DOI:
10.1039/D2QI02076A
(Review Article)
Inorg. Chem. Front., 2023,
10, 13-36
Regulation strategy of white emission from organic–inorganic hybrid metal halide perovskites
Received
27th September 2022
, Accepted 4th November 2022
First published on 8th November 2022
Abstract
White-light emitting materials (WLEMs), especially single-component WLEMs, have always been a research hotspot for their great application potential in white-light emitting diodes (WLEDs). However, the white-light performance of WLEMs, including the quantum yield, color rendering index and so on, has not been greatly improved. The organic–inorganic hybrid perovskites (OIHPs) emerging in recent years have brought vitality to the development of WLEMs and WLEDs because of their excellent white-light emission characteristics. By adjusting the types and proportions of metal cations, halogen anions and organic components, the band gap width and Stokes shift of OIHPs can be adjusted effectively, thus realizing a wide emission covering the whole visible region. In this review, the structure, photophysical properties, and white-light mechanism of OIHPs with white emission are summarized, emphasizing white-light performance adjustment strategies. The further research directions and challenges of white-light emitting OIHPs are proposed to develop high-performance WLEMs meeting various application requirements in the future.
1. Introduction
Lighting technology has attracted more and more attention in the context of advocating energy conservation and environmental protection because the energy consumption of lighting occupies an important part of total power consumption. Light-emitting diodes (LEDs) and organic light-emitting diodes (OLEDs) have received extensive attention due to their advantages of low energy consumption and high efficiency.1–6 Among them, white-light emitting diodes (WLEDs), as candidates for traditional lighting sources,7 have gradually replaced incandescent and fluorescent lamps in illumination fields (Fig. 1a), such as street lighting, indoor lighting, and car lighting, etc. However, there are still some problems with the illumination of WLEDs: impure light color, poor stability and a low color rendering index (CRI). Therefore, it is of great significance to develop WLEDs with high-quality white light.
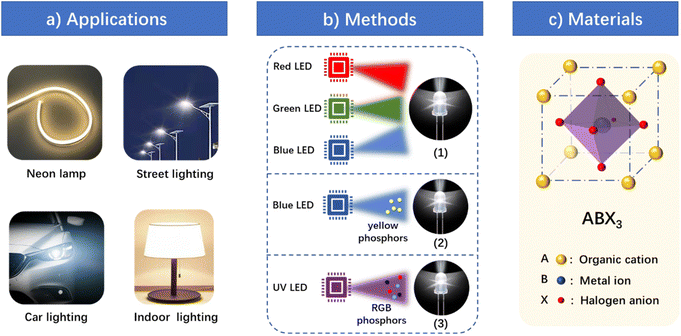 |
| Fig. 1 (a) Various application fields of WLEDs. (b) Main methods realizing the white emission of WLEDs. (c) Common structure of OIHPs. | |
The main manufacturing methods of WLEDs can be divided into the following three kinds (Fig. 1b). The first one is to combine the red, green and blue chips and excite them at the same time to realize white electroluminescence.8 Due to the existence of multiple chips, such WLEDs have a high cost, complex manufacturing process and great difficulty with heat dissipation. The second one is to use blue chips to stimulate yellow phosphor to achieve white light emission.9–13 Although this method can reduce the number of chips and thus reduce the cost, the CRI of this kind of WLED is not high, and the blue light from the chips is harmful to the eyes. The third one is to simultaneously excite the red, green and blue phosphors by a UV chip to obtain white emission covering the whole visible light region with a high CRI. However, this method has a big drawback because there is energy transfer and different decay rates between these phosphors, which leads to changes in the emission spectrum during the lighting process, thus resulting in low luminescence efficiency and an unstable light color.14 Single-component white-light emitting materials (WLEMs) can not only overcome this shortcoming, but also have many advantages over other materials in WLEDs: low cost, simple fabrication techniques and high stability. Consequently, the development of single-component WLEMs is very popular in the field of WLED lighting.
With the continuous efforts of researchers, great progress has been made in the development of single-component WLEMs.15 Various single-component WLEMs emerge endlessly.16,17 To date, the single-component WLEMs can be mainly divided into inorganic (such as ZnCdS,18 CdSe,19etc.), organic (including small molecules and polymers),20–23 and organic–inorganic hybrid materials (such as metal–organic frameworks, semiconductors, and metal complexes etc.).24–27 However, the inorganic materials and organic small molecules have some problems, such as complex synthesis and difficult processing, while polymers have poor repeatability and relatively low luminescence efficiency. Organic–inorganic hybrid materials, which combine the advantages of inorganic and organic WLEMs, are expected to overcome the above problems, showing great potential in the field of WLEDs. Therefore, it is necessary to develop single-component WLEMs based on organic–inorganic hybrid materials with excellent performance.
Organic–inorganic hybrid metal halide perovskites (OIHPs) with unique photoelectric properties, low costs, and low-temperature solution processing28–32 have recently stimulated a broad range of research interest for great application prospects in solar cells, LEDs, X-ray scintillators, lasers, photodetectors and so on.33–37 In particular, OIHPs have the advantages of a tunable energy band, wide emission, high absorption coefficient and luminescence quantum yield (Φ), which makes them important candidates for single-component WLEMs.38–40 After nearly ten years of development, the white-light performance of OIHPs has been greatly enhanced with Φ improved from 1.5% to 100%,41,42 the CRI reaching 97,43 and Commission Internationale de l'Eclairage (CIE) coordinates close to (0.33, 0.34).44 There are several reviews on the synthesis methods, optical properties, luminescence mechanisms and applications of OIHPs,45–47 but few on the white-light properties of OIHPs.48 In order to acquire a high-quality white emission from OIHPs, this review focuses on the regulation of the white-light performance of OIHPs.
2. Structure and luminescence properties of OIHPs
In 1985, Møller et al. discovered three-dimensional (3D) metal halide perovskite for the first time.49 3D metal halide perovskites are a class of compounds with the general formula ABX3. These compounds were initially found in the form of CaTiO3 in calcium titanium ores, so they are collectively called perovskite. The 3D structure is usually a regular octahedral skeleton formed by organic cations A (CH3NH3+, HC(NH2)2+, etc.), divalent metal cations B (Pb2+, Sn2+, Ge2+, Cu2+, Eu2+, Co2+, etc.) and halogen anions X (Cl−, Br−, I−) (Fig. 1c). According to the connection mode of BX6 octahedra, OIHPs can be divided into four structures at the molecular level (Fig. 2a).50–53 The 3D structure of OIHPs is formed by connecting BX6 octahedra along three axes in a corner-shared manner. The stability of the ABX3 perovskite structure is determined by the Goldschmidt tolerance factor (t) and the octahedral factor (μ): | t = RA + RX/√2(RB + RX) | (1) |
where RA, RB and RX represent the radii of the corresponding ions A+, B2+, X−, respectively. When the tolerance factor is between 0.8 and 1, and the octahedral factor is between 0.44 and 0.9, 3D perovskite can be formed.53
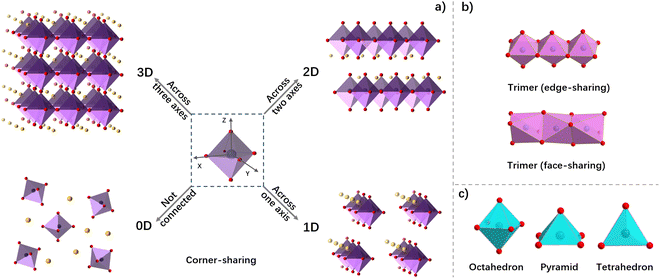 |
| Fig. 2 (a) Crystal structure diagram of 3D, 2D, 1D and 0D hybrid metal halide perovskites. (b) The variant structure of metal halides in OIHPs. (c) The metal halide structure: octahedra, pyramids, and tetrahedra. | |
If large-volume organic cations are introduced into the framework of 3D perovskites, low-dimensional perovskites can be obtained. Two-dimensional (2D) perovskites can be regarded as a slice or layer of 3D perovskite stripped along a specific crystallographic direction.54 The layered structure of 2D perovskites is formed by the self-assembly of organic ammonium salt and metal halide through hydrogen bonding and electrostatic interaction.46,52,55 The organic cations can be used as an encapsulation layer to prevent water and oxygen from entering into the perovskite skeleton, thus increasing the stability of the perovskite structure.56 The alternating appearance of inorganic and organic layers in 2D perovskites forms a large number of quantum traps, which is beneficial to stabilize excitons and increase the exciton binding energy, thus showing good luminescence properties.52 One-dimensional (1D) hybrid perovskites are obtained by slicing perpendicular to the layered 2D structure, in which inorganic octahedra are connected along an octahedral axis to form a chain or ribbon structure.57 In the 1D perovskites, the octahedron coordinated by metal ions and halogen ions can form the framework through angle-sharing, edge-sharing or face-sharing (Fig. 2b). Zero-dimensional (0D) structures are formed by metal halides which are not connected with each other. In 0D hybrid perovskites, each metal halide octahedral unit is completely isolated and surrounded by organic spacers, regularly and repeatedly arranged to form block materials.58 In addition, the structure of inorganic metal halides can be octahedra, pyramids, or tetrahedra (Fig. 2c). These variant structures of metal halides have been found in various OIHPs, especially 0D perovskites for their large tolerance to organic cations.59
It is well known that OIHPs have excellent luminescence properties. It is necessary to understand their exciton dynamics and photophysical mechanisms for designing novel materials with better luminescence performance. The diagram of a typical exciton energy level structure is shown in Fig. 3a. When an exciton is excited to the excited state, free excitons (FEs) are generated, and part of the FEs can directly return to the ground state in the form of photons to form FE emission. Another part of the FEs generates self-trapping excitons (STEs) through lattice self-trapping, and then returns to the ground state in the form of photons, resulting in a long-wavelength emission of STEs.45 FE emission is characterized by a smaller Stokes shift and narrower full width at half maximum (FWHM), relative to that of STEs.40,60–63 For 3D perovskites, the luminescence properties are mainly dominated by FE emissions with nanosecond lifetimes. In the low-dimensional OIHP structure, the soft lattice in the excited state will undergo significant photoinduced deformation relative to the ground state, thus resulting in STEs due to the strong electron–phonon coupling. The transient lattice deformation and exciton self-trapping reduce the energy of the system, resulting in a large Stokes shift of STE emission.64,65 In addition, all kinds of self-trapping states with different self-trapping depths resulting from strong exciton–lattice interactions contribute to the broadband emission that can span the whole visible spectrum.40,55,66,67
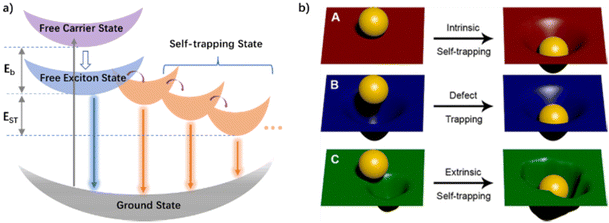 |
| Fig. 3 (a) Schematic diagram of a self-trapping energy level structure: Eb (exciton binding energy); EST (self-trapping energy). (b) Intrinsic self-trapping (A), trapping at permanent defects (B), and self-trapping influenced by permanent defects (C) represented by a ball interacting with a rubber sheet. Reproduced with permission.40 Copyright 2018, American Chemical Society. | |
According to whether there are doping ions or intrinsic defects in the host lattice, the self-trapping can be divided into two types: intrinsic and extrinsic. The difference in several self-trapping is vividly described in Fig. 3b, in which the hard sphere is regarded as an exciton formed by electrons and holes, while the soft rubber plate is compared with a deformable lattice of perovskites. In the case of the intrinsic self-trapping shown in Fig. 3b(A), the sinking of the ball can cause the rubber sheet deformation which is elastic and will return to its original state when the ball is removed. The process of intrinsic defects does not require permanent material defects, as shown in Fig. 3b(B). The permanent defect is the lattice deformation existing before the ball contacts the rubber plate. In the case of the extrinsic self-trapping shown in Fig. 3b(C), the ball touches the plate and sinks to different depths near the permanent defect. In addition, the existence of self-trapping is related to the lattice size, and low-dimensional OIHPs are conducive to the formation of self-trapped states.68 3D perovskites have a weak STE emission only at low temperatures. 2D and 1D perovskites have both FE and STE emission at room temperature. There is a driving force inducing exciton transfer from FE to STE energy levels in 0D perovskites, thus exhibiting mainly STE emission. Because the metal halide anions are separated by organic cations, 0D perovskites can form a quantum dot structure with a strong quantum confinement effect and large exciton binding energy. Therefore, 0D perovskites eliminate the interaction between adjacent metals, and produce high-efficiency STE luminescence,69 which has important potential for single-component WLEMs in the field of WLEDs.
3. The mechanism of OIHP white light emission
Since Karunadasa's group first reported two single-component white light OIHPs based on lead halide in 2014,41,70 researchers have developed various WELMs based on OIHPs. Due to the rich raw material resources of OIHPs, white emission can be realized by adjusting the types of organic cation, metal ion or halogen anion. Fig. 4 shows most of the organic cations employed to synthesize the white-light emitting OIHPs. Inorganic metal ions commonly used include Pb2+, Cd2+, Mn2+etc., while halogen anions commonly used are Cl−, Br−, etc. The combination of different organic cations and metal halides can yield white emission with different properties. Table 1 summarizes the structure and composition of white-light emitting OIHPs, as well as their corresponding white-light performance. Because OIHPs can realize white emission through one or multiple emission centers, this section will summarize the mechanism of white-light emission according to the classification of inorganic luminescence centers, organic luminescence centers, and the combination of organic and inorganic light-emitting centers.
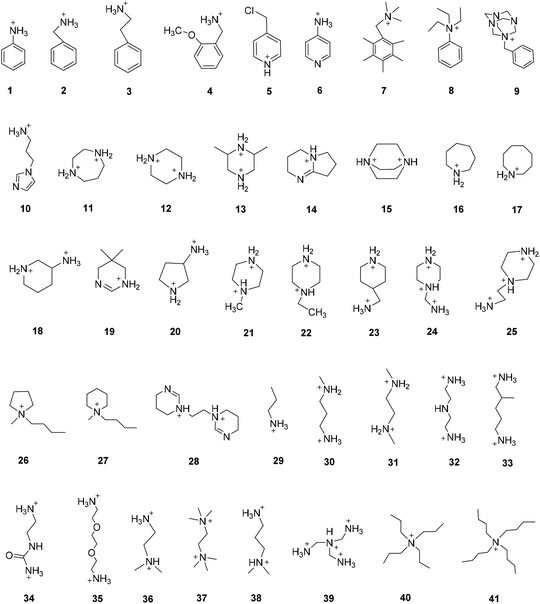 |
| Fig. 4 Structure of organic cations used in OIHPs with white emission. | |
Table 1 Structure, composition and corresponding performance of OIHPs with white emission
Entry |
Dimension |
Organic cation |
Metal halides |
CIE (x, y) |
CRI |
Φ (%) |
Ref. |
1 |
3D |
15 |
PbCl2 |
(0.33, 0.34) |
96 |
2.5 |
44
|
2 |
3D |
11 |
PbBr2 |
(0.41, 0.39) |
90 |
0.46 |
71
|
3 |
2D |
3 |
PbCl2 |
(0.33, 0.36) |
78 |
43 |
72
|
4 |
2D |
3 |
PbBr2, MnBr2 |
(0.37, 0.29) |
91 |
39 |
73
|
5 |
2D |
3 |
PbCl2, PbBr2 |
(0.32, 0.35) |
90 |
<16.9 |
74
|
6 |
2D |
3 |
PbBr2, PbCl2 |
(0.34, 0.37) |
75 |
— |
75
|
7 |
2D |
36 |
PbCl2 |
(0.21, 0.24) |
— |
<1 |
76
|
8 |
2D |
38 |
PbCl2 |
(0.22, 0.25) |
— |
4.9 |
76
|
9 |
2D |
4 |
PbCl2 |
(0.31, 0.38) |
86 |
5 |
77
|
10 |
2D |
35 |
PbBr2, MnBr2 |
(0.42, 0.41) |
84.9 |
12.54 |
78
|
11 |
2D |
35 |
PbCl2 |
(0.33, 0.39) |
82 |
2 |
70
|
12 |
2D |
20 |
PbCl2 |
— |
85 |
— |
79
|
13 |
2D |
2 |
PbCl2, PbBr2 |
(0.31, 0.36) |
<91 |
10.76 |
80
|
14 |
2D |
33 |
PbBr2 |
(0.24, 0.23) |
91 |
3.37 |
67
|
15 |
2D |
10 |
PbCl2 |
(0.36, 0.37) |
93 |
<1 |
81
|
16 |
2D |
33 |
PbCl2, PbBr2 |
(0.29, 0.33) |
86 |
1.67 |
82
|
17 |
2D |
30 |
PbCl2, PbBr2 |
(0.31, 0.36) |
85 |
1.5 |
41
|
18 |
2D |
36 |
PbBr2 |
(0.28, 0.36) |
73 |
— |
83
|
19 |
2D |
29 |
PbCl2, PbBr2 |
(0.30, 0.35) |
83 |
— |
84
|
20 |
2D |
22 |
PbBr2 |
(0.44, 0.44) |
84 |
0.97 |
71
|
21 |
2D |
23 |
PbBr2 |
(0.31, 0.39) |
76 |
0.54 |
71
|
22 |
2D |
21 |
PbBr2 |
(0.38, 0.42) |
86 |
0.33 |
71
|
23 |
1D |
13 |
PbBr2 |
(0.44, 0.46) |
77 |
12.24 |
71
|
24 |
1D |
17 |
PbBr2 |
(0.52, 0.41) |
89 |
0.63 |
71
|
25 |
1D |
16 |
PbBr2 |
(0.54, 0.40) |
88 |
0.35 |
71
|
26 |
1D |
31 |
PbBr2 |
(0.21, 0.28) |
63 |
20 |
60
|
27 |
1D |
12 |
PbBr2 |
— |
84 |
60 |
85
|
28 |
1D |
25 |
PbBr2 |
(0.41, 0.47) |
— |
9 |
86
|
29 |
1D |
5 |
CdCl2 |
(0.33, 0.30) |
83.7 |
12.3 |
87
|
30 |
1D |
28 |
PbCl2 |
(0.27, 0.32) |
83 |
6.99 |
88
|
31 |
1D |
19 |
PbCl2 |
(0.29, 0.37) |
76 |
5.29 |
88
|
32 |
1D |
14 |
PbCl2 |
(0.25, 0.29) |
82 |
1.49 |
88
|
33 |
1D |
18 |
PbCl2 |
(0.39, 0.37) |
93.9 |
1 |
24
|
34 |
0D |
39 |
PbCl2 |
(0.30, 0.33) |
96 |
— |
89
|
35 |
0D |
37 |
CdBr2 |
(0.26, 0.31) |
83 |
1 |
66
|
36 |
0D |
32 |
PbCl2, MnCl2 |
(0.33, 0.34) |
90 |
40.3 |
90
|
37 |
0D |
11 |
SnCl4 |
(0.39, 0.43) |
97 |
— |
43
|
38 |
0D |
6 |
HgBr2, ZnBr2 |
(0.34, 0.38) |
<96 |
<19.18 |
31
|
39 |
0D |
24 |
ZnCl2 |
(0.24, 0.25) |
85 |
11.52 |
91
|
40 |
0D |
40 |
CuI |
(0.33, 0.35) |
92.2 |
91.9 |
63
|
41 |
0D |
41 |
CuI |
(0.29, 0.28) |
— |
72.5 |
92
|
42 |
0D |
26 |
SbCl3, PbCl2 |
— |
90 |
>70 |
93
|
43 |
0D |
3 |
SbBr3, InBr3 |
(0.40, 0.36) |
84 |
23.36 |
94
|
44 |
0D |
27 |
PbBr2, SnBr2 |
(0.32, 0.23) |
18.5 |
39 |
58
|
45 |
0D |
34 |
PbBr2 |
(0.39, 0.42) |
— |
9.6 |
95
|
46 |
0D |
9 |
PbBr2, MnBr2, SnBr2 |
(0.32, 0.33) |
95 |
73 |
96
|
47 |
0D |
7 |
ZnBr2 |
(0.26, 0.30) |
— |
3.07 |
97
|
48 |
0D |
1 |
SbCl3, InCl3 |
(0.33, 0.37) |
90 |
100 |
42
|
3.1 Inorganic luminescence center
The broadband white emission of many OIHP materials is attributed to the radiation recombination of STEs caused by the inorganic lattice distortion induced by electron–phonon coupling. Smith et al. found that the broadband PL emission intensity has a great correlation with the distortion degree of the inorganic layer, by comparing the structural parameters of several 2D perovskites.61 Through an analysis of a series of (001) Pb–Br perovskite structures, it is found that the average Pb–(μ-Br)–Pb angle (θtilt) is 155° with a standard deviation of 9° (Fig. 5a). As shown in Fig. 5b and c, θtilt can be separated into two components: in-plane (θin) and out-of-plane (θout) projections, where the plane is defined by three adjacent Pb atoms within an inorganic layer. In-plane and out-of-plane distortions (Din and Dout) are defined as the deviation of these angles from linearity, so that Din = 180° − θin and Dout = 180° − θout. Organic cations can affect Din and Dout through space size and hydrogen-bonding interactions with metal halides. Then authors demonstrated the relationship between Dout and ln(IBE·INE−1) across the series of Pb–Br perovskites, where IBE represents the intensity of broadband emission, and INE represents the intensity of narrowband emission (Fig. 5d). They found that there was a strong linear correlation between Dout and ln(IBE·INE−1). It can be proved that exciton self-trapping is mainly determined by the local geometric structure in the inorganic layer.
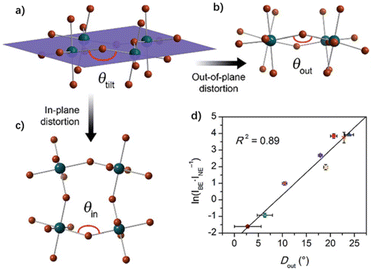 |
| Fig. 5 (a) Schematic of interoctahedral tilting (θtilt) in a single Pb–Br layer of a (001) perovskite, and its decomposition into (b) out-of-plane (θout) and (c) in-plane (θin) components. (d) Values of ln(IBE·INE−1) for the series of (001) Pb–Br perovskites plotted as a function of the largest measured Dout value. Reproduced with permission.61 Copyright 2017, The Royal Society of Chemistry. | |
The organic cations are not the luminescence centers, but act as a framework to form the perovskite structure or as an energy donor to transfer energy to the inorganic luminescence centers.41,68,70,98,99 For example, Luo et al. synthesized a 2D hybrid lead chloride perovskite based on N-(3-aminopropyl) imidazole (10 in Fig. 4). It achieved a warm-white emission with a CRI as high as 93, and a correlated color temperature (CCT) of 4203 K, due to the self-trapped excited states in the highly distorted perovskite framework.81 Ma et al. synthesized a 0D metal halide (bmpy)9[SbCl5]2[Pb3Cl11] (bmpy = 1-butyl-1-methylpyrrolidine, 26 in Fig. 4) by combining two inorganic luminescence centers. As shown in Fig. 6a and b, the OIHP crystal structure shows that the distance between two adjacent octahedra is long enough, which eliminates the influence of the intermolecular force on the luminescence performance, and acquires a higher quantum yield. It can be seen from Fig. 6d that the material has double emission peaks at 516 nm (Em I) and 673 nm (Em II) when excited at 350 nm. According to the transient luminescence decay curves, the lifetimes of Em I and II were calculated to be 0.51 μs and 4.3 μs, corresponding to the emission of Pb3Cl115− and SbCl52− ions, respectively (Fig. 6e). Therefore, the warm-white emission with Φ > 70% is realized by the combination of a blue-green emission of Pb3Cl115− and orange-red emission of SbCl52−.93
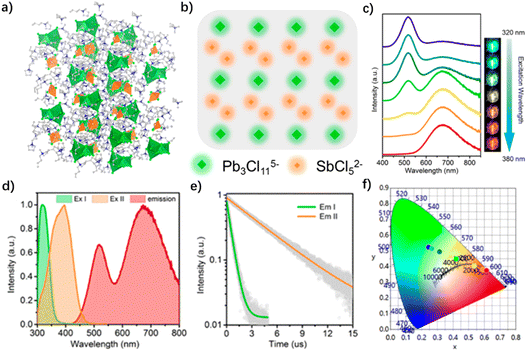 |
| Fig. 6 (a) Single crystal structure diagram of (bmpy)9[SbCl5]2[Pb3Cl11] (where the green polyhedra represent Pb3Cl115− and the orange pyramids represent SbCl52−). (b) Scheme of the 0D “host–guest” structure with multiple metal halide species. (c) Excitation-dependent photoluminescence spectra. (d) Excitation and emission spectra (excited at 350 nm). (e) Emission decay curves monitored at 510 and 630 nm. (f) CIE coordinates for the excitation-dependent photoluminescence. Reproduced with permission.93 Copyright 2020, American Chemical Society. | |
3.2 Organic luminescence centers
The low Φ has always been a major problem of 2D perovskites, because the strong interaction between inorganic metal ions can lead to a thermal quenching and self-absorption of excitons.73,100 To solve the problem, an effective method is to excite only the organic luminescence centers without generating inorganic excitons. As a result, by doping with organic luminophores with lower excitation energy than inorganic FEs, the energy loss caused by the excitation of the inorganic components can be effectively avoided. Although the inorganic components do not act as luminescence centers, they can fix organic luminophores to reduce the thermal quenching effect of organic excitons, and provide an external heavy atom effect to promote the ISC transition from the singlet state to the triplet state of organic excitons.75 For example, Lin et al. first used the new strategy to realize highly efficient white emission by doping the organic luminophores with different band gaps into 2D OIHPs with a high-energy free exciton band (FEB). Since the excitation energy of the organic luminophores is lower than that of the FEB, it is possible to select a specific wavelength to excite only the organic luminophores, thus producing the emission of organic excitons with different band gaps (Fig. 7a). Because the excitation of the inorganic metal halide is avoided, highly efficient white emission can be realized. According to this strategy, the authors firstly introduced an organic fluorophore termed NLAC into a 2D hybrid perovskite (named PEPC) with a wide band gap (>3.54 eV), and obtained a green emission at 495 nm with a Φ of 81% (Fig. 7c). Based on this, another blue fluorophore (named NAAC) and red organic phosphor (named NIAC) were co-doped with NLAC into PEPC to obtain a white light emission with a Φ of 43%, and a CIE of (0.33, 0.36) (Fig. 7d). Interestingly, the resulting perovskite shows a dual-color organic afterglow and excitation-wavelength-dependent emission, which can be applied in the fields of afterglow WLEDs and information storage.72
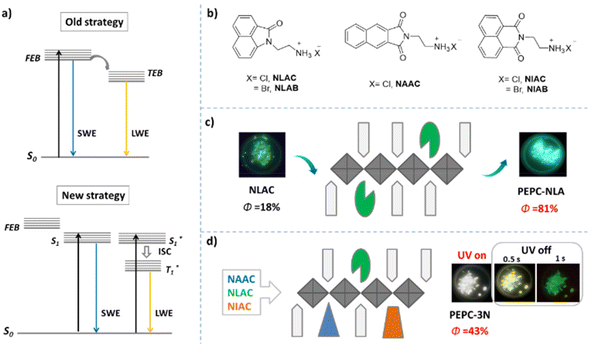 |
| Fig. 7 (a) Schematic diagram of white emission from 2D hybrid perovskites: free exciton band (FEB); self-trapped exciton band (TEB); short-wavelength emission (SWE); long-wavelength emission (LWE). (b) Structure of organic luminophores. Schematic diagram of the structure of OIHP with green (c) and white (d) emission: lead chloride (square); PEAC (pentagon); NAAC (triangle); NLAC (sector), NIAC (trapezoid). Reproduced with permission.72 Copyright 2021, American Chemical Society. | |
3.3 Organic–inorganic hybrid luminescence centers
In addition to inorganic or organic emission centers, the combination of inorganic and organic luminescence centers can also produce broadband white emission covering the whole visible region.44,70,89–91,99,101–106 The energy transfer between the organic and inorganic components can enhance the utilization rate of excitons, avoiding an energy loss of excited photons. Consequently, the synergistic effect of the organic and inorganic components can improve the white-light efficiency. Saparov et al. reported two low-dimensional OIHPs based on 4-aminopyridinium (4AMP, 6 in Fig. 4) and metal halides (HgBr2 and ZnBr2), showing a white emission comprising organic fluorescence and inorganic STE emission.31 The CIE of (4AMP)2HgBr4·H2O and (4AMP)2ZnBr4 are (0.34, 0.38) and (0.25, 0.26), respectively. Their quantum yield is 14.87% (for Hg-based halide) and 19.18% (for Zn-based halide). In recent years, organic room temperature phosphorescence (RTP) materials have been developed rapidly.107–111 The combination of RTP and inorganic halide luminescence can also produce white-light emission of OIHPs.
In 2018Lin's group proposed a new strategy to develop highly efficient RTP perovskites. They doped organic 1,8-naphthalimide (NI) as guest cations into a 2D perovskite based on phenethylamine, realizing a yellow RTP of NI with Φ up to 56.1% (Fig. 8a). The triplet excitons of NI are mainly derived from Wannier excitons of inorganic perovskite through energy transfer (for films), and from singlet excitons of NI through intersystem crossing (for powder) (Fig. 8b). Interestingly, a white emission comprising the blue one from the perovskite and yellow RTP from NI, was obtained in PbBr2-based OIHPs in powder (Fig. 8c). When applied in an WLED driven by a UV chip, the OIHP powder displayed a white electroluminescence with a CIE of (0.32, 0.36), which is close to that of pure white light of (0.33, 0.33). By changing the loading amount of the powder, the CCT and CRI values of the WLED could be tuned from 5030 K to 12
483 K and from 73 to 87, respectively.75 This work indicates that it is an effective method for realizing white light to combine organic phosphorescence and inorganic exciton emission. In particular, they recently acquired a white-light emission with a near-unity quantum yield by using organic blue RTP and inorganic STE triplet emission. This work will be described in detail in a later section.42
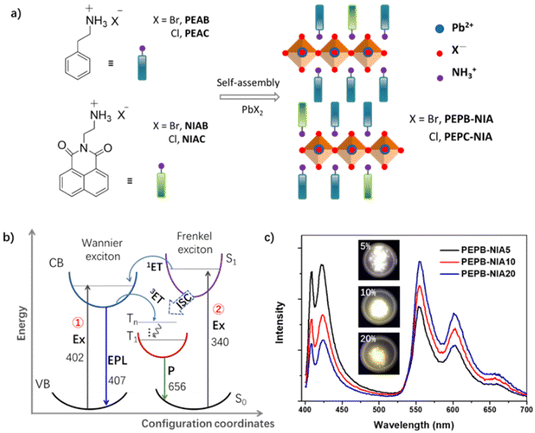 |
| Fig. 8 (a) Synthesis and structural illustration of the doped perovskites. (b) Schematics summarizing the main possible energy transfer (ET) in films and ISC in powders: Ex = excitation, EPL = excitonic photoluminescence, P = phosphorescence, and F = fluorescence. (c) Photos under UV light and the photoluminescence spectra of PEPB-NIA. Reproduced with permission.75 Copyright 2018, The Royal Society of Chemistry. | |
4. Regulation strategy of white emission from OIHPs
It was found that the composition and structure of metal halide perovskites, as well as external stimuli, have a great influence on their white light properties. Therefore, the white light performance can be adjusted by changing the spatial dimensions, organic molecular structure (site A), metal cations (site B), halogen anions (site X), excitation wavelengths and external factors etc. This section will discuss in detail how these factors affect the white light emission of OIHPs.
4.1. Organic molecular structure
The photophysical mechanism of OIHPs with white emission is closely related to the organic molecular structure.80,112–114 The length of the molecular chains, volume of aromatic rings, and isomerization of organic molecules impose a great effect on the structure and luminescence properties of OIHPs. Consequently, the organic cation structure can be used to control the degree of structural distortion of OIHPs, and realize the white-light emission. As mentioned in 3.1 above, Smith et al. realized broadband white light emission by adjusting the kinds of organic cation, and proposed that the relative intensity of broadband emission was related to the inorganic lattice distortion of OIHPs.61 Consequently, it is feasible to adjust the lattice distortion by changing the chain length, molecular isomerism and heterocyclic structure of organic cations to achieve the white-light emission of OIHPs.
4.1.1 Length of the chain.
For OIHPs based on alkyl amine salts, the length of the alkyl chains will lead to different degrees of lattice distortion and a change of luminescence performance, which arouses researchers’ interest to regulate the white-light performance by adjusting the length of the organic cation chains. Kanatzidis et al. synthesized three 2D perovskites from different alkyl diamines: 2-(dimethylamino)ethylamine (DMEN), 3-(dimethylamino)-1-propylamine (DMAPA), and 4-dimethylaminobutylamine (DMABA) (Fig. 9a).83 Among them, α-(DMEN)PbBr4 features (110) oriented 2D layered crystals with a corrugated “3 × 3” layer, while (DMAPA)PbBr4 and (DMABA)PbBr4 are conventional (100)-oriented 2D layered crystals. It was found that only α-(DMEN)PbBr4 exhibits white-light emission among the three 2D perovskites. To ascertain the reasons, authors calculated the distortion degree (Δd) of the “PbBr6” octahedron by the following equation: | 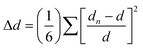 | (3) |
where d is the mean Pb–Br bond distance and dn are the six individual Pb–Br bond distances. The calculated results indicate that α-(DMEN)PbBr4 has the largest distortion degree of the three 2D perovskites, which results in the broadband white emission (Fig. 9b). It can be seen from Fig. 9c that the FWHM of all the compounds increases linearly with the degree of octahedral distortion. The authors proposed that the corrugated (110)-oriented 2D structure of α-(DMEN)PbBr4 is stabilized by a special “chelating effect” of hydrogen bonding. The severely distorted structure of α-(DMEN)PbBr4 leads to a white-light emission with a CRI of 73 and CCT of 7863 K (Fig. 9d). In 2020, Lei et al. also found that the Φ of white-light OIHPs is related to the length of the alkyl chains. They improved the Φ of 2D perovskite with white emission from <1% to 4.9% through changing the alkyl amine from N,N-dimethylethylenediamine (DMEDA) to N,N-dimethyl-1,3-diaminopropane (DMPDA).76 This correlation between the luminescence properties and the length of the alkyl amine chains can be used as a good strategy to regulate the white-light emission characteristics of OIHPs.
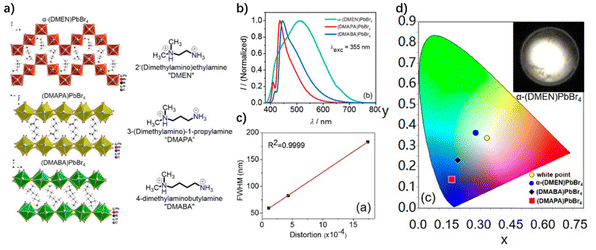 |
| Fig. 9 (a) Crystal structures of α-(DMEN)PbBr4, (DMAPA)PbBr4, and (DMABA)PbBr4 and the organic cations incorporated in each structure. (b) Steady-state emission spectra. (c) Correlation between the FWHM and octahedral distortion of the three compounds. (d) Chromaticity coordinates of the three compounds in a 1931 color space chromaticity diagram. Inserted: white light emission picture of polycrystalline α-(DMEN)PbBr4 excited at 365 nm. Reproduced with permission.83 Copyright 2017, American Chemical Society. | |
4.1.2 Isomerization of organic molecules.
In addition to alkyl amine cations, a large number of arylamine cations were used to construct OIHPs. The isomerization of organic aromatic groups can affect the luminescence performance of OIHPs. To explore the relationship between the organic isomer structure and the photoluminescence properties of perovskites, Lam et al. synthesized a series of lead bromide perovskites (4-MOP, 3-MOP, and 2-MOP) from the para-isomer (4-MBA), meta-isomer (3-MBA) and ortho-isomer (2-MBA) of methoxybenzyl ammonium (MBA), respectively (Fig. 10a).77
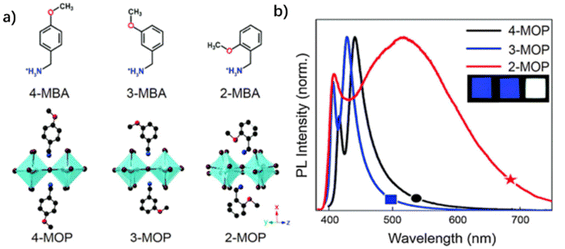 |
| Fig. 10 (a) Organic cations of 4-MOP, 3-MOP and 2-MOP and the corresponding crystal structures. (b) Emission spectrum and photos of three OIHPs thin films under an ultraviolet lamp. Reproduced with permission.77 Copyright 2018, the Royal Society of Chemistry. | |
The methoxy groups located in the different positions of the ammonium ions could induce the distortion of the inorganic layer structure in different degrees. By comparing the crystallographic data of three kinds of 2D perovskite, it was found that 2-MOP shows a larger octahedral tilt than 4-MOP and 3-MOP. This is because the distance between the C–O bond and the bromine in 2-MOP is closer than that in 4-MOP and 3-MOP. The strong repulsion between Br and O in 2-MOP forces the octahedron to form a relatively disordered structure. By analyzing the optical properties of three 2D perovskites, it was found that 4-MOP and 3-MOP emit blue light, and 2-MOP emits white light with a wide emission band (Fig. 10b), and that their FWHM is consistent with the degree of distortion. In addition, the authors have proved that their narrow-band and broad-band emission comes from the radiation recombination of FEs and STEs, respectively. Due to the distortion lattice, it is easy for 2-MOP to form STEs, thus leading to a broadband white emission with an FWHM of 184 nm. By the isomerization of organic molecules, the author realized the white-light emission of OIHPs, and finally obtained a WLED with a CCT of 6600 K and a CRI of 86.
4.1.3 Heterocyclic structure.
Besides the chain length and isomerization of organic molecules, changing the organic heterocyclic structure is also an effective strategy to regulate the white light emission of OIHPs.71 By selecting a series of pyridine derivatives as organic cations and PbCl2 as an inorganic precursor, Lei et al. synthesized a series of 1D OIHPs: (DTHPE)0.5PbCl3, DMTHPPbCl3, and DBNPbCl3 (corresponding to entries 30, 31, and 32, respectively, in Table 1).88 The octahedral structure of the three perovskites is connected in a face-shared manner to form 1D [PbCl3]− chains. These perovskites adopt a similar stacking mode which can be considered as a quasi-honeycomb (6,3) topological network by linking each organic ring as a 3-connected node (Fig. 11a). The 1D [PbCl3]− chains with parallel arrangements provide a tunable filling space and structural tolerance to accommodate significant variations in the geometries of organic cations. The distortion degree of the inorganic octahedral chain of these perovskites is calculated to be 2.757 × 10−3, 1.925 × 10−3, and 2.126 × 10−3, respectively. The different distortion degrees of the inorganic octahedra makes the three perovskites have different photoluminescence characteristics. The maximum emission peaks of (DTHPE)0.5PbCl3, DMTHPPbCl3, and DBNPbCl3 are at 458 nm, 496 nm and 460 nm, respectively (Fig. 11b–d). The Φ of the broadband emission can be enhanced from 1.49% to 6.99%, and the CRI can be improved from 76 to 83 (Fig. 11e). This work further proves that organic cations are not only used as structural templates and energy donors, but also can regulate the broadband white emission of perovskites.
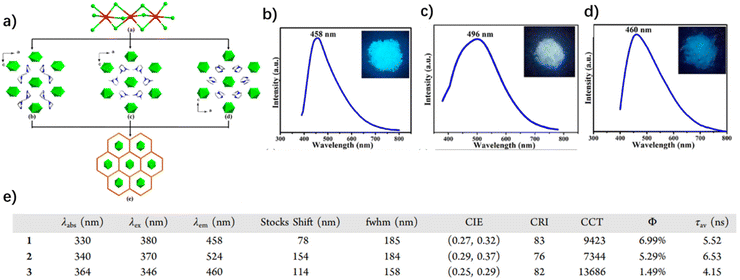 |
| Fig. 11 (a) Structure diagram of the perovskites. Emission spectra of (b) (DTHPE)0.5PbCl3, (c) DMTHPPbCl3, and (d) DBNPbCl3. Inset: photos of the perovskites under a UV lamp. (e) Corresponding parameters of the perovskites. Reproduced with permission.88 Copyright 2020, American Chemical Society. | |
In a word, the change of metal halide octahedral environment is helpful to control the distortion of the inorganic lattice, and thus enhance the emission bandwidth and the luminous efficiency, which are closely related to the type of A-site organic cation.
4.2 Metal cations
B-site metal ions play an important role in the white emission of OIHPs because the type of metal ion directly affects the band gap of the OIHPs. Inorganic components based on metal ions can not only produce FE and STE emission, but also have a certain energy transfer with organic cations, which affects the luminescence performance of the OIHPs. Particularly, the presence of foreign guest metal ions will yield new luminescence centers. Consequently, the emission of OIHPs can be adjusted by doping with different metal ions (such as Mn2+ Sn2+. Sb3+, etc.).58,73,115 This section will discuss how to regulate white light by changing the kinds and contents of metal ions.
Xia et al. doped different amounts of Mn2+ ions into (C6H18N2O2)PbBr4 with a double emission of FEs and STEs, to adjust the white-light performance of the OIHPs by changing the energy transfer from STEs and FEs to Mn2+ ions. When the doping amount is 5%, the emission spectrum of the hybrid material covers all the visible-light region with a FWHM of about 230 nm. A electroluminescent white-light with a CRI of 84.9 and a CCT of 3577 K can be obtained in the WLED based on (C6H18N2O2)Pb0.95Mn0.05Br4.78 Similarly, Liu et al. selected Sn2+ as a doping metal ion and synthesized a series of 0D OIHPs (bmpip)2PbxSn1−xBr4 (0 < x < 1) (bmpip = 1-butyl-1-methyl-piperidinium, 27 in Fig. 4) with dual emissions at 470 nm and 670 nm. The high- and low-energy emissions are derived from the luminescence center of the Pb and Sn, respectively. As a result, the light color of the OIHPs can be adjusted by changing the Pb/Sn molar ratio. When x = 0.16, (bmpip)2Pb0.16Sn0.84Br4 can achieve a white light emission with Φ up to 39a (entry 44 in Table 1).58
Lin et al. recently synthesized two 0D lead-free perovskites (PA6InCl9 and PA4InCl7) from aniline hydrochloride and indium chloride by a hydrothermal method. The two perovskites show that the blue phosphorescence originated from the organic component with an RTP quantum yield up to 42.8% and a lifetime of about 1.2 s.42 Both PA6InCl9 and PA4InCl7 exhibit RTP sensitive to external stimuli (Fig. 12a), which will be discussed in a later section. More interestingly, the authors achieved a high-efficiency white and orange phosphorescence with a near unity quantum yield by using Sb3+ as the doping metal ion. The white pure phosphorescence with a CRI close to 90 consists of a blue RTP of PA6InCl9 and an orange phosphorescence of Sb3+. A series of PA6InCl9-Sb powder crystals were obtained by adding different amounts of Sb3+ (0.5%–2.5%) into PA6InCl9 (Fig. 12b). It can be found that with the increase of Sb3+ content, the color of the doped perovskites in sunlight has no obvious change, while the luminescent color changes from blue to cool white and to warm white under a 254 nm UV lamp. A new orange emission peak appears at 580 nm in the photoluminescence spectra of PA6InCl9-Sb, which is assigned to the triplet state of Sb3+ self-trapping excitons (Fig. 12c). When the doping amount of Sb3+ is more than 2%, the intensity of the orange emission is higher than that of the blue emission, accompanied by the luminous efficiency reaching the maximum value upon excitation at 282 nm (Fig. 12d). The near-unity quantum efficiency should be attributed to the fact that the maximum excitation peaks of blue and orange RTP overlap at 282 nm.
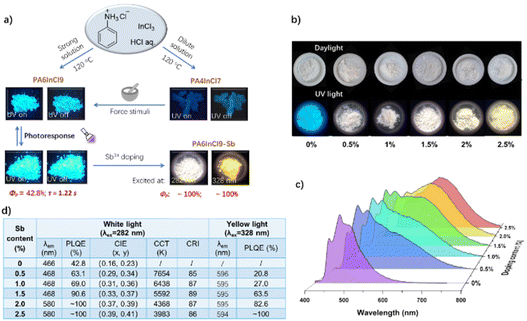 |
| Fig. 12 (a) Synthesis and luminescence properties of PA6InCl9 and PA4InCl7. (b) Photos of PA6InCl9-Sb solids with different amounts of Sb3+ under daylight and 254 nm UV light. (c) Emission spectra of PA6InCl9-Sb solids with different amounts of Sb3+ excited at 282 nm. (d) Summarized photophysical data of PA6InCl9-Sb. Reproduced with permission.42 Copyright 2022, Wiley-VCH. | |
4.3. Halogen anions
The X-site halogen atoms in metal halides mainly include Cl−, Br− and I−. The type and content of halogen atoms have important effects on the band gap, lattice distortion and exciton behavior of OIHPs. Therefore, it is a feasible method to adjust the white light emission of OIHPs by changing the halogen atoms.84,116 For example, Kanatzidis et al. employed a small cyclic diammonium, 3-aminopyrrolidinium (3Apr, 20 in Fig. 4), as the organic cation to synthesize a series of (110)-oriented 2D perovskites, (3APr)PbX4 (X = I, Br, Cl). The experimental bandgaps of (3APr)PbX4 follow the trend I < Br < Cl (2.56 eV, 3.29 eV, and 3.85 eV, respectively). It is proved that these OIHPs have various self-trapping energies and depths (Fig. 13a and b). The CRI of white light emission of the perovskites can be adjusted from 77 for (3APr)PbI4 to 85 for (3APr)PbCl4.79 He et al. synthesized 2D hybrid perovskites AVA2PbClxBr4−x, in which AVA (5-aminovaleric acid) was used as the organic cation. It was found that replacing Br with Cl can not only adjust the band gap of perovskites, but also increase the distortion degree of the inorganic layer. As a result, the luminescence color of these OIHPs evolves from narrow-band blue-violet to broadband white-light, which is attributed to a transformation from FE to STE emission with increasing octahedral distortion caused by the Cl substitution (Fig. 13d–g).117 Karunadasa et al. synthesized three OIHPs: (EDBE)[PbX4] (x = Cl, Br and I) (EDBE = 2,2′-(ethylenedioxy)bis(ethylammonium), 36 in Fig. 4). Through changing the halogen anions of the OIHPs, the luminescence quantum yield increased from 2% for (EDBE)[PbCl4] to 9% for (EDBE)[PbBr4], while the light color was tuned from cold white light to warm white light, due to the tunable exciton absorption of the mixed-halides.70
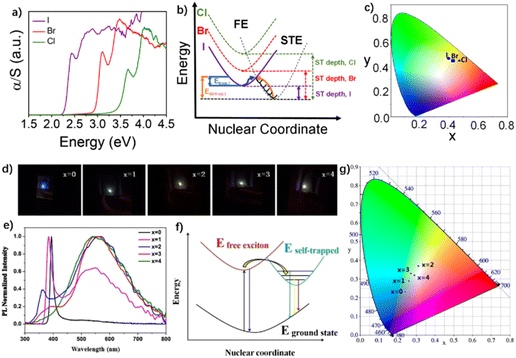 |
| Fig. 13 (a) Optical absorption spectra, (b) mechanism diagram, and (c) chromaticity coordinates of the three compounds in a 1931 color space chromaticity diagram of (3APr)PbX4 (X = I, Br, Cl). Reproduced with permission.79 Copyright 2019, American Chemical Society. (d) Light emission photographs under 325 nm-wavelength UV laser irradiation. (e) Photoluminescence spectra, (f) mechanism diagram, and (g) chromaticity coordinates of the AVA2PbClxBr4−x alloy perovskites in a 1931 color space chromaticity diagram. Reproduced with permission.117 Copyright 2021, Elsevier. | |
Lin et al. reported a series of white-light emitting 2D mixed-halide perovskites: (PEA)2PbBrxCl4−x (0 < x < 4) (PEA = phenylethylamine, 3 in Fig. 4).74 With an increasing content of Br, the Φ of the OIHPs increases gradually from 0.2% to 16.9%, accompanied by a tunable CCT ranging from 4000 K (“warm” white light) to 7000 K (“cold” white light) (Fig. 14a). B2 (x = 2) shows two main emission peaks of high-energy short wavelength (λh) at 395 nm and low-energy long wavelength (λl) at 548 nm, corresponding to the emission of FEs and STEs, respectively. The authors calculated the distortion degree of the three OIHPs: B0 (x = 0), B2, and B4 (x = 4), according to formula (3). It was found that the difference between their distortion degrees is unremarkable, which indicates that these 2D perovskites based on PEA as cations have a similar structural deformation, whether the anion is Cl or Br. According to the luminescence spectra of the mixed-halides (Fig. 14b), the possible decay mechanism of excitons can be speculated as illustrated in Fig. 14c. When the amount of Br is low (such as B0), the excited state energy of FEs is higher than that of STEs. Excitons can spontaneously transfer from FEs to STEs and return through thermal activation, resulting in an equilibrium between two states and a dual emission from the FEs and STEs at λh and λl, respectively. With the increasing amount of Br, the FE energy gradually decreases, while the STE energy increases for a low lattice distortion degree. Consequently, the emission at λh bathochromically shifts from 365 nm for B0 to 410 nm for B3, accompanied by a blue-shifted emission at λl. With an increasing content of Br, the intensities of both emission bands at λh and λl keep increasing. When mixed-halide perovskite powders are applied in the WLEDs, a very high CRI of 91 and pure white emission with a CIE of (0.33, 0.36) could be obtained. Therefore, it is a feasible strategy to adjust the white-light performance of OIHPs by changing the content of Br.
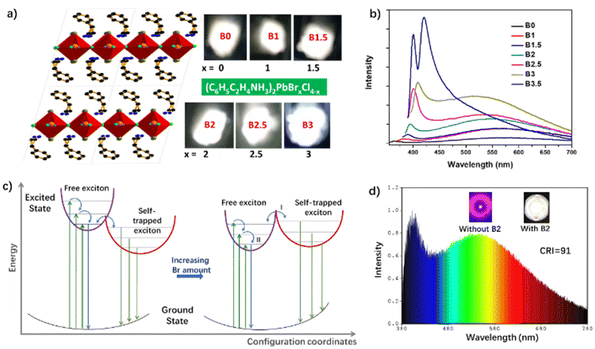 |
| Fig. 14 (a) Crystal structures and photos of (PEA)2PbBrxCl4−x. (b) Emission spectra of (PEA)2PbBrxCl4−x. (c) Effect of Br amount on the free and self-trapped excitons in the mixed-halide perovskites: the straight and curved arrows represent optical transitions and relaxation, respectively. (d) Electroluminescence spectra of B2 loaded with 20% UV-WLED. Inset: photos of LEDs under forward current with and without B2. Reproduced with permission.74 Copyright 2018, American Chemical Society. | |
4.4. Spatial dimension
With the deepening of OIHP research, broad-band white emission has been realized for perovskites of different dimensions (3D, 2D, 1D and 0D), and their luminescence properties are very different. It indicates that there is a close relationship between the spatial dimension and the white-light properties of perovskites.85,118 As a result, the factors that change the spatial dimension of perovskite (such as water, organic cation volume, etc.) can be employed to adjust the white light performance of OIHPs.
4.4.1. Water-induced dimension transformation.
It is found that the presence of water molecules will effectively change the spatial dimension and optical properties of OIHPs.28 Yu et al. synthesized two OIHPs from piperazine and PbBr2: 1D of [C4N2H12]3[PbBr5]2·4DMSO and 0D of [C4N2H12]4[Pb2Br11]Br·4H2O. The 0D perovskite containing water molecules in the lattice shows blue-violet emission with a 2.76% quantum yield. When the water molecules are replaced by DMSO, the 0D perovskite transforms into its 1D counterpart which produces a white emission with a CIE of (0.43, 0.44), CRI of 84 and Φ of 60%.85 By changing the molar ratio of organic cations and inorganic metal ions, Chen et al. have realized the transformation of the perovskite structure from 1D into 0D.95 In this work, the author selected N-(aminocarbonyl)-1,2-diaminoethane hydrobromide (C3N3H9O·2HBr) containing urea amide as organic cations (34 in Fig. 4). When the ratio of (C3N3H9O·2HBr) to PbO is 3
:
2, a 1D molecular structure (C3N3H11O)2Pb2Br9 is formed. When the ratio increases to 2
:
1, urea amide interacts with water molecules through collective hydrogen bonds, forming 0D of (C3N3H11O)2PbBr6·4H2O crystal (Fig. 15a). 1D and 0D crystals are colorless in ambient light, but emit yellow-white light under a UV lamp (Fig. 15d). Both the 0D and 1D perovskites exhibit a wide emission band, centered at 568 nm and 530 nm with CIE coordinates of (0.39, 0.42) and (0.42, 0.44), respectively (Fig. 15c and d), which are suitable for indoor lighting. Because the organic cations in the 0D perovskite combine with water molecules to form large-sized spacers isolating each lead bromide octahedron, the 0D perovskite gives a relatively high quantum yield. The Φ is improved 5 times from 1.7% for 1D to 9.6% for 0D. Therefore, by changing the feeding ratio of the organic and inorganic components, small solvent molecules can be introduced into the crystal to separate the inorganic metal halides and reduce the dimension of the perovskites, which leads to a strong quantum confinement effect, thus improving the white-light quantum yield of the perovskites; consequently, it is a feasible strategy to adjust the luminescence properties by introducing small molecules.
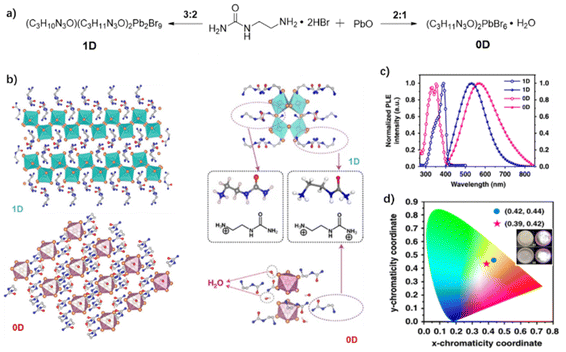 |
| Fig. 15 (a) Synthesis and (b) structural characterization of 1D and 0D lead bromide hybrid crystals. (c) Excitation (open circle) and emission (filled circle) spectra of 1D and 0D crystals at room temperature. (d) CIE chromaticity coordinates of 1D (dot) and 0D (star) crystals, inset: photographs of 1D (up) and 0D (down) crystals taken under ambient light and ultraviolet light. Reproduced with permission.95 Copyright 2019, Springer Nature. | |
4.4.2. Organic cations induce dimension transformation.
The structural change of A-site organic cations can cause different connection modes of inorganic metal halides and spatial dimension of OIHPs. Therefore, in addition to water molecules, the size of the organic cations is also responsible for the transformation of the spatial dimensions. Kanatzidis et al. synthesized a series of OIHPs with 1D, 2D and 3D structures by using different asymmetric diammonium organic cations: 11 (hmp), 13 (2,6-dmpz), 16 (hex), 17 (hep), 21 (mpz), 22 (epz), 23 (4amp) shown in Fig. 4.71 According to single crystal data analysis, it is found that (2,6-dmpz)3Pb2Br10, (hep)PbBr3 and (hex)PbBr3 have a 1D structure connected by edge/corner-sharing or face-sharing modes. Perovskites (mpz)2Pb3Br10, (4amp)PbBr4 and (epz)PbBr4 feature 2D octahedral structures with the connectivity modes of corner- or/and edge-sharing. Perovskite (hmp)PbBr4 shows an edge/corner-sharing 3D octahedral structure (Fig. 16a). Due to the distinct crystal structures of these OIHPs, they show different absorption band gaps and luminescence mechanisms (Fig. 16b). From the emission spectra, it can be seen that the emission peaks have red shifts from 521 nm for 2D (4amp)PbBr4 to 607 nm for 3D (hmp)PbBr4, and then to 674 nm for 1D (hep)PbBr3 (Fig. 16c–e). In addition, the FWHM of various OIHPs is also distinct. For example, the FWHM of 3D (hmp)PbBr4, 2D (4amp)PbBr4, and 1D (hep)PbBr3 is 217 nm, 295 nm, and 435 nm, respectively. By changing the organic cations, the CRI of the OIHPs was adjusted from 76 for (4amp)PbBr4 to 90 for (hmp)PbBr4, while Φ was changed from 0.33% for (mpz)2Pb3Br10 to 12.24% for (2,6-dmpz)3Pb2Br10. Therefore, this work provides a new design strategy for white-light regulation from the perspective of perovskite spatial dimensions.
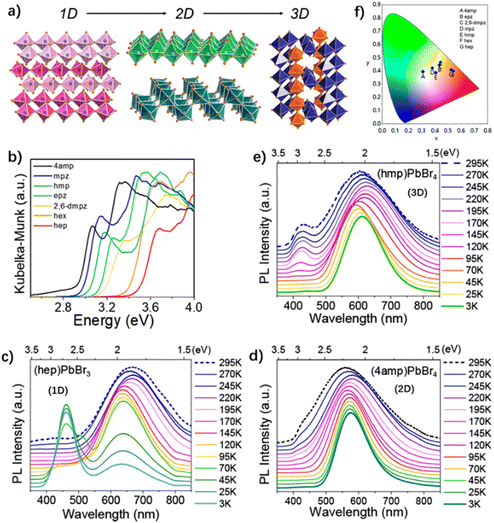 |
| Fig. 16 (a) 1D, 2D, and 3D structure diagram. (b) Optical absorption spectra of the compounds. Temperature-dependent steady-state emission spectra of (c) (hep)PbBr3, (d) (4amp)PbBr4, and (e) (hmp)PbBr4. (f) Chromaticity coordinates of all the compounds in a 1931 color space chromaticity diagram. Reproduced with permission.71 Copyright 2018, American Chemical Society. | |
4.5. Different excitation wavelengths
As mentioned previously, most WLEMs are composed of multiple light-emitting centers with different absorption band gaps, so they usually exhibit excitation-wavelength-dependent characteristics. This provides the possibility to adjust the emission color by changing the excitation wavelength. Liu et al. synthesized a hybrid perovskite, (bmpip)2Pb0.3Sn0.7Br4, which displays an excitation-wavelength-sensitive emission. By changing the excited wavelength, the luminescence color of the OIHP can gradually alter from blue to orange red, and the white emission can be obtained under excitation at 365 nm.58 The same situation applies to the (bmpy)9[SbCl5]2[Pb3Cl11] discussed earlier. Because the SbCl52− and Pb3Cl115− are two independent emission centers of orange-red and blue-green respectively, the emission color of the OIHP can be tuned to warm white light with Φ more than 70% by changing the excitation wavelength (Fig. 6c and f).93 Ma et al. also combined three inorganic metal halide luminescence centers (PbBr42−, MnBr42− and SnBr42−) with the organic cation N-benzyl hexamethylenetetramine (HMTA, 9 in Fig. 4) to synthesize a series of 0D OIHPs with white emission by a host–guest doping method (Fig. 17a).96 The (HMTA)4PbMn0.69Sn0.31Br8 can achieve white light emission with a Φ of 73%. There are three emission peaks at 460 nm, 550 nm and 645 nm in the photoluminescence spectrum of the OIHP excited at 296 nm (Fig. 17b). On the basis of its emission decay curves, the blue (460 nm), green (550 nm) and red (645 nm) emissions are attributed to the PbBr42−,119 MnBr42−,120 and SnBr42− (ref. 121) luminescence centers, respectively (Fig. 17c). Due to the independence of the three light-emitting centers, the effective adjustment from the cold white light to the warm white light of (HMTA)4PbMn0.69Sn0.31Br8 can be realized by changing the excitation wavelength (Fig. 17d and e).
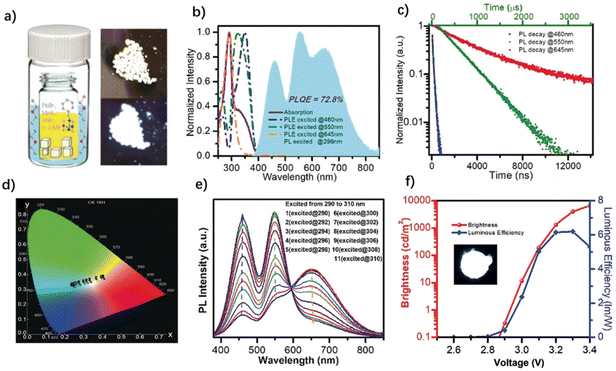 |
| Fig. 17 (a) Illustration of crystal growth and images of (HMTA)4PbMn0.69Sn0.31Br8 under ambient light (top) and UV light (bottom). (b) Absorption, excitation, and emission spectra and (c) emission decay curves of (HMTA)4PbMn0.69Sn0.31Br8. (d) CIE 1931 diagram and (e) excitation-dependent emission with the excitation wavelength ranging from 290 to 380 nm. (f) Voltage versus brightness and luminous efficiency of a UV-pumped LED (inset: image of a UV-pumped LED). Reproduced with permission.96 Copyright 2020, Wiley-VCH. | |
4.6. External stimuli
The aforementioned methods regulating white-light performance often require precise control of the perovskite composition and structure, which increases the difficulty of the design and synthesis of OIHPs. Some OIHP materials exhibit certain instability to the external environment, which makes them have great potential in the field of stimulus response. External stimulation provides a new dimension for the optical regulation of OIHPs. It can effectively control the crystal structure, exciton behavior and physical properties of OIHPs without changing the chemical composition.122 It also plays a vital role in discovering new luminescence phenomena, mechanisms and purposes of OIHPs. For example, the white emission can be achieved by changing the binding energy and proportion of STEs under the external force or heat stimuli.73,123 This section will mainly introduce how to regulate the white-light emission through external stimulation from pressure (or mechanical force), light and heat.
4.6.1. Pressure.
Due to the potential application of pressure-induced emission (PIE) in pressure sensing, great progress has been made in the development of materials with PIE in the past decade.124 Meanwhile, research on the relationship between pressure and the luminescence characteristics of metal halide perovskites has also attracted extensive attention.125–130 PIE phenomena have been found in various low-dimension OIHPs including C4N2H14SnBr4, C4N2H14PbBr4, and (BA)4AgBiBr8 (BA
CH3(CH2)3NH3+), etc.131,132 For example, Zou et al. synthesized a 2D perovskite material (PEA)2PbCl4, which shows a tunable emission from warm-white to cold-white light by a pressure stimulus (Fig. 18a).123 The emission of the perovskite reached the maximum value at 0.4 GPa and increased to 5 times the original value, which is highly associated with the enhanced radiative recombination of STEs. This is because pressure-induced structural amorphization promotes the lattice distortion of the perovskite, which leads to the enhancement of the binding energy and the radiation decay of STEs. The increase of luminescence intensity induced by a pressure stimulus is irreversible. When the pressure is released to the ambient pressure, the emission strength can still reach 1.6 times the original one (Fig. 18b). At the same time, the CIE of the OIHP changes from (0.37, 0.42) to (0.24, 0.29), and the CCT alters from 4403k to 14295k (Fig. 18c). With the change of pressure, there are different conversion behaviors between FEs and STEs (Fig. 18d–f), which indicates that it is an effective strategy to regulate the white-light properties of OIHPs by pressure stimulus.
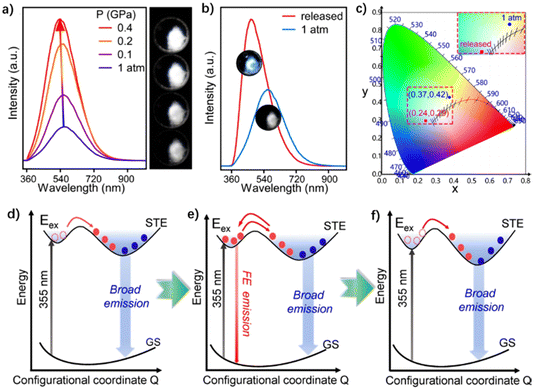 |
| Fig. 18 (a) Emission spectra and photomicrographs of (PEA)2PbCl4 under pressure from 1 atm to 0.4 GPa. (b) Emission spectra of (PEA)2PbCl4 at 1 atm and after release of pressure; inset shows the corresponding photographs. (c) Chromaticity coordinates for 1 atm and after pressure release in a 1931 color space chromaticity diagram. PIE mechanism of (PEA)2PbCl4 at (d) 1 atm, (e) 2.1 GPa, and (f) 10 GPa. Reproduced with permission.123 Copyright 2021, Wiley-VCH. | |
4.6.2. Light.
Among all kinds of external stimuli, photostimulation is clean, non-invasive, easy to operate and remote controlled. Therefore, light is widely used in organic synthesis and property manipulation.133–135 It is very significant to develop OIHP materials with a light-stimulus response, which can regulate their luminous characteristics through illumination. For example, Lin et al. synthesized the 0D perovskite (PA6InCl9) showing an ultralong lifetime (1.2 s) RTP sensitive to a light stimulus (Fig. 12a).42 After illumination for 30 min, the phosphorescence quantum yield of PA6InCl9 increased from 25.2% to 42.8%. The emission intensity of activated PA6InCl9 decreases gradually when it is placed in the dark for a period. After 90 min, the emission intensity almost returned to the level before illumination. It proves that the photoresponse process of the material is reversible. The light-induced phosphorescence enhancement is due to the consumption of triplet oxygen caused by illumination.
The white-light emitting intensity of the OIHPs can also be enhanced upon illumination with UV light. In view of the low white-light efficiency and poor stability of perovskites in high-humidity environments, Lin et al. synthesized a series of Mn-doped and mixed-halide perovskites and their composites with silica gel (Fig. 19a).73 Through optimizing the reaction conditions, highly efficient white-emissive perovskite powders and composite films with Φ up to 39% have been obtained. The resulting composite films exhibit very stable warm white emission under a high humidity and temperature environment. The UV-driven WLEDs based on the films give electroluminescent white light with a CRI of 83–91 and CCT of 2300–4014 K. Interestingly, both perovskite powders and composite films display photoactivated properties under 365 nm of UV light. After 15 min of photoactivation, their emissive intensity at 614 nm is improved 2.1 (for powders) and 2.7 (for films) times (Fig. 19b and c). After stopping the illumination, the emission intensity of the perovskite powders gradually decreases to the original state, indicating that the photoactivation effect of the perovskite powders is reversible. For instance, after 15 minutes of photactivation, the emission intensity of perovskite powders and blend films can be restored to their initial value in 15 minutes and 25 minutes, respectively (Fig. 19d and 19e). It is believed that the photoactivation process is correlated with the surface defects, which can easily lead to a non-radiative recombination of excitons and reduce the luminous intensity of excitons. Consequently, surface passivation of the perovskite powders and composite films induced by small molecules (such as H2O, etc.) and groups (such as –Me, –OH, etc.) would effectively reduce the surface defects and improve the luminous intensity (Fig. 19f).
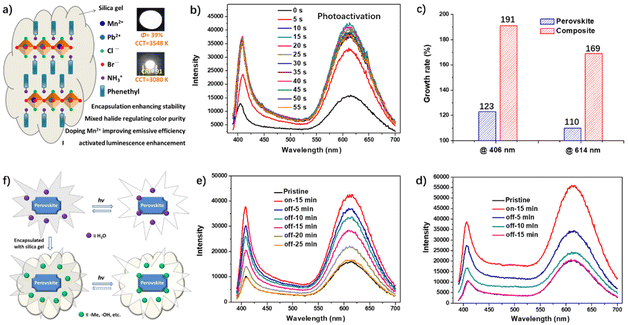 |
| Fig. 19 (a) Structure diagram of the designed perovskite composites. Insert: photos of blend film and LEDs made of perovskite composite. (b) Changes in the photoluminescence spectra of the composite film in the process of photoactivation. (c) Changes of the emissive intensity of the composite film at 406 nm and 614 nm. Changes in the photoluminescence spectra of (d) perovskite powder and (e) blend films in the process of deactivation after 15 min of photoactivation. (f) Possible mechanism of photoactivated luminescence enhancement. Reproduced with permission.73 Copyright 2020, The Royal Society of Chemistry. | |
4.6.3. Heat.
In addition to pressure and light, ambient temperature (or heat) can also affect the exciton distribution and regulate the luminescence properties of perovskite.63 Liu et al. reported that the luminescence centers of the perovskite (bmpip)2Pb0.6Sn0.4Br4 have different responses to temperature. When the temperature rises from 80 to 380 K, the emitting color of the perovskite changes from blue to orange red, achieving a white-light emission with a CIE of (0.36, 0.26) at room temperature.58 By employing tetrabutylammonium (TBA, 41 in Fig. 4) as an organic cation and CuI as an inorganic metal halide, Zou et al. synthesized a 0D perovskite (TBA)2Cu2I4 (Fig. 20a).92 From Fig. 20b and d, it can be seen that (TBA)2Cu2I4 has dual STE emission peaks at 473 nm (peak 1) and 699 nm (peak 2), respectively. With the increase of temperature, the electron phonon coupling strength will gradually increase, thus causing the increasing ratio of the two emission peak intensities (Ipeak 2/Ipeak 1) (Fig. 20c). The authors proposed the possible luminescence mechanism of the OIHP (Fig. 20e). At a low temperature, most excitons are in the emitting state 1 (ES1), and only a few excitons can eclipse the barrier to reach the emitting state 2 (ES2); as a result, the intensity of peak 1 is stronger than that of peak 2. With the increase of temperature, FEs can overcome the energy barrier and reach ES2, which makes the intensity of peak 2 gradually enhance (Fig. 20e). Therefore, the external heat stimulus can effectively control the population of STEs in the emission state, resulting in the special photoluminescence thermochromic behavior of (TBA)2Cu2I4, The CIE of (TBA)2Cu2I4 can gradually move from (0.159, 0.058) at 78 K to (0.293, 0.289) at 298 K with the increase of temperature (Fig. 20f). The corresponding light color can be adjusted from blue at low temperature to cold-white with a Φ of 72.5% at room temperature. The above results show that the white-light properties of (TBA)2Cu2I4 can be regulated by the ambient temperature, which can be used for temperature sensing.
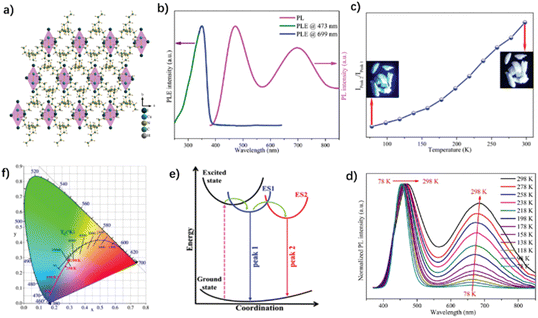 |
| Fig. 20 (a) Crystal structure diagram of (TBA)2Cu2I4. (b) Excited and photoluminescence spectra of (TBA)2Cu2I4 at room temperature. (c) Ratio of the Ipeak 2/Ipeak 1 at different temperatures. (d) Temperature-dependent emission spectra in the temperature range of 78–298 K. (e) Mechanism diagram of (TBA)2Cu2I4. (f) CIE color coordinates of (TBA)2Cu2I4 at different temperatures. Reproduced with permission.92 Copyright 2021, The Royal Society of Chemistry. | |
5. Summary and outlook
In this review, we have summarized the recent progress on OIHPs with white emission, especially the white-light performance regulation. The main contents of this review can be divided into the following aspects. (1) The structures of OIHPs and their luminescence properties. According to the connection mode of metal halide, OIHPs can form four different dimensional structures (3D, 2D, 1D and 0D), corresponding to the different exciton behaviors and optical properties. In the low-dimensional structures, especially the 0D structure, it is easier to generate the broadband emission of STEs. (2) The white-light emission mechanism of OIHPs. There are various types of exciton in perovskite, including the FEs and STEs of inorganic halides, and the organic singlet and triplet excitons. They may all contribute to the broadband white-light emission of OIHPs. As a result, the white light of OIHPs can mainly be attributed to the following three mechanisms: (I) the wide emission of STEs of metal cations, (II) trichromatic or complementary color luminescence composed of the fluorescence or phosphorescence of organic cations, and (III) the combination of inorganic STE emission and organic fluorescence or phosphorescence. (3) The regulation strategy of white emission from OIHPs. Since the optical properties of perovskites (ABX3) are closely related to their composition, structure and external environment, the white-light performance (such as Φ, CIE, CRI and CCT) can be adjusted by changing the organic cations at the A-site, metal ions at the B-site, halogen anions at the X-site, spatial dimension, excitation wavelengths, and external stimuli (light, force, heat, etc.). White-light emissions with Φ up to 100%,42 CRI up to 97,43 and CIE of (0.32, 0.33)96 have been achieved through the regulation of the white emission of OIHPs.
Although significant progress has been made in the photophysical mechanism and performance regulation of white-light emitting OIHPs, a lot of work remains to be explored to meet the needs of lighting applications. Further efforts can be devoted to the following areas:
(1) Improving the stability of the white-light emission of OIHPs. Because the inorganic lattice of hybrid perovskite materials is relatively soft and easily destroyed by water and oxygen in the air, the stability of white light perovskite is poor for long-term use. In addition to the silica gel packaging,73 it is important to develop more methods that can improve the stability of OIHPs. For example, specific functional groups are introduced into the organic cations to passivate crystal defects, thereby reducing the non-radiative transitions of OIHPs. Alternately, the types of metal cation and halogen anion, or the reaction conditions, are changed to increase the rigidity and the stability of the perovskite crystals.
(2) Improving the comprehensive performance of white light. White-light OIHPs with good performance in a single index have been reported, but the comprehensive performance of white light is not satisfactory. For example, Guo et al. synthesized a 3D perovskite (DABCO)(Pb2Cl6) (H2DABCO = 1,4-diazabicyclo[2.2.2]octane-1,4-diium, 15 in Fig. 4). It shows white emission with a very high CRI (up to 96), but very low quantum yield (only 2.5%).44 Therefore, it is still a great challenge to develop white light OIHPs with a high CRI and quantum yield, as well as CIE and CCT close to standard white light. Sophisticated molecular design and complex synthesis processes are needed to achieve high-quality white light.
(3) Development of lead-free OIHPs with white emission. At present, white-light emitting OIHPs with a high quantum yield are mostly lead-containing perovskites. However, lead will do great harm to human health, because it easily enters the human body through the skin, mouth and respiratory tract, to affect human intellectual and bone development, and damage kidney and immune function. The toxicity of Pb hinders the further development of white light OIHPs. Consequently, it is imperative to develop high-performance white light OIHPs without Pb by replacing Pb ions with non-toxic or low toxic metal ions. In particular, the development of metal-free perovskites with white light emission is also a promising direction.136
(4) In-depth understanding about the photophysical mechanism of white emission. The broad-band white light emission of OIHPs often contains multiple emission centers derived from inorganic or/and organic excitons. The existence of organic singlet and triplet excitons, and inorganic FEs and STEs, complicates the white light mechanism of OIHPs. The energy state and decay pathways of these excitons, as well as the energy transfer between them, need to be further explored by combining theoretical calculations, transient absorption/emission spectroscopy, and other advanced tools.
(5) Developing new external stimuli. The luminescence properties of organic/inorganic hybrid perovskites can be changed upon the external stimuli of pressure, light and heat, which can effectively achieve white light emission. In addition to illumination, external force and heating, other external stimuli, such as electricity, magnetism, pH and organic vapor, also need to be developed to achieve a better white-light performance of OIHPs. These new externally stimulated white-light OIHPs can not only be used for solid-state lighting, but also have important application potential in intelligent fields, such as sensing, recognition and information processing, etc.
(6) Exploring more dimension-controllable OIHPs. As previously mentioned, the introduction of water molecules could realize the change of space dimension of perovskites, thus regulating white-light performance. However, there are still few OIHPs that can realize the space dimension transition. Consequently, it is an interesting research direction to explore more factors (such as the stimuli of small organic molecules, pressure or light) that can control the spatial dimension of organic–inorganic hybrid perovskites.
In a word, as an excellent single component white light emitting material, organic–inorganic hybrid perovskite will surely become a dazzling new star to illuminate the world and the future.
Author contributions
The manuscript was written through contributions of all authors. All authors have given approval to the final version of the manuscript.
Conflicts of interest
There are no conflicts to declare.
Acknowledgements
This work was supported by the National Natural Science Foundation of China (22075044).
References
- S. Tonzani, Lighting technology: Time to change the bulb, Nature, 2009, 459, 312–314 CrossRef CAS PubMed
.
- J. Y. Tsao, M. H. Crawford, M. E. Coltrin, A. J. Fischer, D. D. Koleske, G. S. Subramania, G. T. Wang, J. J. Wierer and R. F. Karlicek, Toward Smart and Ultra-efficient Solid-State Lighting, Adv. Opt. Mater., 2014, 2, 809–836 CrossRef CAS
.
- Y. Zhao, Z. H. Lin, Z. G. Zhou, H. M. Yao, W. Lv, H. Y. Zhen and Q. D. Ling, White light-emitting devices based on star-shape like polymers with diarylmaleimde fluorophores on the side chain of polyfluorene arms, Org. Electron., 2016, 31, 183–190 CrossRef CAS
.
- P. Venkatakrishnan, P. Natarajan, J. N. Moorthy, Z. H. Lin and T. J. Chow, Twisted bimesitylene-based oxadiazoles as novel host materials for phosphorescent OLEDs, Tetrahedron, 2012, 68, 7502–7508 CrossRef CAS
.
- Y. S. Lee, Z. H. Lin, Y. Y. Chen, C. Y. Liu and T. J. Chow, Asymmetric indolylmaleimides as non-dopant type red color emitting dyes, Org. Electron., 2010, 11, 604–612 CrossRef CAS
.
- Z. H. Lin, Y. S. Wen and T. J. Chow, White light-emitting devices with a single emitting layer based on bisindolylmaleimide fluorophores, J. Mater. Chem., 2009, 19, 5141–5148 RSC
.
- M. H. Fang, Z. Bao, W. T. Huang and R. S. Liu, Evolutionary Generation of Phosphor Materials and Their Progress in Future Applications for Light-Emitting Diodes, Chem. Rev., 2022, 122, 11474–11513 CrossRef CAS
.
- M. Roushan, X. Zhang and J. Li, Solution-Processable White-Light-Emitting Hybrid Semiconductor Bulk Materials with High Photoluminescence Quantum Efficiency, Angew. Chem., Int. Ed., 2012, 51, 436–439 CrossRef CAS
.
- J. L. Wu, G. Gundiah and A. K. Cheetham, Structure–property correlations in Ce-doped garnet phosphors for use in solid state lighting, Chem. Phys. Lett., 2007, 441, 250–254 CrossRef CAS
.
- S.-P. Lee, C.-H. Huang, T.-S. Chan and T.-M. Chen, New Ce3+-Activated Thiosilicate Phosphor for LED Lighting—Synthesis, Luminescence Studies, and Applications, ACS Appl. Mater. Interfaces, 2014, 6, 7260–7267 CrossRef CAS
.
- Y.-C. Wu, D.-Y. Wang, T.-M. Chen, C.-S. Lee, K.-J. Chen and H.-C. Kuo, A Novel Tunable Green- to Yellow-Emitting β-YFS:Ce3+ Phosphor for Solid-State Lighting, ACS Appl. Mater. Interfaces, 2011, 3, 3195–3199 CrossRef CAS
.
- W. B. Im, N. George, J. Kurzman, S. Brinkley, A. Mikhailovsky, J. Hu, B. F. Chmelka, S. P. DenBaars and R. Seshadri, Efficient and Color-Tunable Oxyfluoride Solid Solution Phosphors for Solid-State White Lighting, Adv. Mater., 2011, 23, 2300–2305 CrossRef CAS PubMed
.
- D. B. Wu, W. J. Gong, H. M. Yao, L. M. Huang, Z. H. Lin and Q. D. Ling, Highly efficient solid-state emission of diphenylfumaronitriles with full-color ATE, and application in explosive sensing, data storage and WLEDs, Dyes Pigm., 2020, 172, 107829 CrossRef
.
- H. Chen, W. G. Zhang, Z. H. Lin and Q. D. Ling, White-light hydrotalcite-like compound emission from the incorporation of red-, green-, and blue-emitting metal complexes, Opt. Mater. Express, 2013, 3, 105–113 CrossRef CAS
.
- N.-N. Zhang, C. Sun, X.-M. Jiang, X.-S. Xing, Y. Yan, L.-Z. Cai, M.-S. Wang and G.-C. Guo, Single-component small-molecule white light organic phosphors, Chem. Commun., 2017, 53, 9269–9272 RSC
.
- V. Anand, R. Mishra and Y. Barot, Recent advances in the development of pure organic white light emitters, Dyes Pigm., 2021, 191, 109390 CrossRef CAS
.
- X.-D. Wang, Y. Song, W.-Y. Pei and J.-F. Ma, Single-Component White Light Emission
from a Metal-Coordinated Cyclotriveratrylene-Based Coordination Polymer, Inorg. Chem., 2022, 61, 10768–10773 CrossRef CAS
.
- T.-T. Xuan, J.-Q. Liu, R.-J. Xie, H.-L. Li and Z. Sun, Microwave-Assisted Synthesis of CdS/ZnS:Cu Quantum Dots for White Light-Emitting Diodes with High Color Rendition, Chem. Mater., 2015, 27, 1187–1193 CrossRef CAS
.
- M. M. Krause, J. Mooney and P. Kambhampati, Chemical and Thermodynamic Control of the Surface of Semiconductor Nanocrystals for Designer White Light Emitters, ACS Nano, 2013, 7, 5922–5929 CrossRef CAS PubMed
.
- S. Mukherjee and P. Thilagar, Organic white-light emitting materials, Dyes Pigm., 2014, 110, 2–27 CrossRef CAS
.
- C. Tang, X.-D. Liu, F. Liu, X.-L. Wang, H. Xu and W. Huang, Recent Progress in Polymer White Light-Emitting Materials and Devices, Macromol. Chem. Phys., 2013, 214, 314–342 CrossRef CAS
.
- S. Q. Wang, H. M. Yao, D. B. Wu, Z. H. Lin and Q. D. Ling, Highly efficient white emission from UV-driven hybrid LEDs through down-conversion of arylmaleimide-based branched polymers, J. Lumin., 2021, 230, 117742 CrossRef CAS
.
- X. Zheng, Y. Huang, D. Xiao, S. Yang, Z. Lin and Q. Ling, Space conjugation induced white light and room-temperature phosphorescence from simple organic small molecules: single-component WLED driven by both UV and blue chips, Mater. Chem. Front., 2021, 5, 6960–6968 RSC
.
- Y. Peng, Y. Yao, L. Li, Z. Wu, S. Wang and J. Luo, White-light emission in a chiral one-dimensional organic–inorganic hybrid perovskite, J. Mater. Chem. C, 2018, 6, 6033–6037 RSC
.
- Y. Lu and B. Yan, Lanthanide organic–inorganic hybrids based on functionalized metal–organic frameworks (MOFs) for a near-UV white LED, Chem. Commun., 2014, 50, 15443–15446 RSC
.
- W. Ki and J. Li, A Semiconductor Bulk Material That Emits Direct White Light, J. Am. Chem. Soc., 2008, 130, 8114–8115 CrossRef CAS
.
- J. Li, X. Li, Y. Tan, X. Yu, F. Yuan, Z. Liu, Z. Bian, Q. Jin, Z. Lu and C. Huang, Construction of High-Quality Cu(I) Complex-Based WOLEDs with Dual Emissive Layers Achieved by an “On-and-Off” Deposition Strategy, Adv. Opt. Mater., 2019, 7, 1801612 CrossRef
.
- B. A. H. Huisman, F. Palazon and H. J. Bolink, Zero-Dimensional Hybrid Organic–Inorganic Lead Halides and Their Post-Synthesis Reversible Transformation into Three-Dimensional Perovskites, Inorg. Chem., 2021, 60, 5212–5216 CrossRef CAS PubMed
.
- P. Fu, M. Huang, Y. Shang, N. Yu, H.-L. Zhou, Y.-B. Zhang, S. Chen, J. Gong and Z. Ning, Organic−Inorganic Layered and Hollow Tin Bromide Perovskite with Tunable Broadband Emission, ACS Appl. Mater. Interfaces, 2018, 10, 34363–34369 CrossRef CAS
.
- V. Morad, Y. Shynkarenko, S. Yakunin, A. Brumberg, R. D. Schaller and M. V. Kovalenko, Disphenoidal Zero-Dimensional Lead, Tin, and Germanium Halides: Highly Emissive Singlet and Triplet Self-Trapped Excitons and X-ray Scintillation, J. Am. Chem. Soc., 2019, 141, 9764–9768 CrossRef CAS PubMed
.
- A. Yangui, R. Roccanova, T. M. McWhorter, Y. Wu, M.-H. Du and B. Saparov, Hybrid Organic–Inorganic Halides (C5H7N2)2MBr4 (M=Hg, Zn) with High Color Rendering Index and High-Efficiency White-Light Emission, Chem. Mater., 2019, 31, 2983–2991 CrossRef CAS
.
- S. M. Yang, B. Zhou, Q. Q. Huang, S. Q. Wang, H. Y. Zhen, D. P. Yan, Z. H. Ling and Q. D. Ling, Highly Efficient Organic Afterglow from a 2D Layered Lead-Free Metal Halide in Both Crystals and Thin Films under an Air Atmosphere, ACS Appl. Mater. Interfaces, 2020, 12, 1419–1426 CrossRef CAS
.
- J. Y. Kim, J.-W. Lee, H. S. Jung, H. Shin and N.-G. Park, High-Efficiency Perovskite Solar Cells, Chem. Rev., 2020, 120, 7867–7918 CrossRef CAS PubMed
.
- H. Chen, L. Fan, R. Zhang, C. Bao, H. Zhao, W. Xiang, W. Liu, G. Niu, R. Guo, L. Zhang and L. Wang, High-Efficiency Formamidinium Lead Bromide Perovskite Nanocrystal-Based Light-Emitting Diodes Fabricated via a Surface Defect Self-Passivation Strategy, Adv. Opt. Mater., 2020, 8, 1901390 CrossRef CAS
.
- J. Cao, Z. Guo, S. Zhu, Y. Fu, H. Zhang, Q. Wang and Z. Gu, Preparation of Lead-free Two-Dimensional-Layered (C8H17NH3)2SnBr4 Perovskite Scintillators and Their Application in X-ray Imaging, ACS Appl. Mater. Interfaces, 2020, 12, 19797–19804 CrossRef CAS PubMed
.
- S. Yakunin, L. Protesescu, F. Krieg, M. I. Bodnarchuk, G. Nedelcu, M. Humer, G. De Luca, M. Fiebig, W. Heiss and M. V. Kovalenko, Low-threshold amplified spontaneous emission and lasing from colloidal nanocrystals of caesium lead halide perovskites, Nat. Commun., 2015, 6, 8056 CrossRef CAS PubMed
.
- H. Huang, B. Pradhan, J. Hofkens, M. B. J. Roeffaers and J. A. Steele, Solar-Driven Metal Halide Perovskite Photocatalysis: Design, Stability, and Performance, ACS Energy Lett., 2020, 5, 1107–1123 CrossRef CAS
.
- G. Zhou, M. Li, J. Zhao, M. S. Molokeev and Z. Xia, Single-Component White-Light Emission in 2D Hybrid Perovskites with Hybridized Halogen Atoms, Adv. Opt. Mater., 2019, 7, 1901335 CrossRef CAS
.
- H. Wu, S. Xu, H. Shao, L. Li, Y. Cui and C. Wang, Single component Mn-doped perovskite-related CsPb2ClxBr5−x nanoplatelets with a record white light quantum yield of 49%: a new single layer color conversion material for light-emitting diodes, Nanoscale, 2017, 9, 16858–16863 RSC
.
- M. D. Smith and H. I. Karunadasa, White-Light Emission from Layered Halide Perovskites, Acc. Chem. Res., 2018, 51, 619–627 CrossRef CAS PubMed
.
- E. R. Dohner, E. T. Hoke and H. I. Karunadasa, Self-Assembly of Broadband White-Light Emitters, J. Am. Chem. Soc., 2014, 136, 1718–1721 CrossRef CAS PubMed
.
- S. Feng, Y. Ma, S. Wang, S. Gao, Q. Huang, H. Zhen, D. Yan, Q. Ling and Z. Lin, Light/Force-Sensitive 0D Lead-Free Perovskites: From Highly Efficient Blue Afterglow to White Phosphorescence with Near-Unity Quantum Efficiency, Angew. Chem., Int. Ed., 2022, 61, e202116511 CAS
.
- G. Song, Z. Li, P. Gong, R.-J. Xie and Z. Lin, Tunable White Light Emission in a Zero-Dimensional Organic–Inorganic Metal Halide Hybrid with Ultra-High Color Rendering Index, Adv. Opt. Mater., 2021, 9, 2002246 CrossRef CAS
.
- G.-E. Wang, G. Xu, M.-S. Wang, L.-Z. Cai, W.-H. Li and G.-C. Guo, Semiconductive 3-D haloplumbate framework hybrids with high color rendering index white-light emission, Chem. Sci., 2015, 6, 7222–7226 RSC
.
- L. Zhou, J.-F. Liao and D.-B. Kuang, An Overview for Zero-Dimensional Broadband Emissive Metal-Halide Single Crystals, Adv. Opt. Mater., 2021, 9, 2100544 CrossRef CAS
.
- G. Zhou, B. Su, J. Huang, Q. Zhang and Z. Xia, Broad-band emission in metal halide perovskites: Mechanism, materials, and applications, Mater. Sci. Eng., R, 2020, 141, 100548 CrossRef
.
- F. Zhang, H. Lu, J. Tong, J. J. Berry, M. C. Beard and K. Zhu, Advances in two-dimensional organic–inorganic hybrid perovskites, Energy Environ. Sci., 2020, 13, 1154–1186 RSC
.
- M. Bidikoudi, E. Fresta and R. D. Costa, White perovskite based lighting devices, Chem. Commun., 2018, 54, 8150–8169 RSC
.
- C. K. Møller, Crystal Structure and Photoconductivity of Cæsium Plumbohalides, Nature, 1958, 182, 1436–1436 CrossRef
.
- S. Gonzalez-Carrero, R. E. Galian and J. Pérez-Prieto, Organic-inorganic and all-inorganic lead halide nanoparticles [Invited], Opt. Express, 2016, 24, A285–A301 CrossRef CAS PubMed
.
- M. I. Saidaminov, O. F. Mohammed and O. M. Bakr, Low-Dimensional-Networked Metal Halide Perovskites: The Next Big Thing, ACS Energy Lett., 2017, 2, 889–896 CrossRef CAS
.
- S. Ghimire and C. Klinke, Two-dimensional halide perovskites: synthesis, optoelectronic properties, stability, and applications, Nanoscale, 2021, 13, 12394–12422 RSC
.
- L. Chouhan, S. Ghimire, C. Subrahmanyam, T. Miyasaka and V. Biju, Synthesis, optoelectronic properties and applications of halide perovskites, Chem. Soc. Rev., 2020, 49, 2869–2885 RSC
.
- L. Dou, A. B. Wong, Y. Yu, M. Lai, N. Kornienko, S. W. Eaton, A. Fu, C. G. Bischak, J. Ma, T. Ding, N. S. Ginsberg, L.-W. Wang, A. P. Alivisatos and P. Yang, Atomically thin two-dimensional organic-inorganic hybrid perovskites, Science, 2015, 349, 1518–1521 CrossRef CAS PubMed
.
- Y. Han, J. Yin, G. Cao, Z. Yin, Y. Dong, R. Chen, Y. Zhang, N. Li, S. Jin, O. F. Mohammed, B.-B. Cui and Q. Chen, Exciton Self-Trapping for White Emission in 100-Oriented Two-Dimensional Perovskites via Halogen Substitution, ACS Energy Lett., 2022, 7, 453–460 CrossRef CAS
.
- W. Ke, L. Mao, C. C. Stoumpos, J. Hoffman, I. Spanopoulos, A. D. Mohite and M. G. Kanatzidis, Compositional and Solvent Engineering in Dion–Jacobson 2D Perovskites Boosts Solar Cell Efficiency and Stability, Adv. Energy Mater., 2019, 9, 1803384 CrossRef
.
- Z. Qi, Y. Chen, Y. Guo, X. Yang, F.-Q. Zhang, G. Zhou and X.-M. Zhang, Broadband white-light emission in a one-dimensional organic–inorganic hybrid cadmium chloride with face-sharing CdCl6 octahedral chains, J. Mater. Chem. C, 2021, 9, 88–94 RSC
.
- L. Fan, K. Liu, Q. Zeng, M. Li, H. Cai, J. Zhou, S. He, J. Zhao and Q. Liu, Efficiency-Tunable Single-Component White-Light Emission Realized in Hybrid Halides Through Metal Co-Occupation, ACS Appl. Mater. Interfaces, 2021, 13, 29835–29842 CrossRef CAS
.
- J. Zhou, M. Li, L. Ning, R. Zhang, M. S. Molokeev, J. Zhao, S. Yang, K. Han and Z. Xia, Broad-Band Emission in a Zero-Dimensional Hybrid Organic [PbBr6] Trimer with Intrinsic Vacancies, J. Phys. Chem. Lett., 2019, 10, 1337–1341 CrossRef CAS
.
- Z. Yuan, C. Zhou, Y. Tian, Y. Shu, J. Messier, J. C. Wang, L. J. van de Burgt, K. Kountouriotis, Y. Xin, E. Holt, K. Schanze, R. Clark, T. Siegrist and B. Ma, One-dimensional organic lead halide perovskites with efficient bluish white-light emission, Nat. Commun., 2017, 8, 14051 CrossRef CAS PubMed
.
- M. D. Smith, A. Jaffe, E. R. Dohner, A. M. Lindenberg and H. I. Karunadasa, Structural origins of broadband emission from layered Pb–Br hybrid perovskites, Chem. Sci., 2017, 8, 4497–4504 RSC
.
- R. Zhang, X. Mao, Y. Yang, S. Yang, W. Zhao, T. Wumaier, D. Wei, W. Deng and K. Han, Air-Stable, Lead-Free Zero-Dimensional Mixed Bismuth-Antimony
Perovskite Single Crystals with Ultra-broadband Emission, Angew. Chem., Int. Ed., 2019, 58, 2725–2729 CrossRef CAS PubMed
.
- H. Peng, Y. Tian, X. Wang, T. Huang, Z. Yu, Y. Zhao, T. Dong, J. Wang and B. Zou, Pure White Emission with 91.9% Photoluminescence Quantum Yield of [(C3H7)4N]2Cu2I4 out of Polaronic States and Ultra-High Color Rendering Index, ACS Appl. Mater. Interfaces, 2022, 14, 12395–12403 CrossRef CAS
.
- J. Luo, X. Wang, S. Li, J. Liu, Y. Guo, G. Niu, L. Yao, Y. Fu, L. Gao, Q. Dong, C. Zhao, M. Leng, F. Ma, W. Liang, L. Wang, S. Jin, J. Han, L. Zhang, J. Etheridge, J. Wang, Y. Yan, E. H. Sargent and J. Tang, Efficient and stable emission of warm-white light from lead-free halide double perovskites, Nature, 2018, 563, 541–545 CrossRef CAS PubMed
.
- S. Li, J. Luo, J. Liu and J. Tang, Self-Trapped Excitons in All-Inorganic Halide Perovskites: Fundamentals, Status, and Potential Applications, J. Phys. Chem. Lett., 2019, 10, 1999–2007 CrossRef CAS PubMed
.
- C. Sun, W.-L. He, M.-J. Liu, W.-J. Pan, L.-F. Dong, G. Chen, G.-D. Liu and X.-W. Lei, Zero-Dimensional Hybrid Cd-Based Perovskites with Broadband Bluish White-Light Emissions, Chem. – Asian J., 2020, 15, 3050–3058 CrossRef CAS PubMed
.
- S. Wang, Y. Yao, J. Kong, S. Zhao, Z. Sun, Z. Wu, L. Li and J. Luo, Highly efficient white-light emission in a polar two-dimensional hybrid perovskite, Chem. Commun., 2018, 54, 4053–4056 RSC
.
- T. Hu, M. D. Smith, E. R. Dohner, M.-J. Sher, X. Wu, M. T. Trinh, A. Fisher, J. Corbett, X. Y. Zhu, H. I. Karunadasa and A. M. Lindenberg, Mechanism for Broadband White-Light Emission from Two-Dimensional (110) Hybrid Perovskites, J. Phys. Chem. Lett., 2016, 7, 2258–2263 CrossRef CAS PubMed
.
- C. Zhou, H. Lin, Y. Tian, Z. Yuan, R. Clark, B. Chen, L. J. van de Burgt, J. C. Wang, Y. Zhou, K. Hanson, Q. J. Meisner, J. Neu, T. Besara, T. Siegrist, E. Lambers, P. Djurovich and B. Ma, Luminescent zero-dimensional organic metal halide hybrids with near-unity quantum efficiency, Chem. Sci., 2018, 9, 586–593 RSC
.
- E. R. Dohner, A. Jaffe, L. R. Bradshaw and H. I. Karunadasa, Intrinsic White-Light Emission from Layered Hybrid Perovskites, J. Am. Chem. Soc., 2014, 136, 13154–13157 CrossRef CAS PubMed
.
- L. Mao, P. Guo, M. Kepenekian, I. Hadar, C. Katan, J. Even, R. D. Schaller, C. C. Stoumpos and M. G. Kanatzidis, Structural Diversity in White-Light-Emitting Hybrid Lead Bromide Perovskites, J. Am. Chem. Soc., 2018, 140, 13078–13088 CrossRef CAS PubMed
.
- Q. Huang, S. Yang, S. Feng, H. Zhen, Z. Lin and Q. Ling, Multicolor Output from 2D Hybrid Perovskites with Wide Band Gap: Highly Efficient White Emission, Dual-Color Afterglow, and Switch between Fluorescence and Phosphorescence, J. Phys. Chem. Lett., 2021, 12, 1040–1045 CrossRef CAS PubMed
.
- S. Wang, D. Wu, S. Yang, H. Zhen, Z. Lin and Q. Ling, Highly-efficient and stable warm white emission from perovskite/silica composites with photoactivated luminescence enhancement, J. Mater. Chem. C, 2020, 8, 12623–12631 RSC
.
- S. Yang, Z. Lin, J. Wang, Y. Chen, Z. Liu, E. Yang, J. Zhang and Q. Ling, High Color Rendering Index White-Light Emission from UV-Driven LEDs Based on Single Luminescent Materials: Two-Dimensional Perovskites (C6H5C2H4NH3)2PbBrxCl4−x, ACS Appl. Mater. Interfaces, 2018, 10, 15980–15987 CrossRef CAS
.
- S. Yang, D. Wu, W. Gong, Q. Huang, H. Zhen, Q. Ling and Z. Lin, Highly efficient room-temperature phosphorescence and afterglow luminescence from common organic fluorophores in 2D hybrid perovskites, Chem. Sci., 2018, 9, 8975–8981 RSC
.
- C.-Q. Jing, J. Wang, H.-F. Zhao, W.-X. Chu, Y. Yuan, Z. Wang, M.-F. Han, T. Xu, J.-Q. Zhao and X.-W. Lei, Improving Broadband White-Light Emission Performances of 2D Perovskites by Subtly Regulating Organic Cations, Chem. – Eur. J., 2020, 26, 10307–10313 CrossRef CAS PubMed
.
- H. Hu, S. A. Morris, X. Qiao, D. Zhao, T. Salim, B. Chen, E. E. M. Chia and Y. M. Lam, Molecular engineering of two-dimensional hybrid perovskites with broadband emission for white light-emitting diodes, J. Mater. Chem. C, 2018, 6, 10301–10307 RSC
.
- G. Zhou, X. Jiang, M. Molokeev, Z. Lin, J. Zhao, J. Wang and Z. Xia, Optically Modulated Ultra-Broad-Band Warm White Emission in Mn2+-Doped (C6H18N2O2)PbBr4 Hybrid Metal Halide Phosphor, Chem. Mater., 2019, 31, 5788–5795 CrossRef CAS
.
- X. Li, P. Guo, M. Kepenekian, I. Hadar, C. Katan, J. Even, C. C. Stoumpos, R. D. Schaller and M. G. Kanatzidis, Small Cyclic Diammonium Cation Templated (110)-Oriented 2D Halide (X=I, Br, Cl) Perovskites with White-Light Emission, Chem. Mater., 2019, 31, 3582–3590 CrossRef CAS
.
- M.-H. Jung, White-Light Emission from the Structural Distortion Induced by Control of Halide Composition of Two-Dimensional Perovskites ((C6H5CH2NH3)2PbBr4−xClx), Inorg. Chem., 2019, 58, 6748–6757 CrossRef CAS PubMed
.
- Z. Wu, C. Ji, Z. Sun, S. Wang, S. Zhao, W. Zhang, L. Li and J. Luo, Broadband white-light emission with a high color rendering index in a two-dimensional organic–inorganic hybrid perovskite, J. Mater. Chem. C, 2018, 6, 1171–1175 RSC
.
- S. Wang, Y. Yao, Z. Wu, Y. Peng, L. Li and J. Luo, Realization of “warm” white light via halide substitution in polar two-dimensional hybrid perovskites (2meptH2)PbClxBr4−x, J. Mater. Chem. C, 2018, 6, 12267–12272 RSC
.
- L. Mao, Y. Wu, C. C. Stoumpos, M. R. Wasielewski and M. G. Kanatzidis, White-Light Emission and Structural Distortion in New Corrugated Two-Dimensional Lead Bromide Perovskites, J. Am. Chem. Soc., 2017, 139, 5210–5215 CrossRef CAS
.
- L. Mao, Y. Wu, C. C. Stoumpos, B. Traore, C. Katan, J. Even, M. R. Wasielewski and M. G. Kanatzidis, Tunable White-Light Emission in Single-Cation-Templated Three-Layered 2D Perovskites (CH3CH2NH3)4Pb3Br10−xClx, J. Am. Chem. Soc., 2017, 139, 11956–11963 CrossRef CAS PubMed
.
- G. Yu, F. Lin, K. Zhou, S. Fang, Y. Shi, W. Liu, H. Hu, B. Ma and H. Lin, One-Dimensional Organic–Metal Halide with Highly Efficient Warm White-Light Emission and Its Moisture-Induced Structural Transformation, Chem. Mater., 2021, 33, 5668–5674 CrossRef CAS
.
- A. Biswas, R. Bakthavatsalam, S. R. Shaikh, A. Shinde, A. Lohar, S. Jena, R. G. Gonnade and J. Kundu, Efficient
Broad-Band Emission from Contorted Purely Corner-Shared One Dimensional (1D) Organic Lead Halide Perovskite, Chem. Mater., 2019, 31, 2253–2257 CrossRef CAS
.
- H. Xu, Z. Zhang, X. Dong, L. Huang, H. Zeng, Z. Lin and G. Zou, Corrugated 1D Hybrid Metal Halide [C6H7ClN]CdCl3 Exhibiting Broadband White-Light Emission, Inorg. Chem., 2022, 61, 4752–4759 CrossRef CAS PubMed
.
- W.-F. Zhang, W.-J. Pan, T. Xu, R.-Y. Song, Y.-Y. Zhao, C.-Y. Yue and X.-W. Lei, One-Dimensional Face-Shared Perovskites with Broad-Band Bluish White-Light Emissions, Inorg. Chem., 2020, 59, 14085–14092 CrossRef CAS
.
- S. Elleuch, A. Lusson, S. Pillet, K. Boukheddaden and Y. Abid, White Light Emission from a Zero-Dimensional Lead Chloride Hybrid Material, ACS Photonics, 2020, 7, 1178–1187 CrossRef CAS
.
- A. Yangui, R. Roccanova, Y. Wu, M.-H. Du and B. Saparov, Highly Efficient Broad-Band Luminescence Involving Organic and Inorganic Molecules in a Zero-Dimensional Hybrid Lead Chloride, J. Phys. Chem. C, 2019, 123, 22470–22477 CrossRef CAS
.
- X. Zhang, L. Li, S. Wang, X. Liu, Y. Yao, Y. Peng, M. Hong and J. Luo, [(N-AEPz)ZnCl4]Cl: A “Green” Metal Halide Showing Highly Efficient Bluish-White-Light Emission, Inorg. Chem., 2020, 59, 3527–3531 CrossRef CAS PubMed
.
- H. Peng, Y. Xiao, Y. Tian, X. Wang, T. Huang, T. Dong, Y. Zhao, J. Wang and B. Zou, Dual self-trapped exciton emission of (TBA)2Cu2I4: optical properties and high anti-water stability, J. Mater. Chem. C, 2021, 9, 16014–16021 RSC
.
- C. Zhou, S. Lee, H. Lin, J. Neu, M. Chaaban, L.-J. Xu, A. Arcidiacono, Q. He, M. Worku, L. Ledbetter, X. Lin, J. A. Schlueter, T. Siegrist and B. Ma, Bulk Assembly of Multicomponent Zero-Dimensional Metal Halides with Dual Emission, ACS Mater. Lett., 2020, 2, 376–380 CrossRef CAS
.
- Z. Li, G. Song, Y. Li, L. Wang, T. Zhou, Z. Lin and R.-J. Xie, Realizing Tunable White Light Emission in Lead-Free Indium(III) Bromine Hybrid Single Crystals through Antimony(III) Cation Doping, J. Phys. Chem. Lett., 2020, 11, 10164–10172 CrossRef CAS
.
- B.-B. Cui, Y. Han, B. Huang, Y. Zhao, X. Wu, L. Liu, G. Cao, Q. Du, N. Liu, W. Zou, M. Sun, L. Wang, X. Liu, J. Wang, H. Zhou and Q. Chen, Locally collective hydrogen bonding isolates lead octahedra for white emission improvement, Nat. Commun., 2019, 10, 5190 CrossRef
.
- L.-J. Xu, S. Lee, X. Lin, L. Ledbetter, M. Worku, H. Lin, C. Zhou, H. Liu, A. Plaviak and B. Ma, Multicomponent Organic Metal Halide Hybrid with White Emissions, Angew. Chem., Int. Ed., 2020, 59, 14120–14123 CrossRef CAS PubMed
.
- R. Roccanova, M. Houck, A. Yangui, D. Han, H. Shi, Y. Wu, D. T. Glatzhofer, D. R. Powell, S. Chen, H. Fourati, A. Lusson, K. Boukheddaden, M.-H. Du and B. Saparov, Broadband Emission in Hybrid Organic–Inorganic Halides of Group 12 Metals, ACS Omega, 2018, 3, 18791–18802 CrossRef CAS
.
- A. Yangui, D. Garrot, J. S. Lauret, A. Lusson, G. Bouchez, E. Deleporte, S. Pillet, E. E. Bendeif, M. Castro, S. Triki, Y. Abid and K. Boukheddaden, Optical Investigation of Broadband White-Light Emission in Self-Assembled Organic–Inorganic Perovskite (C6H11NH3)2PbBr4, J. Phys. Chem. C, 2015, 119, 23638–23647 CrossRef CAS
.
- A. Yangui, S. Pillet, E.-E. Bendeif, A. Lusson, S. Triki, Y. Abid and K. Boukheddaden, Broadband Emission in a New Two-Dimensional Cd-Based Hybrid Perovskite, ACS Photonics, 2018, 5, 1599–1611 CrossRef CAS
.
- K. Thirumal, W. K. Chong, W. Xie, R. Ganguly, S. K. Muduli, M. Sherburne, M. Asta, S. Mhaisalkar, T. C. Sum, H. S. Soo and N. Mathews, Morphology-Independent Stable White-Light Emission from Self-Assembled Two-Dimensional Perovskites Driven by Strong Exciton–Phonon Coupling to the Organic Framework, Chem. Mater., 2017, 29, 3947–3953 CrossRef CAS
.
- M.-S. Wang and G.-C. Guo, Inorganic–organic hybrid white light phosphors, Chem. Commun., 2016, 52, 13194–13204 RSC
.
- S. Krishnamurthy, R. Naphade, W. J. Mir, S. Gosavi, S. Chakraborty, R. Vaidhyanathan and S. Ogale, Molecular and Self-Trapped Excitonic Contributions to the Broadband Luminescence in Diamine-Based Low-Dimensional Hybrid Perovskite Systems, Adv. Opt. Mater., 2018, 6, 1800751 CrossRef
.
- H. Barkaoui, H. Abid, A. Yangui, S. Triki, K. Boukheddaden and Y. Abid, Yellowish White-Light Emission Involving Resonant Energy Transfer in a New One-Dimensional Hybrid Material: (C9H10N2)PbCl4, J. Phys. Chem. C, 2018, 122, 24253–24261 CrossRef CAS
.
- C. Sun, Y.-H. Guo, Y. Yuan, W.-X. Chu, W.-L. He, H.-X. Che, Z.-H. Jing, C.-Y. Yue and X.-W. Lei, Broadband White-Light Emission in One-Dimensional Organic–Inorganic Hybrid Silver Halide, Inorg. Chem., 2020, 59, 4311–4319 CrossRef CAS PubMed
.
- C. Zhou, Y. Tian, O. Khabou, M. Worku, Y. Zhou, J. Hurley, H. Lin and B. Ma, Manganese-Doped One-Dimensional Organic Lead Bromide Perovskites with Bright White Emissions, ACS Appl. Mater. Interfaces, 2017, 9, 40446–40451 CrossRef CAS
.
- J.-H. Wei, J.-F. Liao, L. Zhou, J.-B. Luo, X.-D. Wang and D.-B. Kuang, Indium-antimony-halide single crystals for high-efficiency white-light emission and anti-counterfeiting, Sci. Adv., 2021, 7, eabg3989 CrossRef CAS PubMed
.
- Q. Q. Huang, H. Q. Gao, S. M. Yang, D. Ding, Z. H. Lin and Q. D. Ling, Ultrastable and colorful afterglow from organic luminophores in amorphous nanocomposites: advanced anti-counterfeiting and in vivo imaging application, Nano Res., 2020, 13, 1035–1043 CrossRef CAS
.
- S. Q. Wang, X. L. Wang, S. W. Feng, W. Lv, M. J. Lin, Q. D. Ling and Z. H. Lin, Cluster-luminescent polysiloxane nanomaterials: adjustable full-color ultralong room temperature phosphorescence and a highly sensitive response to silver ions, Inorg. Chem. Front., 2022, 9, 3619–3626 RSC
.
- X. Zheng, Y. S. Huang, W. Lv, J. Z. Fan, Q. D. Ling and Z. H. Lin, Nearly Unity Quantum Yield Persistent Room-Temperature Phosphorescence from Heavy Atom-Free Rigid Inorganic/Organic Hybrid Frameworks, Angew. Chem., Int. Ed., 2022, 61, e202207104 CAS
.
- Y. Huang, X. Zheng, Z. Yao, W. Lv, S. Xiang, Q. Ling and Z. Lin, Multimode stimuli responsive dual-state organic room temperature phosphorescence from a phenanthrene derivative, Chem. Eng. J., 2022, 444, 136629 CrossRef CAS
.
- Q. Huang, Z. Lin and D. Yan, Tuning Organic Room-Temperature Phosphorescence through the Confinement Effect of Inorganic Micro/Nanostructures, Small
Struct., 2021, 2, 2100044 CrossRef CAS
.
- T. Sheikh, V. Nawale, N. Pathoor, C. Phadnis, A. Chowdhury and A. Nag, Molecular Intercalation and Electronic Two Dimensionality in Layered Hybrid Perovskites, Angew. Chem., Int. Ed., 2020, 59, 11653–11659 CrossRef CAS PubMed
.
- J.-Q. Zhao, C.-Q. Jing, J.-H. Wu, W.-F. Zhang, L.-J. Feng, C.-Y. Yue and X.-W. Lei, Systematic Approach of One-Dimensional Lead Perovskites with Face-Sharing Connectivity to Realize Efficient and Tunable Broadband Light Emission, J. Phys. Chem. C, 2021, 125, 10850–10859 CrossRef CAS
.
- A. A. Koegel, E. M. Mozur, I. W. H. Oswald, N. H. Jalarvo, T. R. Prisk, M. Tyagi and J. R. Neilson, Correlating Broadband Photoluminescence with Structural Dynamics in Layered Hybrid Halide Perovskites, J. Am. Chem. Soc., 2022, 144, 1313–1322 CrossRef CAS PubMed
.
- H. Yuan, F. Massuyeau, N. Gautier, A. B. Kama, E. Faulques, F. Chen, Q. Shen, L. Zhang, M. Paris and R. Gautier, Doped Lead Halide White Phosphors for Very High Efficiency and Ultra-High Color Rendering, Angew. Chem., Int. Ed., 2020, 59, 2802–2807 CrossRef CAS PubMed
.
- M. Li, Y. Li, M. S. Molokeev, J. Zhao, G. Na, L. Zhang and Z. Xia, Halogen Substitution in Zero-Dimensional Mixed Metal Halides toward Photoluminescence Modulation and Enhanced Quantum Yield, Adv. Opt. Mater., 2020, 8, 2000418 CrossRef CAS
.
- H. He, G. Tong, Y. Shi, R. Wang, Y. Liu, J. Chen, N. Thirugnanam, J. Chen and Y. He, Tunable bandgap and luminescence characters in single-phase two-dimensional perovskite AVA2PbClxBr4-x alloys, J. Mater. Res. Technol., 2021, 15, 5353–5359 CrossRef CAS
.
- L. Mao, P. Guo, M. Kepenekian, I. Hadar, C. Katan, J. Even, R. D. Schaller, C. C. Stoumpos and M. G. Kanatzidis, Structural Diversity in White-Light-Emitting Hybrid Lead Bromide Perovskites, J. Am. Chem. Soc., 2018, 140, 13078–13088 CrossRef CAS
.
- H. Lin, C. Zhou, M. Chaaban, L.-J. Xu, Y. Zhou, J. Neu, M. Worku, E. Berkwits, Q. He, S. Lee, X. Lin, T. Siegrist, M.-H. Du and B. Ma, Bulk Assembly of Zero-Dimensional Organic Lead Bromide Hybrid with Efficient Blue Emission, ACS Mater. Lett., 2019, 1, 594–598 CrossRef CAS
.
- L.-J. Xu, C.-Z. Sun, H. Xiao, Y. Wu and Z.-N. Chen, Green-Light-Emitting Diodes based on Tetrabromide Manganese(II) Complex through Solution Process, Adv. Mater., 2017, 29, 1605739 CrossRef PubMed
.
- C. Zhou, H. Lin, H. Shi, Y. Tian, C. Pak, M. Shatruk, Y. Zhou, P. Djurovich, M.-H. Du and B. Ma, A Zero-Dimensional Organic Seesaw-Shaped Tin Bromide with Highly Efficient Strongly Stokes-Shifted Deep-Red Emission, Angew. Chem., Int. Ed., 2018, 57, 1021–1024 CrossRef CAS
.
- A. A. Zhumekenov, M. I. Saidaminov, O. F. Mohammed and O. M. Bakr, Stimuli-responsive switchable halide perovskites: Taking advantage of instability, Joule, 2021, 5, 2027–2046 CrossRef CAS
.
- R. Fu, W. Zhao, L. Wang, Z. Ma, G. Xiao and B. Zou, Pressure-Induced Emission toward Harvesting Cold White Light from Warm White Light, Angew. Chem., Int. Ed., 2021, 60, 10082–10088 CrossRef CAS PubMed
.
- Z. Wang and X. Huang, Luminescent Organic-Inorganic Hybrid Metal Halides: An Emerging Class of Stimuli-Responsive Materials, Chem. – Eur. J., 2022, 28, e202200609 CAS
.
- L. Wang, P. Yao, F. Wang, S. Li, Y. Chen, T. Xia, E. Guo, K. Wang, B. Zou and H. Guo, Pressure-Induced Structural Evolution and Bandgap Optimization of Lead-Free Halide Double Perovskite (NH4)2SeBr6, Adv. Mater., 2020, 7, 1902900 CAS
.
- S. Guo, K. Bu, J. Li, Q. Hu, H. Luo, Y. He, Y. Wu, D. Zhang, Y. Zhao, W. Yang, M. G. Kanatzidis and X. Lü, Enhanced Photocurrent of All-Inorganic Two-Dimensional Perovskite Cs2PbI2Cl2 via Pressure-Regulated Excitonic Features, J. Am. Chem. Soc., 2021, 143, 2545–2551 CrossRef CAS
.
- L. Meng, J. M. D. Lane, L. Baca, J. Tafoya, T. Ao, B. Stoltzfus, M. Knudson, D. Morgan, K. Austin, C. Park, P. Chow, Y. Xiao, R. Li, Y. Qin and H. Fan, Shape Dependence of Pressure-Induced Phase Transition in CdS Semiconductor Nanocrystals, J. Am. Chem. Soc., 2020, 142, 6505–6510 CrossRef CAS PubMed
.
- Q. Li, Z. Chen, B. Yang, L. Tan, B. Xu, J. Han, Y. Zhao, J. Tang and Z. Quan, Pressure-Induced Remarkable Enhancement of Self-Trapped Exciton Emission in One-Dimensional CsCu2I3 with Tetrahedral Units, J. Am. Chem. Soc., 2020, 142, 1786–1791 CrossRef CAS PubMed
.
- Y. Wang, H. Zhang, J. Zhu, X. Lü, S. Li, R. Zou and Y. Zhao, Antiperovskites with Exceptional Functionalities, Adv. Mater., 2020, 32, 1905007 CrossRef CAS
.
- G. Xiao, Y. Cao, G. Qi, L. Wang, C. Liu, Z. Ma, X. Yang, Y. Sui, W. Zheng and B. Zou, Pressure Effects on Structure and Optical Properties in Cesium Lead Bromide Perovskite Nanocrystals, J. Am. Chem. Soc., 2017, 139, 10087–10094 CrossRef CAS
.
- Z. Ma, F. Li, L. Sui, Y. Shi, R. Fu, K. Yuan, G. Xiao and B. Zou, Tunable Color Temperatures and Emission Enhancement in 1D Halide Perovskites under High Pressure, Adv. Opt. Mater., 2020, 8, 2000713 CrossRef CAS
.
- Y. Fang, L. Zhang, L. Wu, J. Yan, Y. Lin, K. Wang, W. L. Mao and B. Zou, Pressure-Induced Emission (PIE) and Phase Transition of a Two-dimensional Halide Double Perovskite (BA)4AgBiBr8 (BA=CH3(CH2)3NH3+), Angew. Chem., Int. Ed., 2019, 58, 15249–15253 CrossRef CAS PubMed
.
- X. Zheng, J. Wang, D. Xiao, H. Chen, Z. Lin and Q. Ling, Highly emissive fused diarylmaleimides synthesized by a cascade reaction of selective bromination and visible-light-driven cyclization, Dyes Pigm., 2021, 187, 109113 CrossRef CAS
.
- X.-Y. Yu, J.-R. Chen and W.-J. Xiao, Visible Light-Driven Radical-Mediated C–C Bond Cleavage/Functionalization in Organic Synthesis, Chem. Rev., 2021, 121, 506–561 CrossRef CAS PubMed
.
- W. Lv, C. Wang, X. C. Lin, X. F. Mei, W. Wang, E. Yang, Q. D. Ling and Z. H. Lin, Dithienylmaleimide-based D-A Conjugated Polymer Film: Photo-Responsive Behavior and Application in Electrical Memory and Logic Gates, Chin. J. Polym. Sci., 2021, 39, 1177–1184 CrossRef CAS
.
- S. Feng, Q. Huang, S. Yang, Z. Lin and Q. Ling, A metal-free 2D layered organic ammonium halide framework realizing full-color persistent room-temperature phosphorescence, Chem. Sci., 2021, 12, 14451–14458 RSC
.
|
This journal is © the Partner Organisations 2023 |
Click here to see how this site uses Cookies. View our privacy policy here.