emulsions are also widely applied in cleaning protocols and are particularly attractive in art conservation. Oil-in-water emulsions present as their main advantage the possibility to obtain a controlled cleaning, minimizing the use of organic solvents. However, uncontrolled spreading and penetration of emulsions can cause serious damage to artifacts. Cavallaro et al. fabricated ecocompatible emulsion gels by the combination of halloysite nanotubes and biopolyelectrolytes (pectin and chitosan) and used them for artifact cleaning.26 The emulsion in the pectin-based gel phase was suitable for wax layer removal from a marble surface. In particular, a controlled cleaning could be achieved by tuning the contact time between the gel and the marble surface.
5. Conclusions and outlook
Here, we have tried to paint a coherent and complete picture of emulsion gels, from their gelation mechanisms, structural characteristics, mechanical properties, preparation methods, and the main factors that govern the structures and rheological properties, to applications, highlighting significant recent progress. Emulsion gels are semi-solid emulsion systems with a three-dimensional gel network, which combine the virtues of both emulsions and gels. They can be produced by gelling the continuous phase of an emulsion (i.e., emulsion-filled gel) or aggregating the emulsified droplets to a high level (i.e., emulsion particulate gel). The rheological behaviors of the two differ due to the differences between their structures, the former being primarily determined by the gel matrix formed by the gelling agents in the continuous phase, while the latter depends mainly on the network structure formed by the aggregated emulsified droplets. Furthermore, it is possible to get tailor-made microstructures and rheological properties of the gels by controlling the fabrication methods, formulations, as well as other factors, such as temperature, pH, and ionic strength. The exceptional stability and tunable properties (morphology, rheology, and functionality) of emulsion gels make them promising candidates for a wide range of current and emerging applications, including fat replacement, encapsulation and delivery of functional food ingredients, drug delivery, enzyme immobilization, cosmetic delivery, and functional materials (e.g., thermal insulation, conductivity, and cleaning) synthesis.
Further fundamental and applied research needs to be carried out on a deeper scale. Firstly, considering the cost-effectiveness and simplicity of preparing emulsion gels, more research into the selection of raw materials and technology optimization is necessary. The use of natural biopolymer particles (e.g., starch granule), for instance, is in accordance with the Principles of Green Chemistry, but complex surface modification on the particle surface remains a great challenge. Secondly, the lack of taste caused by fat substitutes and relatively low loading and stability of bioactive factors in the gel system need to be addressed. The digestive behaviors of the emulsion gel systems within the human body should be carried out for the precise release of nutrition as well. Thirdly, in-depth understanding of the relationship between the gel structure and performance, from the nanoscale to the macroscale, and then intelligent tuning, could pave the way to active, adaptable, and responsive gel systems, which lead to novel applications. Finally, it has been reported that emulsion gels had great potential as the template for the preparation of advanced materials due to their distinct structural and functional features, further applications in multiple fields need to be explored. For example, polypyrrole (PPy) decorated emulsion gel-templated materials can be expected for photothermal conversion in seawater desalination and wastewater treatment. Applying the functional nanoparticles, such as TiO2 with excellent chemical stability and optical properties and MXene with high conductivity and electromagnetism, into emulsion gel systems, may empower emulsion gel-templated materials with potential for applications in the fields of catalysis, energy storage, and sensors, among others. We believe that the advancements on these subjects will contribute greatly to both fabrication techniques and applications of the emulsion gel systems.
Author contributions
C. H. conceived the review subject idea. C. W. did the literature review and wrote the original draft. Q. C. and M. Z. provided valuable guidance and writing assistance. All authors discussed the results and contributed to the final manuscript.
Conflicts of interest
There are no conflicts to declare.
Acknowledgements
This work was supported by the National Natural Science Foundation of China (No. 21903033 and 22275063, and 52293474), the Fundamental Research Funds for the Central Universities (No. 5003013050), and start-up funds from the Huazhong University of Science and Technology.
References
- T. Farjami and A. Madadlou, Trends Food Sci. Technol., 2019, 86, 85 CrossRef CAS.
- E. Dickinson, Food Hydrocolloids, 2012, 28, 224 CrossRef CAS.
- O. Torres, B. Murray and A. Sarkar, Trends Food Sci. Technol., 2016, 55, 98 CrossRef CAS.
- B. Wu, C. Yang, Q. Xin, L. Kong, M. Eggersdorfer, J. Ruan, P. Zhao, J. Shan, K. Liu, D. Chen, D. A. Weitz and X. Gao, Adv. Mater., 2021, 33, e2102362 CrossRef PubMed.
- L. Mao, Y. H. Roos and S. Miao, J. Agric. Food Chem., 2014, 62, 11420 CrossRef CAS PubMed.
- Y. Lu, L. Mao, Z. Hou, S. Miao and Y. Gao, Food Eng. Rev., 2019, 11, 245 CrossRef.
- Y. Ren, L. Huang, Y. Zhang, H. Li, D. Zhao, J. Cao and X. Liu, Foods, 2022, 11, 1950 CrossRef CAS PubMed.
- X. Li, L. Fan, Y. Liu and J. Li, Crit. Rev. Food Sci. Nutr., 2021, 1–23 Search PubMed.
- D. Lin, A. L. Kelly and S. Miao, Trends Food Sci. Technol., 2020, 102, 123 CrossRef CAS.
- T. van Vliet, Colloid Polym. Sci., 1988, 266, 518 CrossRef CAS.
- N. Luo, A. Ye, F. M. Wolber and H. Singh, Food Hydrocolloids, 2020, 108, 106076 CrossRef CAS.
- K. Wijarnprecha, A. de Vries, P. Santiwattana, S. Sonwai and D. Rousseau, LWT--Food Sci. Technol., 2019, 115, 108067 CrossRef CAS.
- M. Mantelet, M. Panouillé, F. Boué, V. Bosc, F. Restagno, I. Souchon and V. Mathieu, Food Hydrocolloids, 2019, 87, 506 CrossRef CAS.
- J. V. C. Silva, B. Jacquette, L. Amagliani, C. Schmitt, T. Nicolai and C. Chassenieux, Colloids Surf., A, 2019, 569, 85 CrossRef CAS.
- A. M. Herrero, P. Carmona, T. Pintado, F. Jiménez-Colmenero and C. Ruíz-Capillas, Food Hydrocolloids, 2011, 25, 12 CrossRef CAS.
- Q. Guo, A. Ye, M. Lad, D. Dalgleish and H. Singh, Soft Matter, 2014, 10, 1214 RSC.
- F. Liu and C.-H. Tang, Food Chem., 2011, 127, 1641 CrossRef CAS.
- I. M. Geremias-Andrade, N. P. D. B. G. Souki, I. C. F. Moraes and S. C. Pinho, LWT--Food Sci. Technol., 2017, 86, 166 CrossRef CAS.
- P. Lv, D. Wang, L. Dai, X. Wu, Y. Gao and F. Yuan, Food Res. Int., 2020, 132, 109032 CrossRef CAS PubMed.
- C.-H. Bi, P.-I. Wang, D.-Y. Sun, Z.-M. Yan, Y. Liu, Z.-G. Huang and F. Gao, J. Food Eng., 2020, 277, 109923 CrossRef CAS.
- O. Torres, N. M. Tena, B. Murray and A. Sarkar, Carbohydr. Polym., 2017, 178, 86 CrossRef CAS PubMed.
- S. Li, B. Zhang, C. P. Tan, C. Li, X. Fu and Q. Huang, Food Hydrocolloids, 2019, 91, 40 CrossRef CAS.
- S. Li, B. Zhang, C. Li, X. Fu and Q. Huang, Food Chem., 2020, 305, 125476 CrossRef CAS PubMed.
- M. Paciulli, P. Littardi, E. Carini, V. M. Paradiso, M. Castellino and E. Chiavaro, LWT--Food Sci. Technol., 2020, 133, 109888 CrossRef CAS.
- H. Yan, X. Chen, M. Feng, Z. Shi, W. Zhang, Y. Wang, C. Ke and Q. Lin, Colloids Surf., B, 2019, 177, 112 CrossRef CAS PubMed.
- G. Cavallaro, S. Milioto, L. Nigamatzyanova, F. Akhatova, R. Fakhrullin and G. Lazzara, ACS Appl. Nano Mater., 2019, 2, 3169 CrossRef CAS.
- Z. Liu, B. Bhandari, S. Prakash, S. Mantihal and M. Zhang, Food Hydrocolloids, 2019, 87, 413 CrossRef CAS.
-
B. Maria, PhD thesis, University of Szeged, 2008.
- R. Jost, R. Baechler and G. Masson, J. Food Sci., 1986, 51, 440 CrossRef CAS.
- G. Balakrishnan, B. T. Nguyen, C. Schmitt, T. Nicolai and C. Chassenieux, Food Hydrocolloids, 2017, 73, 213 CrossRef CAS.
- C. Schmitt, J. V. C. Silva, L. Amagliani, C. Chassenieux and T. Nicolai, Curr. Opin. Food Sci., 2019, 27, 43 CrossRef.
- Q. Guo, N. Bellissimo and D. Rousseau, Food Hydrocolloids, 2017, 69, 264 CrossRef CAS.
- X. Zhao, Y.-F. Zou, J.-J. Shao, X. Chen, M.-Y. Han and X.-L. Xu, J. Food Process. Preserv., 2017, 41, e12884 CrossRef.
- E. Ruffin, T. Schmit, G. Lafitte, J. M. Dollat and O. Chambin, Food Chem., 2014, 151, 324 CrossRef CAS PubMed.
- Y. Jiang, L. Liu, B. Wang, X. Yang, Z. Chen, Y. Zhong, L. Zhang, Z. Mao, H. Xu and X. Sui, Food Hydrocolloids, 2019, 91, 232 CrossRef CAS.
- J. Chen, E. Dickinson, M. Langton and A.-M. Hermansson, LWT--Food Sci. Technol., 2000, 33, 299 CrossRef CAS.
- G. Delgado-Pando, S. Cofrades, C. Ruiz-Capillas, M. Teresa Solas and F. Jiménez-Colmenero, Eur. J. Lipid Sci. Technol., 2010, 112, 791 CrossRef CAS.
- J. Glusac, S. Isaschar-Ovdat and A. Fishman, Food Chem., 2020, 315, 126301 CrossRef CAS PubMed.
- A. L. Gaspar and S. P. de Goes-Favoni, Food Chem., 2015, 171, 315 CrossRef CAS PubMed.
- E. Romeih and G. Walker, Trends Food Sci. Technol., 2017, 62, 133 CrossRef CAS.
- F. Alavi, Z. Emam-Djomeh, M. Salami and M. Mohammadian, Int. J. Biol. Macromol., 2020, 153, 523 CrossRef CAS PubMed.
- C.-H. Tang, M. Yang, F. Liu and Z. Chen, LWT--Food Sci. Technol., 2013, 53, 15 CrossRef CAS.
- L. Feng, X. Jia, Q. Zhu, Y. Liu, J. Li and L. Yin, Food Hydrocolloids, 2019, 89, 813 CrossRef CAS.
- M. Yang, F. Liu and C.-H. Tang, Food Res. Int., 2013, 52, 409 CrossRef CAS.
- C.-H. Tang, L.-J. Luo, F. Liu and Z. Chen, Food Res. Int., 2013, 51, 804 CrossRef CAS.
- L. Flaiz, M. Freire, S. Cofrades, R. Mateos, J. Weiss, F. Jimenez-Colmenero and R. Bou, Food Chem., 2016, 213, 49 CrossRef CAS PubMed.
- S. H. Lim, H. R. Kim, S. J. Choi and T. W. Moon, Food Sci. Biotechnol., 2015, 24, 2023 CrossRef CAS.
- Z. S. Ladjevardi, S. M. Gharibzahedi and M. Mousavi, Carbohydr. Polym., 2015, 125, 272 CrossRef CAS PubMed.
- A. C. Alting, R. J. Hamer, C. G. de Kruif and R. W. Visschers, J. Agric. Food Chem., 2000, 48, 5001 CrossRef CAS PubMed.
- V. Venugopal, S. N. Doke and P. M. Nair, Food Chem., 1994, 50, 185 CrossRef CAS.
- P. Rosa, G. Sala, T. V. Vliet and F. V. Velde, J. Texture Stud., 2006, 37, 516 CrossRef.
- X. Gu, L. J. Campbell and S. R. Euston, Food Res. Int., 2009, 42, 925 CrossRef CAS.
- F. Li, X. Kong, C. Zhang and Y. Hua, Food Hydrocolloids, 2012, 29, 347 CrossRef CAS.
- L. Ramasubramanian, B. R. D’Arcy, H. C. Deeth and H. E. Oh, J. Food Eng., 2014, 130, 45 CrossRef CAS.
- L. Lin, M. Wong, H. C. Deeth and H. E. Oh, Food Chem., 2018, 245, 97 CrossRef CAS PubMed.
- L. Lin, H. E. Oh, H. C. Deeth and M. Wong, Int. Dairy J., 2021, 113, 104893 CrossRef CAS.
- X. Wang, K. Luo, S. Liu, B. Adhikari and J. Chen, J. Food Eng., 2019, 244, 32 CrossRef CAS.
- V. L. Sok Line, G. E. Remondetto and M. Subirade, Food Hydrocolloids, 2005, 19, 269 CrossRef CAS.
- X. Lu, Z. Lu, L. Yin, Y. Cheng and L. Li, Food Res. Int., 2010, 43, 1673 CrossRef CAS.
- X. Wang, Z. He, M. Zeng, F. Qin, B. Adhikari and J. Chen, Food Chem., 2017, 221, 130 CrossRef CAS PubMed.
- M. E. Helgeson, S. E. Moran, H. Z. An and P. S. Doyle, Nat. Mater., 2012, 11, 344 CrossRef CAS PubMed.
- S. M. Hashemnejad, A. Z. M. Badruddoza, B. Zarket, C. Ricardo Castaneda and P. S. Doyle, Nat. Commun., 2019, 10, 2749 CrossRef PubMed.
- K. Kim, S. Kim, J. Ryu, J. Jeon, S. G. Jang, H. Kim, D. G. Gweon, W. B. Im, Y. Han, H. Kim and S. Q. Choi, Nat. Commun., 2017, 8, 14305 CrossRef CAS PubMed.
- J. Chae, S. Q. Choi and K. Kim, Soft Matter, 2022, 18, 53 RSC.
- X.-Y. Wang and M.-C. Heuzey, RSC Adv., 2016, 6, 89776 RSC.
- E. Dickinson, Colloids Surf., B, 2010, 81, 130 CrossRef CAS PubMed.
- X.-Y. Wang, J. Wang, D. Rousseau and C.-H. Tang, Food Hydrocolloids, 2020, 105, 105811 CrossRef.
- M. N. Lee, H. K. Chan and A. Mohraz, Langmuir, 2012, 28, 3085 CrossRef CAS PubMed.
- M. Kaganyuk and A. Mohraz, Soft Matter, 2017, 13, 2513 RSC.
- N. Zhang, L. Zhang and D. Sun, Langmuir, 2015, 31, 4619 CrossRef CAS PubMed.
- K. J. Lissant, J. Colloid Interface Sci., 1966, 22, 462 CrossRef CAS.
- Q.-H. Chen, J. Zheng, Y.-T. Xu, S.-W. Yin, F. Liu and C.-H. Tang, Food Hydrocolloids, 2018, 75, 125 CrossRef CAS.
- A. M. Bago Rodriguez and B. P. Binks, Curr. Opin. Colloid Interface Sci., 2022, 57, 101556 CrossRef CAS.
- V. O. Ikem, A. Menner and A. Bismarck, Angew. Chem., Int. Ed., 2008, 47, 8277 CrossRef CAS PubMed.
- H. Yu, Q. Wang, Y. Zhao and H. Wang, ACS Appl. Mater. Interfaces, 2020, 12, 14607 CrossRef CAS PubMed.
- B. Jiao, A. Shi, Q. Wang and B. P. Binks, Angew. Chem., Int. Ed., 2018, 57, 9274 CrossRef CAS PubMed.
- J. Wu, X. Guan, C. Wang, T. Ngai and W. Lin, J. Colloid Interface Sci., 2022, 610, 994 CrossRef CAS PubMed.
- Y.-T. Xu, T.-X. Liu and C.-H. Tang, Food Hydrocolloids, 2019, 88, 21 CrossRef CAS.
- X.-H. Chen and C.-H. Tang, Colloids Surf., A, 2021, 608, 125596 CrossRef CAS.
- H. Wu, Y. Nian, Y. Liu, Y. Zhang and B. Hu, J. Funct. Foods, 2022, 94, 105110 CrossRef CAS.
- T. Yang, J. Zheng, B.-S. Zheng, F. Liu, S. Wang and C.-H. Tang, Food Hydrocolloids, 2018, 82, 230 CrossRef CAS.
- S. Chang, X. Chen, S. Liu and C. Wang, Int. J. Biol. Macromol., 2020, 152, 703 CrossRef CAS PubMed.
- Y. Jie, F. Chen, T. Zhu and D. Lv, Food Hydrocolloids, 2022, 127, 107554 CrossRef CAS.
- X. Wang, Y. Nian, Z. Zhang, Q. Chen, X. Zeng and B. Hu, Colloids Surf., B, 2019, 183, 110459 CrossRef CAS PubMed.
- B. Tirgarian, J. Farmani, R. Farahmandfar, J. M. Milani and F. Van Bockstaele, Food Chem., 2022, 393, 133427 CrossRef CAS PubMed.
- I. Masalova, N. N. Tshilumbu, E. Mamedov and N. Sanatkaran, Chem. Eng. Commun., 2018, 205, 1 CrossRef CAS.
- S. Shaheen, M. Kamal, C. Zhao and M. A. Farag, Food Rev. Int., 2022 DOI:10.1080/87559129.2022.2073368.
- M. Giarnetti, V. M. Paradiso, F. Caponio, C. Summo and A. Pasqualone, LWT--Food Sci. Technol., 2015, 63, 339 CrossRef CAS.
- S. Pandey, K. Senthilguru, K. Uvanesh, S. S. Sagiri, B. Behera, N. Babu, M. K. Bhattacharyya, K. Pal and I. Banerjee, Int. J. Biol. Macromol., 2016, 92, 504 CrossRef CAS PubMed.
- H. Li, K. Qian, H. Zhang, L. Li, L. Yan, S. Geng, H. Zhao, H. Zhang, B. Xiong, Z. Li, C. Zheng, Y. Zhao and X. Yang, Chem. Eng. J., 2021, 418, 129534 CrossRef CAS.
- J. Marto, L. F. Gouveia, L. Goncalves, B. G. Chiari-Andreo, V. Isaac, P. Pinto, E. Oliveira, A. J. Almeida and H. M. Ribeiro, J. Photochem. Photobiol., B, 2016, 162, 56 CrossRef CAS PubMed.
- Y. de Lafuente, A. Ochoa-Andrade, M. E. Parente, M. C. Palena and A. F. Jimenez-Kairuz, Int. J. Cosmet. Sci., 2020, 42, 548 CrossRef CAS PubMed.
- J. Thornton, BMJ, 2018, 361, k2154 CrossRef PubMed.
- V. M. Paradiso, M. Giarnetti, C. Summo, A. Pasqualone, F. Minervini and F. Caponio, Food Hydrocolloids, 2015, 45, 30 CrossRef CAS.
- M. Geng, T. Hu, Q. Zhou, A. Taha, L. Qin, W. Lv, X. Xu, S. Pan and H. Hu, Int. J. Food Sci. Technol., 2021, 56, 1649 CrossRef CAS.
- X. Liu, X.-W. Chen, J. Guo, S.-W. Yin and X.-Q. Yang, Food Hydrocolloids, 2016, 61, 747 CrossRef CAS.
- M. Veverka, T. Dubaj, E. Veverková and P. Šimon, Colloids Surf., A, 2018, 537, 390 CrossRef CAS.
- C. de Souza Paglarini, V. A. Vidal, W. Ribeiro, A. P. Badan Ribeiro, O. D. Bernardinelli, A. M. Herrero, C. Ruiz-Capillas, E. Sabadini and M. A. Rodrigues Pollonio, J. Sci. Food Agric., 2021, 101, 505 CrossRef CAS PubMed.
- T. Pintado, A. M. Herrero, C. Ruiz-Capillas, M. Triki, P. Carmona and F. Jimenez-Colmenero, Food Sci. Technol. Int., 2016, 22, 132 CrossRef CAS PubMed.
- M. F. Dias, A. S. Guimarães, A. A. Benevenuto Júnior, V. R. O. Silva, P. R. Fontes, A. D. L. S. Ramos and E. M. Ramos, Br. Food J., 2021, 124, 50 CrossRef.
- S. Calligaris, S. Plazzotta, L. Barba and L. Manzocco, Eur. J. Lipid Sci. Technol., 2021, 123, 2000206 CrossRef CAS.
- Abdullah, L. Liu, H. U. Javed and J. Xiao, Front. Nutr., 2022, 9, 890188 CrossRef CAS PubMed.
- K. Nishinari, M. Takemasa, T. Brenner, L. Su, Y. Fang, M. Hirashima, M. Yoshimura, Y. Nitta, H. Moritaka, M. Tomczynska-Mleko, S. Mleko and Y. Michiwaki, J. Texture Stud., 2016, 47, 284 CrossRef.
- S. Saric and R. K. Sivamani, Int. J. Mol. Sci., 2016, 17, 1521 CrossRef PubMed.
- L. Zhang, J. Zheng, Y. Wang, X. Ye, S. Chen, H. Pan and J. Chen, Food Hydrocolloids, 2022, 128, 107592 CrossRef CAS.
- T. Pintado, I. Munoz-Gonzalez, M. Salvador, C. Ruiz-Capillas and A. M. Herrero, Food Chem., 2021, 340, 128095 CrossRef CAS PubMed.
- B. N. Estevinho, I. Carlan, A. Blaga and F. Rocha, Powder Technol., 2016, 289, 71 CrossRef CAS.
- L. S. Ferreira, T. C. Brito-Oliveira and S. C. Pinho, Food Biosci., 2022, 45, 101455 CrossRef CAS.
- S. Roy, B. Kar, S. Das and R. Datta, Chem. Zvesti, 2020, 74, 2635 CrossRef CAS.
- H. A. Lee, S. J. Choi and T. W. Moon, J. Food Sci., 2006, 71, C352 CrossRef CAS.
- Q. Guo, A. Ye, M. Lad, D. Dalgleish and H. Singh, Soft Matter, 2014, 10, 4173 RSC.
- L. Mao, S. Miao, F. Yuan and Y. Gao, Food Res. Int., 2018, 103, 1 CrossRef CAS PubMed.
- S. Li, X. Tang, Y. Lu, J. Xu, J. Chen and H. Chen, J. Sep. Sci., 2021, 44, 539 CrossRef CAS PubMed.
- X. Du, M. Hu, G. Liu, B. Qi, S. Zhou, K. Lu, F. Xie, X. Zhu and Y. Li, J. Food Eng., 2022, 314, 110784 CrossRef CAS.
- N. Luo, A. Ye, F. M. Wolber and H. Singh, Molecules, 2021, 26, 1379 CrossRef CAS PubMed.
- V. V. S. R. Karri, S. K. Raman, G. Kuppusamy, B. K. R. Sanapalli, A. Wadhwani, V. Patel and R. Malayandi, J. Nanosci. Curr. Res., 2018, 3, 1000119 Search PubMed.
- G. de Figueiredo Furtado, R. N. C. Pereira, A. A. Vicente and R. L. Cunha, Food Res. Int., 2018, 103, 371 CrossRef PubMed.
- M. Betz, B. Steiner, M. Schantz, J. Oidtmann, K. Mäder, E. Richling and U. Kulozik, Food Res. Int., 2012, 47, 51 CrossRef CAS.
- P. Lv, D. Wang, Y. Chen, S. Zhu, J. Zhang, L. Mao, Y. Gao and F. Yuan, Food Hydrocolloids, 2020, 108, 105992 CrossRef CAS.
- I. J. Haug, L. B. Sagmo, D. Zeiss, I. C. Olsen, K. I. Draget and T. Seternes, Eur. J. Lipid Sci. Technol., 2011, 113, 137 CrossRef CAS.
- G. D. Rees, B. H. Robinson and G. R. Stephenson, Biochim. Biophys. Acta, 1995, 1259, 73 CrossRef PubMed.
- S. Backlund, F. Eriksson, G. Hedström, A. Laine and M. Rantala, Colloid Polym. Sci., 1996, 274, 540 CrossRef CAS.
- F. R. Lupi, D. Gabriele, L. Seta, N. Baldino, B. de Cindio and R. Marino, Rheol. Acta, 2014, 54, 41 CrossRef.
- Q. Wang, X. Ji, X. Liu, Y. Liu and J. Liang, ACS Nano, 2022, 16, 12677 CrossRef CAS PubMed.
- J. Johannesson, J. Khan, M. Hubert, A. Teleki and C. A. S. Bergstrom, Int. J. Pharm., 2021, 597, 120304 CrossRef CAS PubMed.
- S. Huan, R. Ajdary, L. Bai, V. Klar and O. J. Rojas, Biomacromolecules, 2019, 20, 635 CrossRef CAS PubMed.
- Q. Jiang, B. P. Binks and Z. Meng, Food Hydrocolloids, 2022, 133, 107969 CrossRef CAS.
- Y. Zhu, S. Huan, L. Bai, A. Ketola, X. Shi, X. Zhang, J. A. Ketoja and O. J. Rojas, ACS Appl. Mater. Interfaces, 2020, 12, 11240 CrossRef CAS PubMed.
- C. Chai, M. Yi, Z. Zhang, Z. Huang, Q. Fan and J. Hao, Chemistry, 2021, 27, 13161 CrossRef CAS PubMed.
- Y. Zheng, X. Luo, J. You, T. Li and Q. Hou, J. Am. Ceram. Soc., 2020, 104, 1902 CrossRef.
- H. Gui, T. Zhang, S. Ji, G. Guan and Q. Guo, Polymer, 2020, 202, 122708 CrossRef CAS.
|
This journal is © The Royal Society of Chemistry 2023 |
Click here to see how this site uses Cookies. View our privacy policy here.