DOI:
10.1039/D2GC03879J
(Paper)
Green Chem., 2023,
25, 978-985
Tandem electrocatalytic aziridination – ring expansion of simple aromatic olefins using ammonia and carbon dioxide†
Received
17th October 2022
, Accepted 17th January 2023
First published on 19th January 2023
Abstract
N-heterocycles are prominent structural motifs frequently occurring in organic synthesis and applications. Therefore, straightforward and green synthesis methods using common starting materials like alkenes are highly desired. Here, the metal-free electrochemical coupling of aromatic olefins with NH3 and CO2 or CS2 in a telescoped sequence is reported, forming 2-oxazolidinones or 2-thiazolidinethiones. The reaction proceeds via a N–H aziridine, which subsequently undergoes ring expansion with CO2 or CS2. Both steps are mediated by the same simple iodide catalyst and total yields of up to 91% referring to the alkene are achieved with excellent regioselectivities. This sustainable reaction using readily available materials possesses an excellent atom efficiency with only H2 as a potentially useful by-product.
Introduction
2-Oxazolidinones are attractive heterocyclic compounds that are increasingly investigated for their pharmacological activities. For example, linezolid (Zyvox®) and tedizolid (Sivextro®) among others are marketed worldwide for their unique antibacterial activity, even towards multidrug-resistant bacteria.1–5 Additionally, 2-oxazolidinones are used as intermediates in organic synthesis,6–8 as chiral auxiliaries9–15 and in polymers.16–18 Depending on the starting substrate, intermolecular synthetic routes for these cyclic urethanes may lead through aziridines,19–25 propargylamines26–31 or aminoalcohols32–37 (Scheme 1a). In all three methods, formation of the oxazolidinone scaffold is achieved by using CO2 as the second reactant, thereby contributing to the global demand for sustainable uses of CO2.38–40 However, drawbacks are the need for specialized starting substrates that need to be synthesized beforehand, creating extra waste, and the use of complex metal catalysts.26,41,42 In addition, only a limited number of reports are successful in directly synthesizing N-unsubstituted 2-oxazolidinones.35,43 Having N–H 2-oxazolidinones is desired for their use as a chiral auxiliary9–15 and additionally offers the possibility to functionalize the nitrogen atom as required in downstream syntheses, for instance by performing the well-known Buchwald–Hartwig amination,44,45 even immediately towards active pharmaceutical ingredients.46,47 By replacing CO2 with its sulfur analogue CS2, it is possible to synthesize 2-thiazolidinethiones19,41,48–53 (Scheme 1a) which possess analogous properties and applications as 2-oxazolidinones regarding both biological activity9,54–56 and organic synthetic use.57–60 Moreover, they are used as a less toxic accelerator in polychloroprene production, an essential class of commercial rubber.61
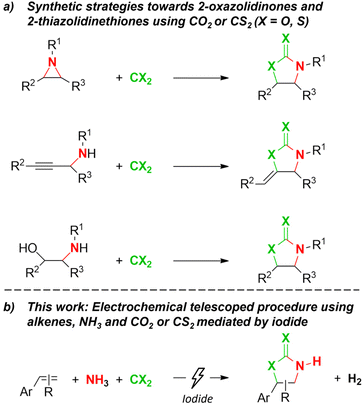 |
| Scheme 1 Synthetic strategies towards 2-oxazolidinones and 2-thioazolidinethiones using CX2 (X = O, S) reported in literature (a) and this work (b). | |
Synthesizing these important heterocycles directly from abundant commodity chemicals like olefins can provide a highly efficient and sustainable approach. However, recent reports using alkenes require the use of a very specific hydroxylamine reagent like 2,2,2-trichloroethyl (2,4-dichlorobenzoyl)oxycarbamate with a homogeneous Fe(II) catalyst62 or a double protected carbamate (N-(fluorosulfonyl)-benzyl carbamate) together with a hypervalent iodine oxidant,63 both methods resulting in suboptimal atom efficiency. In our previous research, we synthesized unprotected N–H aziridines electrochemically from simple aromatic alkenes using NH3 as the atom efficient nitrogen source and LiI as the mediator.64 On the grounds that iodide is frequently used in organic transformations65–70 and more specifically that iodide-catalyzed ring expansions of N-substituted aziridines with CO2 or CS2 are known,71,72 we envisioned that it should be possible to obtain 2-oxazolidinones or 2-thiazolidinethiones from the N–H aziridines by simply adding CO2 or CS2 in our system, thereby using iodide twice as the mediator. We here report a telescoped electrochemical synthesis procedure towards N-unprotected 2-oxazolidinones and 2-thiazolidinethiones by using NH3 and CO2 or CS2 (Scheme 1b). This sustainable, metal-free method shows excellent atom efficiency and is able to synthesize these unprotected heterocycles directly from simple aromatic olefins using renewable energy and readily available and abundant materials. Additionally, waste formation and energy loss are prevented due to the telescoped sequence while also using the greenhouse gas CO2 as a sustainable building block.
Results and discussion
To evaluate the telescoped synthesis procedure using CO2, we performed an electrocatalytic N–H aziridination of styrene with NH3 according to our previous procedure (94% yield, see ESI†).64 After completion of the electrolysis, the reaction mixture was transferred into a stainless steel pressure reactor after which the conditions for the CO2 ring expansion were optimized (Table 1). With a CO2 pressure of 5 bar at a temperature of 70 °C for 18 h, an overall yield based on styrene of 5-phenyl-2-oxazolidinone 2a of 86% was achieved (entry 1). The overall yield was diminished to 42% and 73% when operating at room temperature or at 90 °C, respectively (entries 2 and 3). A shorter reaction time of 4 h provided a total yield of 71% (entry 4). Increasing the CO2 pressure to 15 bar afforded an excellent total yield of 87% (92% CO2 insertion yield in step 2, entry 5). The 4-phenyl-2-oxazolidinone 3a was found in a yield of only 4%, showing a remarkable 96% regioselectivity for 2a. In addition, working with 15 bar CO2 ensured better reproducibility compared to 5 bar CO2. Working at room temperature with 15 bar CO2 provided a lower overall yield of 72% (entry 6). It is known that 1,8-diazabicyclo[5.4.0]undec-7-ene (DBU) can facilitate the uptake of CO2 into solution and catalyze CO2 insertion reactions.73–76 In our system, however, it did not improve the yield any further (entries 7 and 8) nor did it provide any catalytic effect with shorter reaction times (entry 9). Also, omitting DBU is beneficial for the environmental factor of the telescoped reaction sequence.
Table 1 Optimization of the telescoped CO2 ring expansion of electrocatalytically synthesized 2-phenylaziridine
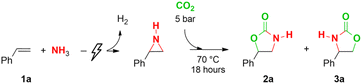
|
Entry |
Deviation from optimization conditionsa |
Yield 2a (%) |
Yield 3a (%) |
Conditions for the electrocatalytic N–H aziridination with LiI: see ESI.† Reaction mixture is transferred to a stainless steel pressure reactor. Optimization conditions: 5 bar CO2 pressure, 70 °C, 18 h. Yields are based on 1H-NMR spectra of crude mixtures, referring to the starting alkene. Number in parentheses indicates the isolated yield. DBU = 1,8-diazabicyclo[5.4.0]undec-7-ene. |
1 |
— |
86 |
5 |
2 |
Room temperature |
42 |
4 |
3 |
90 °C |
73 |
5 |
4 |
4 hours |
71 |
9 |
5
|
15 bar CO
2
|
87 (81)
|
4
|
6 |
15 bar CO2, room temperature |
72 |
3 |
7 |
+DBU (0.2 equiv.) |
84 |
9 |
8 |
+DBU (1.4 equiv.) |
77 |
5 |
9 |
+DBU (0.2 equiv.), 4 hours |
67 |
5 |
With the optimized conditions in hand (Table 1, entry 5), we applied this procedure to different aromatic olefins (Table 2). Generally, the yield of the CO2 insertion step varies between 82% and 99%, which results in good to excellent total yields up to 91% with respect to the starting olefin (2b–h). Only when both ortho-positions are occupied, lower total yields are achieved due to a poor aziridination step (2i–j). Whereas 2-vinylpyridine still shows a good overall yield of 76% (2k), 4-vinylpyridine showed a moderate yield in the CO2 insertion step, therefore leading to a lower total yield of 38% (2l). Moreover, a CO2 insertion yield of 84% and a total yield of 80% are obtained with 2-vinylnaphthalene (2m). When α-methylstyrene (2n) is used, steric hindrance impedes the CO2 insertion and a total yield of 49% is achieved, despite the 98% aziridination yield. In the N–H aziridination of trans-β-methyl styrene, a mixture of cis- and trans-2-methyl-3-phenylaziridine in a combined yield of 52% was obtained with the trans isomer as the major product (83/17 ratio).64 Ring expansion with CO2 resulted in an almost exclusive formation of trans-4-methyl-5-phenyl-2-oxazolidinone (2o) in a 95/5 ratio over the cis isomer in a combined overall yield of 48%. Finally, a gram-scale synthesis of compound 2a resulted in an overall yield of 80% (see ESI†).
Table 2 Telescoped CO2 ring expansion of N–H aziridines synthesized electrocataytically from simple aromatic olefinsa
Reaction conditions: see Table 1, entry 5. Yields are based on 1H-NMR spectra of crude mixtures, referring to the starting alkene. Yields in parentheses refer to the CO2 insertion of the N–H aziridine.
|
|
Next, we also investigated if this telescoped procedure could be extrapolated to CS2, thereby synthesizing 2-thiazolidinethiones. We started with modified conditions compared to the CO2 ring expansion, exchanging the 15 bar CO2 for 15 equivalents of CS2 at a temperature of 40 °C for 18 h (Table 3). A total yield of 51% of 5-phenyl-2-thiazolidinethione 4a was achieved (entry 1). No improvement of the yield was observed at a higher temperature of 70 °C (49%, entry 2), while room temperature lowered the total yield to 34% (entry 3). Interestingly, a higher overall yield of 64% was obtained with a shorter reaction time of 4 h (entry 4). Moreover, the same yield is already observed after just 10 minutes of reaction time (66%, entry 5). Similar as for CO2, the CS2 ring expansion shows an excellent regioselectivity for the 5-phenyl isomer 4a of 97%. Additionally, with a lower CS2 amount of 5 equivalents, a slightly decreased yield of 61% is obtained (entry 6).
Table 3 Optimization of the telescoped CS2 ring expansion of electrocatalytically synthesized 2-phenylaziridine
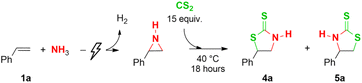
|
Entry |
Deviation from optimization conditionsa |
Yield 4a (%) |
Yield 5a (%) |
Conditions for the electrocatalytic N–H aziridination with LiI: see ESI.† Reaction mixture is transferred to a glass reaction vial. Optimization conditions: 15 equiv. CS2, 40 °C, 18 h. Yields are based on 1H-NMR spectra of crude mixtures, referring to the starting alkene. Number in parentheses indicates the isolated yield. |
1 |
— |
51 |
3 |
2 |
70 °C |
49 |
1 |
3 |
Room temperature |
34 |
2 |
4 |
4 hours |
64 |
3 |
5
|
10 minutes
|
66 (58)
|
2
|
6 |
10 minutes, 5 equiv. CS2 |
61 |
2 |
Different aromatic olefins were subjected to the optimized conditions (Table 3, entry 5) to synthesize 2-thiazolidinethiones (Table 4). Generally, the yield of the CS2 insertion step is around 15% lower than for CO2, with values ranging from 62% to 83%, resulting in total yields of up to 73% (4b–h). Additionally, the substituent type on the aromatic ring, i.e. electron-withdrawing versus electron-donating, did not influence the CO2 insertion yield (Table 2, 2b–h), whereas a noticeable difference in the CS2 insertion reaction is found between them of around 10–15%. For 2,6-dichlorostyrene, together with a poor aziridination yield, this results in a low total yield of 20% (4i). Good yields for both steps are achieved with 2- and 4-vinylpyridine, resulting in total yields of 62% and 50% respectively (4j–k). A low overall yield of 12% is achieved with α-methylstyrene (4l), despite the 98% aziridination yield, most likely due to the increased steric hindrance of the larger S-atoms compared to CO2 (Table 2, n). Using trans-β-methyl styrene, a cis/trans product mixture in an overall yield of 36% is obtained (4m), but interestingly this time with the cis isomer as the major product (59/41 ratio cis/trans). Finally, a gram-scale synthesis of compound 4a resulted in an overall yield of 59% (see ESI†).
Table 4 Telescoped CS2 ring expansion of N–H aziridines electrocatalytically synthesized from simple aromatic olefinsa
Reaction conditions: see Table 3, entry 5. Yields are based on 1H-NMR spectra of crude mixtures, referring to the starting alkene. Yields in parentheses refer to the CS2 insertion of the N–H aziridine.
|
|
Next, control experiments were performed to obtain mechanistic insights (Fig. 1). To see if iodide, which is already needed for the electrocatalytic N–H aziridination, also catalyzes the ring expansion of the aziridine,65,66 we removed the salts after the aziridination step according to our work-up procedure (see ESI†). Afterwards, the solvent was added again and the homogeneous mixture was equally divided over three pressure reactors. LiI was added in three different amounts of 0, 0.2 and 2 equivalents and standard reaction conditions for the CO2 insertion were applied (dark blue curve). As mentioned before, a CO2 insertion yield of 92% is observed with our telescoped condition of 2 equivalents of LiI. Reducing the amount of LiI greatly decreases the yield, even down to only 9% when the halide is completely absent, despite a full conversion of the aziridine. Most likely formation of oligomers and homo- or copolymerization with CO2 occur as reported before in similar conditions with elevated temperatures.19,22,71,76,77 These results strongly suggest that an increasing amount of LiI suppresses these parasitic side reactions and favors the selective CO2 fixation. When standard conditions for the CS2 insertion are applied, a similar trend is observed (light blue curve). Analogous experiments where LiBr was added resulted in similar trends compared to the iodide counterparts, albeit to a lesser extent, especially for CS2 (red curves). The ratio of the 2-oxazolidinone isomers 2a and 3a for all three halide contents was also determined and is depicted on the right y-axis of Fig. 1. With LiI (grey bars) a strong increase is observed up to a ratio of 18 which reflects the yields observed for both products (Table 1, entry 5). Again, a similar trend is found with LiBr but less pronounced (green bars). In summary, not only does iodide mediates both ring expansion reactions; it also strongly improves the regioselectivity and it is the better halide to perform these reactions.
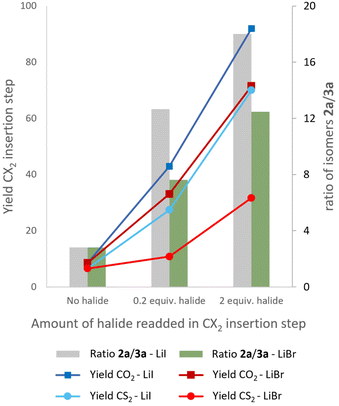 |
| Fig. 1 Control experiments where the influence of the halide is studied regarding the yield of the CX2 insertion step towards products 2a or 4a (curves, left y-axis) and the distribution of isomers 2a and 3a (bars, right y-axis) using 1H-NMR. See ESI for more experimental details.† | |
Based on the results described above, a reaction mechanism is proposed in Scheme 2. The iodide-mediated N–H aziridination proceeds via a reaction of an electrochemically generated NH2I with styrene towards a vicinal iodoamine. Base-assisted cyclization provides the N–H aziridine.64 Next, the lone pair of the aziridine nitrogen can bond with a molecule of CO2 to create a charged adduct.21–23,71,72 Due to electronic effects of the aryl substituent, nucleophilic substitution with iodide occurs on the benzylic carbon, even though it is sterically less accessible.21,24,78 This also explains the excellent regioselectivity of the resulting products, as was also found in the control experiments (Fig. 1). Finally, ring closing with expulsion of the iodide mediator yields the 2-oxazolidinone product. An analogous mechanism with CS2 can be envisioned.
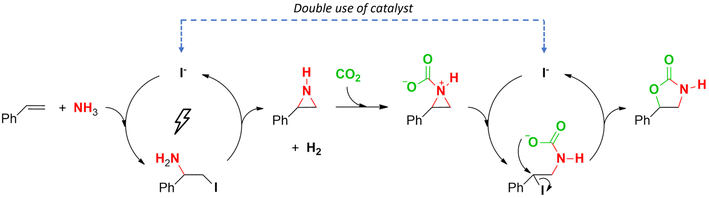 |
| Scheme 2 Proposed reaction mechanism for the telescoped electrocatalytic coupling of simple aromatic alkenes with NH3 and CO2. A similar mechanism for CS2 can be imagined. | |
Conclusion
In summary, we demonstrate for the first time the coupling of simple aromatic olefins with NH3 and CO2 or CS2 in an electrochemical telescoped procedure to synthesize 2-oxazolidinones or 2-thiazolidinethiones. Overall yields referring to the alkene of up to 91% are obtained with remarkable regioselectivities for the 5-aryl-2-oxazolidinone and 5-aryl-2-thiazolidinethione of 96% and 97% respectively. Additionally, gram-scale experiments resulted in an overall yield of 80% and 59% of compound 2a and 4a, respectively. Valuable assets in this sustainable metal-free procedure towards these important heterocycles include starting from commodity chemicals, waste prevention due to a telescoped reaction sequence, CO2 utilization, excellent atom efficiency and an efficient twofold use of a simple iodide catalyst.
Conflicts of interest
There are no conflicts to declare.
Acknowledgements
J. R. V. and D. E. D. V. thank the FWO (Fonds voor Wetenschappelijk Onderzoek, Flanders, Belgium) for SB funding (FWO Grant number 1SB1522N).
References
- Q. Zhao, L. Xin, Y. Liu, C. Liang, J. Li, Y. Jian, H. Li, Z. Shi, H. Liu and W. Cao, Current Landscape and Future Perspective of Oxazolidinone Scaffolds Containing Antibacterial Drugs, J. Med. Chem., 2021, 64, 10557–10580, DOI:10.1021/acs.jmedchem.1c00480.
- R. Siddaraj, S. Govindaiah, R. Ningegowda, N. Shivananju and B. Priya, A novel and expeditious synthesis of oxazolidinone drugs linezolid and eperezolid, Eur. J. Chem., 2018, 9, 353–359, DOI:10.5155/eurjchem.9.4.353-359.1783.
- H. Zhao, Y. Lu, L. Sheng, Z. Yuan, B. Wang, W. Wang, Y. Li, C. Ma, X. Wang, D. Zhang and H. Huang, Discovery of Fluorine-Containing Benzoxazinyl-oxazolidinones for the Treatment of Multidrug Resistant Tuberculosis, ACS Med. Chem. Lett., 2017, 8, 533–537, DOI:10.1021/acsmedchemlett.7b00068.
- S. Ding, J.-C. Ji, M.-J. Zhang, Y.-S. Yang, R. Wang, X.-L. Zhu, L.-H. Wang, Y. Zhong, L. Gao, M. Lu, J. Liu and Y. Chen, Exploration of the structure–activity relationship and druggability of novel oxazolidinone-based compounds as Gram-negative antibacterial agents, Arch. Pharm. Chem. Life Sci., 2019, 352, 1900129, DOI:10.1002/ardp.201900129.
- C. Foti, A. Piperno, A. Scala and O. Giuffrè, Oxazolidinone Antibiotics: Chemical, Biological and Analytical Aspects, Molecules, 2021, 26, 4280–4293, DOI:10.3390/molecules26144280.
- T. Andreou, A. Costa, L. Esteban, L. Gonzàlez, G. Mas and J. Vilarrasa, Synthesis of (−)-Amphidinolide K Fragment, C9–C22, Org. Lett., 2005, 7, 4083–4086, DOI:10.1021/ol051200o.
- R. Watson, D. Batty, A. Baxter, D. Hannah, D. Owen and J. Montana, An enantioselective synthesis of sulphonamide hydroxamic acids as matrix metalloproteinase inhibitors, Tetrahedron Lett., 2002, 43, 683–685, DOI:10.1016/S0040-4039(01)02151-7.
- P.-Q. Huang and H. Geng, Ni-Catalyzed chemoselective alcoholysis of N-acyloxazolidinones, Green Chem., 2018, 20, 593–599, 10.1039/C7GC03534A.
- F. Velazquez and H. Olivo, The Application of Chiral Oxazolidinethiones and Thiazolidinethiones in Asymmetric Synthesis, Curr. Org. Chem., 2002, 6, 303–340, DOI:10.2174/1385272024605023.
- M. Crimmins, B. King, E. Tabet and K. Chaudhary, Asymmetric Aldol Additions: Use of Titanium Tetrachloride and (−)-Sparteine for the Soft Enolization of N-Acyl Oxazolidinones, Oxazolidinethiones, and Thiazolidinethiones, J. Org. Chem., 2001, 66, 894–902, DOI:10.1021/jo001387r.
- D. Evans, J. Bartroli and T. Shih, Enantioselective aldol condensations. 2. Erythro-selective chiral aldol condensations via boron enolates, J. Am. Chem. Soc., 1981, 103, 2127–2129, DOI:10.1021/ja00398a058.
- A. Nazari, M. Heravi and V. Zadsirjan, Oxazolidinones as chiral auxiliaries in asymmetric aldol reaction applied to natural products total synthesis, J. Organomet. Chem., 2021, 932, 121629, DOI:10.1016/j.jorganchem.2020.121629.
- M. Heravi, V. Zadsirjan and B. Farajpour, Applications of oxazolidinones as chiral auxiliaries in the asymmetric alkylation reaction applied to total synthesis, RSC Adv., 2016, 6, 30498–30551, 10.1039/C6RA00653A.
- V. Zadsirjan and M. Heravi, Oxazolidinones as Chiral Auxiliaries in the Asymmetric 1,4-Conjugate Addition Reaction Applied to the Total Synthesis of Natural Products: A Supplemental Mini-Review, Curr. Org. Chem., 2018, 15, 3–20, DOI:10.2174/1570179414666170601115831.
-
A. Mourad and C. Czekelius, Oxazolidinones and Related Heterocycles as Chiral Auxiliaries/Evans and Post-Evans Auxiliaries, in Heterocycles as Chiral Auxiliaries in Asymmetric Synthesis. Topics in Heterocyclic Chemistry, ed. M. Braun, Springer, Cham, 2020, vol. 55, pp. 113–156. DOI:10.1007/7081_2020_36.
- C. Mero and N. Porter, Free-Radical Polymerization and Copolymerization of Acrylimides: Homopolymers of Oxazolidinone Acrylimide and Control of 1,5-Stereochemistry in Copolymers Derived from Isobutylene and an Oxazolidinone Acrylimide, J. Org. Chem., 2000, 65, 775–781, DOI:10.1021/jo9914420.
- Z. Wang, C. Detrembleur and A. Debuigne, Reversible deactivation radical (co)polymerization of dimethyl methylene oxazolidinone towards responsive vicinal aminoalcohol-containing copolymers, Polym. Chem., 2020, 11, 7207–7220, 10.1039/D0PY01255F.
- T. Fujita and S. Yamago, Lewis-Acid-Mediated Stereospecific Radical Polymerization of Acrylimides Bearing Chiral Oxazolidinones, Chem. – Eur. J., 2015, 21, 18547–18550, DOI:10.1002/chem.201504184.
- A. Liu, L. He, S. Peng, Z. Pan, J. Wang and J. Gao, Environmentally benign chemical fixation of CO2 catalyzed by the functionalized ion-exchange resins, Sci. China: Chem., 2010, 53, 1578–1585, DOI:10.1007/s11426-010-4028-6.
- R. Watile, D. Bagal, K. Deshmukh, K. Dhake and B. Bhanage, Polymer supported diol functionalized ionic liquids: An efficient, heterogeneous and recyclable catalyst for 5-aryl-2-oxazolidinones synthesis from CO2 and aziridines under mild and solvent free condition, J. Mol. Catal. A: Chem., 2011, 351, 196–203, DOI:10.1016/j.molcata.2011.10.007.
- Y. Du, Y. Wu, A.-H. Liu and L.-N. He, Quaternary Ammonium Bromide Functionalized Polyethylene Glycol: A Highly Efficient and Recyclable Catalyst for Selective Synthesis of 5-Aryl-2-oxazolidinones from Carbon Dioxide and Aziridines Under Solvent-Free Conditions, J. Org. Chem., 2008, 73, 4709–4712, DOI:10.1021/jo800269v.
- Z.-Z. Yang, L.-N. He, S.-Y. Peng and A.-H. Liu, Lewis basic ionic liquids-catalyzed synthesis of 5-aryl-2-oxazolidinones from aziridines and CO2 under solvent-free conditions, Green Chem., 2010, 12, 1850–1854, 10.1039/C0GC00286K.
- C. Phung, R. Ulrich, M. Ibrahim, N. Tighe, D. Lieberman and A. Pinhas, The solvent-free and catalyst-free conversion of an aziridine to an oxazolidinone using only carbon dioxide, Green Chem., 2011, 13, 3224–3229, 10.1039/C1GC15850C.
- X.-Y. Dou, L.-N. He and Z.-Z. Yang, Proline-Catalyzed Synthesis of 5-Aryl-2-oxazolidinones from Carbon Dioxide and Aziridines Under Solvent-Free Conditions, Synth. Commun., 2012, 42, 62–74, DOI:10.1080/00397911.2010.521903.
- A. Ueno, Y. Kayaki and T. Ikariya, Cycloaddition of tertiary aziridines and carbon dioxide using a recyclable organocatalyst, 1,3-di-tert-butylimidazolium-2-carboxylate: straightforward access to 3-substituted 2-oxazolidones, Green Chem., 2013, 15, 425–430, 10.1039/C2GC36414J.
- S. Arshadi, E. Vessally, M. Sobati, A. Hosseinian and A. Bekhradnia, Chemical fixation of CO2 to N-propargylamines: A straightforward route to 2-oxazolidinones, J. CO2 Util., 2017, 19, 120–129, DOI:10.1016/j.jcou.2017.03.009.
- M. Shi and Y.-M. Shen, Transition-Metal-Catalyzed Reactions of Propargylamine with Carbon Dioxide and Carbon Disulfide, J. Org. Chem., 2002, 67, 16–21, DOI:10.1021/jo0014966.
- M. Yoshida, T. Mizuguchi and K. Shishido, Synthesis of oxazolidinones by efficient fixation of atmospheric CO2 with propargylic amines by using a silver/1,8-diazabicyclo[5.4.0]undec-7-ene (DBU) Dual-catalyst system, Chem. – Eur. J., 2012, 18, 15578–15581, DOI:10.1002/chem.201203366.
- P. Brunel, J. Monot, C. Kefalidis, L. Maron, B. Martin-Vaca and D. Bourissou, Valorization of CO2: Preparation of 2-Oxazolidinones by Metal–Ligand Cooperative Catalysis with SCS Indenediide Pd Complexes, ACS Catal., 2017, 7, 2652–2660, DOI:10.1021/acscatal.7b00209.
- Y. Shunsuke, F. Kosuke, K. Satoshi and Y. Tohru, Silver-catalyzed Preparation of Oxazolidinones from Carbon Dioxide and Propargylic Amines, Chem. Lett., 2009, 38, 786–787, DOI:10.1246/cl.2009.786.
- R. Maggi, C. Bertolotti, E. Orlandini, C. Oro, G. Sartori and M. Selva, Synthesis of oxazolidinones in supercritical CO2 under heterogeneous catalysis, Tetrahedron Lett., 2007, 48, 2131–2134, DOI:10.1016/j.tetlet.2007.01.116.
- S. Foo, Y. Takada, Y. Yamazaki and S. Saito, Dehydrative synthesis of chiral oxazolidinones catalyzed by alkali metal carbonates under low pressure of CO2, Tetrahedron Lett., 2013, 54, 4717–4720, DOI:10.1016/j.tetlet.2013.06.100.
- C. Bu, Y. Gong, M. Du, C. Chen, S. Chaemchuen, J. Hu, Y. Zhang, H. Velázquez, Y. Yuan and F. Verpoort, Green Synthesis of 2-Oxazolidinones by an Efficient and Recyclable CuBr/Ionic Liquid System via CO2, Propargylic Alcohols, and 2-Aminoethanols, Catalysts, 2021, 11, 1–15, DOI:10.3390/catal11020233.
- K. Tominaga and Y. Sasaki, Synthesis of 2-Oxazolidinones from CO2 and 1,2-Aminoalcohols Catalyzed by n-Bu2SnO, Synlett, 2002, 307–309, DOI:10.1055/s-2002-19780.
- A. Casadei, M. Feroci, A. Inesi, L. Rossi and G. Sotgiu, The Reaction of 1,2-Amino Alcohols with Carbon Dioxide in the Presence of 2-Pyrrolidone Electrogenerated Base. New Synthesis of Chiral Oxazolidin-2-ones, J. Org. Chem., 2000, 65, 4759–4761, DOI:10.1021/jo0002386.
- T. Niemi, I. Fernández, B. Steadman, J. Mannisto and T. Repo, Carbon dioxide-based facile synthesis of cyclic carbamates from amino alcohols, Chem. Commun., 2018, 54, 3166–3169, 10.1039/C8CC00636A.
- S. Pulla, C. Felton, Y. Gartia, P. Ramidi and A. Ghosh, Synthesis of 2-Oxazolidinones by Direct Condensation of 2-Aminoalcohols with Carbon Dioxide Using Chlorostannoxanes, ACS Sustainable Chem. Eng., 2013, 1, 309–312, DOI:10.1021/sc300077m.
- S. Arshadi, A. Banaei, S. Ebrahimiasl, A. Monfared and E. Vessally, Solvent-free incorporation of CO2 into 2- oxazolidinones: a review, RSC Adv., 2019, 9, 19465–19482, 10.1039/C9RA00551J.
- S. Pulla, C. Felton, P. Ramidi, Y. Gartia, N. Ali, U. Nasini and A. Ghosh, Advancements in oxazolidinone synthesis utilizing carbon dioxide as a C1 source, J. CO2 Util., 2013, 2, 49–57, DOI:10.1016/j.jcou.2013.07.005.
- N. Kielland, C. Whiteoak and A. Kleij, Stereoselective Synthesis with Carbon Dioxide, Adv. Synth. Catal., 2013, 355, 2115–2138, DOI:10.1002/adsc.201300422.
- Y. Xie, C. Lu, B. Zhao, Q. Wang and Y. Yao, Cycloaddition of Aziridine with CO2/CS2 Catalyzed by Amidato Divalent Lanthanide Complexes, J. Org. Chem., 2019, 84, 1951–1958, DOI:10.1021/acs.joc.8b02924.
- M. Sengoden, M. North and A. Whitwood, Synthesis of Oxazolidinones by using Carbon Dioxide as a C1 Building Block and an Aluminium-Based Catalyst, ChemSusChem, 2019, 12, 3296–3303, DOI:10.1002/cssc.201901171.
- Y.-M. Shen, W.-L. Duan and M. Shi, Chemical Fixation of Carbon Dioxide Co-Catalyzed by a Combination of Schiff Bases or Phenols and Organic Bases, Eur. J. Org. Chem., 2004, 3080–3089, DOI:10.1002/ejoc.200400083.
- P. Ruiz-Castillo and S. L. Buchwald, Applications of Palladium-Catalyzed C–N Cross-Coupling Reactions, Chem. Rev., 2016, 116, 12564–12649, DOI:10.1021/acs.chemrev.6b00512.
- J. M. Dennis, N. A. White, R. Y. Liu and S. L. Buchwald, Breaking the Base Barrier: An Electron-Deficient Palladium Catalyst Enables the Use of a Common Soluble Base in C−N Coupling, J. Am. Chem. Soc., 2018, 140, 4721–4725, DOI:10.1021/jacs.8b01696.
- A. Ghosh, J. E. Sieser, M. Riou, W. Cai and L. Rivera-Ruiz, Palladium-Catalyzed Synthesis of N-Aryloxazolidinones from Aryl Chlorides, Org. Lett., 2003, 5, 2207–2210, DOI:10.1021/ol034428p.
- B. Mallesham, B. M. Rajesh, P. R. Reddy, D. Srinivas and S. Trehan, Highly Efficient CuI-Catalyzed Coupling of Aryl Bromides with Oxazolidinones Using Buchwald's Protocol: A Short Route to Linezolid and Toloxatone, Org. Lett., 2003, 5, 963–965, DOI:10.1021/ol026902h.
- N. Chen, W. Jia and J. Xu, A Versatile Synthesis of Various Substituted Taurines from Vicinal Amino Alcohols and Aziridines, Eur. J. Org. Chem., 2009, 5841–5846, DOI:10.1002/ejoc.200900759.
- A. Cruz, I. Padilla-Martínez and E. García-Báez, Efficient synthesis of cis-thiazolidinethiones derived from ephedrines, Tetrahedron Assymetry, 2011, 22, 394–398, DOI:10.1016/j.tetasy.2011.02.016.
- Y. Shi, B. Tang, X.-L. Jiang, Y.-E. Jiao, H. Xu and B. Zhao, Highly effective CS2 conversion with aziridines catalyzed by novel [Dy24] nano-cages in MOFs under mild conditions, J. Mater. Chem. A, 2022, 10, 4889–4894, 10.1039/D1TA10522A.
- A. Singh and N. Goel, Cycloaddition of CS2 to N–benzylaziridine to synthesize N–benzylthiazolidine–2−thione: a novel reaction route proposed by DFT Study, J. Phys. Org. Chem., 2016, 29, 544–549, DOI:10.1002/poc.3578.
- A. Nechaev, A. Peshkov, K. Van Hecke, V. Peshkov and E. Van der Eycken, Synthesis of Thiazolidine-2-thiones through a One-Pot A3-Coupling–Carbon Disulfide Incorporation Process, Eur. J. Org. Chem., 2017, 1063–1069, DOI:10.1002/ejoc.201601103.
- D. Delaunay, L. Toupet and M. Le Corre, Reactivity of .beta.-Amino Alcohols with Carbon Disulfide Study on the Synthesis of 2-Oxazolidinethiones and 2-Thiazolidinethiones, J. Org. Chem., 1995, 60, 6604–6607, DOI:10.1021/jo00125a059.
- N. Chen, H. Du, W. Liu, S. Wang, X. Li and J. Xu, Synthesis and Fungicidal Activity of Simple Structural 1,3-Thiazolidine-2-Thione Derivatives, Phosphorus, Sulfur Silicon Relat. Elem., 2015, 190, 112–122, DOI:10.1080/10426507.2014.931399.
- M. Mehrabani, K. Safa, M. Rahimi, M. Alyari, K. Ganbarov and H. Kafil, Thiazolidine-2-Thione and 2-Imino-1,3-Dithiolane Derivatives: Synthesis and Evaluation of Antimicrobial Activity, Pharm. Chem. J., 2020, 54, 588–595, DOI:10.1007/s11094-020-02244-5.
- A. Das, M. Ashraf and B. Banik, Thione Derivatives as Medicinally Important Compounds, ChemistrySelect, 2021, 6, 9069–9100, DOI:10.1002/slct.202102398.
- M. Crimmins and K. Chaudhary, Titanium Enolates of Thiazolidinethione Chiral Auxiliaries: Versatile Tools for Asymmetric Aldol Additions, Org. Lett., 2000, 2, 775–777, DOI:10.1021/ol9913901.
- M. Hodge and H. Olivo, Stereoselective aldol additions of titanium enolates of N-acetyl-4-isopropyl-thiazolidinethione, Tetrahedron, 2004, 60, 9397–9403, DOI:10.1016/j.tet.2004.08.008.
- Y. Zhang and T. Sammakia, Synthesis of a New N-Acetyl Thiazolidinethione Reagent and Its Application to a Highly Selective Asymmetric Acetate Aldol Reaction, Org. Lett., 2004, 6, 3139–3141, DOI:10.1021/ol048810t.
- A. Osorio-Lozada and H. Olivo, Indene-Based Thiazolidinethione Chiral Auxiliary for Propionate and Acetate Aldol Additions, Org. Lett., 2008, 10, 617–620, DOI:10.1021/ol702980p.
-
R. Schubart, Dithiocarbamic Acid and Derivatives, in Ullmann's encyclopedia of Industrial chemistry, 5th edn, Wiley-VCH, Weinheim, 2012, 11, 495–522. DOI:10.1002/14356007.a09_001.
- D.-F. Lu, C.-L. Zhu, Z.-X. Jia and H. Xu, Iron(II)-Catalyzed Intermolecular Amino-Oxygenation of Olefins through the N−O Bond Cleavage of Functionalized Hydroxylamines, J. Am. Chem. Soc., 2014, 136, 13186–13189, DOI:10.1021/ja508057u.
- C. Wata and T. Hashimoto, Organoiodine-Catalyzed Enantioselective Intermolecular Oxyamination of Alkenes, J. Am. Chem. Soc., 2021, 143, 1745–1751, DOI:10.1021/jacs.0c11440.
- J. R. Vanhoof, P. De Smedt, B. Krasniqi, R. Ameloot, D. Sakellariou and D. De Vos, Direct Electrocatalytic N–H Aziridination of Aromatic Alkenes Using Ammonia, ACS Sustainable Chem. Eng., 2021, 9, 11596–11603, DOI:10.1021/acssuschemeng.1c04473.
- X.-Y. Liu, B.-Q. Cheng, Y.-C. Gua, X.-Q. Chu, Y.-X. Li, T.-P. Loh and Z.-L. Shen, Bismuth-Mediated Diastereoselective Allylation Reaction of Carbonyl Compounds with Cyclic Allylic Halides or Cinnamyl Halide, Adv. Synth. Catal., 2019, 361, 542–549, DOI:10.1002/adsc.201801297.
- X.-R. Li, W.-X. Li, Z.-W. Zhang, C. Shen, X. Zhou, X.-Q. Chu, W. Rao and Z.-L. Shen, Stereoselective synthesis of fluoroalkylated (Z)-alkene via nickel-catalyzed and iron-mediated hydrofluoroalkylation of alkynes, Org. Chem. Front., 2021, 8, 6377–6383, 10.1039/D1QO00983D.
- B.-Z. Chen, M.-L. Zhi, C.-X. Wang, X.-Q. Chu, Z.-L. Shen and T.-P. Loh, Synthesis of Alkyl Indium Reagents by Using Unactivated Alkyl Chlorides and Their Applications in Palladium-Catalyzed Cross-Coupling Reactions with Aryl Halides, Org. Lett., 2018, 20(7), 1902–1905, DOI:10.1021/acs.orglett.8b00441.
- P. M. Jadhav, A. B. Rode, L. Kótai, R. P. Pawar and S. U. Tekale, Revisiting applications of molecular iodine in organic synthesis, New J. Chem., 2021, 45, 16389–16425, 10.1039/D1NJ02560K.
- M. S. Yusubov and V. V. Zhdankin, Iodine catalysis: A green alternative to transition metals in organic chemistry and technology, Resour.-Effic. Technol., 2015, 1, 49–67, DOI:10.1016/j.reffit.2015.06.001.
-
K. Ishihara and K. Muniz, Iodine Catalysis in Organic Synthesis, John Wiley & Sons, ltd, New York, 2022, 978-3-527-82956-9 Search PubMed.
- M. Hancock and A. Pinhas, A convenient and inexpensive conversion of an aziridine to an oxazolidinone, Tetrahedron Lett., 2003, 44, 5457–5460, DOI:10.1016/S0040-4039(03)01325-X.
- Y. Wu and G. Liu, Organocatalyzed cycloaddition of carbon dioxide to aziridines, Tetrahedron Lett., 2011, 52, 6450–6452, DOI:10.1016/j.tetlet.2011.09.092.
- X.-C. Chen, K.-C. Zhao, Y.-Q. Yao, Y. Lu and Y. Liu, Synergetic activation of CO2 by the DBU-organocatalyst and amine substrates towards stable carbamate salts for synthesis of oxazolidinones, Catal. Sci. Technol., 2021, 11, 7072–7082, 10.1039/D1CY01298C.
- D. Heldebrant, P. Jessop, C. Thomas, C. Eckert and C. Liotta, The Reaction of 1,8-Diazabicyclo[5.4.0]undec-7-ene (DBU) with Carbon Dioxide, J. Org. Chem., 2005, 70, 5335–5338, DOI:10.1021/jo0503759.
- T. Weidlich and B. Kamenická, Utilization of CO2-Available Organocatalysts for Reactions with Industrially Important Epoxides, Catalysts, 2022, 12, 298–340, DOI:10.3390/catal12030298.
- O. Ihata, Y. Kayaki and T. Ikariya, Synthesis of Thermoresponsive Polyurethane from 2-Methylaziridine and Supercritical Carbon Dioxide, Angew. Chem., Int. Ed., 2004, 43, 717–719, DOI:10.1002/anie.200352215.
- O. Ihata and Y. Kayaki, Aliphatic Poly(urethane-amine)s Synthesized by Copolymerization of Aziridines and Supercritical Carbon Dioxide, Macromolecules, 2005, 38, 6429–6434, DOI:10.1021/ma050549o.
- K. Lamb, I. Ingram, M. North and M. Sengoden, Valorization of Carbon Dioxide into Oxazolidinones by Reaction with Aziridines, Curr. Green Chem., 2019, 6, 32–43, DOI:10.2174/2213346106666190321142328.
|
This journal is © The Royal Society of Chemistry 2023 |
Click here to see how this site uses Cookies. View our privacy policy here.