DOI:
10.1039/D2QI02484E
(Research Article)
Inorg. Chem. Front., 2023,
10, 1436-1446
Immobilization of Brønsted basic hexaniobate on the Lewis acidic zirconia using an emulsion assisted self-assembly strategy for synergistic boosting of nerve agent simulant decontamination†
Received
23rd November 2022
, Accepted 13th January 2023
First published on 13th January 2023
Abstract
The hydrolytic decontamination of organophosphorus nerve agents can be promoted using Brønsted bases or Lewis acids, but the fabrication of catalytic materials with both Lewis acidic centers and Brønsted base sites is a great challenge due to their essential incompatibility. Herein, a facile and straightforward emulsion assisted self-assembly approach has been developed to immobilize a Brønsted basic hexaniobate cluster on the Lewis acidic zirconia. The obtained [C16H33N(CH3)3]6KHNb6O19/ZrO2 (C16N-Nb6/ZrO2) exhibits a remarkable catalytic activity for the hydrolysis of a Sarin simulant (DMNP): 100% of DMNP was converted under ambient conditions without using basic additives. Based on the control experiments and spectroscopic analyses, a possible reaction mechanism was proposed, suggesting that such excellent catalytic performance of C16N-Nb6/ZrO2 is attributed to the synergistic effect between molecular level accessible Lewis acidic ZrO2 (activating substrate molecules) and Brønsted basic hexaniobates (activating water molecules). As a broad-spectrum catalyst, C16N-Nb6/ZrO2 also shows satisfactory performance for the oxidative decontamination of a sulfur mustard simulant.
Introduction
Organophosphates have historically been used as chemical warfare agents (nerve agents, such as Sarin, Soman and VX) since World War I, and to date, they are still employed as insecticides or used for terrorist purposes. They continue to pose a safety and security threat to both environment and humans as they can cause rapid death by inhibiting signal transmission from the central nervous system to muscles.1–4 In this regard, there is a compelling need to develop viable strategies to decontaminate organophosphorus nerve agents in a facile and eco-friendly way. Hydrolysis using Brønsted bases or Lewis acids is a primary way to break the P–X bonds (X = F, CN, etc.) in organophosphorus nerve agents.5 Although basic hydrolysis is effective for Sarin and Soman, the use of stoichiometric strong alkalis makes this route less attractive. Recently, the hydrolysis using Lewis acid catalysts, especially Zr-containing catalysts, has attracted increasing attention.6–11 Since the strongly Lewis acidic Zr sites are available to bind and activate the P
O bond, the hydrolysis kinetics of organophosphonates is significantly improved.12–14 The work of G. W. Peterson's group reveals that zirconium hydroxide is a promising candidate for hydrolytic decontamination of Soman and VX.15 In addition, a series of Zr-based metal–organic frameworks (MOFs) exhibit excellent catalytic activities for the hydrolysis of nerve agents, but their performance highly depends on the use of a basic buffer (N-ethylmorpholine, pH = 10).16–19 Such results imply that the incorporation of Lewis acid catalysts with Brønsted base sites can boost the hydrolytic decontamination of nerve agents.20,21 Nevertheless, the combination of the Lewis acid catalyst with the Brønsted base site is a great synthetic challenge due to their essential incompatibility.
Polyoxoniobates (PONbs) are a subfamily of polyoxometalates (POMs)22–24 and both their synthesis chemistry and catalysis chemistry are far behind those of other POM members.25–31 Recently, the catalytic activities of PONbs in the decontamination of chemical warfare agents and/or their simulants have been explored. Our investigations show that PONbs can promote the oxidative detoxification of sulfur mustard and VX simulants due to their ability to activate hydrogen peroxide.32–36 In addition, PONbs have basic surface oxygen atoms,37–39 which can accelerate the hydrolysis of nerve agents.40,41 Nyman et al. initially demonstrated the hydrolysis of Sarin and Soman using homogeneous Lindqvist [Nb6O19]8−.42 Then, Hill and Zheng reported that Keggin-type PONb, K12[Ti2O2][XNb12O40] (X = Ge or Si), and copper-containing PONb, H2Li5Na5K5[Cu(en)2]7[Nb47O128(OH)6(CO3)2]·20H2O (en = ethylenediamine), as solid bases can catalyze the hydrolysis of nerve agent simulants, but their sluggish hydrolysis kinetics leaves much room for improvement.43,44 Considering the good catalytic performances of Zr-based catalysts in the hydrolysis reaction, the immobilization of basic PONbs on the Lewis acidic supports would provide an efficient way to realize the rapid decontamination of nerve agents. However, the immobilization of PONbs, which are stable under alkaline conditions with a high negative charge density, is difficult.33,45,46 To our knowledge, the combination of PONbs with a Lewis acid supporter is rarely explored.
Emulsion assisted self-assembly has been proved to be a facile and straightforward method to prepare functionalized materials, such as polymer particles, graphene oxide and metal oxides.47–50 In the self-assembly process, an emulsion was formed in the presence of surfactants and the reactions (such as polymerization, hydrolysis, etc.) occur at the interface of the emulsion droplets, leading to the materials with a high specific surface area, tunable size and structure, and narrow molecular weight distribution. Recently, a series of amphiphilic POMs were prepared by the electrostatic assembly of polyanions with surfactants, which not only can stabilize an emulsion but also catalyze organic reactions at the liquid/liquid interface.51–54 However, the emulsion stabilized by surfactant modified POMs was rarely used to prepare POM-based materials. To our knowledge, the only example was reported in Liu's group where a POM modified polystyrene latex particle was prepared by the emulsion assisted assembly using methyl methacrylate functionalized tri-vacant Keggin-type {PW9O37} as a surfactant.55
In our previous study, amphiphilic PONbs ([CnH2n+1N(CH3)3]7HNb6O19, n = 14, 16 and 18) were synthesized by the electrostatic assembly of hexaniobate with quaternary ammoniums with a long alkyl chain, which can catalyze hydrolytic and oxidative detoxification of nerve agent and sulfur mustard simulants in an emulsion system.35 To advance our research in the design and construction of PONb-based decontamination materials, herein the Brønsted basic hexaniobate was immobilized on the Lewis acidic zirconia by a facile emulsion assisted self-assembly using amphiphilic [C16H33N(CH3)3]6KHNb6O19 (C16N-Nb6) as an emulsifier. As a combination of the Brønsted basic Nb6 with Lewis acidic Zr sites, the synthesized C16N-Nb6/ZrO2 exhibits remarkable catalytic performance towards the hydrolytic degradation of dimethyl 4-nitrophenyl phosphate (DMNP), a nerve agent simulant, under basic additive-free conditions. Moreover, the synergistic catalytic mechanism was supported by the control experiments and spectroscopic analyses.
Results and discussion
Synthesis and characterization of the C16N-Nb6/ZrO2 composite
The fabrication of the C16N-Nb6/ZrO2 composite is illustrated in Scheme 1. First, a stable water-in-oil (W/O) type emulsion (Fig. S1†) was formed by vigorously stirring a mixture of water and toluene containing the amphiphilic hexaniobate (C16N-Nb6). Then, zirconium n-butoxide (ZB) was dropped slowly into the emulsion and gradually dispersed to the water–oil interface, where ZrO2 was in situ generated by hydrolysis and simultaneously assembled with C16N-Nb6 to form the C16N-Nb6/ZrO2 composite. During the assembly process, the amphiphilic hexaniobate plays dual roles: as a PONb source and an emulsifier. To our knowledge, this is the first example of using an emulsion to prepare PONb-based materials. Compared with the reported methods for preparing POM-based materials,56–59 the emulsion assisted self-assembly strategy is facile and straightforward. In addition, the control experiments reveal that the Nb6 loading amount can be readily regulated by the mass ratio of C16N-Nb6 to the zirconium n-butoxide precursor, and according to inductively coupled plasma atomic emission spectrometry (ICP-AES), five composites with Nb6 loading amounts of 4% (4%-C16N-Nb6/ZrO2), 6% (6%-C16N-Nb6/ZrO2), 9% (9%-C16N-Nb6/ZrO2), 11% (11%-C16N-Nb6/ZrO2) and 19% (19%-C16N-Nb6/ZrO2) were obtained (Table S1†), respectively. C16N-Nb6/ZrO2 with an Nb6 loading amount of 11% was used in the following characterization.
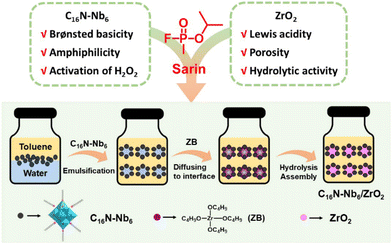 |
| Scheme 1 Illustration of the design and fabrication of the C16N-Nb6/ZrO2 composite using an emulsion assisted self-assembly approach. | |
The Fourier transform infrared (FT-IR) spectra of C16N-Nb6/ZrO2, C16N-Nb6 and ZrO2 are illustrated in Fig. 1a and S2.† C16N-Nb6 displays three characteristic peaks in the region of 500–1000 cm−1, attributed to the stretching vibrations of the Nb–Ob–Nb (555 and 645 cm−1, b: bridge oxygen atoms) and Nb
Ot (879 cm−1, t: terminal oxygen atoms) bonds in the hexaniobate cluster. The peaks at 2971 and 2848 cm−1 belong to the asymmetric vibration of CH2 in the organic ammonium cation.35 For ZrO2, the weak peak at 838 cm−1 is assigned to the stretching vibration of the Zr–O bond and the broad bands at 1622 and 1331 cm−1 are associated with the bending vibration of water molecules.60 The characteristic peaks of Nb6, cetyltrimethyl ammonium cation and ZrO2 can be observed in the FT-IR spectrum of C16N-Nb6/ZrO2 (red line in Fig. 1a and S2†), suggesting the successful combination of C16N-Nb6 with ZrO2. The vibrations of Nb6 are rather weak due to its overlap with the strong absorption bands of ZrO2. We speculate that during the assembly process, the organic ammonium cation acts as a protective agent to improve the compatibility of the basic Nb6 cluster with the acidic ZrO2. According to the TG curves of C16N-Nb6 and C16N-Nb6/ZrO2 (Fig. S3†), in the composite the weight percentages of C16N-Nb6 and ZrO2 are about 49.4 wt% and 50.6 wt%, respectively.
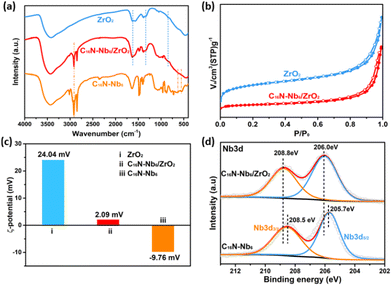 |
| Fig. 1 (a) The FT-IR spectra of C16N-Nb6/ZrO2, C16N-Nb6 and ZrO2; (b) N2 adsorption and desorption isotherms of C16N-Nb6/ZrO2 and ZrO2 measured at 77 K; (c) zeta potential distribution of C16N-Nb6/ZrO2, C16N-Nb6 and ZrO2 in water; (d) high-resolution Nb 3d XPS spectra of C16N-Nb6/ZrO2 and C16N-Nb6. | |
The N2 adsorption and desorption isotherms of C16N-Nb6/ZrO2 and ZrO2 are presented in Fig. 1b. C16N-Nb6/ZrO2 shows a type IV isotherm pattern with a hysteresis loop, indicating the presence of mesopores in the composite. The Brunauer–Emmett–Teller (BET) surface area of C16N-Nb6/ZrO2 is 41.6 m2 g−1, which is obviously lower than that of the ZrO2 (242.8 m2 g−1, Table S2†). As shown in Fig. 1c, the ZrO2 dispersed in an aqueous medium exhibits a positive charge (ξ = 24.04 mV), probably due to the protonation of the surface O atom.61,62 After combining with C16N-Nb6 (ξ = −9.76 mV), the zeta potential of C16N-Nb6/ZrO2 shifts to a lower value (ξ = 2.09 mV). Based on the above results, it can be concluded that the change in the specific surface area and surface potential distribution reveals the successful immobilization of C16N-Nb6 on the ZrO2.
To further confirm the elemental compositions and oxidation states, the X-ray photoelectron spectrum (XPS) of the as-synthesized C16N-Nb6/ZrO2 was recorded and compared with those of C16N-Nb6 and ZrO2. The survey XPS spectrum in Fig. S3† exhibits the presence of Zr, Nb, C, N and O elements in C16N-Nb6/ZrO2. The high-resolution XPS Nb 3d spectrum of C16N-Nb6/ZrO2 displays two peaks at 208.8 eV and 206.0 eV (Fig. 1d), which can be assigned to the Nb 3d5/2 and Nb 3d3/2 with a +5 oxidation state, respectively.33 Compared with that of the C16N-Nb6 precursor (two peaks at 208.5 eV and 205.7 eV correspond to Nb 3d5/2 and Nb 3d3/2, respectively), the binding energy of Nb 3d in C16N-Nb6/ZrO2 shifts to a higher region by about 0.3 eV, demonstrating the strong interaction between C16N-Nb6 and ZrO2 in the C16N-Nb6/ZrO2 composite.
The morphology of C16N-Nb6/ZrO2 is investigated by scanning electron microscopy (SEM) and high-resolution transmission electron microscopy (HRTEM). The SEM image of C16N-Nb6/ZrO2 (Fig. 2a) shows small particles with a diameter of about 20 nm, which is in line with the morphology of the reported ZrO2-based materials.63,64 No lattice fringe was observed in the HRTEM image (Fig. 2b), demonstrating the amorphous structure of the as-prepared composite. In addition, high-angle annular dark-field scanning transmission electron microscopy (HAADF-STEM) and energy dispersive X-ray (EDX) elemental mapping were carried out to further determine the elemental distribution. As shown in the HAADF-STEM image (Fig. 2c), the heavy atoms (such as Zr and Nb) are brighter than the light atoms (such as O and N). The EDX mapping analysis reveals that niobium, zirconium and nitrogen are evenly dispersed in the C16N-Nb6/ZrO2 composite (Fig. 2d–f). Moreover, broad diffraction PXRD peaks of C16N-Nb6/ZrO2 indicate that the as-prepared composite is amorphous (Fig. S4†). There is no characteristic diffraction of C16N-Nb6, further revealing that the hexaniobate clusters are uniformly dispersed in the C16N-Nb6/ZrO2 composite. This result indicates that the emulsion droplets stabilized by amphiphilic PONb provide a special reaction environment, where C16N-Nb6 assembles with in situ formed ZrO2 in a controlled way.
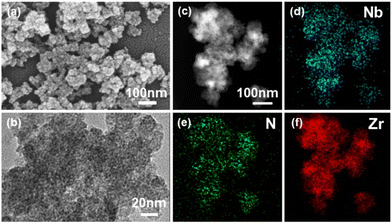 |
| Fig. 2 (a) SEM image, (b) TEM image, (c) HAADF-STEM image, and (d–f) EDX elemental mappings of the C16N-Nb6/ZrO2 composite. | |
In addition, the water contact angles of C16N-Nb6/ZrO2, ZrO2, and C16N-Nb6 were measured. ZrO2 is hydrophilic with a water contact angle of 26° (Fig. S5†). The combination of ZrO2 with C16N-Nb6 makes the C16N-Nb6/ZrO2 composite (with a contact angle of 61°) to have similar surface wettability to that of C16N-Nb6 (with a contact angle of 64°). Importantly, the enhancement of the surface hydrophobicity would promote the contact of organic substrates (such as DMNP) with the hexaniobate and Zr sites on the composite, thereby accelerating the hydrolytic rate of nerve agents.
Hydrolytic decontamination of the nerve agent simulant
Given the coexistence of Zr centers (Lewis acid sites) and hexaniobate clusters (Brønsted base sites) in the as-prepared C16N-Nb6/ZrO2 composite, the hydrolysis of DMNP, a nerve agent simulant, was selected to evaluate its catalytic activity. Before the decontamination study, we tested the acid and base sites of the composite. The surface basicity was first investigated using phenolphthalein as an indicator. As shown in the Fig. 3a inset, a colour change from pale to light pink was observed when phenolphthalein was dropped into the slurry containing C16N-Nb6/ZrO2, while the colour of the ZrO2 slurry remains unchanged. This phenomenon reveals that the introduction of C16N-Nb6 endows the material with Brønsted basicity. To measure the basic site distribution and the basicity capacity of the composite, temperature-programmed desorption of CO2 (CO2-TPD) was conducted. A comparison of the CO2-TPD profiles of C16N-Nb6/ZrO2 and ZrO2 is shown in Fig. 3a, C16N-Nb6/ZrO2 has the maximum CO2 desorption at 126 °C (corresponding to the weak base sites), while ZrO2 reaches the desorption peak at 106 °C. The total basicity capacity of C16N-Nb6/ZrO2 and ZrO2 was calculated to be 13.5 and 0.92 mmol g−1, respectively. These results verify that the immobilization of hexaniobate on the ZrO2 renders the composite with Brønsted base sites. In addition, temperature-programmed desorption of ammonia (NH3-TPD) measurement was performed to detect the Lewis acid sites. As shown in Fig. 3b, the broad desorption peaks at about 148 °C were observed in both the NH3-TPD profiles of C16N-Nb6/ZrO2 and ZrO2, suggesting the presence of weak acid sites.65,66 The density of the Lewis acid sites on C16N-Nb6/ZrO2 was calculated to be 121 mmol g−1, which is slightly lower than that of ZrO2 (133 mmol g−1). The coexistence of Lewis acid sites and Brønsted base sites makes the C16N-Nb6/ZrO2 composite a promising catalyst for the hydrolytic decontamination of nerve agents.
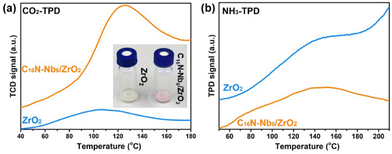 |
| Fig. 3 (a) CO2-TPD of C16N-Nb6/ZrO2 and ZrO2, inset: optical photographs of C16N-Nb6/ZrO2 and ZrO2 slurry after adding phenolphthalein. (b) NH3-TPD of C16N-Nb6/ZrO2 and ZrO2. | |
In a typical reaction, the C16N-Nb6/ZrO2 composite was dispersed in a mixture solution of H2O and CD3CN (volume ratio 3
:
2) with an initial pH value of about 9.3 and DMNP was injected to start the hydrolytic reaction. The decontamination process was performed under ambient conditions without using any basic co-catalysts and the reaction progress was monitored by 31P NMR spectroscopy at various time intervals. As shown in Fig. 4b, the 31P NMR spectral measurements reveal a gradual disappearance of DMNP (δ = −4.8 ppm) and an increase of the peak at δ = 2.6 ppm, attributed to the hydrolysis product, dimethyl phosphate (DMP). Moreover, no toxic byproduct was detected during the hydrolysis (Fig. 4b and S7†). As shown in Fig. 4c, DMNP was completely consumed within 6 h under basic additive-free conditions and the half-life of DMNP is about 30 min. In contrast, a negligible amount of DMNP was converted in the blank experiment without a catalyst (Fig. 5). To our knowledge, the catalytic performance of the C16N-Nb6/ZrO2 composite in the present work outperforms those of most of the reported catalytic materials, such as POMs,26,43,44,67,68 MOFs69 and oxides/hydroxides70 (Table S3†).
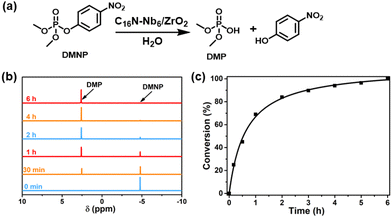 |
| Fig. 4 (a) Catalytic hydrolysis reaction of the phosphonate-based nerve agent simulant DMNP using C16N-Nb6/ZrO2; (b) 31P NMR of DMNP hydrolysis catalyzed by C16N-Nb6/ZrO2 at various intervals of time; (c) time profile for DMNP degradation. Reaction conditions: DMNP (5 mg), H2O (300 μL), CD3CN (200 μL), C16N-Nb6/ZrO2 (15 mg), and room temperature for 6 h. | |
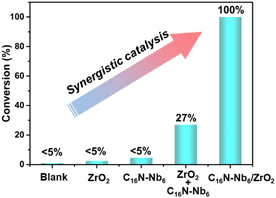 |
| Fig. 5 Catalytic hydrolysis of DMNP with different catalysts without using any basic co-catalysts. Reaction conditions: DMNP (5 mg), H2O (300 μL), CD3CN (200 μL), catalyst, and room temperature for 6 h. | |
To further investigate the influence of Lewis acid sites and Brønsted base sites on the catalytic hydrolysis reaction, the following control experiments were conducted. When ZrO2 was used, only a trace amount of DMNP was converted under the standard decontamination conditions (Table 1, entry 6 and Fig. 5). The amphiphilic C16N-Nb6 also gives a negligible conversion (<5%) (Table 1, entry 7 and Fig. 5). The above results indicate that neither the Lewis acidic ZrO2 nor the Brønsted basic C16N-Nb6 alone can effectively accelerate the decontamination reaction, implying the interesting synergistic effect between the two components. Although the conversion of DMNP can be increased to 27% using a physical mixture of ZrO2 and C16N-Nb6 (Fig. 5), its catalytic activity is still much lower than that of the as-prepared C16N-Nb6/ZrO2 composite (100%). We speculate that the molecular level accessible Lewis acidic sites and Brønsted basic sites play a key role in the rapid hydrolysis of DMNP. In addition, the catalytic performance of C16N-Nb6/ZrO2 is affected by the content of Nb6, the conversion of DMNP increased from 83% to 100% with the loading amount of Nb6 being improved from 4% to 11% (Table 1, entries 1–5), and the C16N-Nb6/ZrO2 with 11% Nb6 loading was used in the following experiments.
Table 1 Hydrolytic degradation of DMNP using various catalystsa
Entry |
Catalyst |
Conditions |
Conv. (%) |
Reaction conditions: DMNP (5 mg), H2O (300 μL), CD3CN (200 μL), catalyst, and room temperature for 6 h. All the hydrolysis of DMNP was performed in a heterogeneous system.
NaOH was added to the reaction mixture to adjust the pH value.
|
1 |
19%-C16-Nb6/ZrO2 |
15 mg |
100 |
2 |
11%-C16-Nb6/ZrO2 |
15 mg |
100 |
3 |
9%-C16-Nb6/ZrO2 |
15 mg |
97 |
4 |
6%-C16-Nb6/ZrO2 |
15 mg |
88 |
5 |
4%-C16-Nb6/ZrO2 |
15 mg |
83 |
6 |
ZrO2 |
13.3 mg |
Trace |
7 |
C16N-Nb6 |
1.7 mg |
Trace |
8b |
ZrO2 + NaOH |
13.3 mg, pH = 9.3 |
29 |
To understand the catalytic role of C16N-Nb6/ZrO2 in the hydrolysis reaction, the composite was treated with DMNP for 1 h in a non-aqueous system and the XPS measurement was performed on the isolated sample. As shown in Fig. 6a, after treating with DMNP, the high-resolution Zr 3d spectrum of C16N-Nb6/ZrO2 exhibits an obvious binding energy shift from 183.3 to 184.2 eV for Zr 3d3/2 and from 181.0 to 181.9 eV for Zr 3d5/2, respectively. The obvious increase of the Zr 3d binding energy (0.9 eV) implies the strong interaction of Zr sites with the substrate, which is consistent with previous reports.71 Furthermore, a new peak at 133.5 eV appeared in the high-resolution P 2p XPS spectrum of DMNP-treated C16N-Nb6/ZrO2 (Fig. 6b), which is assigned to P
O of the surface-bound phosphate species.71 The above results demonstrate that the Lewis acidic Zr sites in the composite can bind and activate the P
O bond of DMNP during the catalytic reaction. The role of solid base Nb6 was also investigated. As the initial pH value of the reaction solution was 9.3 in the presence of C16N-Nb6/ZrO2, we first think that the role of basic C16N-Nb6 is to generate OH− to attack the P center of the substrate. Then, the control experiment using ZrO2 with a pH of 9.3 adjusted using NaOH was performed, which only converted 29% of DMNP (Table 1, entry 8 and Fig. S8†). This result implies that the main role of hexaniobate is not to accept the proton of water to release OH−, but to activate water molecules by its basic surface oxygen atoms.
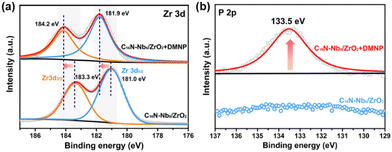 |
| Fig. 6 High-resolution XPS spectra of the C16N-Nb6/ZrO2 composite treated with DMNP in a non-aqueous system: (a) Zr 3d and (b) P 2p. | |
Based on the previous hydrolysis studies in the Zr-containing catalytic system and the above experimental data, we proposed a possible mechanism for the catalytic decontamination of DMNP by C16N-Nb6/ZrO2 (Fig. 7). First, the Lewis acidic Zr centers coordinate with the phosphoryl oxygen of DMNP, activating the P
O bond. Meanwhile, water molecules interact with the bridging oxygen atoms of the Lindqvist hexaniobate cluster to form intermediate A. Subsequently, the oxygen atom of the activated water molecule attacks the P center of adjacent DMNP giving intermediate B with a pentacoordinated P center and a protonated hexaniobate. Then, the elimination of nitrophenoxide consumes the proton on the hexaniobate cluster, leaving the intermediate C (DMP binds to the Zr sites). Finally, the bonded product dissociates to the reaction solution regenerating the C16N-Nb6/ZrO2 catalyst.
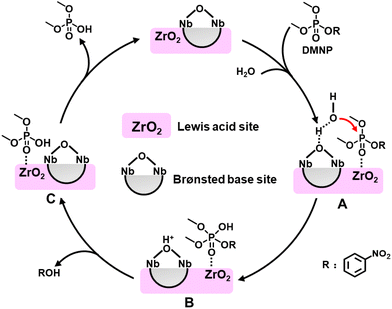 |
| Fig. 7 The possible hydrolytic degradation mechanism for DMNP on the C16N-Nb6/ZrO2 catalyst. | |
The heterogeneous nature of the C16N-Nb6/ZrO2 catalyst was evaluated using the leaching test. After the hydrolysis was performed for 1 h with a conversion of about 70%, the solid catalyst was filtered off from the reaction mixture and the resulting solution continued to react under the otherwise identical conditions. The negligible conversion was observed for another 5 h (Fig. 8a), suggesting that the hexaniobate is stably immobilized on ZrO2. Then, we investigated the long-term stability of the C16N-Nb6/ZrO2. After the first run, the solid catalyst was filtered from the reaction system and used for the next run after washing. Unfortunately, a decrease in DMNP conversion (about 20%) was observed for the first three cycles (Fig. 8b). Based on the literature research,20,21 we speculate that the decrease in the catalytic performance is probably related to the adsorption of the acidic product (DMP) on the catalytic active sites. This point was proved by the following control experiments and spectroscopic analysis. First, an obvious decrease in the catalytic activity of C16N-Nb6/ZrO2 (the conversion of DMNP decreases from 100% to 69%) was observed upon the addition of extra DMP to the standard catalytic reaction. Moreover, after the third run, the isolated catalyst was washed with acetonitrile thoroughly and measured by FT-IR spectroscopy. As shown in Fig. S9,† the characteristic peaks of hexaniobate and ZrO2 were maintained, but new peaks at 1164 cm−1 and 1110 cm−1 assigned to the νa(O–P–O) and νs(O–P–O),71 respectively, were detected. The isolated sample was further characterized by XPS. After three cycles, the peaks of Zr 3d3/2 and Zr 3d5/2 shift to a higher binding energy (Fig. 8c) and a similar trend is observed in the Nb 3d XPS spectra (Fig. 8d), which indicates the interaction of the Zr center and hexaniobate with the acid hydrolysis product (DMP). To gain a better recycle performance, after three cycles the C16N-Nb6/ZrO2 composite was activated by soaking in KOH solution (1 M) and the catalytic activity of C16N-Nb6/ZrO2 was basically recovered after regeneration with 92% and 90% of DMNP conversion in the fourth and fifth runs, respectively (Fig. 8b).
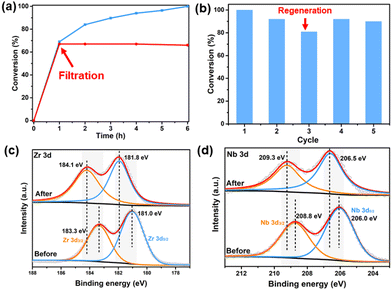 |
| Fig. 8 (a) Leaching experiment for the hydrolysis of DMNP over C16N-Nb6/ZrO2; (b) recycle test for the decontamination reaction using C16N-Nb6/ZrO2 as the catalyst. Reaction conditions: DMNP (5 mg), H2O (300 μL), CD3CN (200 μL), C16N-Nb6/ZrO2 (15 mg), and room temperature for 6 h; (c) high-resolution Zr 3d XPS spectra of C16N-Nb6/ZrO2 before and after the catalytic reaction; and (d) high-resolution Nb 3d XPS spectra of C16N-Nb6/ZrO2 before and after the catalytic reaction. | |
Oxidative decontamination of the sulfur mustard simulant
Our previous works indicate that PONbs are active in the oxidative decontamination of the sulfur mustard simulant 2-chloroethyl ethyl sulfide (CEES) in the presence of dilute aqueous H2O2 (3 wt%).32–36 To explore the broad-spectrum decontamination activity of the as-prepared C16N-Nb6/ZrO2 composite, oxidative degradation of CEES was selected as the second model reaction (Fig. 9a). Under the optimized conditions, 93% of the toxic substrate was consumed after 2 h and 83% of selectivity for the less-toxic product, 2-chloroethyl ethyl sulfoxide (CEESO), was observed using C16N-Nb6/ZrO2 as the catalyst (Fig. 9b and S10, S11†). In contrast, the test without the catalyst (blank test) shows a negligible CEES removal (Conv. 7%).
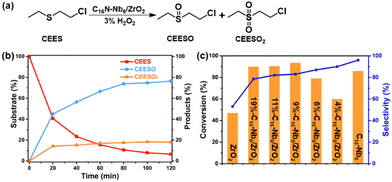 |
| Fig. 9 (a) Catalytic oxidation degradation of the sulfur mustard simulant CEES by C16N-Nb6/ZrO2; (b) concentration profiles of CEES, CEESO, and CEESO2 (2-chloroethyl ethyl sulfone) versus reaction time over C16N-Nb6/ZrO2; reaction conditions: CEES (0.4 mmol), 1,3-dichlorobenzene (0.2 mmol), C16N-Nb6/ZrO2 (10 mg), and aqueous H2O2 (3 wt%, 0.44 mmol) at room temperature for 2 h. (c) Oxidative decontamination of CEES over different catalysts. | |
To evaluate the contribution of ZrO2 and the hexaniobate cluster to the oxidative process, control experiments with different catalysts were conducted. As shown in Fig. 9c, C16N-Nb6 converted 86% of CEES, and a satisfactory selectivity of 96% towards the less toxic CEESO was obtained, while ZrO2 can also interact with H2O2 to form the Zr-peroxo species (Fig. S12†), showing a moderate catalytic performance (Conv. 47%, Sele. 53%). Furthermore, the catalytic activities of the C16N-Nb6/ZrO2 composites with various C16N-Nb6 loadings were tested. A significant improvement in the conversion (from 60% to 94%) was obtained by increasing the loading of Nb6 from 4% to 9%, but a further increase in Nb6 loading did not lead to enhanced performance. Based on the above results, we can conclude that the Nb6 cluster is the main activity center towards the selective oxidation of CEES. In addition, the comparison of FT-IR spectra (Fig. S13†) of the catalyst before and after the oxidative decontamination reaction indicates that the structure of C16N-Nb6/ZrO2 remains stable under the turnover conditions.
Conclusions
In this report, a catalytic decontamination material containing both Brønsted basic hexaniobate and the Lewis acidic Zr sites has been successfully prepared using a facile emulsion-assisted self-assembly strategy. The obtained C16N-Nb6/ZrO2 composite shows remarkable catalytic performance towards the hydrolytic degradation of an organophosphorus nerve agent simulant, DMNP, while C16N-Nb6 or ZrO2 alone shows negligible activity. The in-depth mechanism study indicates that the synergistic effect between C16N-Nb6 and ZrO2 is of great importance to boost the hydrolytic kinetics. Moreover, the C16N-Nb6/ZrO2 composite also shows catalytic activity in the oxidative decontamination of the sulfur mustard simulant. The reported investigation not only provides a highly effective catalyst for the decontamination of chemical warfare agent simulants but also opens up new prospects for the design and fabrication of PONb-based catalytic materials. The combination of surfactant modified PONbs with other functionalized supports is under investigation in our lab.
Experimental section
Materials and methods
All the starting chemicals and solvents were of reagent grade, purchased from commercial sources, and used without further purification. CAUTION: The simulants of CWAs, DMNP and CEES, are highly toxic and must be handled only by trained personnel using applicable safety procedures in a closed system or a hood under good ventilation.
The XRD patterns were obtained on a Shimadzu XRD-6000 instrument with graphite-monochromatized Cu Kα (λ = 1.5406 Å). The XPS analysis was conducted on a PHI VersaProbe III spectrometer using an Al Kα radiation as the X-ray source. The FT-IR spectra were recorded on a JASCO 6300D instrument in the region of 400–4000 cm−1. The Brunauer–Emmett–Teller (BET) specific surface areas were measured at 77 K with a Micromeritics ASAP 2000 instrument, and the pore size distributions were estimated using the Barrett–Joyner–Halenda (BJH) method. Elemental analysis (C, H and N) was performed on an ElementarVario EL cube Elmer CHN elemental analyzer and ICP-MS was performed on a ThermoiCAP 6000 atomic emission spectrometer. SEM (JOEL JSE-7500F), TEM (JEOL JEM-2010) and field emission transmission electron microscopy (FEI Talos F200X) were used to observe the morphology and microstructure of the samples. The acidic and basic sites of the synthesized material were measured by NH3-TPD and CO2-TPD, respectively, with a PCA-1200 instrument. The gas chromatographic (GC) analyses were performed on a Shimadzu GC-2014C equipped with an FID detector and an HP-5 ms capillary column. The GC-MS spectra of the products were obtained using an Agilent 7890A-5975C instrument. The 31P NMR spectra were recorded on a Bruker 400 MHz instrument in CD3CN, and all the chemical shifts were referenced to an aqueous H3PO4 solution (85%).
Preparation of C16N-Nb6/ZrO2
The C16N-Nb6/ZrO2 composite was prepared using an emulsion assisted self-assembly method. First, a certain amount of [C16H33N(CH3)3]4K3HNb6O19 (C16N-Nb6)32 was dispersed in toluene (10 mL) and treated with ultrasound for about 5 min. Then, deionized water (20 mL) was added to the above toluene solution and the mixture was strongly shocked and stirred until a stable emulsion was obtained. A toluene solution (1 mL) containing zirconium n-butoxide was dropped slowly into the above emulsion system (in an ice-bath) and the reaction mixture was stirred for another 6 h. The final white precipitate, C16N-Nb6/ZrO2, was filtered from the reaction mixture, washed with ethanol several times, and dried under vacuum. Various C16N-Nb6/ZrO2 composites with different Nb6 loading amounts were obtained by controlling the ratio of zirconium n-butoxide to C16N-Nb6: 0.3 g of C16N-Nb6 and 0.5 g of zirconium n-butoxide for C16N-Nb6/ZrO2-19%, 0.2 g of C16N-Nb6 and 0.6 g of zirconium n-butoxide for C16N-Nb6/ZrO2-11%, 0.1 g of C16N-Nb6 and 0.4 g of zirconium n-butoxide for C16N-Nb6/ZrO2-9%, 0.1 g of C16N-Nb6 and 0.6 g of zirconium n-butoxide for C16N-Nb6/ZrO2-6%, and 0.1 g of C16N-Nb6 and 0.8 g of zirconium n-butoxide for C16N-Nb6/ZrO2-4%.
Catalytic hydrolysis of DMNP using C16N-Nb6/ZrO2
The as-prepared C16N-Nb6/ZrO2 catalyst (15 mg) was added to a mixture solution of CD3CN (0.2 mL) and water (0.3 mL) and stirred for 5 min to disperse homogeneously. Then, DMNP (100 mg mL−1 in CH3CN, 50 μL) was injected to start the decontamination process. The catalytic hydrolysis reaction was performed with stirring at room temperature and the hydrolysis profiles were recorded by 31P NMR measurements at various intervals of time. The conversion of DMNP was calculated depending on the peak area ratio of the detected DMNP to DMNP adding the products.
Catalytic oxidation of CEES using C16N-Nb6/ZrO2
The as-prepared C16N-Nb6/ZrO2 catalyst (10 mg) was dispersed in CH3CN (3 mL) and to this solution CEES (0.4 mmol) and 1,3-dichlorobenzene (0.2 mmol, internal standard) were added. After stirring for 5 min, aqueous H2O2 (3 wt%, 0.44 mmol) was dropped to initiate the decontamination reaction. The products were qualitatively analyzed by GC-MS and the oxidation profiles were monitored using GC.
Procedure for NH3-TPD and CO2-TPD measurements
NH3-TPD measurements were performed to gain the density distribution and strength of acid sites in C16N-Nb6/ZrO2. First, C16N-Nb6/ZrO2 (25 mg) was pretreated at 150 °C for 120 min under an Ar blow at a flow rate of 30 mL min−1. Then, the sample was exposed to ammonia at 100 °C until saturation and purged under an Ar flow for 30 min to remove the physically adsorbed ammonia before cooling to room temperature. The sample was heated from 40 to 400 °C at a ramping rate of 10 °C min−1 under an Ar atmosphere, and ammonia was detected using a thermal conductivity detector.
CO2-TPD measurements were performed to gain the density distribution and strength of base sites in C16N-Nb6/ZrO2. The procedure for CO2-TPD measurements was similar to that of NH3-TPD, except that He gas was used instead of Ar gas and the adsorption temperature of CO2 was 80 °C.
Treatment of C16N-Nb6/ZrO2 with DMNP
C16N-Nb6/ZrO2 was dispersed in the anhydrous CH3CN solution of DMNP and consecutively stirred for 1 h at room temperature. Then, the solid was separated by filtration, washed and characterized by XPS.
Author contributions
H. F. Liu synthesized and characterized the C16N-Nb6/ZrO2 composite catalyst and J. Dong performed the catalytic hydrolysis of DMNP as well as the oxidation of CEES. X. R. Sun and C. P. Liu helped with data analysis and graphic design. Y. N. Chi and C. W. Hu conceived and directed the research. W. Lu, Z. M. Xu, N. Zhen and D. Zhang gave some advice about the preparation of catalysts and the catalytic experiments.
Conflicts of interest
There are no conflicts to declare.
Acknowledgements
This work was financially supported by the National Natural Science Foundation of China (21871026, 21971010 and 22101012).
Notes and references
- J. B. DeCoste and G. W. Peterson, Metal-Organic Frameworks for Air Purification of Toxic Chemicals, Chem. Rev., 2014, 114, 5695–5727 CrossRef CAS PubMed
.
- N. S. Bobbitt, M. L. Mendonca, A. J. Howarth, T. Islamoglu, J. T. Hupp, O. K. Farha and R. Q. Snurr, Metal-Organic Frameworks for the Removal of Toxic Industrial Chemicals and Chemical Warfare Agents, Chem. Soc. Rev., 2017, 46, 3357–3385 RSC
.
- Y. C. Yang, J. A. Baker and J. R. Ward, Decontamination of Chemical Warfare Agents, Chem. Rev., 1992, 92, 1729–1743 CrossRef CAS
.
- B. M. Smith, Catalytic Methods for the Destruction of Chemical Warfare Agents under Ambient Conditions, Chem. Soc. Rev., 2008, 37, 470–478 RSC
.
- K. Kim, O. G. Tsay, D. A. Atwood and D. G. Churchill, Destruction and Detection of Chemical Warfare Agents, Chem. Rev., 2011, 111, 5345–5403 CrossRef CAS PubMed
.
- K. Vellingiri, L. Philip and K. Kim, Metal-Organic Frameworks as Media for the Catalytic Degradation of Chemical Warfare Agents, Coord. Chem. Rev., 2017, 353, 159–179 CrossRef CAS
.
- Y. Y. Liu, A. J. Howarth, N. A. Vermeulen, S. Y. Moon, J. T. Hupp and O. K. Farha, Catalytic Degradation of Chemical Warfare Agents and their Simulants by Metal-Organic Frameworks, Coord. Chem. Rev., 2017, 346, 101–111 CrossRef CAS
.
- R. B. Balow, J. G. Lundin, G. C. Daniels, W. O. Gordon, M. McEntee, G. W. Peterson, J. H. Wynne and P. E. Pehrsson, Environmental Effects on Zirconium Hydroxide Nanoparticles and Chemical Warfare Agent Decomposition: Implications of Atmospheric Water and Carbon Dioxide, ACS Appl. Mater. Interfaces, 2017, 9, 39747–39757 CrossRef CAS PubMed
.
- J. Colón-Ortiz, J. M. Landers, W. O. Gordon, A. Balboa, C. J. Karwacki and A. V. Neimark, Disordered Mesoporous Zirconium (Hydr)oxides for Decomposition of Dimethyl Chlorophosphate, ACS Appl. Mater. Interfaces, 2019, 11, 17931–17939 CrossRef PubMed
.
- S. Jeon, I. V. Schweigert, P. E. Pehrsson and R. B. Balow, Kinetics of Dimethyl Methylphosphonate Adsorption and Decomposition on Zirconium Hydroxide Using Variable Temperature in Situ Attenuated Total Reflection Infrared Spectroscopy, ACS Appl. Mater. Interfaces, 2020, 12, 14662–14671 CrossRef CAS PubMed
.
- J. W. Long, C. N. Chervin, R. B. Balow, S. Jeon, J. B. Miller, M. E. Helms, J. C. Owrutsky, D. R. Rolison and K. P. Fears, Zirconia-based Aerogels for Sorption and Degradation of Dimethyl Methylphosphonate, Ind. Eng. Chem. Res., 2020, 59, 19584–19592 CrossRef CAS
.
- J. E. Mondloch, M. J. Katz, W. C. Isley III, P. Ghosh, P. L. Liao, W. Bury, G. W. Wagner, M. G. Hall, J. B. DeCoste, G. W. Peterson, R. Q. Snurr, C. J. Cramer, J. T. Hupp and O. K. Farha, Destruction of Chemical Warfare Agents using Metal-Organic Frameworks, Nat. Mater., 2015, 14, 512–516 CrossRef CAS PubMed
.
- M. J. Katz, S. Y. Moon, J. E. Mondloch, M. H. Beyzavi, C. J. Stephenson, J. T. Hupp and O. K. Farha, Exploiting Parameter Space in MOFs: A 20-fold Enhancement of Phosphate-Ester Hydrolysis with UiO-66-NH2, Chem. Sci., 2015, 6, 2286–2291 RSC
.
- K. K. Ma, T. Islamoglu, Z. J. Chen, P. Li, M. C. Wasson, Y. W. Chen, Y. F. Wang, G. W. Peterson, J. H. Xin and O. K. Farha, Scalable and Template-Free Aqueous Synthesis of Zirconium-Based Metal-Organic Framework Coating on Textile Fiber, J. Am. Chem. Soc., 2019, 141, 15626–15633 CrossRef CAS PubMed
.
- T. J. Bandosz, M. Laskoski, J. Mahle, G. Mogilevsky, G. W. Peterson, J. A. Rossin and G. W. Wagner, Reactions of VX, GD, and HD with Zr(OH)4: Near Instantaneous Decontamination of VX, J. Phys. Chem. C, 2012, 116, 11606–11614 CrossRef CAS
.
- S. Y. Moon, Y. Y. Liu, J. T. Hupp and O. K. Farha, Instantaneous Hydrolysis of Nerve-Agent Simulants with a Six-Connected Zirconium-Based Metal-Organic Framework, Angew. Chem., Int. Ed., 2015, 54, 6795–6799 CrossRef CAS PubMed
.
- T. Islamoglu, M. A. Ortuño, E. Proussaloglou, A. J. Howarth, N. A. Vermeulen, A. Atilgan, A. M. Asiri, C. J. Cramer and O. K. Farha, Presence versus Proximity: The Role of Pendant Amines in the Catalytic Hydrolysis of a Nerve Agent Simulant, Angew. Chem., Int. Ed., 2018, 57, 1949–1953 CrossRef CAS PubMed
.
- S. Y. Moon, E. Proussaloglou, G. W. Peterson, J. B. DeCoste, M. G. Hall, A. J. Howarth, J. T. Hupp and O. K. Farha, Detoxification of Chemical Warfare Agents Using a Zr6-Based Metal-Organic Framework/Polymer Mixture, Chem. – Eur. J., 2016, 22, 14864–14868 CrossRef CAS PubMed
.
- Y. Y. Liu, S. Y. Moon, J. T. Hupp and O. K. Farha, Dual-Function Metal Organic Framework as a Versatile Catalyst for Detoxifying Chemical Warfare Agent Simulants, ACS Nano, 2015, 9, 12358–12364 CrossRef CAS PubMed
.
- E. López-Maya, C. Montoro, L. M. Rodríguez-Albelo, S. D. A. Cervantes, A. A. Lozano-Pérez, J. L. Cenís, E. Barea and J. A. R. Navarro, Textile/Metal-Organic-Framework Composites as Self-Detoxifying Filters for Chemical-Warfare Agents, Angew. Chem., Int. Ed., 2015, 54, 6790–6794 CrossRef PubMed
.
- R. Gil-San-Millan, E. López-Maya, M. Hall, N. M. Padial, G. W. Peterson, J. B. DeCoste, L. M. Rodríguez-Albelo, J. E. Oltra, E. Barea and J. A. R. Navarro, Chemical Warfare Agents Detoxification Properties of Zirconium Metal-Organic Frameworks by Synergistic Incorporation of Nucleophilic and Basic Sites, ACS Appl. Mater. Interfaces, 2017, 9, 23967–23973 CrossRef CAS PubMed
.
- H. Y. Zhao, Y. Z. Li, J. W. Zhao, L. Wang and G. Y. Yang, State-of-the-Art Advances in the Structural Diversities and Catalytic Applications of Polyoxoniobate-based Materials, Coord. Chem. Rev., 2021, 440, 213966 CrossRef
.
- M. Nyman, Polyoxoniobate Chemistry in the 21st Century, Dalton Trans., 2011, 40, 8049–8058 RSC
.
- H. L. Wu, Z. M. Zhang, Y. G. Li, X. L. Wang and E. B. Wang, Recent Progress in Polyoxoniobates Decorated and Stabilized via Transition Metal Cations or Clusters, CrystEngComm, 2015, 17, 6261–6268 RSC
.
- Y. Y. Wu, J. Dong, C. P. Liu, X. T. Jing, H. F. Liu, Y. Guo, Y. N. Chi and C. W. Hu, Reduced Polyoxomolybdate Immobilized on Reduced Graphene Oxide for Rapid Catalytic Decontamination of a Sulfur Mustard Simulant, Dalton Trans., 2021, 50, 9796–9803 RSC
.
- D. Zhang, W. Q. Zhang, Z. G. Lin, J. Dong, N. Zhen, Y. N. Chi and C. W. Hu, Mono- and Di-Sc-Substituted Keggin Polyoxometalates: Effective Lewis Acid Catalysts for Nerve Agent Simulant Hydrolysis and Mechanistic Insights, Inorg. Chem., 2020, 59, 9756–9764 CrossRef CAS PubMed
.
- X. R. Sun, J. Dong, Z. Li, H. F. Liu, X. T. Jing, Y. N. Chi and C. W. Hu, Mono-Transition-Metal-Substituted Polyoxometalate Intercalated Layered Double Hydroxides for the Catalytic Decontamination of Sulfur Mustard Simulant, Dalton Trans., 2019, 48, 5285–5291 RSC
.
- X. Q. Huang, S. Liu, G. Liu, Y. W. Tao, C. R. Wang, Y. L. Zhang, Z. Li, H. W. Wang, Z. Zhou, G. D. Shen, Z. C. Xue and D. Sun, An Unprecedented 2-fold Interpenetrated Lvt Open Framework Built from Zn6 Ring Seamed Trivacant Polyoxotungstates used for Photocatalytic Synthesis of Pyridine Derivatives, Appl. Catal., B, 2023, 323, 122134 CrossRef CAS
.
- G. P. Yang, K. Li and C. W. Hu, Recent Advances in Uranium-Containing Polyoxometalates, Inorg. Chem. Front., 2022, 9, 5408–5433 RSC
.
- G. P. Yang, X. L. Zhang, Y. F. Liu, D. D. Zhang, K. Li and C. W. Hu, Self-Assembly of Keggin-type U(VI)-Containing Tungstophosphates with a Sandwich Structure: An Efficient Catalyst for the Synthesis of Sulfonyl Pyrazoles, Inorg. Chem. Front., 2021, 8, 4650–4656 RSC
.
- J. Zhou, T. Yu, K. Li, K. Zeng, G. P. Yang and C. W. Hu, Two U(VI)-Containing Silicotungstates with Sandwich Structures: Lewis Acid-Base Synergistic Catalyzed Synthesis of Benzodiazepines and Pyrazoles, Inorg. Chem., 2022, 61, 3050–3057 CrossRef CAS PubMed
.
- J. Dong, J. F. Hu, Y. N. Chi, Z. G. Lin, B. Zou, S. Yang, C. L. Hill and C. W. Hu, Polyoxoniobate-Polyoxovanadate Double-Anion Catalyst for Simultaneous Oxidative and Hydrolytic Decontamination of Chemical Warfare Agent Simulants, Angew. Chem., Int. Ed., 2017, 56, 4473–4477 CrossRef CAS PubMed
.
- J. Dong, H. J. Lv, X. R. Sun, Y. Wang, Y. M. Ni, B. Zou, N. Zhang, A. X. Yin, Y. N. Chi and C. W. Hu, A Versatile Self-Detoxifying Material Based on Immobilized Polyoxoniobate for Decontamination of Chemical Warfare Agent Simulants, Chem. – Eur. J., 2018, 24, 19208–19215 CrossRef CAS PubMed
.
- J. Dong, X. R. Sun, N. Zhen, Z. Li, D. Liu, B. Zou, Q. P. Dai, Y. N. Chi, S. L. Chen, J. M. Poblet and C. W. Hu, Oxidative Detoxification of Nerve Agent VX Simulant by Polyoxoniobate: Experimental and Theoretical Insights, J. Catal., 2021, 394, 83–93 CrossRef CAS
.
- X. Q. Li, J. Dong, H. F. Liu, X. R. Sun, Y. N. Chi and C. W. Hu, Recoverable Amphiphilic Polyoxoniobates Catalyzing Oxidative and Hydrolytic Decontamination of Chemical Warfare Agent Simulants in Emulsion, J. Hazard. Mater., 2018, 344, 994–999 CrossRef CAS PubMed
.
- N. Zhen, J. Dong, Z. G. Lin, X. X. Li, Y. N. Chi and C. W. Hu, Self-Assembly of Polyoxovanadate-Capped Polyoxoniobates and their Catalytic Decontamination of Sulfur Mustard Simulants, Chem. Commun., 2020, 56, 13967–13970 RSC
.
- S. Kikkawa, S. Fukuda, J. Hirayama, N. Shirai, R. Takahata, K. Suzuki, K. Yamaguchi, T. Teranishi and S. Tamazoe, Dual Functional Catalysis of [Nb6O19]8−-Modified Au/Al2O3, Chem. Commun., 2022, 58, 9018–9021 RSC
.
- S. Hayashi, S. Yamazoe and T. Tsukuda, Base Catalytic Activity of [Nb10O28]6−: Effect of Countercations, J. Phys. Chem. C, 2020, 124, 10975–10980 CrossRef CAS
.
- S. Hayashi, N. Sasaki, S. Yamazoe and T. Tsukuda, Superior Base Catalysis of Group 5 Hexametalates [M6O19]8− (M = Ta, Nb) over Group 6 Hexametalates [M6O19]2− (M = Mo, W), J. Phys. Chem. C, 2018, 122, 29398–29404 CrossRef CAS
.
- A. L. Kaledin, D. M. Driscoll, D. Troya, D. L. Collins-Wildman, C. L. Hill, J. R. Morris and D. G. Musaev, Impact of Ambient Gases on the Mechanism of [Cs8Nb6O19]-Promoted Nerve-Agent Decomposition, Chem. Sci., 2018, 9, 2147–2158 RSC
.
- Y. Y. Tian, A. L. Kaledin, D. L. Collins-Wildman, V. G. Snider, D. G. Musaev, C. L. Hill and A. I. Frenkel, Polyniobate Nanothreads for Decomposition of the Nerve Agent Simulant Dimethyl Chlorophosphate, ACS Appl. Nano Mater., 2021, 4, 5649–5654 CrossRef CAS
.
- M. K. Kinnan, W. R. Creasy, L. B. Fullmer, H. L. Schreuder-Gibson and M. Nyman, Nerve Agent Degradation with Polyoxoniobates, Eur. J. Inorg. Chem., 2014, 2361–2367 CrossRef CAS
.
- W. W. Guo, H. J. Lv, K. P. Sullivan, W. O. Gordon, A. Balboa, G. W. Wagner, D. G. Musaev, J. Bacsa and C. L. Hill, Broad-Spectrum Liquid- and Gas-Phase Decontamination of Chemical Warfare Agents by One-Dimensional Heteropolyniobates, Angew. Chem., Int. Ed., 2016, 55, 7403–7407 CrossRef CAS PubMed
.
- Y. L. Wu, X. X. Li, Y. J. Qi, H. Yu, L. Jin and S. T. Zheng, {Nb288O768(OH)48(CO3)12}: A Macromolecular Polyoxometalate with Niobium Atoms Close to 300, Angew. Chem., Int. Ed., 2018, 57, 8572–8576 CrossRef CAS PubMed
.
- S. L. Heng, L. Li, W. W. Li, H. Y. Li, J. Y. Pang, M. Z. Zhang, Y. Bai and D. B. Dang, Enhanced Photocatalytic Hydrogen Production of the Polyoxoniobate Modified with RGO and PPy, Nanomaterials, 2020, 10, 2449 CrossRef CAS PubMed
.
- J. Zheng, S. H. Fan, S. Liu, G. D. Shen, W. D. Si, X. Y. Dong, X. Q. Huang, Y. L. Zhang, Q. X. Yao, Z. Li and D. Sun, In Situ Ball-Milling Gram-Scale Preparation of Polyoxoniobate-Intercalated MgAl-Layered Double Hydroxides for Selective Aldol and Michael Addition Cascade Reactions, Inorg. Chem. Front., 2022, 9, 5607–5615 RSC
.
- Q. S. Xu, T. Huang, S. L. Li, K. Li, C. L. Li, Y. N. Liu, Y. L. Wang, C. Y. Yu and Y. F. Zhou, Emulsion-Assisted Polymerization-Induced Hierarchical Self-Assembly of Giant Sea Urchin-like Aggregates on a Large Scale, Angew. Chem., Int. Ed., 2018, 57, 8043–8047 CrossRef CAS PubMed
.
- F. Yue, G. Q. Gao, F. T. Li, Y. Q. Zheng and S. F. Hou, Size-Controlled Synthesis of Urchin-like Reduced Graphene Oxide Microspheres with Highly Packed Density by Emulsion-Assisted in-situ Assembly and their Supercapacitor Performance, Carbon, 2018, 134, 112–122 CrossRef CAS
.
- W. Liu, G. Yang, M. P. Huang, J. W. Liang, B. B. Zeng, C. Fu and H. D. Wu, Ultrarobust and Biomimetic Hierarchically Macroporous Ceramic Membrane for Oil-Water Separation Templated by Emulsion-Assisted Self-Assembly Method, ACS Appl. Mater. Interfaces, 2020, 12, 35555–35562 CrossRef CAS PubMed
.
- Z. Yin, D. Ma and X. H. Bao, Emulsion-Assisted Synthesis of Monodisperse Binary Metal Nanoparticles, Chem. Commun., 2010, 46, 1344–1346 RSC
.
- J. H. Xu, S. Zhao, Y. C. Ji and Y. F. Song, Deep Desulfurization by Amphiphilic Lanthanide-Containing Polyoxometalates in Ionic-Liquid Emulsion Systems under Mild Conditions, Chem. – Eur. J., 2013, 19, 709–715 CrossRef CAS PubMed
.
- A. Nisar, Y. Lu, J. Zhuang and X. Wang, Polyoxometalate Nanocone Nanoreactors: Magnetic Manipulation and Enhanced Catalytic Performance, Angew. Chem., 2011, 123, 3245–3250 CrossRef
.
- A. Nisar, J. Zhuang and X. Wang, Construction of Amphiphilic Polyoxometalate Mesostructures as a Highly Efficient Desulfurization Catalyst, Adv. Mater., 2011, 23, 1130–1135 CrossRef CAS PubMed
.
- H. L. Li, H. Sun, W. Qi, M. Xu and L. X. Wu, Onionlike Hybrid Assemblies Based on Surfactant-Encapsulated Polyoxometalates, Angew. Chem., Int. Ed., 2007, 46, 1300–1303 CrossRef CAS PubMed
.
- X. Y. Chen, H. Li, P. C. Yin and T. B. Liu, Design of Polystyrene Latex Particles Covered with Polyoxometalate Clusters via Multiple Covalent Bonding, Chem. Commun., 2015, 51, 6104–6107 RSC
.
- S. Mukhopadhyay, J. Debgupta, C. Singh, A. Kar and S. K. Das, A Keggin Polyoxometalate Shows Water Oxidation Activity at Neutral pH: POM@ZIF-8, an Efficient and Robust Electrocatalyst, Angew. Chem., Int. Ed., 2018, 57, 1918–1923 CrossRef CAS PubMed
.
- Z. M. Zhang, T. Zhang, C. Wang, Z. K. Lin, L. S. Long and W. B. Lin, Photosensitizing Metal-Organic Framework Enabling
Visible-Light-Driven Proton Reduction by a Wells-Dawson-Type Polyoxometalate, J. Am. Chem. Soc., 2015, 137, 3197–3200 CrossRef CAS PubMed
.
- Y. W. Liu, S. M. Liu, X. Y. Lai, J. Miao, D. F. He, N. Li, F. Luo, Z. Shi and S. X. Liu, Polyoxometalate-Modified Sponge-Like Graphene Oxide Monolith with High Proton-Conducting Performance, Adv. Funct. Mater., 2015, 25, 4480–4485 CrossRef CAS
.
- H. M. Asif, Y. S. Zhou, L. J. Zhang, N. Shaheen, D. Yang, J. Q. Li, Y. Long, A. Iqbal and Y. Q. Li, Covalent Synthesis of Two Hybrids Composed of Dawson-Type Polyoxometalate and Porphyrin with Remarkable Third-Order Optical Nonlinearities Reflecting the Effect of Polyoxometalates, Inorg. Chem., 2017, 56, 9436–9447 CrossRef CAS PubMed
.
- L. Ullah, G. Y. Zhao, J. X. Ma, M. Usman, R. Khan and N. Hedin, Pd-Promoted Heteropolyacid on Mesoporous Zirconia as a Stable and Bifunctional Catalyst for Oxidation of Thiophenes, Fuel, 2022, 310, 122462 CrossRef CAS
.
- K. Shehzad, M. Ahmad, J. Y. He, T. Liu, W. H. Xu and J. H. Liu, Synthesis of Ultra-Large ZrO2 Nanosheets as Novel Adsorbents for Fast and Efficient Removal of As(III) from Aqueous Solutions, J. Colloid Interface Sci., 2019, 533, 588–597 CrossRef CAS PubMed
.
- S. Z. Wang, S. F. Zhou, J. Huang, G. Z. Zhao and Y. Q. Liu, Attaching ZrO2 Nanoparticles onto the Surface of Graphene Oxide via Electrostatic Self-Assembly for Enhanced Mechanical and Tribological Performance of Phenolic Resin Composites, J. Mater. Sci., 2019, 54, 8247–8261 CrossRef CAS
.
- B. Akram, Q. C. Lu and X. Wang, Polyoxometalate-Zirconia Coassembled Microdumbbells for Efficient Capture of Iodine, ACS Mater. Lett., 2020, 2, 461–465 CrossRef CAS
.
- S. M. Zhang, N. Liu, H. W. Wang, Q. C. Lu, W. X. Shi and X. Wang, Sub-Nanometer Nanobelts based on Titanium Dioxide/Zirconium Dioxide-Polyoxometalate Heterostructures, Adv. Mater., 2021, 33, 2100576 CrossRef CAS PubMed
.
- B. Sudduth, D. M. Yun, J. M. Sun and Y. Wang, Facet-Dependent Selectivity of CeO2 Nanoparticles in 2-Propanol Conversion, J. Catal., 2021, 404, 96–108 CrossRef CAS
.
- X. T. Jing, Z. Li, B. Lu, Y. Y. Han, Y. N. Chi and C. W. Hu, Assembly of Polyoxometalate with Graphene Foam as a Compressible Monolithic Catalyst for Biodiesel Production, Appl. Catal., A, 2020, 598, 117613 CrossRef CAS
.
- D. L. Collins-Wildman, M. Kim, K. P. Sullivan, A. M. Plonka, A. I. Frenkel, D. G. Musaev and C. L. Hill, Buffer-Induced Acceleration and Inhibition in Polyoxometalate Catalyzed Organophosphorus Ester Hydrolysis, ACS Catal., 2018, 8, 7068–7076 CrossRef CAS
.
- Y. L. Wu, Y. J. Wang, Y. Q. Sun, X. X. Li and S. T. Zheng, Two High-Nuclearity Isopolyoxoniobates Containing {Nb54O151}-based Helical Nanotubes for the Decomposition of Chemical Warfare Agent Simulants, Chem. Commun., 2022, 58, 3322–3325 RSC
.
- M. C. Koning, M. van Grol and T. Breijaert, Degradation of Paraoxon and the Chemical Warfare Agents VX, Tabun, and Soman by the Metal-Organic Frameworks UiO-66-NH2, MOF-808, NU-1000, and PCN-777, Inorg. Chem., 2017, 56, 11804–11809 CrossRef PubMed
.
- S. Kim, W. B. Ying, H. Jung, S. G. Ryu, B. Lee and K. J. Lee, Zirconium Hydroxide-Coated Nanofiber Mats for Nerve Agent Decontamination, Chem. – Asian J., 2017, 12, 698–705 CrossRef CAS PubMed
.
- Y. Y. Tian, A. M. Plonka, A. M. Ebrahim, R. M. Palomino, S. D. Senanayake, A. Balboa, W. O. Gordon, D. Troya, D. G. Musaev, J. R. Morris, M. B. Mitchell, D. L. Collins-Wildman, C. L. Hill and A. I. Frenke, Correlated Multimodal Approach Reveals Key Details of Nerve Agent Decomposition by Single-Site Zr-based
Polyoxometalates, J. Phys. Chem. Lett., 2019, 10, 2295–2299 CrossRef CAS PubMed
.
|
This journal is © the Partner Organisations 2023 |
Click here to see how this site uses Cookies. View our privacy policy here.