DOI:
10.1039/D3MA00623A
(Review Article)
Mater. Adv., 2023,
4, 6464-6477
Advances in graphene-based nanoplatforms and their application in Parkinson's disease
Received
30th August 2023
, Accepted 4th November 2023
First published on 7th November 2023
Abstract
Parkinson's disease (PD) is a progressive, complex, and chronic neurodegenerative disorder that remains challenging to cure and diagnose in the early stage. The neuropathological hallmark of PD are Lewy bodies, which are intracellular protein aggregates composed primarily of α-synuclein (α-syn). The unmet therapeutic and diagnostic needs are projected to be managed by exploring alternative strategies. In this direction, research on using tailored graphene-based nanomaterials (GBNs) is gaining attention due to their capability to affect pathogenic forms of α-syn. In this review, we discuss computational and experimental approaches and look at the benefits of GBNs to target mechanisms contributed to PD neurodegeneration, including α-syn aggregation, autophagy, inflammation, and oxidative stress, for the development of an entirely new class of antiparkinsonian therapy. We overview advanced GBN-based nanomedicines, regenerative medicine, and biosensors supporting the application of GBNs in PD management. Moreover, this review highlights the biocompatibility and safety aspects of emergent GBNs. Although GBNs represent a new and promising approach to PD treatment, as of now, it is limited to computational and early-stage experimental studies, as carefully discussed in this article.
Introduction
Parkinson's disease (PD) is the second most common neurodegenerative movement disorder of the central nervous system (CNS). The pathological hallmarks are aggregation of misfolded α-syn protein in inclusions called Lewy bodies (LBs) and related loss of dopaminergic (DAergic) neurons, resulting in severe motor dysfunction.1 At a prevalence rate of 41 per 100
000 persons, this condition is estimated to affect more than 10 million people globally. Interestingly, the incidence rate rises to 1900 per 100
000 adults over 80, while PD is rarely present in under 40 year-olds. By 2040, 12.9 million people are predicted to be afflicted by this escalating health problem.2 Approximately 10% of PD cases can be associated with genetic factors that run in families. These familial PD cases have been attributed to variants in genes such as SNCA, PRKN, PINK1, LRRK2, PARK2, and PARK7.3 The current evidence on PD suggests that the illness has a complex etiology rather than a single cause. Not all PD patients experience all symptoms at the same time or with the same severity.4 Idiopathic PD can take years to develop, during which numerous risk or protective determinants may come into play to trigger pathogenesis or modify its progression to clinical PD.5 Many pathological processes contribute to PD, including aggregation of normal and abnormal α-syn strains, synaptic and neuronal network dysfunction, impaired proteostasis, cytoskeletal abnormalities, altered energy metabolism, genetic defects, inflammation, and neuronal cell death.6 A clinical diagnosis of PD includes slowness of movement, resting tremor, and muscular rigidity and postural instability.7 Typical late-onset PD with the presence of LBs represents a “complex disease” resulting collectively from exposure history, genetic defects, and related tissue abnormalities. The pathological state is determined by the onset of cellular abnormalities ensuing propagation of its remarkable hallmarks, including pathological α-syn inclusions and chronic inflammation, which progresses with age.8,9 The pathological processes and hallmarks of PD are shown in Fig. 1.
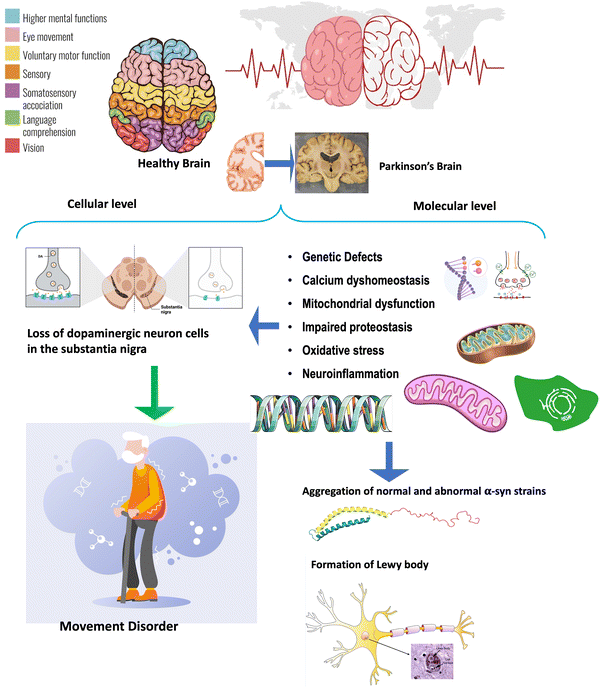 |
| Fig. 1 The neuropathology and pathogenesis of PD. The remarkable neuropathological hallmarks of PD are the loss of DAergic neurons and the presence of LBs, which are intracellular protein aggregates composed primarily of α-syn. Molecular features underlying neurodegeneration are disrupted calcium (Ca2+) homeostasis, mitochondrial dysfunction, impaired proteostasis, oxidative stress, neuroinflammation, and genetic mutations. | |
Dopamine replacement therapy (DRT) – mainly through actual dopamine (DA) and its prodrug, l-dopa – is the gold standard drug treatment for alleviating PD symptoms. However, DRT faces many challenges, such as poor blood–brain barrier (BBB) penetration and decreased response to therapy over time.10 Moreover, the pooling of drugs in the blood may produce side effects due to their distribution to peripheral organs rather than the brain10 as well as various interactions.11 Accordingly, the bioavailability of therapeutics in the brain is compromised. Therefore, developing alternative strategies to overcome these complications is an important challenge in modern medicine.12
Available therapies for advanced PD also include continuous subcutaneous apomorphine infusion, radiofrequency ablation, stereotactic radiosurgery, magnetic resonance imaging-guided focused ultrasound, and deep brain stimulation.13,14 Strategies such as gene- and cell-based therapies, immunotherapy, and fecal microbiota transplantations are being developed at clinical stages.13,15
Interestingly, nanomaterials with unique physicochemical characteristics can traverse the BBB via different mechanisms, exhibit high drug-loading capacity, provide sustained release, protect cargo from immune recognition, increase drug half-life, permit cell-specific targeting, and offer a physical structure that can support cell growth. In addition, due to their intrinsic properties they may bind to fibrils, that results in their disaggregation or contribute to a reduction in oxidative stress. Hence, nanotechnology is an emerging approach in the battle against PD, both in transforming the delivery of therapeutics and creating an entirely new class of therapies able to target canonical challenges of this complex disorder.16
Graphene is investigated as a potential nanomaterial in biomedical applications, stem cell research, cell imaging, and drug/gene delivery due to its excellent conductivity, stability, and biocompatibility.17 Graphene-based nanomaterials (GBNs) with large surface area, high electrical conductivity, and excellent mechanical performance have made their way to the forefront of nanomedicine. GBNs offer a possible effective nano-enabled biosensor for the safe and targeted administration of therapeutic agents for the treatment of PD.18
In this review, we started by presenting the complexity of PD. This is followed by an overview of GBNs and their advances, biocompatibility, applications, and perspectives. This report overviews various attractive possibilities that GBNs offer for multihit therapy to halt PD progression. We discuss experimental approaches taking the benefits of graphene and GBNs to target mechanisms contributing to PD, including α-syn aggregation, autophagy, inflammation, and oxidative stress. Moreover, this review provides updated information on advanced graphene-based nanomedicines, regenerative medicine, and biosensors, supporting their applications in combinatorial PD management.
Graphene-based nanomaterials
Nanomaterials are materials with a size of 1–100 nm and a volume sphere surface area of more than 60 m2 cm−3 in volume, which can be categorized into five groups based on size, origin, potential toxicity, structural configuration, and pore diameters. Nanoparticles have unique chemical, physical, and biological properties at nanoscales compared to their respective particle at higher scales.19 Depending on their structure, nanoparticles are divided into four groups: organic/dendrimers, inorganic, carbon-based, and composite. GBNs belong to the carbon-based nanomaterials.20
Graphene is a kind of carbon nanostructure material that was first produced in 2004.21 It is a two-dimensional layer of sp2 hybridized carbon atoms arranged in a honeycomb lattice, which is the basic building block of other carbon. Each carbon atom has the s, px, and py atomic orbitals that hybridize to form covalent sp2 bonds, which create C–C–C bond angles of 120°. In the hexagonal ring, the σ bond brings carbon atoms together. A π orbital is formed by the remaining pz orbital on each carbon atom and overlaps with its neighbors.22 sp2 bonds provide high electronic mobility, giving graphene excellent electrical conductivity (1738 siemens per m) and good flexibility23 (Fig. 2). Graphene includes a high surface area (2630 m2 g−1), strong mechanical strength (Young's modulus 223C1100 GPa, fracture strength ∼125 GPa), and unparalleled high thermal conductivity (5000 W m−1 K−1).24 GBNs may aggregate in the aqueous medium containing ions, salts, and proteins. GBNs adsorb a variety of aromatic amino acids through a π–π stacking and electrostatic interaction for constructing biosensors and loading drugs.24,25 Thus, there is a need to either modify or functionalize graphene materials.
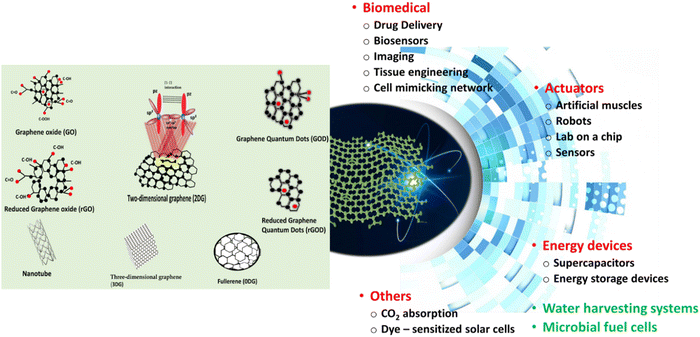 |
| Fig. 2 The structures of graphene and its derivatives and the related potential of advanced technological applications. | |
The characteristic of graphene and its electrochemical, mechanical, and optical excellent properties render it suitable for various biomedical applications. Since 2004, when graphene was discovered, there has been a growing interest in the potential use of graphene and its derivatives, such as graphene oxide (GO), reduced GO (rGO), or graphene quantum dots (GQD) in numerous technologies.26 Various ways of modification of graphene and its derivatives, including covalent functionalization, noncovalent functionalization, substitutional doping, hybridization with nanoparticles, nanowires, and other materials, have been developing to respond to the growing interest in using graphene. Moreover, the monolayer of carbon atoms in graphene can be arranged in three-dimensional graphene (3DG) foams.17 Significantly, modifications and the 3-D manipulation of GBNs could be potentially tailored toward their specific applications.27 These make GBNs, excellent candidates for components of hybrid nanocomposites that show promise in diverse sectors, including energy storage and generation, sensors, actuators, electromagnetic shielding, and biomedical sectors.28 The structures of graphene and its derivatives, alongside the applications, are presented in Fig. 2.
Biocompatibility of GBNs
Nanomaterials are revolutionizing various aspects of medicine by promoting novel sensing, diagnostic, and therapeutic approaches. At the same time, concerns have been raised about their nanotoxicity and general biocompatibility.29 According to the International Standards Organization, the term biocompatibility is the response of living body tissues to materials and is related to compatibility between the material and the host.30 Regarding GNPs, the size, surface functionalization, number of layers, and production method of graphene structures are significant variables determining their biocompatibility and toxicity.31 In addition, the biological effects of GNPs depend on the dose, route, exposure time, and type of cell related to differences in absorption efficiency and degradation capabilities.32 Therefore, various tests using in vitro and in vivo models are required to understand the interactions of GNPs with biological systems to enable further development and safe use.33 Hydrophilic GBNs (such as GO and GQDs) offer good cytocompatibility and low toxicity and strongly promote cell adhesion and differentiation.27 The size of GBNs was found to be closely linked to cytocompatibility. The studies found that nanoscale graphene oxide (GO) caused a less severe inflammatory response compared to micron-scale GO (2 μm).27 In this regard, improving cell compatibility is generally achieved with high hydrophilicity and reduced particle size. However, GO shows dose- and time-dependent cytotoxicity at higher concentrations. Large GBNs can be trapped in the membrane cytoskeleton. Consequently, aggregation of GBNs leads to rupture, deformation, or cell death. Moreover, besides size, the morphology, shape and charge of graphene nanoparticles can influence their cellular uptake, whereas the presence of functional groups impacts their interactions with biomolecules. Importantly, nanoparticle–biomolecular “corona” formation implicates potential nanotoxicity, immune safety, and biocompatibility of GBNs.34 Therefore, surface modifications and coating with biocompatible moieties are developed to mitigate cytotoxicity.35 Interestingly, Rodriguez-Losada et al. found better biocompatibility of the film form than that of the powder. Partially reduced GO-film showed a fully biocompatible surface with no negative impact on mitochondrial function.36In vivo, GBNs' biocompatibility is affected by the route of administration and the rate of biodegradation.30
The potential of GBNs in the battle against PD
GBNs show good biocompatibility, which makes them attractive biomaterials for a wide range of biomedical applications,37 including gene38,39 and drug40 delivery, tissue engineering,41 biosensing,18,42 imaging,17 photothermal,43 and antibacterial44 therapies. Research efforts have also been made regarding the advancements of GBNs in the battle against PD, which tackled targeting synucleinopathy, improving clearance of misfolded proteins, antioxidant, anti-inflammatory and antiviral activities, biosensing, drug delivery and gene and cell therapies.
Synucleinopathy
Proteins in their normal state (soluble monomers) exert physiological functions, which are lost after converting them into cross fibers (insoluble amyloids). However, there is a lack of scientific consensus on whether the accrual of amyloids (proteinopathy) triggers neurodegeneration. Regarding PD, accumulation of misfolded α-syn (synucleinopathy) is a current paradigm, although the depletion of normal α-syn (synucleinopenia) is also suggested.45 α-Syn is a small 140 amino acid residue protein with three regions playing diverse roles in its folding and aggregation. The N-terminal, consisting of amino acids 1–60, is an amphipathic domain, which enables membrane binding with high-curvature membranes enriched in phospholipids, synaptic vesicles that stabilize these helices. Of note, three familial PD mutations: A30P, A53T, and E46K affect the N-terminal portion of the protein and its affinity for lipid membranes as a result. The central region of α-syn (amino acids 61–95) comprises the hydrophobic non-amyloid component (NAC) domain, highly aggregation-prone, which is defended from the cytoplasm via transient intramolecular interactions to prevent protein aggregation. The third, the acidic C-terminal tail of α-syn, is composed of negatively charged amino acids and proline residues, which are known to disrupt secondary protein structure. This region probably interacts with the N-terminal one, forming compact aggregation-resistant monomeric structures and thereby protecting the NAC domain. Phosphorylation at Ser129 or nitration at Tyr125, Tyr133, and Tyr136 promotes the formation of α-syn fibrils or oligomers and reduces the membrane-binding affinity of this protein. Under physiological conditions, α-syn is an intrinsically disordered protein lacking a stable 3D structure and characterized by exacerbated structural plasticity and conformational adaptability. Therefore, it can be easily influenced by neighbouring proteins, membrane lipids, redox state or local pH.46 This high complexity structure and molecular interactions make α-syn cluster into oligomeric species of variable size, shapes, hydrophobicity and content of β-sheet structures, then transforms into amyloid fibrils and megadalton stable assemblies.47 This is in line with clinical evidence that the amyloid fibrils of α-syn have different conformations in different PD patients contributing to the heterogeneity of the disease. Nevertheless, targeting the surfaces of α-syn inclusions is suggested to hold therapeutic and diagnostic potential.47 Amounting evidence indicates that the oligomeric and fibrillar a α-syn are the most toxic assemblies.48 Using molecular dynamics (MD) simulations, Alimohammadi et al. showed that GBNs inhibited α-syn amyloid fibrillation, with nitrogen-doped graphene (N-graphene) having the most significant activity. The observed reduced compactness of α-syn was related to the high absolute value of interaction energy between α-syn and N-graphene, making the bonds stronger and thus preventing the formation of α-syn amyloids. Moreover, N-graphene caused a high increase in the number of hydrogen bonds between water and amyloid molecules and a significant decrease in the area of contact between amyloid particles, which contributed to blocking the active elongation site of the fibrils and a decline in the probability of amyloid formation, respectively.49 Ghaeidamini et al. demonstrated that GO and GQDs under most experimental conditions effectively inhibited α-syn aggregation, revealing that aggregation-enhancing effects can be obtained at low nanoparticle-to-protein ratios. Their study revealed that GO and GQDs inhibited α-syn aggregation by different mechanisms. GO layers sequestrated α-syn monomer, thereby preventing primary nucleation and elongation, while GQDs were adsorbed on aggregated species reducing secondary processes of aggregation.50 Alimohammadi et al. studied the interaction between graphene, CNTs, Si (silicon)–graphene, and Si-CNTs to understand their performance against α-syn pathology. The results of MD showed that nanoparticles, specifically Si-graphene, induced desirable conformational changes promoting a less compact and more unstable α-syn conformation. Significantly, these nanoparticles affected the secondary structures of α-syn, reducing the β-sheets forms that support their capability to prevent the folding and aggregation of α-syn.51 Based on the MD results by Mohammad-Beigi and co-workers (2019), positively and negatively charged graphene sheets interact with terminally charged α-syn N/C residues and then with hydrophobic residues (61–95) of the non-amyloid-β component region driving α-syn fibrillation. Accordingly, graphene sheets not only inhibited α-syn fibrillation (both nucleation and elongation), but even disrupted the mature fibrils.52 This is consistent with the results of He and Zhou, who reported that the (61–95) region of α-syn interacted strongly with planar graphene sandwiched with a hexagonal boron nitride.53 Graphene oxide quantum dots, similarly to carbon quantum dots (CQDs),54 also inhibited fibrillation in a model amyloid-forming protein hen egg-white lysozyme in a dose-dependent manner, presumably through interfacial charge transfer.55 Indeed, GBNs can sequester both α-syn monomers and mature fibrils on their surface and thus inhibit its fibrillation (both nucleation and elongation) and disaggregate the fibrils. This prevents α-syn misfolding, fibrillation and aggregation that holds promises against α-syn pathology-induced neurodegeneration (Fig. 3).56 Of relevance in this context, Mohammadi et al.50 demonstrated that GQDs at a relatively high concentration of 0.5 g mL−1 can promote aggregation of A53T mutant α-syn by shortening the nucleation step of fibrillation.57
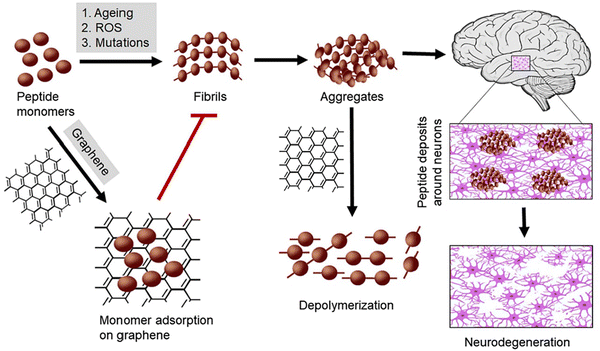 |
| Fig. 3 Neurodegeneration prevention by GBNs. GBNs inhibit the fibrillation of α-syn monomers and depolymerize their aggregated forms. Figure reproduced from reference (Tiwari and Tiwari, 2022)56 with permission from Elsevier, copyright 2023. | |
The most significant research effort on this issue, however, is to examine the effectiveness of GBNs treatment against α-syn toxicity in animal models, and so far, there are only two reports (Table 1). Kim et al. experimentally verified the potential role of GQDs in the fibrillization of α-syn in PD mice models. They found that GQDs successfully passed through the BBB and protected against α-syn pathology and related DAergic neuron loss and neuroinflammation in the substantia nigra (SN) that was accompanied by improvement in motor activity. In vitro, they found that incubating preformed fibrils (PFFs) with GQDs decreased the number of fibrils in a time-depended manner and even direct interaction with mature fibrils with disaggregating effect was demonstrated.1 Ysselstein and Krainc58 considered the significance of this work as a promising novel therapeutic approach to combat PD. However the authors stressed the necessity of understanding the effect of GQDs on the fate of α-syn oligomers in the cell.58 Most recent evidence showed that the engineered surface of GQD with methyl N-allyl N-benzoylmethioninate (MABM) administered to PFFs-injected mice triggered α-syn defibrillation leading to an increase in TH+ positive cells in the stratum and the SN and decline in the inflammatory response that was accompanied by the improvement of locomotor and rearing activity (Table 1).59
Table 1 Animal studies on the multidirectional potential of GBNs for PD management
Dose and route of administration |
Experimental model |
Outcomes |
Ref. |
6-OHDA, 6-hydroxydopamine; 5-HT, 5-hydroxytryptamine; BACE1, β-secretase 1; CAT, catalase, CBF, cerebral blood flow, βCTF, β-secretase C-terminal fragment; DA, dopamine; DAergic, dopaminergic; DOPAC, 3,4-dihydroxyphenylacetic acid; GFAP, glial fibrillary acidic protein; GO, graphene oxide; GONPs, GSH – reduced glutathione, graphene oxide nanoparticles; GOQDs, graphene oxide quantum dots; GQD, graphene quantum dots; HVA, homovanillic acid; LAMP1, lysosomal associated membrane protein 1; LBs, Lewy bodies; Iba-1, ionized calcium binding adapter protein 1; MABM, methyl N-allyl N-benzoylmethioninate; MDA, malondialdehyde; MPP+, 1-methyl-4-phenyl-pyridinium ion; MPTP, 1-methyl-4-phenyl-1,2,3,6-tetrahydropyridine; NR2B, N-methyl D-aspartate receptor subtype 2B; Pue, Puerarin; POCD, postoperative cognitive dysfunction; PSD95, postsynaptic density protein 95, ROS, reactive oxygen species, SN, substantia nigra; SOD, superoxide dismutase; α-syn, α-synuclein; SYP, synaptophysin; TCA, trichloroacetic acid; TH, tyrosine hydroxylase. |
Synucleinopathy |
50 μg GQDs/mouse, i.p., biweekly for 4 months |
α-syn-A53T tg mice |
↑ Motor activity |
Kim et al. 20181 |
↓ p-α-syn |
↑ TH+ and Nissl+ neurons |
↓ Iba-1, GFAP in the SN |
|
50 μg GQDs/mouse, i.p., biweekly for 6 months |
C57BL/6 mice/PFFs stereotaxic intrastriatal injection |
↑ Motor activity |
Kim et al. 20181 |
↑ TH+ and Nissl+ neurons |
↓ Iba-1, GFAP in the SN |
|
50 μL of MABM-GQDs (10 μM), i.p., 3 times weekly for 3 months |
C57BL/6 mice PFFs stereotaxic intrastriatal injection |
↓ p-α-syn+ cells |
Kaliyaperumal et al. 202359 |
↑ TH+ neurons |
↓ Inflammatory response in the SN and ST |
↑CBF |
↑ Motor activity |
|
Misfolded protein clearance |
30 mg GO per kg bw, i.p., for 14 days |
5xFAD mice |
↑ NR2B, SYP and PSD-95 |
Chu et al. 202162 |
↑ Cells and dendritic spines |
↓ Aβ plaque in hippocampus |
↑ Cognitive function |
2 μL of GO (10 mg mL−1) bilateral intracerebral injection |
C57BL/6 mice/POCD |
↓ β-CTF, Aβ42, Aβ40 |
Zhang et al. 202063 |
↑ BACE1 LAMP1 in the hippocampus |
↑ Cognitive function |
|
Antioxidant and anti-inflammatory response |
300 ng mL−1–60 μg mL−1 daNGO for 24 hours |
Rats/6-OHDA |
↑ TH+ neurons |
Kim et al. 202365 |
↓ Iba-1 in the SN |
↑ Motor activity |
|
25 mg kg−1 GONPs |
C57BL/6 mice/reserpine |
↑ CAT ↓ MDA level |
Oukhrib et al. 202366 |
↑ Motor activity |
Drug delivery |
Lf-GO-Pue, i.v., 8-days (Pue equivalent dose = 5 mg kg−1) |
C57BL/6 mice/MPTP |
Pue accumulation in the brain |
Xiong et al. 202191 |
↑ TH+ neurons |
↑ DA, DOPAC, HVA, 5-TH |
↓ MDA ↑ GSH |
↑SOD activity in the SN |
↑ Motor activity |
Misfolded protein clearance
Aberrant proteostasis in PD results not only from the loss of control over α-syn misfolding but also from impaired clearance of their inclusions. The autophagy-lysosomal pathway and the ubiquitin-proteasomal system are involved in the proteolytic degradation of α-syn. Pharmacological enhancement of these clearance mechanisms is an attractive strategy to combat α-syn aggregation in PD.60 Significantly, GO can inhibit mTOR signaling by activating AMP-activated protein kinase (AMPK) to induce autophagy of microglia and neurons, thereby promoting the clearance of amyloid beta (Aβ).61 GO administration alleviating Aβ accumulation through upregulation autophagic response in AD mice62 or acceleration of its endosomal delivery to lysosomes in mice with postoperative cognitive dysfunction,63 thus improving the cognitive performance (Table 1).62,63In vitro rGO increased the level of ATG5 and LC3-II protein expression64 (Fig. 4).
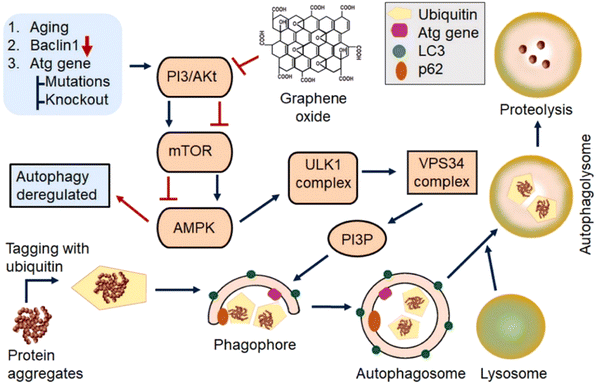 |
| Fig. 4 Role of GBNs in the modulation of autophagy. GBNs inhibit PI3/AKT and mTOR signaling while activating the AMPK pathway triggering the formation of phagophores. AMPK phosphorylates and activates Unc-51-like kinase (ULK1) complex leading to enhanced vacuolar protein sorting 34 (VPS34) activity and subsequent increased production of phosphatidylinositol 3-phosphate (PI3P) and autophagy-related protein (ATG) responsible for the creation of the phagophore. As the phagophore expands by recruiting additional lipids, it engulfs α-syn aggregates recognized via adapter proteins such as p62, which binds ubiquitin and LC3. Once the cargo is engulfed, the autophagosome fuses with the lysosome, accompanied by the digestion of α-syn aggregates. Figure reproduced from reference (Tiwari and Tiwari, 2022)56 with permission from Elsevier, copyright 2023. | |
Anti-inflammatory and antioxidant activity
Inflammation is considered beyond synucleinopathy as a critical factor in PD pathophysiology. Kim et al. demonstrated the neuroprotective effects of deca nano-graphene oxide (daNGO) against the 6-hydroxydopamine (6-OHDA)-induced toxicity in the human neuroblastoma cell line (SH-SY5Y) and 6-OHDA-induced PD rat model. daNGO scavenged 6-OHDA-induced ROS in SH-SY5Y and protected against related neurotoxicity. Accordingly, in Parkinsonian rats, daNGO protected DAergic neurons and alleviated neuroinflammation in the SN which was accompanied by improved motor behavior (Table 1).65 GO treatment has been reported to protect also against neurobehavioral damage and oxidative stress in reserpine-induced PD mice (Table 1).66
Antiviral activity
Epidemiological and basic scientific evidence indicate bacterial or viral infection as a potential risk factor for PD. The biological plausibility for the role of infectious agents is supported by the known neurotropic effects of specific viruses, including severe acute respiratory syndrome coronavirus 2 (SARS-CoV-2), the susceptibility of the SN, inflammatory response, and the promotion of α-syn aggregation.67 Thus, the antiviral strategy is of relevance for PD.68 In this context, the well-known antiviral performance of GBNs69 is favorable. Importantly, GO inhibited the infection of SARS-CoV-2 in VERO cells,70 which can be related to the inactivation of the SARS-CoV-2 on the surface of GO through the decomposition of the viral surface components.71 Moreover ultrasensitive and reusable graphene biosensing platforms has been developed to detect SARS-CoV-2 spike (S1) protein,72 a promising technique for COVID-19 diagnostics.
Biosensing
Since PD is a neurodegenerative disorder with the loss of nigrostriatal DAergic neurons, the DA measurement may be a useful diagnostic tool to target the disease's early stages and optimize DRT. In this context, the graphene-based biosensors for DA detection offer acceptable selectivity and sensitivity in human serum/plasma and urine samples with a LOD ranging from 1 pM to 1.5 M.73 Combining an array of graphene field-effect transistors with a selective DNA aptamer increased DA detection sensitivity significantly with a LOD of up to 1 aM in biological samples.74 5-S-Cysteinyl-dopamine, a metabolite of DA oxidation found in PD patients, was suggested to be also a biomarker detectable by graphene sensors for PD diagnosis.75 Recent studies designed a more complex biosensor that can detect α-syn in real samples towards the early stage diagnosis of PD. Aminabad et al. developed an electrochemical immunosensor based on gold nanoparticle-modified graphene for bioconjugation with a biotinylated antibody (bioreceptor) for the highly sensitive and specific monitoring of the α-syn protein in human plasma.76 Tao et al. created an ultrasensitive poly (D-glucosamine)/gold nanoparticles/multi-walled carbon nanotubes/reduced graphene oxide (PDG/AuNPs/MWCNTs/rGO) modified immunosensor, which can also be used for the selective detection of α-syn in human plasma samples.77 DA and acetylcholine (Ach) play a crucial role in motor control and cognitive performance.78 Since the balance in PD patients shifts toward Ach,79 its level is considered a critical biomarker of the disease.80 Park et al. demonstrated that the polyaniline-grafted graphene-based field-effect transistor biosensor for real-time monitoring of Ach in flow configuration had great potential to be used as a drug screening platform to monitor the efficacy of acetylcholinesterase (AchE) inhibitors also prescribed PD patients.80 However, the potential effect of GBNs on neuro mediators turn should be considered. GO exposure was revealed to decrease AChE activity,81,82 and DA level,81 while Cao et al. reported that exposure to carboxyl graphene oxide resulted in an increase in AchE.83
Soluble oligomeric species of α-syn are considered the primary cause of neurotoxicity with a critical role in PD. Therefore, developing surrogate measurements of their cerebral concentrations in plasma or CSF may facilitate the diagnosis of the disease before irreversible damage to the brain tissue occurs.84 Jang et al. described a simple and sensitive electrochemical sensor to monitor the aggregation of α-syn for early PD diagnosis. The sensor worked on methylene blue labeled aptamer adsorbed on electrochemically rGO enabling the highly sensitive and selective detection of α-syn oligomer based on the voltammetric change. The measurement ability was also demonstrated in human blood serum;48 however, the specificity towards oligomeric forms of α-syn was not supported by relevant characterization of the model analyte. Significantly, FAM-labeled aptamer on the GO nanosheet specifically detected α-syn oligomers with a LOD of 6.3 nM, and it exhibited a good analytical performance in spiked human saliva samples.85
Graphene also offers a promising application in bioelectrical studies. Guo et al. presented a graphene biosensor to sense electrical signaling in N27 cells, which can be used for monitoring cell electrophysiology due to good biocompatibility.42
Graphene-based sensors sensing medicines can be developed for antiparkinsonian therapy optimization. A sensitive photoelectrochemical sensor using conjugated microporous polymer-coated benzothiadiazole-based graphene heterostructures has been demonstrated to detect levodopa with the LOD of 0.0027 μM.86 Entacapone (EN) is a peripherally acting reversible inhibitor of catechol-O-methyltransferase used in combination with levodopa/carbidopa to slow their biotransformation, thus increasing the duration of response to these antiparkinsonian drugs.87 Ahmadi et al. presented a graphene oxide quantum dots (GOQDs)-molecularly imprinted silica fluorescent chemical nano bio/chemical sensor for the selective and sensitive determination of EN in spiked plasma samples and some pharmaceutical formulations in a zebrafish model.88
Drug delivery
Graphene is an ideal candidate for drug delivery because of its large surface area, abundant surface chemistry, specific geometry with double side, and high efficiency loading capacity.89 GBNs can be loaded with therapeutical agents to achieve highly specific disease site targeting and controlled drug release in the brain.90 Puerarin (Pue), a natural isoflavone extracted from Pueraria lobata exhibiting anti-PD properties due to low water solubility and poor pharmacokinetic properties, including inadequate bioavailability and incomplete penetration of BBB, has limited use for treatment. Xiong et al. utilized Pue-loaded lactoferrin-conjugated GO to achieve stable and sustained release of the phytochemical in the brain. This GO-based nanoplatform effectively limited neuronal damage accompanied by neurobehavioral improvements and antioxidant effects with no noticeable side effects and toxicity in 1-methyl-4-phenyl-1,2,3,6-tetrahydropyridine (MPTP)-induced PD mice (Table 1).91
Gene and cell therapies
GBNs are also promising candidates for wound dressing applications.92 Due to their regenerative properties, GBNs also offer the potential for PD. Rodriguez-Losada et al. tested different GO forms and found that partially reduced GO film efficiently promoted differentiation, maturation, and survival of DAergic cells without adversely affecting cellular metabolism or mitochondrial function. Moreover, a decreased α-syn level has been observed in DAergic neurons exposed to rotenone-induced oxidative stress. Thus, the authors suggest that this material is a suitable scaffold for developing constructs for PD cell replacement therapy.36
Compared to symptomatic treatment, with DRT as the gold standard, gene and cell therapies have the potential to provide more tuned solutions to a complex problem by restoring neural signaling and, in some cases, even circuits in the diseased brain.93 However, cell-based therapies behind ethical and conceptual challenges face technical ones related to poor survival and differentiation rates of transplanted stem cells, inefficient homing ability, and difficulties in post-transplant follow-up, which limit their clinical use.94 In this context, biomaterials, including GBNs, offer the application for the controlled and site-specific delivery of neurotrophins, increased engraftment of implanted neural stem cells, and the redirection of endogenous stem cell populations away from their niche to promote repair mechanisms.95,96
The biomimetic graphene scaffold mimicking the extracellular matrix can promote cell adhesion, proliferation, and differentiation, enabling optimal regenerative tissue processes. Moreover, the high homogeneity and conductivity of the GBNs layers offer the potential for their use in electrostimulation neural stem cells. Dybowska-Sarapuk et al. demonstrated that using GBNs as cellular scaffolds to electrostimulate mouse NE-4C neural stem cells promoted their suitable development.97 Fang et al. focused on the metabolomics of neural stem cells (NCSs) induced by culturing on 3DG foam. The authors established a correlation between metabolic reconfiguration and the proliferation rate of NSCs on a different scaffold. Culturing on 3DG modulates pathways affected in PD patients, resulting in increased amino acid incorporation and enhanced glucose metabolism, likely the driving force for faster NSC growth.17 Moreover, collagen coating of the 3DG foam was demonstrated to promote the differentiation of mouse MSCs into DA neurons.98 By targeting the pathological mechanisms contributing to neurodegeneration, regenerative properties, and the capability to be used as carriers, biosensors, and imaging agents, GBNs from green processes offer a broad spectrum of diversified modalities for PD management (Fig. 5).
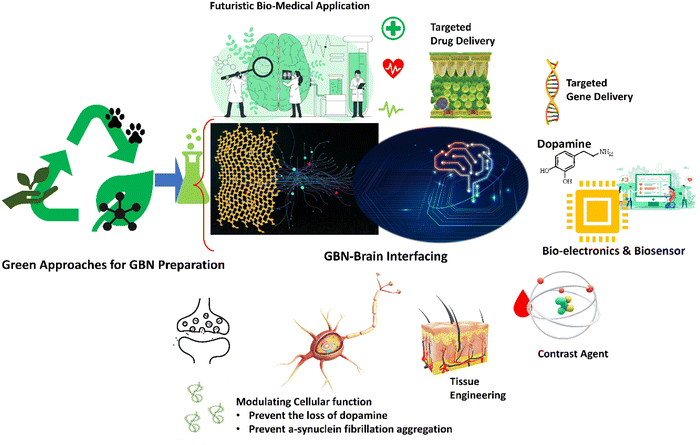 |
| Fig. 5 The significant potential of GBNs for PD management. | |
Limitations of graphene-based nanostructures
Despite the wide application of GBNs in various fields of biotechnology, they present some risks to humans. Research has revealed that the very properties responsible for the beneficial activity of GBNs can also contribute to their adverse effects. Therefore, a comprehensive understanding of the growing interest in graphene materials raises questions about their short- and long-term cytotoxicity. Numerous studies demonstrated cytotoxicity in various cell types99 and organ distribution with lung toxicity in animals.100 However, the neurotoxicity of GNPs is a subject of debate. In general, the neurotoxicity of GBNs stems from the generation of reactive oxygen species and related oxidative stress.101 Ren et al. reported that GO exposure induced PD-like symptoms in zebrafish, which showed a loss of DAergic neurons and an increase in LBs accompanied by impaired locomotor activity.102 On the other hand, GQDs inhibited 1-methyl-4-phenyl-pyridinium ion (MPP+)-induced oxidative stress and related apoptosis and α-syn accumulation in PC12 cells. Additionally, pretreating of MPP+-challenged zebrafish with GQDs protected against excessive ROS generation and related apoptosis, mitochondrial impairment as well as decreased senescent cells. Moreover, a beneficial shift in metabolic pathways contributed to antioxidant response and neurotransmission was observed.103 These opposite effects of GBNs can be explained based on their different physical interaction with cells depending on factors such as synthetic methods, crystallinity, surface functionality, size, and shape.56 Thus, studies on brain injury or neurotoxicity of GBNs deserve more attention. As outlined in the literature review, several mechanisms such as physical destruction, oxidative stress, DNA damage, inflammatory response, apoptosis, autophagy, and necrosis underly GBNs toxicity. Toll-like receptors, transforming growth factor, and tumor necrosis factor alpha-dependent signaling pathways are considered to contribute to these mechanisms.104
Considerable attention, however, must be paid when the cytotoxic effect is observed and measured as an increase in cell apoptosis in cancer cell lines. There is a debate surrounding the controversy related to the proliferative ratio vs. differentiation mediated by apoptosis, where GBNs, according to Rodriguez-Losada and Aguirre,105 promote differentiation, decreasing proliferation. The apoptotic process induced by GBNs could be misinterpreted as cytotoxic rather than differentiation-promoting.105 This idea is supported by earlier studies showing that GBNs promoted the differentiation of normal stem cells towards multiple cell lineages, including neurons, chondrocytes, or adipocytes, with non-toxic effects106–108 while selectively inhibiting the proliferative expansion of cancer stem cells109via arresting the cell cycle at the G0/G1 phase.110 A recent review summarized examples of selective GBNs mediated apoptosis in tumoral and cancer cells.111 Regarding significance in PD, in the model of α-syn overexpression in human dopaminergic-like glioblastomas cells (SH-SY5Y) showing elevated cell proliferation and cell toxicity, rGO-induced apoptosis observed only in cells with moderate α-syn level (low-aSyn clone) was interpreted as a protective activity. At the same time, the preventive effect of rGO seemed ineffective at a high α-syn overexpression conferred to the carcinogenic neurons heightening tumorigenicity and resistance to senescence compared to low-αSyn cells. Accordingly, the authors pointed to an inadequate level of αSyn stimulation, rather than the αSyn overload itself, as one of the factors contributing to α-synucleinopathy.112
Summary and future perspectives
Nanotechnology is having a broader application in biomedical fields such as gene transmission, cell imaging and cancer therapy. A new direction of its application is the development of a treatment for neurodegenerative diseases. An important aspect of this is the design of effective and biodegradable nanomaterials that prevent the formation of oligomers and/or degrade already existing aggregates. Although literature reports support that selected nanostructures can inhibit aggregation of some protein monomers into fibrillar structures at the same time, research shows that nanoparticles can increase the rate of aggregation and fibril formation. Significantly, nanoparticles are a double sword, and depending on the coating, shape, size, surface load, and concentration, they can either promote or inhibit protein aggregation.113
A recent literature review on this topic demonstrated evidence that GBNs effectively inhibit amyloid aggregation. This effect mainly tends to arrest nucleation in the early stages and drive the conversion of preformed insoluble fibrils into soluble peptides that are later pushed across the BBB by efflux pumps, thus preventing neurodegeneration.56 Moreover, since there is strong evidence of a link between SARS-COV-2 infection and PD,67 GBNs such as GO or GQDs, beyond being inhibitors of α-syn aggregation due to antiviral activity, offer unique properties to combat PD.114 The neuroprotection of GBNs also derives from control over protein misfolding attributed to enhanced clearance of aggregated proteins discussed above. Apart from neuroprotection, PD can be managed through the restorative ability of GBNs. Graphene and GBNs are the most promising biomaterials for regenerating the nervous system. Graphene materials significantly advance tissue engineering research and scaffolds for neural tissue with their unique mechanical, electrical, and optical properties.36,97 Graphene nanosheets with the ability to modulate electrophysiology are being intensively studied as materials for the next generation of neural interfaces. Accordingly, GBNs are used to fabricate electro stimulated scaffolds for the suitable development of stem cells and advanced implants, respectively, for replacement- and implant-based therapies for treating PD.17,23,97
Gene therapy holds great promise and opportunities for PD. GBNs can be developed into transporters to deliver oligonucleotides for gene detection and therapy with the ability to protect oligonucleotides from cleavage and deliver oligonucleotides into cells.38 Moreover, graphene-based nanobiosensors enable early detection of PD based on the quantification of circulating miR-195.18 Benefiting from GBNs special properties, many efforts have been directed at developing new DNA biosensors to sequence the nucleic acid base and recognize the specific DNA115 that offers tremendous potential in PD. Graphene-based nanocarriers are applied to deliver high-molecular-weight Cas9/single-guide RNA (sgRNA) complexes for gene editing as a new approach for biomedical research and targeted gene engineering applications.116 Clustered regularly interspaced short palindromic repeats (CRISPR)-Cas9 and GBNs may offer significant potential to be applied to improve our understanding of PD and create successful future treatments targeting the proper genes.9
These features favor the development of GBNs-based multifunctional systems into customized nanomedicine applications. The anti-amyloid and regenerative properties of graphene material can be combined with delivery function in disease-modifying approaches and gene or DRT.90 Moreover, theragnostic strategies that combine diagnostic and therapeutical modalities in a single platform are projected for PD management. Developing intelligent sensor systems integrated into the Internet of Things, 5-generation communications, artificial intelligence, and machine learning strategies has revolutionized their diversified biomedical applications.116,117 Recently, the integration and linking of quantum sensing, signaling, and optimized biological agents have led to the investigation of minute biological events with anomalous sensitivity. Such technologies are anticipated to accelerate the tailored management of neurodegenerative diseases.118
However, some research limitations should be acknowledged, such as a low number of studies in vivo, which are presented in Table 1. This indicates a very early stage of development of technologies presented herein. Therefore, the challenges facing their further advancement and potential translational discordance should be well identified and addressed in future studies to enable them to be dynamically aligned in creating innovative solutions with broad scientific feedback.
Conclusions
GBNs offer many advantages in various fields due to their excellent properties. Recent studies have proven that GBNs acquire great potential in biomedical applications. However, some issues such as their side effects and toxicity are still being debated and should be well understood before considering their development in this area. Significantly, biocompatibility improves with precise modifications. Thus, looking ahead GNPs toxicity needs to be addressed during the tailoring functionality at the development phase. Cytotoxicity-related tests of GBNs are confined to in vitro studies. Hence, it is essential to enhance their safety assessment in in vivo models. Before clinical trials, comprehensive knowledge of the methods for characterizing GBNs should be provided and tested.
Here, we discussed the strengths alongside limitations of GBNs' technologies in PD management. We emphasized GBNs and their relevance to therapeutic, diagnostic, and research applications. A mechanistic understanding of the interactions of GBNs with biological systems will enable the development of novel, safe strategies for treating, preventing, and diagnosing PD. These technologies so far are limited to computational and in vitro experimental studies, which we have overviewed here. Therefore future in vivo studies are required to assess the translational value of the graphene-based approaches in PD management. As PD patients are quite heterogeneous in terms of disease onset, progression, symptoms, and neuropathology, diversification of animal models may address approaching the phenotype observed in humans. Taking into account the complexity and heterogeneity of PD, the overviewed herein multimodality of GBNs can develop toward personalized, combinatorial, and multi-targeted approaches to combat PD. Based on the gathered data, we suggest some prospects, further developments and opportunities in this emerging and promising technology.
Author contributions
Contribution: Oz T and Kujawska M designed the review; Kaushik A – art work. All authors contributed to the writing of the paper and artwork and gave approval of the final version.
Abbreviations
0DG | Fullerene |
2DG | Two-dimensional graphene |
3DG | Three-dimensional graphene |
6-OHDA | 6-Hydroxydopamine |
Ach | Acetylcholine |
AchE | Acetylcholinesterase |
Aβ | Amyloid beta |
AMPK | AMP-activated protein kinase |
BBB | Blood–brain barrier |
Ca2+ | Calcium |
CNS | Central nervous system |
CNTs | Carbon nanotubes |
CR-GO | Chemically reduced graphene oxide |
CRISPR | Clustered regularly interspaced short palindromic repeats |
DA | Dopamine |
DAergic | Dopaminergic |
daNGO | deca Nano-graphene oxide |
DRT | Dopamine replacement therapy |
EN | Entacapone |
ERGO | Electrochemically reduced graphene oxide |
GBNs | Graphene-based nanomaterials |
GO | Graphene oxide |
GOQDs | Graphene oxide quantum dots |
GQD | Graphene quantum dots |
LBs | Lewy bodies |
MABM | Methyl N-allyl N-benzoylmethioninate |
MD | Molecular dynamics |
MPP+ | 1-Methyl-4-phenyl-pyridinium ion |
MPTP | 1-Methyl-4-phenyl-1,2,3,6-tetrahydropyridine |
NCSs | Neural stem cells |
PD | Parkinson's disease |
PFFs | Preformed fibrils |
PDG/AuNPs/MWCNTs/rGO | Poly(D-glucosamine)/gold nanoparticles/multi-walled carbon nanotubes/reduced graphene oxide |
Pue | Puerarin |
rGO | Reduced GO |
ROS | Reactive oxygen species |
SARS-CoV-2 | Severe acute respiratory syndrome coronavirus 2 |
s-GR | Sulfonated graphene |
sgRNA | Single-guide RNA |
SH-SY5Y | Human neuroblastoma cell line |
Si-CNTs | Silicon carbon nanotubes |
SN | Substantia nigra |
α-syn | α-Synuclein |
Conflicts of interest
There is no conflict of interest.
Acknowledgements
The National Science Centre, No. 2021/42/E/NZ7/00246.
References
- D. Kim, J. M. Yoo, H. Hwang, J. Lee, S. H. Lee, S. P. Yun, M. J. Park, M. Lee, S. Choi, S. H. Kwon, S. Lee, S. Kim, Y. J. Park, M. Kinoshita, Y. H. Lee, S. Shin, S. R. Paik, S. J. Lee, B. H. Hong and H. S. Ko, Nat. Nanotechnol., 2018, 13, 812–818 CrossRef CAS PubMed
.
- K. Jagaran and M. Singh, Int. J. Mol. Sci., 2022, 23, 9361 CrossRef CAS PubMed
.
- D. Arango, A. Bittar, N. P. Esmeral, C. Ocasión, C. Muñoz-Camargo, J. C. Cruz, L. H. Reyes and N. I. Bloch, Int. J. Mol. Sci., 2021, 22, 9241 CrossRef CAS PubMed
.
- Z. Tuncer, G. Dereli Can, H. Dönmez Keklikoğlu, F. A. Eren, F. Yülek and O. Deniz, Parkinsons Dis., 2023, 2023, 7739944 Search PubMed
.
- H. Chen, K. Wang, F. Scheperjans and B. Killinger, Neurobiol. Dis., 2022, 163, 105601 CrossRef CAS PubMed
.
- D. M. Wilson, 3rd, M. R. Cookson, L. Van Den Bosch, H. Zetterberg, D. M. Holtzman and I. Dewachter, Cell, 2023, 186, 693–714 CrossRef PubMed
.
- A. Haider, N. H. Elghazawy, A. Dawoud, C. Gebhard, T. Wichmann, W. Sippl, M. Hoener, E. Arenas and S. H. Liang, Mol. Neurodegener., 2023, 18, 11 CrossRef CAS PubMed
.
- L. M. A. Oliveira, T. Gasser, R. Edwards, M. Zweckstetter, R. Melki, L. Stefanis, H. A. Lashuel, D. Sulzer, K. Vekrellis, G. M. Halliday, J. J. Tomlinson, M. Schlossmacher, P. H. Jensen, J. Schulze-Hentrich, O. Riess, W. D. Hirst, O. El-Agnaf, B. Mollenhauer, P. Lansbury and T. F. Outeiro, NPJ Parkinsons Dis., 2021, 7, 65 CrossRef PubMed
.
- M. U. Rahman, M. Bilal, J. A. Shah, A. Kaushik, P. L. Teissedre and M. Kujawska, Pharmaceutics, 2022, 14, 1252 CrossRef CAS PubMed
.
- F. Haddad, M. Sawalha, Y. Khawaja, A. Najjar and R. Karaman, Molecules, 2017, 23, 40 CrossRef PubMed
.
- W. Agnieszka, P. Paweł and K. Małgorzata, Curr. Neuropharmacol., 2022, 20, 1427–1447 CrossRef CAS PubMed
.
-
N. Palaniappan, M. Kujawska and K. Poturcu, Functionalized Carbon Nanotubes for Biomedical Applications, 2023, pp. 117–137 DOI:10.1002/9781119905080.ch6
.
- S. N. Serva, J. Bernstein, J. A. Thompson, D. S. Kern and S. G. Ojemann, Front. Surg., 2022, 9, 863921 CrossRef PubMed
.
- M. Kujawska and A. Kaushik, Neural Regener. Res., 2023, 18, 129–130 CrossRef PubMed
.
- G. Pagano, K. I. Taylor, J. Anzures-Cabrera, M. Marchesi, T. Simuni, K. Marek, R. B. Postuma, N. Pavese, F. Stocchi, J.-P. Azulay, B. Mollenhauer, L. López-Manzanares, D. S. Russell, J. T. Boyd, A. P. Nicholas, M. R. Luquin, R. A. Hauser, T. Gasser, W. Poewe, B. Ricci, A. Boulay, A. Vogt, F. G. Boess, J. Dukart, G. D’Urso, R. Finch, S. Zanigni, A. Monnet, N. Pross, A. Hahn, H. Svoboda, M. Britschgi, F. Lipsmeier, E. Volkova-Volkmar, M. Lindemann, S. Dziadek, Š. Holiga, D. Rukina, T. Kustermann, G. A. Kerchner, P. Fontoura, D. Umbricht, R. Doody, T. Nikolcheva and A. Bonni, N. Engl. J. Med., 2022, 387, 421–432 CrossRef CAS PubMed
.
- A. Bhosale, G. Paul, F. Mazahir and A. K. Yadav, OpenNano, 2023, 9, 100111 CrossRef
.
- Q. Fang, Y. Zhang, X. Chen, H. Li, L. Cheng, W. Zhu, Z. Zhang, M. Tang, W. Liu, H. Wang, T. Wang, T. Shen and R. Chai, Front. Bioeng. Biotechnol., 2019, 7, 436 CrossRef PubMed
.
- Z. Aghili, N. Nasirizadeh, A. Divsalar, S. Shoeibi and P. Yaghmaei, Artif. Cells, Nanomed., Biotechnol., 2018, 46, 32–40 CrossRef CAS PubMed
.
- B. Mekuye and B. Abera, Nano Select, 2023, 4, 486–501 CrossRef CAS
.
- A. A. Yetisgin, S. Cetinel, M. Zuvin, A. Kosar and O. Kutlu, Molecules, 2020, 25, 2193 CrossRef CAS PubMed
.
- K. S. Novoselov, A. K. Geim, S. V. Morozov, D. Jiang, Y. Zhang, S. V. Dubonos, I. V. Grigorieva and A. A. Firsov, Science, 2004, 306, 666–669 CrossRef CAS PubMed
.
- N. Lu, L. Wang, M. Lv, Z. Tang and C. Fan, Nano Res., 2019, 12, 247–264 CrossRef CAS PubMed
.
- R. Fabbri, E. Saracino, E. Treossi, R. Zamboni, V. Palermo and V. Benfenati, Nanoscale, 2021, 13, 4390–4407 RSC
.
- Y. Yang, A. M. Asiri, Z. Tang, D. Du and Y. Lin, Mater. Today, 2013, 16, 365–373 CrossRef CAS
.
- W. Qiao, W. Wei, W. Huo, L. Wang, J. Zhang, S. Meng, J. Di, H. Bai and M. Li, Diamond Relat. Mater., 2023, 131, 109571 CrossRef CAS
.
- M. Z. H. Khan, J. Nanomater., 2017, 2017, 1–11 Search PubMed
.
- V. Georgakilas, M. Otyepka, A. B. Bourlinos, V. Chandra, N. Kim, K. C. Kemp, P. Hobza, R. Zboril and K. S. Kim, Chem. Rev., 2012, 112, 6156–6214 CrossRef CAS PubMed
.
- V. Chaudhary, N. Ashraf, M. Khalid, R. Walvekar, Y. Yang, A. Kaushik and Y. K. Mishra, Adv. Funct. Mater., 2022, 32, 2112913 CrossRef CAS
.
- T. R. Kyriakides, A. Raj, T. H. Tseng, H. Xiao, R. Nguyen, F. S. Mohammed, S. Halder, M. Xu, M. J. Wu, S. Bao and W. C. Sheu, Biomed. Mater., 2021, 16 DOI:10.1088/1748-605X/abe5fa
.
- J. Li, H. Zeng, Z. Zeng, Y. Zeng and T. Xie, ACS Biomater. Sci. Eng., 2021, 7, 5363–5396 CrossRef CAS PubMed
.
- E. Papanikolaou, Y. V. Simos, K. Spyrou, M. Patila, C. Alatzoglou, K. Tsamis, P. Vezyraki, H. Stamatis, D. P. Gournis, D. Peschos and E. Dounousi, Pharmaceutics, 2023, 15, 993 CrossRef CAS PubMed
.
- C. Liao, Y. Li and S. C. Tjong, Int. J. Mol. Sci., 2018, 19, 3564 CrossRef PubMed
.
- S. F. Kiew, L. V. Kiew, H. B. Lee, T. Imae and L. Y. Chung, J. Controlled Release, 2016, 226, 217–228 CrossRef CAS PubMed
.
- V. Palmieri and G. Caracciolo, Nanoscale Adv., 2022, 4, 3300–3308 RSC
.
- G. Lalwani, M. D'Agati, A. M. Khan and B. Sitharaman, Adv. Drug Delivery Rev., 2016, 105, 109–144 CrossRef CAS PubMed
.
- N. Rodriguez-Losada, R. Wendelbob, M. C. Ocaña, A. D. Casares, R. Guzman de Villoría, J. A. Aguirre Gomez, M. A. Arraez, P. Gonzalez-Alegre, M. A. Medina, E. Arenas and J. A. Narvaez, Front. Neurosci., 2020, 14, 570409 CrossRef PubMed
.
- S. K. Bhardwaj, M. Mujawar, Y. K. Mishra, N. Hickman, M. Chavali and A. Kaushik, Nanotechnology, 2021, 32, 50 CrossRef PubMed
.
- C. H. Lu, C. L. Zhu, J. Li, J. J. Liu, X. Chen and H. H. Yang, Chem. Commun., 2010, 46, 3116–3118 RSC
.
- M. Hoseini-Ghahfarokhi, S. Mirkiani, N. Mozaffari, M. A. Abdolahi Sadatlu, A. Ghasemi, S. Abbaspour, M. Akbarian, F. Farjadian and M. Karimi, Int. J. Nanomed., 2020, 15, 9469–9496 CrossRef CAS PubMed
.
- A. Kaushik, J. Rodriguez, D. Rothen, V. Bhardwaj, R. D. Jayant, P. Pattany, B. Fuentes, H. Chand, N. Kolishetti, N. El-Hage, K. Khalili, N. S. Kenyon and M. Nair, ACS Appl. Bio Mater., 2019, 2, 4826–4836 CrossRef CAS PubMed
.
- B. Bakhshandeh, P. Zarrintaj, M. O. Oftadeh, F. Keramati, H. Fouladiha, S. Sohrabi-Jahromi and Z. Ziraksaz, Biotechnol. Genetic Eng. Rev., 2017, 33, 144–172 CrossRef CAS PubMed
.
- J. Guo, A. E. Niaraki Asli, K. R. Williams, P. L. Lai, X. Wang, R. Montazami and N. N. Hashemi, Biosensors, 2019, 9, 112 CrossRef CAS PubMed
.
- M. Hashemi, M. Omidi, B. Muralidharan, H. Smyth, M. A. Mohagheghi, J. Mohammadi and T. E. Milner, ACS Appl. Mater. Interfaces, 2017, 9, 32607–32620 CrossRef CAS PubMed
.
- M.-Y. Xia, Y. Xie, C.-H. Yu, G.-Y. Chen, Y.-H. Li, T. Zhang and Q. Peng, J. Controlled Release, 2019, 307, 16–31 CrossRef CAS PubMed
.
- A. J. Espay and M. S. Okun, JAMA Neurol., 2023, 80, 123–124 CrossRef PubMed
.
- F. Longhena, G. Faustini, M. G. Spillantini and A. Bellucci, Int. J. Mol. Sci., 2019, 20, 141 CrossRef PubMed
.
- L. M. A. Oliveira, T. Gasser, R. Edwards, M. Zweckstetter, R. Melki, L. Stefanis, H. A. Lashuel, D. Sulzer, K. Vekrellis, G. M. Halliday, J. J. Tomlinson, M. Schlossmacher, P. H. Jensen, J. Schulze-Hentrich, O. Riess, W. D. Hirst, O. El-Agnaf, B. Mollenhauer, P. Lansbury and T. F. Outeiro, npj Parkinson’s Disease, 2021, 7, 65 CrossRef PubMed
.
- S. J. Jang, C. S. Lee and T. H. Kim, Nanomaterials, 2020, 10, 832 CrossRef CAS PubMed
.
- E. Alimohammadi, M. Khedri, A. Miri Jahromi, R. Maleki and M. Rezaian, Int. J. Nanomed., 2020, 15, 6887–6903 CrossRef CAS PubMed
.
- M. Ghaeidamini, D. Bernson, N. Sasanian, R. Kumar and E. K. Esbjörner, Nanoscale, 2020, 12, 19450–19460 RSC
.
- E. Alimohammadi, A. Nikzad, M. Khedri, S. Shafiee, A. Miri Jahromi, R. Maleki and N. Rezaei, ACS Appl. Bio Mater., 2021, 4, 6073–6083 CrossRef CAS PubMed
.
- H. Mohammad-Beigi, A. Hosseini, M. Adeli, M. R. Ejtehadi, G. Christiansen, C. Sahin, Z. Tu, M. Tavakol, A. Dilmaghani-Marand, I. Nabipour, F. Farzadfar, D. E. Otzen, M. Mahmoudi and M. J. Hajipour, ACS Nano, 2019, 13, 3243–3256 CrossRef CAS PubMed
.
- Z. He and R. Zhou, Nanoscale, 2020, 12, 13822–13828 RSC
.
- J. Ahlawat and M. Narayan, ACS Sustainable Chem. Eng., 2022, 10, 4610–4622 CrossRef CAS
.
- W. E. Gregory, B. Sharma, L. Hu, A. J. Raghavendra and R. Podila, Biointerphases, 2020, 15, 031010 CrossRef CAS PubMed
.
- P. Tiwari and S. Tiwari, Adv. Colloid Interface Sci., 2023, 311, 102824 CrossRef CAS PubMed
.
- S. Mohammadi, M. Nikkhah and S. Hosseinkhani, Int. J. Nanomed., 2017, 12, 8831–8840 CrossRef CAS PubMed
.
- D. Ysselstein and D. Krainc, Mov. Disord, 2018, 33, 1673 CrossRef PubMed
.
- P. Kaliyaperumal, S. Renganathan, K. Arumugam and B. R. Aremu, Nanomedicine, 2023, 47, 102608 CrossRef CAS PubMed
.
- T. E. Moors, J. J. M. Hoozemans, A. Ingrassia, T. Beccari, L. Parnetti, M.-C. Chartier-Harlin and W. D. J. Van De Berg, Mol. Neurodegener., 2017, 12, 423–448 CrossRef PubMed
.
- X. Li, K. Li, F. Chu, J. Huang and Z. Yang, Chem. Biol. Interact., 2020, 325, 109126 CrossRef CAS PubMed
.
- F. Chu, K. Li, X. Li, L. Xu, J. Huang and Z. Yang, Neurochem. Res., 2021, 46, 309–325 CrossRef CAS PubMed
.
- J. Zhang, S. Zhu, P. Jin, Y. Huang, Q. Dai, Q. Zhu, P. Wei, Z. Yang, L. Zhang, H. Liu, G. Xu, L. Chen, E. Gu, Y. Zhang, L. Wen and X. Liu, Theranostics, 2020, 10, 11908–11920 CrossRef CAS PubMed
.
- R. Krętowski and M. Cechowska-Pasko, Int. J. Mol. Sci., 2022, 23, 9285 CrossRef PubMed
.
- H.-Y. Kim, H. H. Yoon, H. Seong, D. K. Seo, S. W. Choi, J. Ryu, K.-S. Kang and S. R. Jeon, BMB Rep., 2023, 56, 202–207 CrossRef CAS PubMed
.
- M. Oukhrib, L. Tamegart, A. Assafi, L. Hejji, A. Azzouz, L. P. Villarejo, M. Haida, C. Mohamed and H. Gamrani, Food Chem. Toxicol., 2023, 178, 113904 CrossRef CAS PubMed
.
- H. M. Al-Kuraishy, A. I. Al-Gareeb, A. Kaushik, M. Kujawska, E. A. Ahmed and G. E. Batiha, J. Neurosci. Res., 2023, 101, 952–975 CrossRef CAS PubMed
.
- E. J. R. Fletcher, T. Kaminski, G. Williams and S. Duty, Pharmacol. Res. Perspect., 2021, 9, e00841 CrossRef PubMed
.
- S. Gungordu Er, M. Edirisinghe and T. A. Tabish, Adv. Healthcare Mater., 2023, 12, 2201523 CrossRef CAS PubMed
.
- F. De Maio, V. Palmieri, G. Babini, A. Augello, I. Palucci, G. Perini, A. Salustri, P. Spilman, M. De Spirito, M. Sanguinetti, G. Delogu, L. G. Rizzi, G. Cesareo, P. Soon-Shiong, M. Sali and M. Papi, iScience, 2021, 24, 102788 CrossRef CAS PubMed
.
- M. A. Unal, F. Bayrakdar, H. Nazir, O. Besbinar, C. Gurcan, N. Lozano, L. M. Arellano, S. Yalcin, O. Panatli, D. Celik, D. Alkaya, A. Agan, L. Fusco, S. Suzuk Yildiz, L. G. Delogu, K. C. Akcali, K. Kostarelos and A. Yilmazer, Small, 2021, 17, 2101483 CrossRef CAS PubMed
.
- P. K. Sharma, E. S. Kim, S. Mishra, E. Ganbold, R. S. Seong, A. K. Kaushik and N. Y. Kim, ACS Sens., 2021, 6, 3468–3476 CrossRef CAS PubMed
.
- M. Kujawska, S. K. Bhardwaj, Y. K. Mishra and A. Kaushik, Biosensors, 2021, 11, 433 CrossRef CAS PubMed
.
- M. Abrantes, D. Rodrigues, T. Domingues, S. S. Nemala, P. Monteiro, J. Borme, P. Alpuim and L. Jacinto, J. Nanobiotechnol., 2022, 20, 495 CrossRef CAS PubMed
.
- S.-J. Cheng, H.-Y. Chiu, P. V. Kumar, K. Y. Hsieh, J.-W. Yang, Y.-R. Lin, Y.-C. Shen and G.-Y. Chen, Biomater. Sci., 2018, 6, 813–819 RSC
.
- E. D. Aminabad, A. Mobed, M. Hasanzadeh, M. A. Hosseinpour Feizi, R. Safaralizadeh and F. Seidi, RSC Adv., 2022, 12, 4346–4357 RSC
.
- D. Tao, Y. Gu, S. Song, E. P. Nguyen, J. Cheng, Q. Yuan, H. Pan, N. Jaffrezic-Renault and Z. Guo, Microchem. J., 2020, 158, 105195 CrossRef CAS
.
- M. Kujawska, M. Jourdes, L. Witucki, M. Karazniewicz-Lada, M. Szulc, A. Gorska, P. L. Mikolajczak, P. L. Teissedre and J. Jodynis-Liebert, Brain Sci., 2021, 11, 1127 CrossRef CAS PubMed
.
- C. Liu, Acta Pharmacol. Sin., 2020, 41, 453–463 CrossRef CAS PubMed
.
- D. Park, D. Lee, H. J. Kim, D. S. Yoon and K. S. Hwang, Biosensors, 2022, 12, 279 CrossRef CAS PubMed
.
- J. C. Soares, T. C. B. Pereira, K. M. Costa, T. Maraschin, N. R. Basso and M. R. Bogo, Colloids Surf., B, 2017, 157, 335–346 CrossRef CAS PubMed
.
- Z. Clemente, G. H. Silva, M. C. De Souza Nunes, D. S. T. Martinez, C. V. Maurer-Morelli, A. A. Thomaz and V. L. S. S. Castro, Environ. Sci. Pollut. Res., 2019, 26, 30508–30523 CrossRef CAS PubMed
.
- Z. Cao, M. Su, H. Wang, L. Zhou, Z. Meng, G. Xiong, X. Liao and H. Lu, Chemosphere, 2021, 270, 128611 CrossRef CAS PubMed
.
- L. V. Kalia, S. K. Kalia, P. J. McLean, A. M. Lozano and A. E. Lang, Ann. Neurol., 2013, 73, 155–169 CrossRef CAS PubMed
.
- Z. Saedi and M. Nikkhah, Anal. Methods, 2022, 14, 2937–2944 RSC
.
- G. Xiang, X. He, Y. Liu, Q. Huang, W. Huang, C. Zhang and J. Peng, ACS Appl. Mater. Interfaces, 2022, 14, 51329–51340 CrossRef CAS PubMed
.
-
Ann. Neurol., 1997, 42, 747–755 DOI:10.1002/ana.410420511.
- H. Ahmadi, F. Faridbod and M. Mehrzad-Samarin, Anal. Bioanal. Chem., 2019, 411, 1075–1084 CrossRef CAS PubMed
.
- H. Ding, F. Zhang, C. Zhao, Y. Lv, G. Ma, W. Wei and Z. Tian, ACS Appl. Mater. Interfaces, 2017, 9, 27396–27401 CrossRef CAS PubMed
.
- G. Cellot, A. Franceschi Biagioni and L. Ballerini, Pediatr. Res., 2022, 92, 71–79 CrossRef PubMed
.
- S. Xiong, J. Luo, Q. Wang, Z. Li, J. Li, Q. Liu, L. Gao, S. Fang, Y. Li, H. Pan, H. Wang, Y. Zhang, X. Chen and T. Chen, Biomater. Sci., 2021, 9, 1705–1715 RSC
.
- Y. Zhou, R. Chen, T. He, K. Xu, D. Du, N. Zhao, X. Cheng, J. Yang, H. Shi and Y. Lin, ACS Appl. Mater. Interfaces, 2016, 8, 15067–15075 CrossRef CAS PubMed
.
- M. Tomishima and A. Kirkeby, J. Parkinson's Dis., 2021, 11, S135–S140 Search PubMed
.
- P. A. Barbuti, R. A. Barker, P. Brundin, S. Przedborski, S. M. Papa, L. V. Kalia, H. Mochizuki and M. D. S. S. I. Committee, Mov. Disord, 2021, 36, 1772–1780 CrossRef PubMed
.
- S. Mirzaei, K. Kulkarni, K. Zhou, P. J. Crack, M. I. Aguilar, D. I. Finkelstein and J. S. Forsythe, ACS Chem. Neurosci., 2021, 12, 4224–4235 CrossRef CAS PubMed
.
- T. Oz, A. Kaushik and M. Kujawska, World J. Stem Cells, 2023, 15, 687–700 CrossRef PubMed
.
- Ł. Dybowska-Sarapuk, W. Sosnowicz, A. Grzeczkowicz, J. Krzemiński and M. Jakubowska, Front. Mol. Neurosci., 2022, 15, 992494 CrossRef PubMed
.
- N. Tasnim, V. Thakur, M. Chattopadhyay and B. Joddar, Stem Cells Int., 2018, 2018, 3410168 Search PubMed
.
- Y. Li, Y. Liu, Y. Fu, T. Wei, L. Le Guyader, G. Gao, R. S. Liu, Y. Z. Chang and C. Chen, Biomaterials, 2012, 33, 402–411 CrossRef CAS PubMed
.
- B. Li, J. Yang, Q. Huang, Y. Zhang, C. Peng, Y. Zhang, Y. He, J. Shi, W. Li, J. Hu and C. Fan, NPG Asia Mater., 2013, 5, e44 CrossRef CAS
.
- D. Teleanu, C. Chircov, A. Grumezescu, A. Volceanov and R. Teleanu, J. Clin. Med., 2018, 7, 490 CrossRef PubMed
.
- C. Ren, X. Hu, X. Li and Q. Zhou, Biomaterials, 2016, 93, 83–94 CrossRef CAS PubMed
.
- C. Ren, X. Hu and Q. Zhou, Adv. Sci., 2018, 5, 1700595 CrossRef PubMed
.
- L. Ou, B. Song, H. Liang, J. Liu, X. Feng, B. Deng, T. Sun and L. Shao, Part. Fibre Toxicol., 2016, 13, 57 CrossRef PubMed
.
- N. Rodriguez-Losada and J. A. Aguirre, Neural Regener. Res., 2017, 12, 1071–1072 CrossRef PubMed
.
- H. H. Yoon, S. H. Bhang, T. Kim, T. Yu, T. Hyeon and B.-S. Kim, Adv. Funct. Mater., 2014, 24, 6455–6464 CrossRef CAS
.
- D. Yang, T. Li, M. Xu, F. Gao, J. Yang, Z. Yang and W. Le, Nanomedicine, 2014, 9, 2445–2455 CrossRef CAS PubMed
.
- W. C. Lee, C. H. Y. X. Lim, H. Shi, L. A. L. Tang, Y. Wang, C. T. Lim and K. P. Loh, ACS Nano, 2011, 5, 7334–7341 CrossRef CAS PubMed
.
- M. Fiorillo, A. F. Verre, M. Iliut, M. Peiris-Pages, B. Ozsvari, R. Gandara, A. R. Cappello, F. Sotgia, A. Vijayaraghavan and M. P. Lisanti, Oncotarget, 2015, 6, 3553–3562 CrossRef PubMed
.
- Y. Kang, J. Liu, J. Wu, Q. Yin, H. Liang, A. Chen and L. Shao, Int. J. Nanomed., 2017, 12, 5501–5510 CrossRef CAS PubMed
.
- A. Shafiee, S. Iravani and R. S. Varma, MedComm, 2022, 3, e118 CrossRef CAS PubMed
.
- N. Rodriguez-Losada, J. de la Rosa, M. Larriva, R. Wendelbo, J. A. Aguirre, J. S. Castresana and S. J. Ballaz, J. Adv. Res., 2020, 23, 37–45 CrossRef CAS PubMed
.
- M. Pichla, G. Bartosz and I. Sadowska-Bartosz, Oxid. Med. Cell. Longevity, 2020, 2020, 1–11 Search PubMed
.
- M. Ebrahimi, M. Asadi and O. Akhavan, ACS Biomater. Sci. Eng., 2022, 8, 54–81 CrossRef CAS PubMed
.
- X. Wu, F. Mu, Y. Wang and H. Zhao, Molecules, 2018, 23, 2050 CrossRef PubMed
.
- H. Yue, X. Zhou, M. Cheng and D. Xing, Nanoscale, 2018, 10, 1063–1071 RSC
.
- V. Chaudhary, V. Khanna, H. T. Ahmed Awan, K. Singh, M. Khalid, Y. K. Mishra, S. Bhansali, C. Z. Li and A. Kaushik, Biosens. Bioelectron., 2023, 220, 114847 CrossRef CAS PubMed
.
- V. Chugh, A. Basu, A. Kaushik and A. K. Basu, ECS Sensors Plus, 2023, 2, 015001 CrossRef
.
|
This journal is © The Royal Society of Chemistry 2023 |
Click here to see how this site uses Cookies. View our privacy policy here.