DOI:
10.1039/D3QI01501G
(Research Article)
Inorg. Chem. Front., 2023,
10, 6221-6228
Efficient iodine capture by metal–organic cubes based on hexanuclear vanadium clusters†
Received
31st July 2023
, Accepted 2nd September 2023
First published on 5th September 2023
Abstract
The cube is the only regular hexahedron among the Platonic solids, but supramolecules with a cubic configuration have rarely been reported. Herein, two isostructural metal–organic cubes VMOC-6 and VMOC-7 with large cavities are successfully synthesized by face-directed self-assembly of concave hexanuclear vanadium clusters [V6O6(OCH3)9(XO4)(COO)3]n− (X = S, n = 2; X = V, n = 1) and large-size nitrogen-containing tetravalent ligands. VMOC-6 and VMOC-7 are the largest metal–organic cubes based on metal clusters with an outer diameter of 28.47 × 28.17 × 28.44 Å3 and an inner cavity volume of 7785.657 Å3. Moreover, the cages can capture volatile iodine vapor with an uptake value of up to 1.83 g g−1, which is superior to those of most metal–organic materials. Mechanistic studies have shown that their good adsorption capacity is closely related to the presence of large internal cavities, electron-donating pyridine groups and redox-active V sites. The present work shows the potential application of these mesoporous MOPs in the treatment of iodine-containing nuclear waste.
Introduction
Self-assembly processes driven by non-covalent interactions are essential in the proliferation of all biological organisms and offer considerable advantages in the construction of large supramolecular assemblies.1–6 Metal–organic polyhedra (MOPs or molecular coordination cages) constructed by coordination-driven self-assembly processes not only are aesthetically attractive but also have attracted widespread interest due to their large inner cavities and controlled cavity environments, which allow them to be used as advanced functional materials for molecular recognition, catalysis, and optoelectronics applications.7–13 Unlike metal–organic frameworks (MOFs), MOPs are closed discrete structures, and their assembly process requires strict consideration of the harmony of chemical complementarity and spatial compatibility between organic and inorganic building units.14,15 Therefore, the design and assembly of MOPs with large internal cavities and potential functions remains one of the challenges in the field of supramolecular chemistry and materials science.
The cube is the only regular hexahedron among the Platonic solids, consisting of congruent regular squares with three meeting at each vertex. In addition, the cube is the only zonohedron with high symmetry consisting of an even number of side faces, and only a few supramolecules with a cubic configuration have been reported.16–19 To design and construct a molecular cube, at least three conditions must be satisfied: (i) the inorganic building block of the vertex must be trivalent; in other words, it can act as a “3-pyramidal” molecular building block (MBB); (ii) the angle η between the links in the MBB must be close to the desired ideal value, close to 90° for edge-directed models and should be close to 60° for face-directed models; (iii) eight “3-pyramidal” MBBs and the organic building blocks should be assembled into a closed shape arranged in a V8E12 or V8F6 geometry (V, E, and F denote vertices, edges and faces, respectively).19 In other words, the cube can consist of eight identical “3-pyramidal” MBBs connected by twelve “2-linear” or six “4-planar” ligands (Fig. 1).
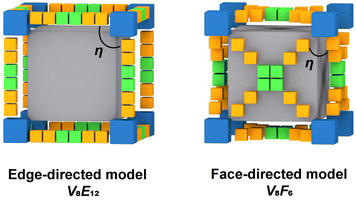 |
| Fig. 1 Schematic diagram of the cube construction guided by edge-directed (left) and face-directed (right) strategies. | |
Therefore, we reasoned that it is easier to construct closed polyhedra from directional metal clusters than from individual metal ions due to their abundant geometric properties. In recent years, we have been investigating polyoxovanadate-based metal–organic polyhedra and have demonstrated that polyoxovanadates are a good category of molecular building blocks (MBBs) for the construction of MOPs, including [V6O6(OCH3)9(SO4)4]5 ({V6(SO4)4}), [V5O9Cl]6, and [WV5O11(SO4)6]8.20–25 All these concave MBBs are favorable for the construction of isolated polyhedra, but only the 3-connected {V6(SO4)4} satisfies the conditions for the construction of cubes. However, in the process of trying to design MOPs with such building blocks, most of the edge-directed strategies are used as guiding principles to obtain MOPs with tetrahedral configurations.20,24,26,27 Because of the rigidity limitation of this MBB itself, it is difficult to reach an η angle of 90°, so it also seems to be difficult to construct cubes using the edge-directed strategy. As confirmed by our previous studies, it is possible to obtain a cubic structure by guiding through a face-directed strategy, since the η angle is 60° at this point.28 A series of polyoxovanadate-based metal–organic cubes constructed from three-coordinated {V6(SO4)(CO2)3} and tetracarboxylic acid ligands of different lengths and of C4-symmetry have been obtained. The size of the cubic cage can be adjusted by changing the ligand spacer portion while keeping the structural topology unchanged. We found that in these structures, the length of the four ligands is a key determinant of the size of the cubic cage. However, increasing the length of the ligands did not increase the cage size, and the side length of the largest VMOC-4 increased by only 0.35 Å compared to the smallest VMOC-1. Therefore, in this work, wider 4,4′,4′′,4′′-(1,4-phenylbis(pyridine-4,2,6-triyl))tetrabenzoic acid (H4PBPTA) was designed as a tetra-connected organic ligand, which was assembled with three-connected {V6O6(OCH3)9(SO4)} or {V6O6(OCH3)9(VO4)} into two isomorphic cubic cages VMOC-6 and VMOC-7 with large size cavities and with side lengths of about 28.36 Å. In addition, their large cavities, electron-donating pyridine groups and redox-active V sites enable them to exhibit excellent performance in iodine capture.
Experiments and methods
Synthesis of (NH2Me2)16[(V6O6(OCH3)9(SO4))8(PBPTA)6] (VMOC-6).
VOSO4·xH2O (0.02 g, 0.12 mmol) and H4PBPTA (0.01 g, 0.03 mmol) were dissolved in 0.5 mL of N,N-dimethylformamide (DMF) and 2.5 mL of methanol (MeOH). The mixed solution was transferred to a reaction vessel and heated to 160 °C for 48 hours. After cooling to room temperature, green rectangular crystals were obtained by filtration. Yield: 40%. Elemental analysis (%): calcd for C368H490N28O200S8V48: C, 39.44; H, 4.41; N, 3.50; found: C, 39.18; H, 4.56; N, 3.25. IR (KBr, cm−1): 3511 (br), 3441 (m), 3385 (m), 3035 (m), 2930 (m), 2810 (m), 1711 (m), 1655 (vs), 1600 (s), 1550 (s), 1480 (s), 1403 (vs), 1242 (m),1172 (m), 1095 (m), 1067 (m), 955 (vs), 864 (m), 829 (m), 780 (m), 696 (m), 668 (m), 577 (s), 542 (s).
Synthesis of (NH2Me2)24[(V6O6(OCH3)9(VO4))8(PBPTA)6] (VMOC-7).
VOSO4·xH2O was replaced with VO(acac)2 (0.02 g, 0.07 mmol) in the above-mentioned procedure and other conditions were kept constant. Brown streaky crystals were obtained in 45% yield. Elemental analysis (%): calcd for C384H544N36O200V56: C, 39.36; H, 4.68; N, 4.30; found: C, 39.18; H, 4.46; N, 4.54. IR (KBr, cm−1): 3595 (br), 3518 (m), 3441 (m), 3363 (m), 3028 (m), 2915 (m), 2818 (m), 1711 (m), 1663 (s), 1600 (vs), 1550 (s), 1487 (s), 1403 (vs), 1312 (m), 1249 (s), 1179 (m), 1060 (s), 1018 (s), 984 (s), 948 (vs), 871 (s), 836 (s), 780 (s), 745 (m), 703 (m), 668 (s), 535 (s).
Results and discussion
Structural description
The strong acidity of vanadium has led to the preferential use of oxygen-donating ligands for the construction of vanadium-based metal–organic materials.29,30 In previous work, a series of metal–organic cubes were constructed by self-assembly of {V6S} with H4BPTC and its derived tetradentate carboxylic acid ligands. These ligands have equal distances between adjacent carboxyl groups at both ends and have the same width. The length of the ligands is adjusted by changing the middle spacing portion of the ligands, thus adjusting the size of the cubic cage, where the longest ligand, tris-biphenyl-3,3′,5,5′-tetracarboxylic acid (H4TCTP), constitutes the largest cubic cage. Therefore, to obtain a larger cubic cage, we chose to increase the width of the ligand while keeping the maximum length unchanged, i.e., by replacing the adjacent carboxylic acid at both ends of the original ligand with benzoic acid, as the addition of the benzene ring broadens the distance between the adjacent carboxyl groups. The larger tetracarboxylic acid ligand H4PBPTA was synthesized (Fig. S1†), and the metal–organic cubes VMOC-6 and VMOC-7 with the same configuration were successfully obtained by reactions with VOSO4 and VO(acac)2, respectively. Thermogravimetric analysis and infrared spectral characterization of both are shown in Fig. S2 and S3.† Single-crystal X-ray diffraction analysis shows that both VMOC-6 and VMOC-7 crystallize in the triclinic space group P
(Table S1†). The good crystallinity and phase purity of VMOC-6 and VMOC-7 were demonstrated by comparing the two powder X-ray diffraction (PXRD) patterns obtained experimentally and calculated from single crystal data (Fig. S4†).
The six {VO6} octahedra form a ring in an edge-sharing pattern and a {XO4} (X = S/V) tetrahedron is coordinated to the center of the unit in an angle-sharing pattern, and the sulfate at the edge is replaced by a C atom from the carboxylic acid to form a concave trigonal “3-pyramidal” MBB [V6O6(OCH3)9(XO4)(COO)3]n− (X = S, n = 2; X = V, n = 1) (abbreviated as {V6(XO4)(CO2)3}) (Fig. 2a and Fig. S5†). Eight such concave {V6(XO4)(CO2)3} MBBs are connected by six PBPTA ligands to form a discrete metal–organic cube with the V8F6 geometry and with Oh symmetry, where the MBBs serve as vertices and PBPTAs serve as faces (Fig. 2b and c). The general formula of the anionic structures of VMOC-6 and VMOC-7 can be expressed as [(V6O6(OCH3)9(XO4))8(BPTC)6]n− (X = S, n = 16; X = V, n = 24). The negative charge is balanced by the corresponding amount of the dimethylamine cation [NH2Me2]+ generated by DMF decomposition.
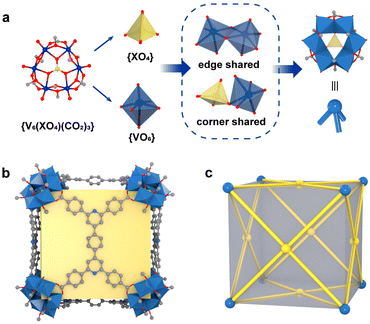 |
| Fig. 2 (a) Structure of {V6(XO4)(CO2)3} (X = S/V) and the coordination mode. The six edge-sharing {VO6} octahedra share the {XO4} tetrahedral vertices to form the “3-pyramidal” MBB. (b) Structures of VMOC-6 and VMOC-7. (c) The simplified cubic model, with the yellow part of H4PBPTA simplified to “4-planar” and the blue part of the hexanuclear vanadium cluster simplified to “3-pyramidal”. | |
In the structures of VMOC-6 and VMOC-7, the dihedral angle (φ) between two adjacent planes formed by connecting four carboxyl carbon atoms of the tetracarboxylic acid ligands one after another of a cube is close to 90° (Fig. S6†) and the angle (η) between the links is close to 60°, which are consistent with the expected values for the cubes constructed using the face-directed strategy (Table S2†). Compared to that of the reported largest cube VMOC-4 (21.55 × 21.56 × 21.60 Å3), the outer diameter (measured from the distance between the outermost carbon atoms of antipodal vertices) of VMOC-6 and VMOC-7 reached 28.47 × 28.17 × 28.44 Å3 due to the expansion of the width of the bridging ligands (Fig. 3a and 3c). To compare the internal space of VMOC-4 and VMOC-6, their internal cavity volumes were calculated from the crystal structure using the VOIDOO program.31 The internal cavity volume of VMOC-6 is 7785.657 Å3, which is 3.2 times larger than that of VMOC-4 (2437.828 Å3, Fig. 3b and d). Unfortunately, due to weak supramolecular interactions between the cages and also larger cavities, VMOP-6 and VMOP-7 were not stable after solvent removal and exhibited lower adsorption capacity for N2 (Fig. S7†).24,32,33
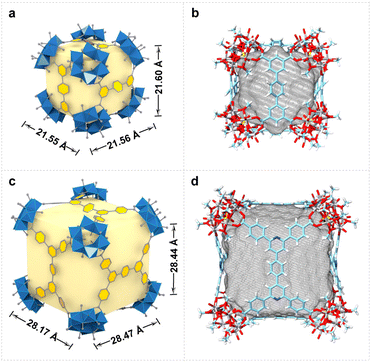 |
| Fig. 3 Crystal structures of VMOC-4 (a and b) and VMOC-6 (c and d). The left column highlights the overall size of each cubic cage and the arrangement of the ligands, while the right column shows the void spaces (gray mesh) calculated using the VOIDOO program. | |
Iodine vapor uptake
Iodine (I2) is a volatile and radioactive by-product of nuclear energy production, and its effective capture is particularly important because of its long isotopic lifetime, which directly affects human metabolic processes. It is widely known that I2 is an electron acceptor, and therefore, it can form host–guest charge transfer complexes with cages containing electron donor groups, which can bind iodine molecules in the cavity. Considering that the structures of VMOC-6 and VMOC-7 contain large internal cavities and active site ligands, their potential application in iodine capture was compared to that of VMOC-4. Simulating typical nuclear waste reprocessing conditions, samples were placed in glass tubes and transferred to large sealed glass vials containing iodine with no direct contact between the solid samples and the iodine (Fig. S8†). Next, the closed containers were placed under ambient pressure and heated in an oven at 75 °C. As the reaction time increased, the iodine loading increased and was weighed at hourly intervals. Afterwards, the glass vials were placed back into the closed containers and the iodine adsorption was continued until the weight of the sample no longer changed and the adsorption equilibrium was reached. After iodine vapor capture, the iodine uptake capacity was calculated according to eqn (1):where Q (g g−1) is the iodine uptake capacity of the sample, and m1 (g) and m2 (g) are the masses of the samples before and after iodine capture, respectively. During iodine capture, the color of the compounds exposed to I2 vapor gradually darkened with time, indicating the successful adsorption of I2 (Fig. 4a). Overall, VMOC-4 first saturated at 6 h of iodine adsorption, and then VMOC-6 and VMOC-7 also saturated in 10 h. Their equilibrium uptake capacities were 0.27 g g−1, 1.83 g g−1 and 1.82 g g−1, respectively, and VMOC-6 has the same adsorption capacity as VMOC-7 and it is 6.8 times that of VMOC-4 (Fig. S9†). Since the adsorption curves of VMOC-6 and VMOC-7 had basically the same trend, the kinetic data of VMOC-6 and VMOC-4 can be analyzed according to a pseudo-second-order kinetic model (Fig. 4b), which can be described using eqn (2): | 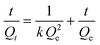 | (2) |
where t is the adsorption time (h) and Qe and Qt (mg g−1) are the amounts of iodine loaded at equilibrium and at time t, respectively. k (g mg−1 h−1) is the pseudo-second-order rate constant for adsorption. The high correlation coefficients (R2 > 0.99) obtained indicate that the uptake of iodine by VMOC-6 and VMOC-4 conforms to a pseudo-second-order kinetic model with rate constants of 0.4484 and 0.0689 g mg−1 h−1, respectively. Further thermogravimetric tests were performed on VMOC-6 before and after iodine uptake, and the TGA curve of I2@VMOC-6 after iodine uptake showed a significant mass loss before 200 °C compared to that of VMOC-6, which was due to the release of captured iodine. The loss attributed to iodine was estimated to be 63.45% of the total weight, which coincided with the iodine content of I2@VMOC-6 (64.15%) (Fig. 4c). To the best of our knowledge, studies on the adsorption of iodine vapor by metal–organic polyhedra are relatively rare.34VMOC-6 has the highest iodine adsorption capacity among MOPs34 and surpasses most MOFs35 (Table S3†), such as the well-known ZIF-8 (1.25 g g−1),36 Uio-66 (1.17 g g−1)37 and HKUST-1 (1.75 g g−1).38
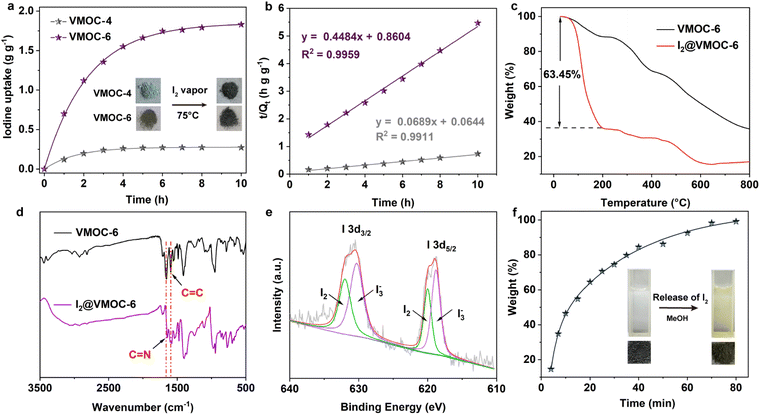 |
| Fig. 4 (a) Iodine vapor adsorption versus time for VMOC-4 and VMOC-6 at 75 °C and 1 atm (inset: photos of samples before and after I2 vapor adsorption). (b) The pseudo-second-order kinetic data of the iodine adsorption by VMOC-4 and VMOC-6. (c) TGA curves of VMOC-6 (black line) and I2@VMOC-6 (red line). (d) FT-IR spectra of VMOC-6 (black line) and I2@VMOC-6 (purple line). (e) XPS spectra of I 3d for I2@VMOC-6 derived signals. (f) Iodine release versus time (inset: photographs of the methanol solution and sample before and after I2 release). | |
To investigate the determinants of iodine capture, scanning electron microscopy (SEM), energy dispersive X-ray spectroscopy (EDS) mapping, Fourier transform infrared (FTIR) analysis, and X-ray photoelectron spectroscopy (XPS) were applied for characterization. SEM images showed that the prepared VMOC-6 cubes were rhombic crystals with no change in morphology after iodine adsorption. The EDS mapping patterns showed that iodine was uniformly distributed throughout VMOC-6 after iodine adsorption (Fig. S10†). Further study by FTIR spectroscopy revealed that the C
N band of VMOC-6 shifted from 1657 cm−1 to 1645 cm−1 after iodine loading and the C
C stretching vibration also changed, indicating that the adsorption sites may be distributed on the benzene and pyridine rings of the ligand (Fig. 4d).39 As shown in Fig. 4e, in the I 3d XPS spectrum, two distinct peaks of I2@VMOC-6 are located at 620.0 and 632.0 eV, attributed to I 3d5/2 and I 3d3/2 of the I2 molecule, respectively, and the other two peaks are located at 618.6 and 630.3 eV, attributed to I3−, indicating that the adsorbed iodine species are present in the I2 and I3− forms. Examples of iodine adsorption using redox metals in some MOFs exist,40,41 and the XPS characterization of I2@VMOC-6 considering the redox properties of vanadium revealed the presence of VV, suggesting that some of the VIV was reduced to VV (Fig. S11†). Thus, it was demonstrated that VMOC-6 and iodine have a driving force for adsorption, probably due to the weak π–π interactions between the pyridine ring and iodine. Moreover, N acts as a molecular trap for iodine due to its strong affinity for iodine.42,43 Notably, it is due to two processes, charge transfer between I2 and the nitrogen atoms in the pyridine ring and redox reactions between I2 and V, that the adsorbed iodine in VMOC-6 can be further converted into the polyiodide anion (I3−).44,45 This indicates that the capture process is a combination of physical and chemical adsorption. Therefore, the high iodine uptake capacity of VMOC-6 can be attributed to the synergistic effect of the larger internal space, the high affinity of the pyridine-containing donor for iodine and the redox activity of V.
The captured iodine can be released from I2@VMOC-6 through contact with a suitable solvent (e.g. MeOH). The iodine release process from I2@VMOC-6 in methanol solution was observed using a UV spectrophotometer at ambient temperature. When 2 mg of the I2@VMOC-6 solid was immersed in MeOH, the captured iodine continuously released and the color of the solution changed from colorless to pale yellow (Fig. 4f). The UV-vis spectrum of the methanol solution showed two bands at 291 and 360 nm and a shoulder at 439 nm (Fig. S12†), which was attributed to the presence of the polyiodide anion in I2@VMOC-6.46–49 The amount of iodine released was calculated from the UV-vis spectral data and the corresponding standard working curve of the I2 methanol solution (Fig. S13†). The time-dependent profile of I2@VMOC-6 in methanol showed that about 84.5% of the captured iodine rapidly released in the first 40 min, after which the release rate of iodine gradually decreased with almost complete release of iodine at 80 min (Fig. 4f).
Iodine adsorption in solution
Encouraged by its excellent performance in iodine vapor capture, the I2 adsorption capacity of VMOC-6 in solution was investigated. As nonpolar iodine is insoluble in polar solvents such as water, cyclohexane was chosen as the solvent for the adsorption experiments and the iodine concentration in the solution was determined by UV-vis spectrometry. The standard curve of iodine in cyclohexane solution obtained using UV-vis spectroscopy showed a good linear relationship between the absorbance value and the concentration of iodine, satisfying the Beer–Lambert law, as shown in Fig. S14.† It is well known that adsorption equilibrium isotherms can be used to describe the diffusion state of the adsorbent molecules and the adsorbent at equilibrium. Different equilibrium data were collected to determine the amount of iodine uptake versus the iodine concentration at equilibrium by varying the amount of adsorbent, that is, by exposing 5–25 mg of VMOC-6 to a solution of cyclohexane with an iodine concentration of 0.002 mmol L−1. As seen in Fig. 5a, the amount of iodine adsorbed continues to increase as the equilibrium concentration increases. The adsorption isotherms can be simulated using the Langmuir and Freundlich models, where the Langmuir isotherm model assumes that the adsorption process occurs on a homogeneous monolayer surface of the adsorbent, while the Freundlich model assumes that the adsorption occurs at a non-homogeneous surface, as follows: | 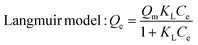 | (3) |
|  | (4) |
where Qm and Qe (mg g−1) are the maximum amount of iodine adsorbed and the amount of iodine adsorbed at equilibrium. Ce (mg L−1) is the concentration of iodine in the cyclohexane solution at adsorption equilibrium. KL and KF are the Langmuir and Freundlich constants related to the heat of adsorption and the Freundlich constant indicates the adsorption capacity at an equilibrium concentration equal to 1, and 1/n represents the adsorption strength. The corresponding values for the two isotherm models are shown in Table 1. It can be seen from the R2 values that the Langmuir model fits better than the Freundlich model, which means that the adsorption sites on the VMOC-6 surface are evenly distributed. Based on the Langmuir model, the saturation adsorption capacity was calculated to be 236.31 mg g−1. The adsorption kinetics of iodine was further investigated by UV-vis monitoring of this adsorption process by immersing the treated 30 mg crystal sample in 35 mL of cyclohexane solution with an iodine concentration of 0.002 mol L−1 at room temperature (Fig. 5b). The concentration of iodine in the solution decreased rapidly with increasing contact time, and the relative removal of iodine reached 53.62% within 2 h. After 24 h, the relative removal of iodine reached 77.26% and the adsorption equilibrium was reached (Fig. 5c). The rapid decrease of iodine concentration in the solution with increasing contact time was accompanied by a change of color from purple to pink. t/Qt showed a linear relationship with t (R2 = 0.9988), demonstrating that the kinetic data were in good agreement with the pseudo-secondary kinetic model.
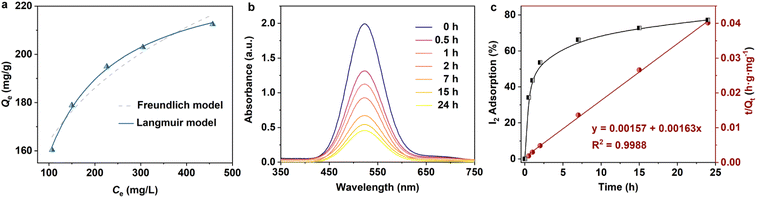 |
| Fig. 5 (a) Langmuir and Freundlich model fitting of the isotherm of iodine adsorption by VMOC-6 in cyclohexane solution. (b) Time evolution of the UV-vis absorption spectra of cyclohexane solutions in the presence of VMOC-6. (c) The kinetics of the adsorption process of iodine on VMOC-6 was plotted with the relative removal rate (%) of iodine (black line) and t/Qt (red line) versus time. | |
Table 1 Adsorption equilibrium constants of Langmuir and Freundlich isotherm equations for iodine adsorption on VMOC-6 adsorbents
Langmuir |
Freundlich |
Q
m (mg g−1) |
K
L (L mg−1) |
R
2
|
K
F (mg g−1) |
n
|
R
2
|
236.3066 |
0.0203 |
0.9965 |
70.3633 |
5.45 |
0.9392 |
Conclusions
In summary, the tetracarboxylic acid ligand H4PBPTA was successfully self-assembled with the 3-connected hexanuclear vanadium clusters {V6(SO4)(CO2)3} and {V6(VO4)(CO2)3} to form two isostructural metal–organic cubes VMOC-6 and VMOC-7 using the face-directed strategy under solvothermal conditions. They are the largest metal–organic cubes based on metal clusters, with a side length of about 28.3 Å and an inner cavity volume of about 7785.657 Å3. The presence of electron-donating pyridine groups and redox-active V and the large inner cavity led to an iodine trapping capacity of 1.83 g g−1 in the gas phase, which is the highest iodine adsorption capacity of metal–organic polyhedra to date and exceeds that of most previously reported metal–organic frameworks. This study provides a new perspective on the design of metal–organic polyhedra with tunable internal cavities and potential applications in the treatment of iodine-containing nuclear waste.
Conflicts of interest
There are no conflicts to declare.
Acknowledgements
This work was financially supported by the NSFC of China (No. 22271023, 22175033).
References
- S. R. Seidel and P. J. Stang, High-symmetry coordination cages via self-assembly, Acc. Chem. Res., 2002, 35, 972–983 CrossRef CAS PubMed.
- T. R. Cook, V. Vajpayee, M. H. Lee, P. J. Stang and K.-W. Chi, Biomedical and Biochemical Applications of Self-Assembled Metallacycles and Metallacages, Acc. Chem. Res., 2013, 46, 2464–2474 CrossRef CAS PubMed.
- L.-J. Chen, H.-B. Yang and M. Shionoya, Chiral metallosupramolecular architectures, Chem. Soc. Rev., 2017, 46, 2555–2576 RSC.
- R. Zhang, S. Ji, N. Wang, L. Wang, G. Zhang and J.-R. Li, Coordination-Driven In Situ Self-Assembly Strategy for the Preparation of Metal–Organic Framework Hybrid Membranes, Angew. Chem., Int. Ed., 2014, 53, 9775–9779 CrossRef CAS PubMed.
- B. H. Northrop, Y.-R. Zheng, K.-W. Chi and P. J. Stang, Self-Organization in Coordination-Driven Self-Assembly, Acc. Chem. Res., 2009, 42, 1554–1563 CrossRef CAS PubMed.
- Z.-Y. Li, Y. Zhang, C.-W. Zhang, L.-J. Chen, C. Wang, H. Tan, Y. Yu, X. Lo and H.-B. Yang, Cross-Linked Supramolecular Polymer Gels Constructed from Discrete Multi-pillar 5 arene Metallacycles and Their Multiple Stimuli-Responsive Behavior, J. Am. Chem. Soc., 2014, 136, 8577–8589 CrossRef CAS PubMed.
- Q.-F. Sun, J. Iwasa, D. Ogawa, Y. Ishido, S. Sato, T. Ozeki, Y. Sei, K. Yamaguchi and M. Fujita, Self-Assembled M24L48 Polyhedra and Their Sharp Structural Switch upon Subtle Ligand Variation, Science, 2010, 328, 1144–1147 CrossRef CAS PubMed.
- M. Eddaoudi, J. Kim, J. B. Wachter, H. K. Chae, M. O'Keeffe and O. M. Yaghi, Porous metal–organic polyhedra: 25 angstrom cuboctahedron constructed from 12 Cu2(CO2)4 paddle-wheel building blocks, J. Am. Chem. Soc., 2001, 123, 4368–4369 CrossRef CAS PubMed.
- A. C. Sudik, A. R. Millward, N. W. Ockwig, A. P. Cote, J. Kim and O. M. Yaghi, Design, synthesis, structure, and gas (N2, Ar, CO2, CH4, and H2) sorption properties of porous metal-organic tetrahedral and heterocuboidal polyhedra, J. Am. Chem. Soc., 2005, 127, 7110–7118 CrossRef CAS PubMed.
- D. J. Tranchemontagne, Z. Ni, M. O'Keeffe and O. M. Yaghi, Reticular Chemistry of Metal–Organic Polyhedra, Angew. Chem., Int. Ed., 2008, 47, 5136–5147 CrossRef CAS PubMed.
- M. L. Saha, X. Yan and P. J. Stang, Photophysical Properties of Organoplatinum(II) Compounds and Derived Self-Assembled Metallacycles and Metallacages: Fluorescence and its Applications, Acc. Chem. Res., 2016, 49, 2527–2539 CrossRef CAS PubMed.
- F. J. Rizzuto and J. R. Nitschke, Stereochemical plasticity modulates cooperative binding in a CoII12L6 cuboctahedron, Nat. Chem., 2017, 9, 903–908 CrossRef CAS PubMed.
- D. Fiedler, D. H. Leung, R. G. Bergman and K. N. Raymond, Selective molecular recognition, C–H bond activation, and catalysis in nanoscale reaction vessels, Acc. Chem. Res., 2005, 38, 349–358 CrossRef CAS PubMed.
- S. Lee, H. Jeong, D. Nam, M. S. Lah and W. Choe, The rise of metal–organic polyhedra, Chem. Soc. Rev., 2021, 50, 528–555 RSC.
- A. Kondinski, A. Menon, D. Nurkowski, F. Farazi, S. Mosbach, J. Akroyd and M. Kraft, Automated Rational Design of Metal–Organic Polyhedra, J. Am. Chem. Soc., 2022 DOI:10.1021/jacs.2c03402.
- W. J. Ramsay, F. T. Szczypinski, H. Weissman, T. K. Ronson, M. M. J. Smulders, B. Rybtchinski and J. R. Nitschke, Designed Enclosure Enables Guest Binding Within the 4200 Å3 Cavity of a Self-Assembled Cube, Angew. Chem., Int. Ed., 2015, 54, 5636–5640 CrossRef CAS PubMed.
- W. J. Ramsay, T. K. Ronson, J. K. Clegg and J. R. Nitschke, Bidirectional Regulation of Halide Binding in a Heterometallic Supramolecular Cube, Angew. Chem., Int. Ed., 2013, 52, 13439–13443 CrossRef CAS PubMed.
- P. Sutar, V. M. Suresh, K. Jayaramulu, A. Hazra and T. K. Maji, Binder driven self-assembly of metal–organic cubes towards functional hydrogels, Nat. Commun., 2018, 9, 3587 CrossRef PubMed.
- J. P. Lang, Q. F. Xu, Z. N. Chen and B. F. Abrahams, Assembly of a supramolecular cube, [CP*WS3CU3]8CI8[CN]12Li4 from a preformed incomplete cubane-like compound [PPh4] [CP*WS3(CuCN)3], J. Am. Chem. Soc., 2003, 125, 12682–12683 CrossRef CAS.
- A. W. Augustyniak, M. Fandzloch, M. Domingo, I. Lakomska and J. A. R. Navarro, A vanadium(IV) pyrazolate metal-organic polyhedron with permanent porosity and adsorption selectivity, Chem. Commun., 2015, 51, 14724–14727 RSC.
- Y. Zhang, H. Gan, C. Qin, X. Wang, Z. Su and M. J. Zaworotko, Self-Assembly of Goldberg Polyhedra from a Concave WV5O11(RCO2)5(SO4)3− Building Block with 5-Fold Symmetry, J. Am. Chem. Soc., 2018, 140, 17365–17368 CrossRef CAS PubMed.
- Y.-T. Zhang, X.-L. Wang, E.-L. Zhou, X.-S. Wu, B.-Q. Song, K.-Z. Shao and Z.-M. Su, Polyoxovanadate-based organic inorganic hybrids: from {V5O9Cl} clusters to nanosized octahedral cages, Dalton Trans., 2016, 45, 3698–3701 RSC.
- H. Gan, N. Xu, C. Qin, C. Sun, X. Wang and Z. Su, Equi-size nesting of Platonic and Archimedean metal-organic polyhedra into a twin capsid, Nat. Commun., 2020, 11, 4103 CrossRef CAS PubMed.
- Y. Gong, Y. Zhang, C. Qin, C. Sun, X. Wang and Z. Su, Bottom-Up Construction and Reversible Structural Transformation of Supramolecular Isomers based on Large Truncated Tetrahedra, Angew. Chem., Int. Ed., 2019, 58, 780–784 CrossRef CAS PubMed.
- N. Xu, H. Gan, C. Qin, X. Wang and Z. Su, From Octahedral to Icosahedral Metal-Organic Polyhedra Assembled from Two Types of Polyoxovanadate Clusters, Angew. Chem., Int. Ed., 2019, 58, 4649–4653 CrossRef CAS.
- Y.-T. Zhang, X.-L. Wang, S.-B. Li, Y.-R. Gong, B.-Q. Song, K.-Z. Shao and Z.-M. Su, Anderson-like alkoxo-polyoxovanadate clusters serving as unprecedented second building units to construct metal–organic polyhedra, Chem. Commun., 2016, 52, 9632–9635 RSC.
- Y.-T. Zhang, S.-B. Li, X.-L. Wang, Y.-R. Gong, K.-Z. Shao and Z.-M. Su, Synthesis, structures, and magnetic properties of metal-organic polyhedra based on unprecedented {V7} isopolyoxometalate clusters, Dalton Trans., 2016, 45, 14898–14901 RSC.
- Y. Gong, C. Qin, Y. Zhang, C. Sun, Q. Pan, X. Wang and Z. Su, Face-Directed Assembly of Molecular Cubes: In Situ Substitution of a Predetermined Concave Cluster, Angew. Chem., Int. Ed., 2020, 59, 22034–22038 CrossRef CAS PubMed.
- J. M. Breen and W. Schmitt, Hybrid organic-inorganic polyoxometalates: Functionalization of VIV/VV nanosized clusters to produce molecular capsules, Angew. Chem., Int. Ed., 2008, 47, 6904–6908 CrossRef CAS PubMed.
- Z. Zhang, W.-Y. Gao, L. Wojtas, Z. Zhang and M. J. Zaworotko, A new family of anionic organic–inorganic hybrid doughnut-like nanostructures, Chem. Commun., 2015, 51, 9223–9226 RSC.
- G. J. Kleywegt and T. A. Jones, Detection, delineation, measurement and display of cavities in macromolecular structures, Acta Crystallogr., Sect. D: Biol. Crystallogr., 1994, 50, 178–185 CrossRef CAS PubMed.
- D. Luo, X. P. Zhou and D. Li, Beyond Molecules: Mesoporous Supramolecular Frameworks Self-Assembled from Coordination Cages and Inorganic Anions, Angew. Chem., Int. Ed., 2015, 54, 6190–6195 CrossRef CAS PubMed.
- A. J. Gosselin, C. A. Rowland and E. D. Bloch, Permanently Microporous Metal–Organic Polyhedra, Chem. Rev., 2020, 120, 8987–9014 CrossRef CAS PubMed.
- W.-Y. Pei, J. Yang, H. Wu, W. Zhou, Y.-W. Yang and J.-F. Ma, A calix 4 resorcinarene-based giant coordination cage: controlled assembly and iodine uptake, Chem. Commun., 2020, 56, 2491–2494 RSC.
- X. Zhang, J. Maddock, T. M. Nenoff, M. A. Denecke, S. Yang and M. Schroder, Adsorption of iodine in metal–organic framework materials, Chem. Soc. Rev., 2022, 51, 3243–3262 RSC.
- D. F. Sava, M. A. Rodriguez, K. W. Chapman, P. J. Chupas, J. A. Greathouse, P. S. Crozier and T. M. Nenoff, Capture of Volatile Iodine, a Gaseous Fission Product, by Zeolitic Imidazolate Framework-8, J. Am. Chem. Soc., 2011, 133, 12398–12401 CrossRef CAS.
- J. Maddock, X. Kang, L. Liu, B. Han, S. Yang and M. Schröder, The Impact of Structural Defects on Iodine Adsorption in UiO-66, Chemistry), 2021, 3, 525–531 CAS.
- D. F. Sava, K. W. Chapman, M. A. Rodriguez, J. A. Greathouse, P. S. Crozier, H. Zhao, P. J. Chupas and T. M. Nenoff, Competitive I2 Sorption by Cu-BTC from Humid Gas Streams, Chem. Mater., 2013, 25, 2591–2596 CrossRef CAS.
- D. Luo, Y. He, J. Tian, J. L. Sessler and X. Chi, Reversible Iodine Capture by Nonporous Adaptive Crystals of a Bipyridine Cage, J. Am. Chem. Soc., 2022, 144, 113–117 CrossRef CAS PubMed.
- H. J. Choi and M. P. Suh, Dynamic and Redox Active Pillared Bilayer Open Framework: Single-Crystal-to-Single-Crystal Transformations upon Guest Removal, Guest Exchange, and Framework Oxidation, J. Am. Chem. Soc., 2004, 126, 15844–15851 CrossRef CAS PubMed.
- X. Zhang, I. Da Silva, R. Fazzi, A. M. Sheveleva, X. Han, B. F. Spencer, S. A. Sapchenko, F. Tuna, E. J. L. McInnes, M. Li, S. Yang and M. Schröder, Iodine Adsorption in a Redox-Active Metal–Organic Framework: Electrical Conductivity Induced by Host–Guest Charge-Transfer, Inorg. Chem., 2019, 58, 14145–14150 CrossRef CAS PubMed.
- D. K. L. Harijan, V. Chandra, T. Yoon and K. S. Kim, Radioactive iodine capture and storage from water using magnetite nanoparticles encapsulated in polypyrrole, J. Hazard. Mater., 2018, 344, 576–584 CrossRef CAS PubMed.
- F. Yu, Y. Chen, Y. Wang, C. Liu and J. Qin, Synthesis of metal-organic framework nanocrystals immobilized with 3D flowerlike Cu-Bi-layered double hydroxides for iodine efficient removal, J. Mater. Res., 2020, 35, 299–311 CrossRef CAS.
- X. Liu, A. Zhang, R. Ma, B. Wu, T. Wen, Y. Ai, M. Sun, J. Jin, S. Wang and X. Wang, Experimental and theoretical insights into copper phthalocyanine-based covalent organic frameworks for highly efficient radioactive iodine capture, Chin. Chem. Lett., 2022, 33, 3549–3555 CrossRef CAS.
- K. Su, W. Wang, B. Li and D. Yuan, Azo-Bridged Calix 4 resorcinarene-Based Porous Organic Frameworks with Highly Efficient Enrichment of Volatile Iodine, ACS Sustainable Chem. Eng., 2018, 6, 17402–17409 CrossRef CAS.
- X. Li, H. Xiong and Q. Jia, A Versatile Solvent-Induced Polymerization Strategy To Synthesize Free-Standing Porous Polymer Nanosheets and Nanotubes for Fast Iodine Capture, ACS Appl. Mater. Interfaces, 2019, 11, 46205–46211 CrossRef CAS PubMed.
- Y. Liao, J. Weber, B. M. Mills, Z. Ren and C. F. J. Faul, Highly Efficient and Reversible Iodine Capture in Hexaphenylbenzene-Based Conjugated Microporous Polymers, Macromolecules, 2016, 49, 6322–6333 CrossRef CAS.
- T. Geng, S. Ye, Z. Zhu and W. Zhang, Triazine-based conjugated microporous polymers with N,N,N′,N’-tetraphenyl-1,4-phenylenediamine,1,3,5-tris(diphenylamino)benzene and 1,3,5-tris (3-methylphenyl)-phenylamino benzene as the core for high iodine capture and fluorescence sensing of o-nitrophenol, J. Mater. Chem. A, 2018, 6, 2808–2816 RSC.
- H. J. Choi and M. P. Suh, Dynamic and redox active pillared bilayer open framework: Single-crystal-to-single-crystal transformations upon guest removal, guest exchange, and framework oxidation, J. Am. Chem. Soc., 2004, 126, 15844–15851 CrossRef CAS PubMed.
Footnote |
† Electronic supplementary information (ESI) available: Additional characterization data, including 1H NMR spectra, single-crystal X-ray diffraction data, IR spectra, TGA curves, PXRD patterns and UV-vis spectra. CCDC 2278526 and 2278527. For ESI and crystallographic data in CIF or other electronic format see DOI: https://doi.org/10.1039/d3qi01501g |
|
This journal is © the Partner Organisations 2023 |
Click here to see how this site uses Cookies. View our privacy policy here.