DOI:
10.1039/D3CC01355C
(Highlight)
Chem. Commun., 2023,
59, 6956-6968
Recent progress in metal complexes functionalized nanomaterials for photodynamic therapy
Received
20th March 2023
, Accepted 2nd May 2023
First published on 3rd May 2023
Abstract
Metal complexes have shown promise as photosensitizers for cancer diagnosis and therapeutics. However, the vast majority of metal photosensitizers are not ideal and associated with several limitations including pharmacokinetic limitations, off-target toxicity, fast systemic clearance, poor membrane permeability, and hypoxic tumour microenvironments. Metal complex functionalized nanomaterials have the potential to construct multifunctional systems, which not only overcome the above defects of metal complexes but are also conducive to modulating the tumour microenvironment (TME) and employing combination therapies to boost photodynamic therapy (PDT) efficacy. In this review, we first introduce the current challenges of photodynamic therapy and summarize the recent research strategies (such as metal coordination bonds, self-assembly, π–π stacking, physisorption, and so on) used for preparing metal complexes functionalized nanomaterials in the application of PDT.
1. Introduction
About 100 years ago, Tappeiner determined that the phenomenon of PS combined with light-killing cells was oxygen dependent, and proposed the concept of the photodynamic effect for the first time to describe this oxygen-dependent photosensitization reaction.1,2 The emergence of this concept has attracted extensive attention from scientists and researchers and has greatly promoted the development of PDT.3 Nowadays, PDT has been widely used in the treatment of many diseases4–8 including malignant tumours9,10 due to its minimal invasiveness, temporospatial specificity, and controllable systemic toxicity. Typically, the mechanism of photodynamic action is complex and is not fully understood. With light stimulation, the PS molecule transits to the triplet excited state via intersystem crossing, entering into a photochemical reaction of type I or II PDT (Fig. 1(B)). In type I, excited PS molecules interact directly with TME-related biomolecules, forming intermediate radical products that then react with oxygen, which leads to the formation of various highly active substances, primarily active forms of oxygen, for further redox reactions. In this case, peroxide radicals (˙OOH), superoxide anions (˙O2−), and hydroxyl radicals (˙OH) are formed, lipid peroxidation is activated, and cell membranes are damaged which interferes with their functions. In type II, excited PS molecules react first with oxygen, converting it into highly active singlet oxygen (1O2). It interacts with the proteins, nucleic acids, and lipids of cell membranes, causing their death by necrosis or apoptosis.11
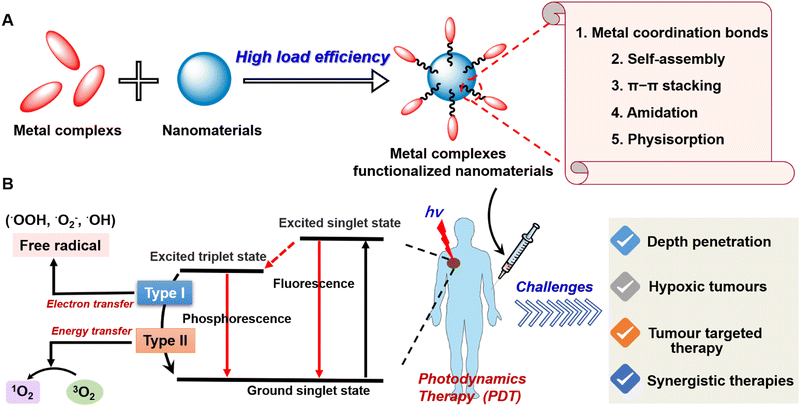 |
| Fig. 1 (A) The framework of metal complexes functionalized nanomaterials. (B) The photophysical mechanism and existing challenges of photodynamic therapy. | |
Photofrin was the first PS to receive approval all over the world for the treatment of cancer. Subsequently, 5-aminolevulinic acid (Levulan), Temoporfin (Foscan), Verteporfin (Visudyne), Telaporfin (Foscan), LUZ111 (Redaporfin) etc. appeared successively and acted as PSs for use in clinical PDT. Owing to their shared structural features, the majority of these PSs have similar drawbacks, including tedious synthesis/purification, poor water solubility and photostability, poor cancer selectivity and slow body clearance causing photosensitivity,12,13 which significantly reduces ROS generation efficiency during PDT processes. Thus, there is an urgent need for the improvement of these compounds and the development of novel PS scaffolds.
With the unremitting efforts of researchers, many metal complexes have started to be reported as PSs in various treatments, including PDT.14 Studies have found that many metal complexes possess excellent properties in the fields of therapeutics and bioimaging, including structural flexibility, excellent photostability, large Stokes shifts, long-lived emission, high 1O2 yield, large two-/multi-photon absorption, remarkable cellular uptake, and organelle-targeting properties, attracting much attention.15–19 For instance, commonly used metal complexes-based PSs in clinical development PDT include metal (Sn, Lu, Pd)-containing tetrapyrrolic PSs20–23 and the ruthenium polypyridine complex TLD-1433.24 Moreover, most organic molecules (methylene blue, protoporphyrin IX, chlorin e6 etc.) have been reported as PSs, which are advantageous for PDT due to their generating a high ROS yield. However, the therapeutic effect of organic PSs for PDT is much lower than that of metal PSs, which is mainly attributed to the complex synthesis, low yield, poor structure stabilization and short fluorescence lifetime of organic PSs.25,26 In a word, these PSs are hydrophobic, lack targeting, and have low bioavailability, so PSs in vivo are prone to self-aggregation, fluorescence quenching, fast systemic clearance and poor membrane permeability, and even because of the hypoxic tumour microenvironment, they usually fail to achieve the desired antitumor effect of PDT.27–29 In recent years, with the development of nanotechnology, supramolecular nanoparticles,30–32 liposomes,33–35 metal–organic frameworks,26,36 and 2D nanosheets,37–39 have been widely used for improving the safety and effectiveness of PSs, capitalizing on their advantages of high biocompatibility, effective targeting, controlled release, and improved pharmacokinetic effect. Based on this, metal complexes functionalized nanomaterials can possess the properties of metal complexes and nanoparticles or new functions. In particular, they have the enormous advantage of increasing solubility, prolonged circulation, accumulation in the tumour site, overcoming tumour hypoxia, enhancing ROS yield, minimizing off-target toxicity to healthy cells and increasing therapeutic efficacy. Hence, it is increasingly evident that new nanomedicines for PDT should overcome the limitations of traditional metal PSs, such as poor water solubility and low efficacy in hypoxic tumours, using novel strategies.
In this review, we first elucidate the challenges of photodynamic therapy and related solutions to the strategy. Moreover, novel design strategies such as metal coordination bonds, self-assembly, π–π stacking, physisorption, and so on to construct metal complexes functionalized nanomaterials for enhancing PDT are also introduced in this review (Fig. 1). Finally, the expected future direction of research into metal complexes functionalized nanomaterials is also discussed.
2. Challenges of photodynamic therapy
Compared to other treatment methods, PDT presents several advantages over conventional therapies because it enables the selective destruction of tumour tissues. This promising therapy combines three components: photosensitizers, light, and oxygen; however, the lack of synergy between these is generally considered to be the main factor causing PDT inefficiency. Compared to existing standard cancer treatments such as surgery or chemotherapy, PDT faces the following challenges, which require unique strategies to overcome.
2.1 Light-penetration depth: short wavelength excitation limits the depth of tissue penetration and damages healthy tissue
Light penetration depth into the skin is one of the preconditions for a PDT procedure. Ideally, a PDT treatment should only cause a therapeutic effect towards cancer tissue while not damaging healthy, underlying tissue. Thus, the wavelength used in a medical procedure is chosen based on the tumour's depth and shape. However, one of the disadvantages of PDT is the short penetration depth of incident light. Generally, the UV-visible light used for activating the photosensitisers has a penetration of about 1–5 mm, and the NIR light-based lasers can penetrate up to 1 cm tissue depth.40 As such, some of the specific nanotechnology, such as upconversion nanotechnology has been developed for converting the lower energy NIR light to UV-vis light to activate the loaded photosensitisers and dyes, including metal complexes for a higher penetration depth.41 Even so, the short penetration depth of the NIR light hindered the application of PDT in deep-seated tumours.
To overcome the limited penetration depth in traditional PDT systems, several strategies have been applied. (1) Light transducers can be used as energy amplifiers, which can absorb light in the NIR region and emit it in the visible region, thereby activating PSs in the vicinity.42 Such examples can be found in two-photon light in addition to up-conversion light as mentioned above.43,44 (2) Bioluminescence resonance energy transfer (BRET) systems combining bioluminescent luciferase and quantum dots (QDs) could allow for in situ production of light and internal activation of the PSs because of the unique optical properties of QDs.45 (3) X-ray as a light source has shown great promise in PDT applications with no tissue penetrating limitations. This strategy employs nano scintillators to convert X-rays into visible light and in turn activates the nearby PSs.46 (4) Ultrasound (US) as a mechanical wave with a frequency beyond human hearing (>20 kHz) can penetrate deep soft tissues up to 10 cm due to the unique advantage of minimal tissue scattering, offering considerable potential for activating sonosensitizers in deep tissues.47 Among the many strategies, metal complexes functionalized nanomaterials have been widely reported for two-photon PDT (TP-PDT) using NIR light irradiation with a deep tissue penetration depth. The Mao group reported a nanohybrid Ru1@CDs composed of CDs modified with a Ru(II) complex (Ru1, 1, Fig. 3) for lysosome-targeted imaging and PDT. Ru1@CDs can photoinduce ROS generation to the lysosomal membrane to obtain one- and two-photon PDT therapy in 2D cells and a 3D multicellular tumour spheroid (MCTS). Using the two-photon characteristics, their in vivo toxicity and capacity for deep imaging were investigated using a zebrafish model.48
2.2 Hypoxic tumours: low oxygen level hampers the generation of therapeutically required ROS
A hypoxic tumour core is one of the main hallmarks of solid tumours.49–51 This tumour microenvironment generally results from the imbalance between the uptake and consumption of oxygen, mainly due to the development of abnormal tumour blood vessels, poor blood flow, and the intensified proliferation of cancer cells.40–49 Statistically, O2 concentrations vary with location in solid tumours, with some interior regions having very low levels (partial pressures of O2 < 5 mmHg, corresponding to 7 μm).50,51 One of the intrinsic limitations of PDT treatments toward aggressive and/or drug-resistant tumours is the low oxygen concentration in hypoxic areas. From the analysis of the action mechanism of PDT, since the more common operation is the type II pathway, most existing PDT systems are highly O2-dependent and involve a dramatic consumption of O2. Based on this, hypoxic solid tumours severely reduce the effectiveness of type II PDT in clinical tumour treatment. Therefore, effective tumour oxygenation is of great significance to promoting this anti-cancer method. To generate a phototoxic effect despite hypoxic conditions, different strategies have been developed to enhance PDT under hypoxia, including (1) reducing the oxygen consumption rates of tumours, (2) O2-evolving synergistic chemoradiotherapy, (3) artificial blood to transport oxygen to the hypoxic core and (4) combining oxygen-enriched gases with vasodilators.52–55
Besides regulation of the oxygen concentration at the tumour site, research efforts have also been devoted to the development of PSs which act by an oxygen-independent mechanism, also referred to as the remote-controlled release of 1O2. Aromatic compounds including naphthalene, anthracene, etc., which capture and store singlet oxygen to generate endoperoxides (EPOs),56 have been widely investigated and employed in material science57 and chemical synthesis.58 After light irradiation or thermal reduction, the physical quenching pathway through intersystem crossing enables the exciplexes to transform into excited triplet complexes, ultimately generating reactive radical products before decomposition into starting materials. A novel Ir(III) complex EPOs (2-O-IrAn, 2, Fig. 3) was recently investigated by the Chao group.59 2-O-IrAn includes an endoperoxides anthracene as the 1O2 supplier, overcoming the problem of PDT in hypoxia. Interestingly, upon two-photon irradiation under hypoxia, 2-O-IrAn was a photosensitizer with low cytotoxicity and released the trapped 1O2 with the generation of highly cytotoxic 2-IrAn and alkoxy radicals. Overall, 2-O-IrAn exhibited mitochondria targeting synergistic PDT/PACT effects to treat solid tumours in a mouse model. Besides the generation of 1O2 or ROS, other types of radical species could also be generated upon light irradiation, including carbon radicals,60 chlorine radicals,61 nitric oxides,62 or sulfate radicals.63 The discovery of potent oxygen-independent mechanisms of action for PDT under hypoxic tumour microenvironment-relevant conditions is ongoing. Using the above strategies to overcome hypoxia will be very beneficial for photodynamic therapy under hypoxia in the future.
2.3 Tumour targeted therapy: avoiding toxic side effects by tumour selective delivery of the photosensitizer
Despite the many positive features of PDT cancer therapy, this form of treatment is still not always fully adapted to clinical settings.64 On the one hand, most PSs often suffer from poor solubility and cancerous affinity, which leads to difficult administration and unsatisfying tumour accumulation.65,66 Generally, conventional PSs rely heavily on passive accumulation into tumour sites (due to EPR). On the other hand, the remainder of the PSs inevitably accumulating in the tumour's surrounding tissue would also be exposed to light irradiation due to light scattering effects as well as practical challenges to irradiate only the tumour site, causing systemic phototoxicity and immunological barriers destruction.67 Additionally, with only a short half-life, the radius of action of 1O2 is at a small extent (≤0.02 μm).68 This means only ROS generated in the vicinity of PS directly affects cancerous tissue. Based on this point, the overall extent of PDT-induced cytotoxicity and photodamage is highly dependent on the PSs’ bioavailability, as well as their extracellular and intracellular localization.69
To solve the above issues, efforts have been made to develop various drug delivery vehicles to improve the accumulation of PS in tumours, the main strategies include active or passive tumour targeting. For an active tumour targeting strategy, the specific interaction of a targeting moiety with cancer cells is utilized, such as antibodies,70 oligosaccharides,71 oligonucleotides,72 proteins,73 vitamins,74 and signal peptides.75 A passive tumour targeting approach mainly adopts nanocarriers such as inorganic nanoparticles,76 micelles,77 polymeric nanoparticles,78 liposomes79 or metal–organic frameworks28,36 to deliver PSs into tumours, capitalizing on the leaky, permeable vasculature and lymphatic properties of the tumour tissue known as the enhanced permeability and retention (EPR) effect. However, the high internal osmotic pressure in solid tumours also leads to a greater distribution of nanomedicines at the tumour periphery.80 These natural barriers seriously limit the extravasation of cytotoxic nanomedicines into the tumour tissues by passive transport. Despite the fact that extravasation of nanomedicine into the tumour through the EPR effect has been widely regarded as an advantage for the successful targeted delivery of nanomedicines into tumours,81 the low success rate of clinical translation of nanomedicine in cancer therapy causes researchers to begin to question the contribution of the EPR effect in the treatment of tumors.82
Besides, from the literature, we know that surface potential, particle size, and targeted polypeptide modification affect cellular distribution.83 Lipophilic cations accumulate in the mitochondria and the nucleus. Most nuclear-targeted nanoparticles are less than 50 nm in diameter. These factors together affect the distribution of nanoparticles in cells. It is worth mentioning that metal complexes have structural flexibility and possess the above conditions, as organelle-targeted PSs are also prevalent fields of research.84 It follows that the rational design of metal complexes and nanomaterials with controlled size has important meaning for organelle precisely targeted therapy. Furthermore, the metal complex functionalized with nanoparticles not only acts as a photosensitizer for one- and two-photon PDT but also as an organelles-targeting and imaging agent for tracking the NPs. Meanwhile, the nanosystems can effectively induce organelle dysfunction via organelles-targeting to enhance the sensitivity of PSs intracellular tumour, eventually triggering a combination of multiple cell death pathways. In a typical example, Chao's group designed a self-assembly of thiol-functionalized Ir(III) complexes into biodegradable coordination polymeric nanoparticles (IrS NPs, 3, Fig. 3).85 The nanomaterials were truncated by intracellular GSH and released the molecular Ir(III) complexes with mitochondrial-targeting and decreased endogenous GSH levels, causing amplifying oxidative stress in the cell. Upon two-photon irradiation, IrS NPs can generate a mixture of 1O2 and superoxide anion radicals (˙O2−) and induce cell death by a combination of apoptosis and ferroptosis pathways.
2.4 Synergistic therapies: overcoming the limitations of a single therapeutic method for PDT
Admitting that PDT as a temporospatial-selective and minimal-invasive modality plays an important role in clinical cancer therapeutics, the therapeutic efficacy of PDT alone against several deep or hypoxic solid tumours is limited due to its inherent drawbacks and the clinical challenges of metastasis, recurrence, and resistance of cancer therapeutics.86,87 The main mechanisms of PDT resistance include the limited penetration of the therapeutic light source, the monotonous activation of signalling pathways, and inadequate efficacy of oxygen-independent phototoxicity. To optimize this, much research effort has been dedicated to the development of PDT-combined strategies. Several studies have reported on the combination of PDT with photothermal therapy,88 sonodynamic therapy,89 chemodynamic therapy,90 chemotherapy,91 starvation therapy,92 radiotherapy93 or immunotherapy,94 and gas therapy.95 From this perspective, by combining PDT with other current cancer modalities, one may be able to exploit the strengths and bypass the weaknesses of different therapies to achieve the goal of mutual benefit (Fig. 2). Moreover, the combination of PDT with imaging techniques has always been the focus of research, which is attributed to precise discrimination between the cancerous and the healthy tissue by using imaging-guided theranostics. The ongoing efforts on the combination of PDT with luminescent imaging,96 positron emission tomography,97 magnetic resonance imaging,98 computed tomography,99 photoacoustic imaging,100 and ultrasonography101 are promising in clinical trials in the future.
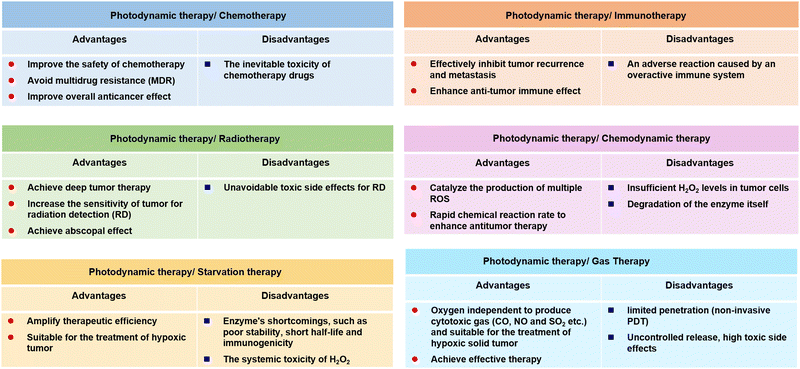 |
| Fig. 2 The advantages and disadvantages of synergistic therapies containing PDT. | |
3. The design strategies of metal complexes functionalized nanomaterials for PDT
It is no secret that currently the use of nanotechnology is gaining in popularity and is usually easily able to form composite nanosystems with other substances, including metal complexes, molecular drugs, organic probes, etc.30,102 Expressly, metal complexes functionalized nanomaterials containing nanocarriers and metal complexes PSs have been mostly reported. Metal complexes can modulate the surface charge and photophysical properties of nanoparticles to improve ROS generation, achieving better PDT efficacy. Many nanocarriers have excellent biocompatibility but produce negligible ROS production. Thus, using a combination of a metal complex with nanoparticles can overcome some deficiencies between the metal complex and nanoparticles. Determining how to provide effective strategies to construct metal complexes functionalized nanomaterials is the focus of this section.
3.1 Metal coordination bonds-based nanomaterials
Currently, there are two typical strategies for the fabrication of metal coordination bonds-based nanomaterials, including (1) M–N coordination bonds between metal ion/metal complex precursors and nanomaterials and (2) M–O coordination bonds between metal complex PSs and nanomaterials. These nanomaterials can not only extend the blood circulation time of metal complex PSs and prevent drug leakage but can also enhance PDT by the synergism of nanomaterials and metal complexes in photophysical modulation, achieving the antitumor effect of 1 + 1 > 2. As a typical example, Chao's group reported the first case of an oxygen self-sufficient photosensitizer (4, Fig. 3) produced via grafting a metal complex precursor ([Ru(bpy)2]2+) onto g-C3N4, according to Ru–N bonding (Fig. 4).103 In this system, the g-C3N4 frame acts as a N^N ligand coordinated with the metal center of [Ru(bpy)2]2+ to obtain stable nano-PSs (Ru-g-C3N4). The PS show high loading capability, good biocompatibility, and high stability, and can catalyze the O2 generation from H2O2 or H2O in a hypoxic tumour under visible light irradiation, simultaneously producing multiple cytotoxic ROS (˙OH, ˙O2−, and 1O2). Finally, nano-PSs have red-shifted luminescence which reduces the interference of biological background emission allowing precisely guided PDT to reduce the potential side effects of irradiation. Unfortunately, the nanomaterials are limited to visible light excitation, resulting in poor penetration of deep tissue. To address the problem, the group also developed functionalization of graphitic carbon nitride nanosheets with mitochondria-targeting Ir(III) complexes (5, Fig. 3) for oxygen self-sufficient two-photon PDT.104 This strategy can not only solve the above problems but also improve the efficacy of two-photon PDT in melanoma tumour models.
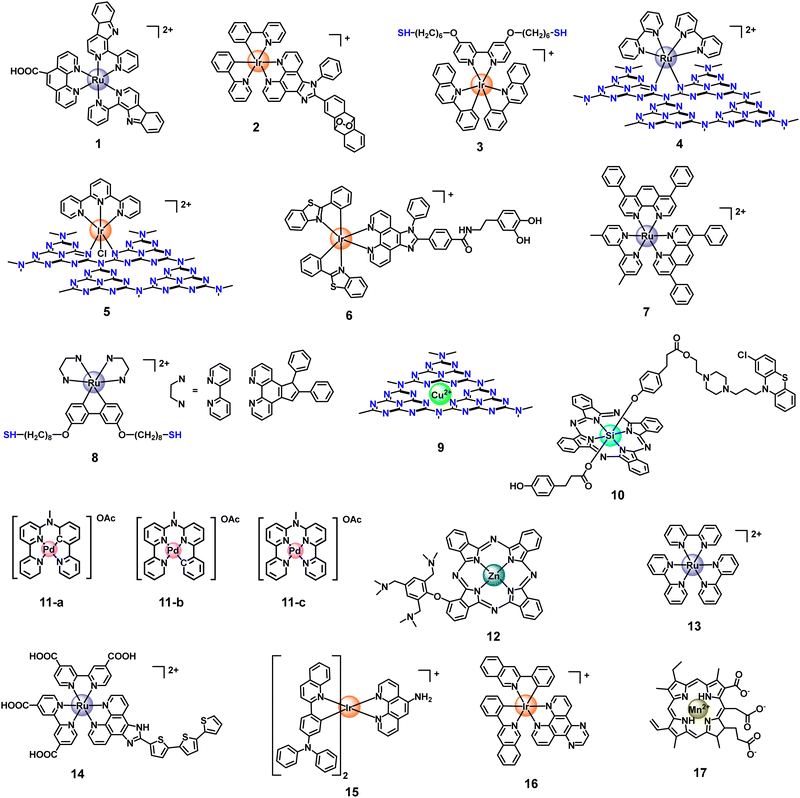 |
| Fig. 3 Structures of Ru, Ir, Cu, Si, Pd, Zn, and Mn-based metal complexes as metal photosensitizers. | |
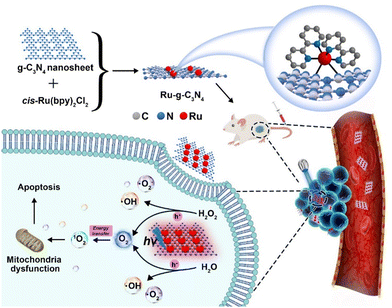 |
| Fig. 4 A schematic illustration of an oxygen self-sufficient photosensitizer (Ru-g-C3N4) with activated multiple ROS (˙OH, ˙O2−, and 1O2) for the efficient photodynamic therapy of hypoxic tumours. Figure adapted from ref. 103 Copyright: 2021, with permission from Elsevier. | |
The second strategy is with the M–O coordination bond, which is excellent in anti-tumour synergistic therapy. We believe that synergistic therapeutic formulations can make up for the deficiency of PDT in anti-tumour treatment, especially oxygen-dependent PDT. Chao et al. prepared a functionalization of black-titanium nanoparticles with iridium complexes (6, Fig. 3) proposed by Ti–O bond,105 which were further encapsulated with cancer cell membranes to perform hierarchical-targeted synergistic photothermal and sonodynamic cancer therapy. Despite the use of ultrasonic and near-infrared-II (NIR-II) region light to improve the light penetration depth, the load rate of the photosensitizer needs to be further improved.
Except for the strategy based on metal coordination bonds between metal complexes and nanomaterials, using metal cations coordinated on nanomaterials to form metal coordination bonds-based nanomaterials has also been the focus of research in recent years.106–108 Classically, Qu's group previously reported the coordinate integration of Cu2+ and g-C3N4 nanosheets (Cu2+-g-C3N4) (9, Fig. 3), rendering improvement in light-triggered ROS generation as well as the depletion of intracellular GSH levels.109 In this system, the author verified the generation of redox-active species Cu+-g-C3N4 under illumination, catalyzing the reduction of O2 to ˙OH or ˙O2−, both of which facilitated the generation of ROS to enhance the efficiency of photodynamic therapy. Unfortunately, the system had not been further verified in vivo, hampered by the short excitation wavelength. Moreover, single-atom catalysts (SACs) have become one of the hottest subjects of research. SACs with distinct properties, such as precisely located metal centres, identical coordination environments, tailorable composition, and structure, can induce multiple ROS (˙OH, ˙O2−, and 1O2) generation via catalyzing H2O2 decomposition, improving PDT in the tumour microenvironment, especially hypoxic tumours.110,111 Recently, inspired by the catalase-like activities of Ru nanoparticles, Wang et al. developed a multifunctional OxgeMCC-r SAC for efficiently degrading H2O2 to O2 (Fig. 5).112 The OxgeMCC-r SACs with coordination environment and unique structure of six unsaturated Ru–C6 coordination sites can endow excellent catalytic activity in the decomposition of H2O2 with the atomic economy and superior stability, which could highly relieve the hypoxic microenvironment of solid tumours, thus facilitating subsequent photocatalytic 1O2 generation, and finally augmenting the PDT efficacy.
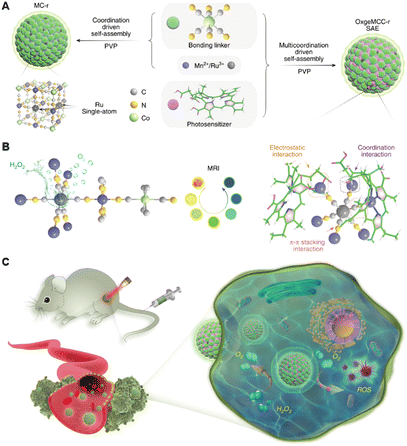 |
| Fig. 5 A schematic Illustration of the synthesis of OxgeMCC-r SAE and mechanism of action of catalyzing oxygen generation and ROS production for enhanced PDT of cancer by OxgeMCC-rSAE. Figure adapted from ref. 112 Copyright: 2020, with permission from Springer Nature. | |
Constructed via coordination bonds between metal cluster secondary building units (SBUs) and bridging ligands, nanoscale metal–organic frameworks (nMOFs) have emerged as a new class of hybrid materials with tunable, crystalline, and porous structures. In recent years, nMOFs have been identified as the most promising nano-PSs. Compared to other nano-PSs, the porous and crystalline structures of nMOFs isolate PSs from each other to avoid self-quenching of PS excited states. As a result, nMOFs can deliver porphyrin, chlorin, and bacteriochlorin PSs for PDT without suffering from self-quenching.113–115 The biodegradability of nMOFs alleviates long-term toxicity, whereas tunable compositions and structures allow the optimization of nMOF nano-PSs for PDT applications. In 2014, Lin et al. first reported a Hf-porphyrin nMOF, DBPHf, as a highly effective photosensitizer for PDT of resistant head and neck cancer.116 nMOFs as PSs have been successively reported for PDT in recent years,28,117,118 but nMOFs have not been explored in depth for PTT monotherapy or other combined therapies.
In brief, metal coordination bonds-based nanomaterials have structural stability and great advantages in anti-hypoxic solid tumour PDT and combination with multiple therapeutic modalities.
3.2 Self-assembling nanomaterials
Self-assembling nanoparticles based on metal complexes deploy a simple yet powerful strategy to construct high-loading rate nanomaterials with improved bioavailability. Aggregates of metal complexes could be effectively conveyed to biological targets while being protected from irrelevant chemical and/or biological degradation. The one strategy is mainly to form nanoparticles through the S–S bond, Se–Se bond, phenyl borate lipid and dithioacetals structure and so on, which can prevent the leakage of photosensitizers or drugs in the drug delivery mechanism and realize the purpose of the most accurate possible release of tumours. Notably, the transformation from nanoparticles to molecules (PSs) by GSH or ROS-responsive controlled release fully uncages the PDT performance of nano-polymers.119–121 Based on this point, Chao's group designed a nano-assembled photosensitizer based on thiol-tailed Ru(II) complexes (8, Fig. 3) linked by disulfide bonds for the two-photon PDT of cancer.122 Xiao's group reported a nano-polymer (NP(Se)s) from the self-assembly of lipophilic Pt(IV) prodrugs and polymer P1 with Se–Se bonds in its main chain for redox-based therapy.123 Furthermore, using intermolecular interactions to form supramolecular self-assembling nano-polymers could deliver high concentrations of a drug to cancer cells by providing a high drug-loading capacity. Che and Pan reported that the cyclometalated platinum/gold/ruthenium complex forms supramolecular self-assembly complexes via intermolecular interactions, producing an increase in drug efficacy.124–127
Another self-assembling strategy is self-assembled polymeric micelles or liposomes with amphiphilic structures for hydrophobic photosensitizers loaded into the hydrophobic core space in aqueous solution. Biocompatible phospholipids or polymers (such as F127 and DSPE-PEG2000et al.)128,129 used in self-assembly dissociate from cargo metal complexes after being endocytosed into the target cells and offer no severe toxicity by themselves. For instance, Gasser's group reported a nanoparticle from the encapsulation of a PSs (Ru(II) polypyridine complex, 7, Fig. 3) in an amphiphilic polymer with terminal folate groups (DSPE-PEG2000-folate) for PDT.130 The authors found that the Ru(II) complex itself has a cytotoxic effect in the dark, but this drawback was avoided after the encapsulation of polymers. Meanwhile, DSPE-PEG2000-folate provides targeting cancer cells, increasing to more than 20 times higher accumulation of the Ru(II) complexes in cancer cells. Upon 480 or 595 nm irradiation, the nanoparticles were found to generate ROS and showed a high phototoxicity effect in the very low micromolar range in 2D monolayer cancer cells and 3D multicellular tumour spheroids. This interesting work is limited to the cellular level attributed to the limited light-penetration depth.
Moreover, researchers found that the polymeric chains could self-assemble into nanoparticles during the transformation process of the aqueous phase. Wei et al. designed a novel type of nucleus-targeting Pt(IV) nanoparticles based on a polymeric chain, which contained a chemotherapeutic agent (oxaliplatin), a photosensitizer with aggregation-induced emission (AIE), and cancerous tissue/nucleus targeting peptides R8K.131 Within an aqueous solution, the functionalized polymer chains self-assemble into spherical nanoparticles. Upon irradiation, the Pt(IV) centre is reduced to Pt(II) and axially coordinated ligands are released. The PS catalytically generates reactive oxygen species (ROS) causing immunogenic cell death (ICD) and presenting a multimodal treatment by chemotherapy and photodynamic immunotherapy. Using a self-assembling strategy to form novel nano-PSs, which need straightforward fabrication procedures, is a method for preparing the highest PSs load rate reported at present. Based on this, only a minimal dose of PS is required to maximize antitumor activity during the PDT process.
3.3 π–π stacking-based nanomaterials
It has been widely recognized that non-covalent interactions between aromatic moieties, which are habitually called π–π stacking interactions, could play a vital role in a wide variety of chemical systems.132 π–π stacking interactions refer to the interactions between aromatic rings containing π orbitals.133 Most metal complexes containing ligand structures of aromatic rings, porphyrins, phthalocyanines and their derivatives, regarded as photosensitizers have large conjugated planar aromatic structures, which have also rarely been reported in self-assembly with nanoparticles by π–π stacking interactions in a crowded environment. This not only can maintain the photosensitivity of the original metal complex but can also change the physical properties of the material to enhance the antitumor effect during the PDT process. Classically, Bonnet's group prepared supramolecular nanorods, which utilize monocationic cyclometalated palladium complexes OAc (N^N^C^N) and OAc (N^N^N^C) (11, Fig. 3) via metallophilic Pd⋯Pd interactions and π–π stacking to self-assemble in aqueous solutions (Fig. 6).134 The authors found that the nanorods improved cellular uptake of the cyclometalated compounds by endocytosis (i.e., an active uptake pathway). Under blue light irradiation, a better singlet oxygen generation ability was achieved, leading to dramatically enhanced photodynamic properties in multicellular tumour spheroids and in mice tumour xenografts. However, the short wavelength blue light used in the PDT process limited light penetration to the tissue and the antitumor activity of the photosensitizer must not be significantly weakened.
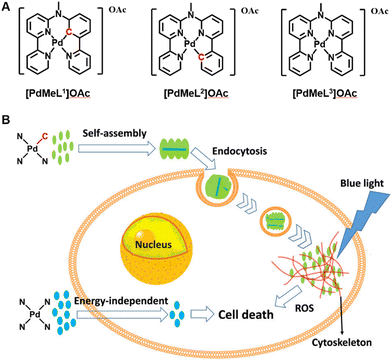 |
| Fig. 6 A schematic illustration of (A) structures of the Pd complexes and (B) synthesis and mechanism of supramolecular nanorods via π–π stacking of Pd complexes to enhance photodynamic therapy under blue light. Figure adapted from ref. 134 Copyright: 2020, with the permission from American Chemical Society. | |
Meanwhile, for PSs molecules, the π–π stacking strategy can make up for the deficiency of traditional PSs, such as poor water solubility, low biocompatibility and highly efficient vibrational relaxation of their electronic excited states. For example, Yoon et al. developed the nanostructured Zn(II) phthalocyanine (12, Fig. 3) assembly (NanoPcA), which serves as a PS for efficient ROS generation via the type I mechanism. In their study, Zn(II) phthalocyanine monomers aggregate to form molecular clusters via mutual diffusion of water in DMF, furtherly ensuing growth through π-stacking. In biological activity studies, NanoPcA was found to display excellent photodynamic antibacterial activity against both common and antibiotic-resistant bacterial strains.135 However, this interesting study was not followed up in vivo. Recently, Huang's group prepared a uniform and stable nanosphere (NanoPcAF) through a spontaneous assembly process of Si(IV) phthalocyanine derivatives (10, Fig. 3) bearing perphenazine groups. NanoPcAF displayed an efficient type I photoreaction to generate abundant superoxide radicals and a significant vibrational relaxation to induce a relatively high photothermal conversion efficiency, which enabled it to overcome tumour hypoxia in PDT in vivo.136 This work solves most of the bottlenecks encountered by PDT.
Besides the above method, some multifunctional nanomaterials (such as graphene,137 carbon nanotubes (CNTs),138 and graphite phase carbon nitride (g-C3N4))139 also contain aromatic ring structures, which means they can combine with metal complexes to form a new class of materials by π–π stacking interactions. Among them, Li et al. prepared a two-photon excited nanocomposite FCRH, which is assembled by π–π stacking between [Ru(bpy)3]2+ (13, Fig. 3) and Fe–C3N4, and modified by a copolymer with poly (ethylene glycol) arms (HOP).140 Under 800 nm two-photon irradiation, the nanocomposite can separate charges and prevent the recombination of electron–hole pairs more efficiently to enhance photocatalytic activity and O2 generation. The activated photosensitizer ([Ru(bpy)3]2+) promotes 1O2 generation to enhance two-photon PDT under hypoxia. Although the nano-platform alleviates the hypoxic tumour environment, the PDT efficacy in hypoxic solid tumours is a limiting attribute to single 1O2 generation.
3.4 Amidation-based nanomaterials
Amidation is a readily achievable chemical synthesis process, which can be used to form an amido linkage as a bridge between metal complexes and nanomaterials to enhance PDT and achieve the purpose of controlled release in cells. Zhou et al. constructed a hypoxia-adaptive nanocomposite TiO2@Ru@siRNA for the prevention and treatment of oral squamous cell carcinoma (OSCC) (Fig. 7).141 In this system, the carboxyl Ru complex PSs (14, Fig. 3) and TiO2 NPs surface with aminopropyltriethoxysilane (APTEs) were linked by an amido bond, ulteriorly loading siRNA targeting HIF-1α. Under visible light (525 nm) irradiation, the nanoparticles can produce ROS through both types I and II PDT, causing lysosomal damage to effectively promote siRNA escape and induce pyroptosis of OSCC cells, which activates multifaceted cancer immune responses. Collectively, the author claims that the nanosystem has outstanding PDT performance and immunomodulatory function. However, the PDT effect is still limited by its short wavelength light penetration, which is the problem with most metal photosensitizers.
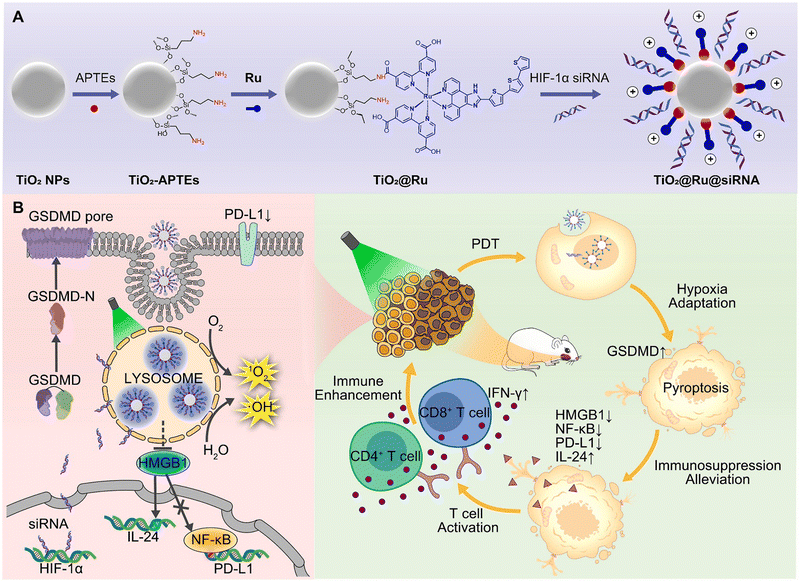 |
| Fig. 7 A schematic illustration of (A) the construction of the nanocomposite TiO2@Ru@siRNA and (B) the action mechanism of TiO2@Ru@siRNA. Figure adapted from ref. 141 Copyright: 2022, with permission from Elsevier. | |
Moreover, as a similar example, Huang's group designed a new type of mesoporous silica-coated lanthanide-doped upconversion nanoparticles, which contain oxygen-sensitive Ir(III) complex (15, Fig. 3) in the outer silica shell.142 An Ir(III) complex and SiO2 NPs were bridged by an amido bond. Regrettably, this nanosystem shows negligible PDT effects but only acts as a nanoprobe, monitoring oxygen concentration by reducing autofluorescence under both downconversion and upconversion channels.
3.5 Physisorption-based nanomaterials
Physisorption, also known as van der Waals adsorption, is caused by the intermolecular force between the adsorbent and the adsorbent, which is also known as the van der Waals force and is weaker than that in the methods mentioned above. Excitingly, physisorption is one of the most common construction methods of metal complexes and nanomaterials, including electrostatic interaction, hydrophobic interaction, polarity interaction and other non-covalent bonding. However, due to the leakage of drugs during nanomaterials drug delivery, the structural stability of this method is still under debate compared to the above strategies.143
Using the lipophilic and cationic properties of metal complexes, the researchers designed nanomaterials with electronegative surfaces, which can combine metal complexes with positive ions to maintain electric neutrality by electrostatic interaction. Based on this point, Gao's group reported a biscyclometalated Ir(III) complex PSs (16, Fig. 3) electrostatically loaded on an MOF decorated with a dual-responsive polycationic polymer to form nanoplatforms (Ir@MOF/P NPs). The endo/lysosome escape ability and the pH & ROS-responsive charge reversal function of Ir@MOF/P NPs are demonstrated to be a promising strategy to improve the PDT performance in vitro (Fig. 8).144 The study was limited to the cellular level because of the limited penetration of single-photon light.
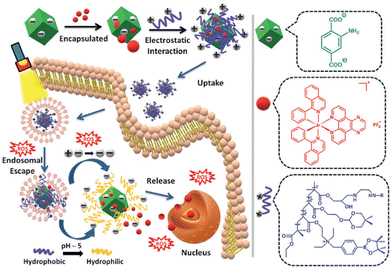 |
| Fig. 8 A schematic illustration of the preparation of Ir@MOF/P NPs and its putative mechanism for intracellular metabolism and ROS-induced tumour inhibition processes. Figure adapted from ref. 144 Copyright: 2020, with the permission from Royal Society of Chemistry. | |
Another strategy is using hydrophobic interaction, which makes hydrophobic groups gather, and avoids burst leakage in the hydrophilic medium, minimizing the non-active surface. Most metal photosensitizers are usually not hydrophilic, the synthesis of metal complexes functionalized nanomaterials are usually dispersed in fat and then transferred to the water phase. This process can adsorb metal complexes into nanomaterials by hydrophobic interaction. For instance, Liu et al.145 proposed pH-responsive CaCO3 nanoparticles with polyethene glycol (PEG) as a multifunctional nano-carrier, following loading both a photosensitizer (Mn2+-chelated chlorin e6) (17, Fig. 3) and a chemotherapy drug (doxorubicin) to form nanoplatforms (CaCO3@Ce6(Mn)-PEG(DOX)). The nanoparticles were found to be highly sensitive to pH and would rapidly degrade under slightly acidic solutions, leading to the efficient release of Ce6(Mn) and doxorubicin (DOX). Under the irradiation of light, the nanoplatforms can execute a synergistic photodynamic chemotherapy of cancer in vivo. Using a CaCO3 nanoparticles loading strategy as a smart tumour-pH responsive drug delivery platform is useful for applications in cancer theranostics.
4. Summary and outlook
Compared to traditional cancer treatments (i.e. surgery, radiotherapy, or chemotherapy), photodynamic therapy has emerged as a complementary medical technique to classical procedures for the treatment of cancer. However, the application of PDT is hindered by classic PSs due to their poor water solubility and poor biocompatibility. In addition, this review discusses some of the challenges facing PDT including light-penetration depth, hypoxic tumour environments, targeting therapy for tumours and synergistic therapy. Although strategies designed by researchers can solve some of these problems, further exploration is needed to realize efficient PDT. The application of nanoparticles, especially metal complexes-based nanoparticles, in PDT is a very promising approach for the imminent breakthrough of classic techniques. The nanoparticles can be applied as carriers of hydrophobic PSs and delivered to the sites of different tumours via the EPR effect, which can handle the off-target effect of metal complexes.
In this review, the recent developments in metal complexes functionalized nanomaterials for PDT are herein presented. The fabrication strategies between nanomaterials and complexes are discussed, including metal complexes metal coordination bonds, self-assembly, π–π stacking, amidation, and physisorption. We believe in using these strategies to aid the judicious choice of complexes and nanomaterials in future nanoformulation design. We envision that the next generation of metal complex functionalized nanomaterials will achieve the optimization of characteristics such as stability of nanoformulations, physicochemical properties, and energy transfer properties. Attention to these factors will aid the development of new nanoplatforms to overcome the challenges of PDT, achieving higher treatment efficiency and outcomes. Furthermore, the biosafety of metal complexes functionalized nanomaterials needs further evaluation. The long-term effects of the nanoformulations, including biocompatibility, pharmacokinetic properties, systemic toxicities, biological metabolic pathways and immunogenicity must be studied in detail in animal experiments before further clinical development and application. Recently, combining PDT with immunotherapy has provided a powerful approach to treating metastatic cancers. As a local treatment, PDT can also produce a strong inflammatory response by inducing immunogenic cell death.146 We expect significant amounts of research into metal complexes functionalized nanomaterials-mediated PDT to induce immunotherapy soon. Thus, we anticipate that metal complexes functionalized nanomaterials will find future applications in the diagnosis and treatment of various diseases for cancer treatment in clinic.
Conflicts of interest
There are no conflicts to declare.
Acknowledgements
This work was supported by the National Natural Science Foundation of China (no. 22120102002 and 21977022). We thank the reviewers for their helpful comments in improving our manuscript.
Notes and references
- D. Dolmans, D. Fukumura and R. Jain, Nat. Rev. Cancer, 2003, 3, 380–387 CrossRef CAS PubMed.
- G. I. Stables and D. V. Ash, Cancer Treat. Rev., 1995, 21, 311–323 CrossRef CAS.
- M. J. Manyak, A. Russo, P. D. Smith and E. Glatstein, J. Clin. Oncol., 1988, 6, 380–391 CrossRef CAS PubMed.
- X. Zhen, P. Cheng and K. Pu, Small, 2019, 15, 1804105 CrossRef.
- S. G. Perlman, L. H. Gerber, R. M. Roberts, T. P. Nigra and W. F. Barth, Ann. Intern. Med., 1979, 91, 717–722 CrossRef CAS PubMed.
- R. Huang, C. Zhang, Y. Bu, Z. Li, X. Zheng, S. Qiu, J. O. A. Machuki, L. Zhang, Y. Yang, K. Guo and F. Gao, Biomaterials, 2021, 277, 121088 CrossRef CAS PubMed.
- E. H. C. van Dijk, S. Fauser, M. B. Breukink, R. Blanco-Garavito, J. M. M. Groenewoud, J. E. E. Keunen, P. J. H. Peters, G. Dijkman, E. H. Souied, R. E. MacLaren, G. Querques, S. M. Downes, C. B. Hoyng and C. J. F. Boon, Ophthalmol., 2018, 125, 1547–1555 CrossRef PubMed.
- Y. Dong, W. Cao and J. Cao, Nanoscale, 2021, 13, 14591–14608 RSC.
- J. Nam, S. Son, L. J. Ochyl, R. Kuai, A. Schwendeman and J. J. Moon, Nat. Commun., 2018, 9, 1074 CrossRef PubMed.
- X. Liang, M. Chen, P. Bhattarai, S. Hameed and Z. Dai, ACS Nano, 2020, 14, 13569–13583 CrossRef CAS PubMed.
- Y. Wu, S. Li, Y. Chen, W. He and Z. Guo, Chem. Sci., 2022, 13, 5085–5106 RSC.
- B. Myrzakhmetov, P. Arnoux, S. Mordon, S. Acherar, I. Tsoy and C. Frochot, Pharmaceuticals, 2021, 14, 138 CrossRef CAS PubMed.
- X. Zhao, J. Liu, J. Fan, H. Chao and X. Peng, Chem. Soc. Rev., 2021, 50, 4185–4219 RSC.
- L. Zeng, P. Gupta, Y. Chen, E. Wang, L. Ji, H. Chao and Z.-S. Chen, Chem. Soc. Rev., 2017, 46, 5771–5804 RSC.
- S. Monro, K. L. Colón, H. Yin, J. Roque, III, P. Konda, S. Gujar, R. P. Thummel, L. Lilge, C. G. Cameron and S. A. McFarland, Chem. Rev., 2019, 119, 797–828 CrossRef CAS PubMed.
- K. K.-W. Lo, M.-W. Louie and K. Y. Zhang, Coord. Chem. Rev., 2010, 254, 2603–2622 CrossRef CAS.
- K. Qiu, H. Zhu, T. W. Rees, L. Ji, Q. Zhang and H. Chao, Coord. Chem. Rev., 2019, 398, 113010 CrossRef.
- E. Meggers, Angew. Chem., Int. Ed., 2017, 56, 5668–5675 CrossRef CAS PubMed.
- J. Shen, T. W. Rees, L. Ji and H. Chao, Coord. Chem. Rev., 2021, 443, 214016 CrossRef CAS.
- F. Heinemann, J. Karges and G. Gasser, Acc. Chem. Res., 2017, 50, 2727–2736 CrossRef CAS PubMed.
- A. E. O’Connor, W. M. Gallagher and A. T. Byrne, Photochem. Photobiol., 2009, 85, 1053–1074 CrossRef PubMed.
- R. Baskaran, J. Lee and S.-G. Yang, Biomater. Res., 2018, 22, 25 CrossRef PubMed.
- P.-C. Lo, M. S. Rodríguez-Morgade, R. K. Pandey, D. K. P. Ng, T. Torres and F. Dumoulin, Chem. Soc. Rev., 2020, 49, 1041–1056 RSC.
- J. Karges, Angew. Chem., Int. Ed., 2022, 61, e202112236 CrossRef CAS PubMed.
- R. Bonnett, Chem. Soc. Rev., 1995, 24, 19–33 RSC.
- A. Kamkaew, S. H. Lim, H. B. Lee, L. V. Kiew, L. Y. Chung and K. Burgess, Chem. Soc. Rev., 2013, 42, 77–88 RSC.
- E. Villemin, Y. C. Ong, C. M. Thomas and G. Gasser, Nat. Rev. Chem., 2019, 3, 261–282 CrossRef CAS.
- Y. Ye, Y. Zhao, Y. Sun and J. Cao, Int. J. Nanomed., 2022, 1, 2367–2395 CrossRef PubMed.
- C.-N. Ko, G. Li, C.-H. Leung and D.-L. Ma, Coord. Chem. Rev., 2019, 381, 79–103 CrossRef CAS.
- M. Abbas, Q. Zou, S. Li and X. Yan, Adv. Mater., 2017, 29, 1605021 CrossRef PubMed.
- Y. Li, Y. Wang, G. Huang and J. Gao, Chem. Rev., 2018, 118, 5359–5391 CrossRef CAS PubMed.
- C. Healy, L. Hermanspahn and P. E. Kruger, Coord. Chem. Rev., 2021, 432, 213756 CrossRef CAS.
- W. Chen, E. M. Goldys and W. Deng, Prog. Lipid Res., 2020, 79, 101052 CrossRef CAS PubMed.
- W. Li, J. Yang, L. Luo, M. Jiang, B. Qin, H. Yin, C. Zhu, X. Yuan, J. Zhang, Z. Luo, Y. Du, Q. Li, Y. Lou, Y. Qiu and J. You, Nat. Commun., 2019, 10, 3349 CrossRef PubMed.
- Y. Yang, L. Wang, H. Cao, Q. Li, Y. Li, M. Han, H. Wang and J. Li, Nano Lett., 2019, 19, 1821–1826 CrossRef CAS PubMed.
- Y. Zhang, H. Fu, S. Chen, B. Liu, W. Sun and H. Gao, Chem. Commun., 2020, 56, 762–765 RSC.
- S.-Y. Liu, Y. Xu, H. Yang, L. Liu, M. Zhao, W. Yin, Y.-T. Xu, Y. Huang, C. Tan, Z. Dai, H. Zhang, J.-P. Zhang and X.-M. Chen, Adv. Mater., 2021, 33, 2100849 CrossRef CAS PubMed.
- L. Fu, Z. Yan, Q. Zhao and H. Yang, Adv. Mater. Interfaces, 2018, 5, 1801094 CrossRef.
- N. Ni, X. Zhang, Y. Ma, J. Yuan, D. Wang, G. Ma, J. Dong and X. Sun, Coord. Chem. Rev., 2022, 458, 214415 CrossRef CAS.
- W. Zhao, Y. Zhao, Q. Wang, T. Liu, J. Sun and R. Zhang, Small, 2019, 15, 1903060 CrossRef CAS PubMed.
- R. Zhang, L. Liang, Q. Meng, J. Zhao, H. T. Ta, L. Li, Z. Zhang, Y. Sultanbawa and Z. P. Xu, Small, 2019, 15, 1803712 CrossRef PubMed.
- N. M. Idris, M. K. G. Jayakumar, A. Bansal and Y. Zhang, Chem. Soc. Rev., 2015, 44, 1449–1478 RSC.
- O. S. Wolfbeis, Chem. Soc. Rev., 2015, 44, 4743–4768 RSC.
- Y. I. Park, K. T. Lee, Y. D. Suh and T. Hyeon, Chem. Soc. Rev., 2015, 44, 1302–1317 RSC.
- M.-K. So, C. Xu, A. M. Loening, S. S. Gambhir and J. Rao, Nat. Biotechnol., 2006, 24, 339–343 CrossRef CAS PubMed.
- A. Kamkaew, F. Chen, Y. Zhan, R. L. Majewski and W. Cai, ACS Nano, 2016, 10, 3918–3935 CrossRef CAS PubMed.
- Y. Zhang, X. Zhang, H. Yang, L. Yu, Y. Xu, A. Sharma, P. Yin, X. Li, J. S. Kim and Y. Sun, Chem. Soc. Rev., 2021, 50, 11227–11248 RSC.
- D.-Y. Zhang, Y. Zheng, H. Zhang, L. He, C.-P. Tan, J.-H. Sun, W. Zhang, X. Peng, Q. Zhan, L.-N. Ji and Z.-W. Mao, Nanoscale, 2017, 9, 18966–18976 RSC.
- V. Bhandari, C. Hoey, L. Y. Liu, E. Lalonde, J. Ray, J. Livingstone, R. Lesurf, Y.-J. Shiah, T. Vujcic, X. Huang, S. M. G. Espiritu, L. E. Heisler, F. Yousif, V. Huang, T. N. Yamaguchi, C. Q. Yao, V. Y. Sabelnykova, M. Fraser, M. L. K. Chua, T. van der Kwast, S. K. Liu, P. C. Boutros and R. G. Bristow, Nat. Genet., 2019, 51, 308–318 CrossRef CAS PubMed.
- X. Li, N. Kwon, T. Guo, Z. Liu and J. Yoon, Angew. Chem., Int. Ed., 2018, 57, 11522–11531 CrossRef CAS PubMed.
- L. Larue, B. Myrzakhmetov, A. Ben-Mihoub, A. Moussaron, N. Thomas, P. Arnoux, F. Baros, R. Vanderesse, S. Acherar and C. Frochot, Pharmaceuticals, 2019, 12, 163 CrossRef CAS PubMed.
- W. Yu, T. Liu, M. Zhang, Z. Wang, J. Ye, C.-X. Li, W. Liu, R. Li, J. Feng and X.-Z. Zhang, ACS Nano, 2019, 13, 1784–1794 CAS.
- Z. He, P. Liu, S. Zhang, J. Yan, M. Wang, Z. Cai, J. Wang and Y. Dong, Angew. Chem., Int. Ed., 2019, 58, 3834–3837 CrossRef CAS PubMed.
- Y. Cheng, H. Cheng, C. Jiang, X. Qiu, K. Wang, W. Huan, A. Yuan, J. Wu and Y. Hu, Nat. Commun., 2015, 6, 8785 CrossRef CAS PubMed.
- Z. Ma, X. Jia, J. Bai, Y. Ruan, C. Wang, J. Li, M. Zhang and X. Jiang, Adv. Funct. Mater., 2017, 27, 1604258 CrossRef.
- L. Wei, Z. Zhang, A. Kumar, S. Banerjee and H. Huang, Chem. – Eur. J., 2022, 28, e202202233 CAS.
- Z. Gao, Y. Han and F. Wang, Nat. Commun., 2018, 9, 3977 CrossRef PubMed.
- A. A. Ghogare and A. Greer, Chem. Rev., 2016, 116, 9994–10034 CrossRef CAS PubMed.
- S. Kuang, F. Wei, J. Karges, L. Ke, K. Xiong, X. Liao, G. Gasser, L. Ji and H. Chao, J. Am. Chem. Soc., 2022, 144, 4091–4101 CrossRef CAS PubMed.
- S. Kuang, L. Sun, X. Zhang, X. Liao, T. W. Rees, L. Zeng, Y. Chen, X. Zhang, L. Ji and H. Chao, Angew. Chem., Int. Ed., 2020, 59, 20697–20703 CrossRef CAS PubMed.
- R. Song, H. Wang, M. Zhang, Y. Liu, X. Meng, S. Zhai, C.-C. Wang, T. Gong, Y. Wu, X. Jiang and W. Bu, Angew. Chem., Int. Ed., 2020, 59, 21032–21040 CrossRef CAS PubMed.
- J. Sun, X. Cai, C. Wang, K. Du, W. Chen, F. Feng and S. Wang, J. Am. Chem. Soc., 2021, 143, 868–878 CrossRef CAS PubMed.
- Y. Liu, W. Zhen, Y. Wang, S. Song and H. Zhang, J. Am. Chem. Soc., 2020, 142, 21751–21757 CrossRef CAS PubMed.
- Y. Song, L. Wang and Z. Xie, Biotechnol. J., 2021, 16, 1900382 CrossRef CAS PubMed.
- E. J. Hong, D. G. Choi and M. S. Shim, Acta Pharm. Sin. B, 2016, 6, 297–307 CrossRef PubMed.
- G. M. Calixto, J. Bernegossi, L. M. De Freitas, C. R. Fontana and M. Chorilli, Molecules, 2016, 21, 342 CrossRef PubMed.
- M. G. Mokwena, C. A. Kruger, M.-T. Ivan and A. Heidi, Photodiagn. Photodyn. Ther., 2018, 22, 147–154 CrossRef CAS PubMed.
- G. Chen, I. Roy, C. Yang and P. N. Prasad, Chem. Rev., 2016, 116, 2826–2885 CrossRef CAS PubMed.
- H. Abrahamse and M. R. Hamblin, Biochem. J., 2016, 473, 347–364 CrossRef CAS PubMed.
- J. Karges, M. Jakubaszek, C. Mari, K. Zarschler, B. Goud, H. Stephan and G. Gasser, ChemBioChem, 2020, 21, 531–542 CrossRef CAS PubMed.
- L. N. Lameijer, S. L. Hopkins, T. G. Brevé, S. H. C. Askes and S. Bonnet, Chem. – Eur. J., 2016, 22, 18484–18491 CrossRef CAS PubMed.
- Y. Huang, W. Huang, L. Chan, B. Zhou and T. Chen, Biomaterials, 2016, 103, 183–196 CrossRef CAS PubMed.
- P. Kaspler, S. Lazic, S. Forward, Y. Arenas, A. Mandel and L. Lilge, Photochem. Photobiol. Sci., 2016, 15, 481–495 CrossRef CAS PubMed.
- J. Karges, J. Li, L. Zeng, H. Chao and G. Gasser, ACS Appl. Mater. Interfaces, 2020, 12, 54433–54444 CrossRef CAS PubMed.
- V. Novohradsky, A. Zamora, A. Gandioso, V. Brabec, J. Ruiz and V. Marchán, Chem. Commun., 2017, 53, 5523–5526 RSC.
- J. Karges, D. Díaz-García, S. Prashar, S. Gómez-Ruiz and G. Gasser, ACS Appl. Biol. Mater., 2021, 4, 4394–4405 CrossRef CAS PubMed.
- W. Sun, Y. Wen, R. Thiramanas, M. Chen, J. Han, N. Gong, M. Wagner, S. Jiang, M. S. Meijer, S. Bonnet, H.-J. Butt, V. Mailänder, X.-J. Liang and S. Wu, Adv. Funct. Mater., 2018, 28, 1804227 CrossRef.
- N. Soliman, L. K. McKenzie, J. Karges, E. Bertrand, M. Tharaud, M. Jakubaszek, V. Guérineau, B. Goud, M. Hollenstein, G. Gasser and C. M. Thomas, Chem. Sci., 2020, 11, 2657–2663 RSC.
- S. H. C. Askes, A. Bahreman and S. Bonnet, Angew. Chem., Int. Ed., 2014, 53, 1029–1033 CrossRef CAS PubMed.
- Y. Boucher, L. T. Baxter and R. K. Jain, Cancer Res., 1990, 50, 4478–4484 CAS.
- K. Greish, Methods Mol. Biol., 2010, 624, 25–37 CrossRef CAS PubMed.
- H. He, L. Liu, E. E. Morin, M. Liu and A. Schwendeman, Acc. Chem. Res., 2019, 52, 2445–2461 CrossRef CAS PubMed.
- R. Wang, X. Li and J. Yoon, ACS Appl. Mater. Interfaces, 2021, 13, 19543–19571 CrossRef CAS PubMed.
- K. Qiu, Y. Chen, T. W. Rees, L. Ji and H. Chao, Coord. Chem. Rev., 2019, 378, 66–86 CrossRef CAS.
- L. Ke, F. Wei, L. Xie, J. Karges, Y. Chen, L. Ji and H. Chao, Angew. Chem., Int. Ed., 2022, 61, e202205429 CAS.
- D. Van Straten, V. Mashayekhi, H. S. De Bruijn, S. Oliveira and D. J. Robinson, Cancers, 2017, 9, 19 CrossRef PubMed.
- Y. Hao, C. K. Chung, Z. Yu, R. V. Huis in ‘t Veld, F. A. Ossendorp, P. ten Dijke and L. J. Cruz, Pharmaceutics, 2022, 14, 120 CrossRef CAS PubMed.
- S. Wang, G. Yu, W. Yang, Z. Wang, O. Jacobson, R. Tian, H. Deng, L. Lin and X. Chen, Adv. Sci., 2021, 8, 2002927 CrossRef CAS PubMed.
- W. Li, Z. Zhang, J. Liu, B. Wang, G. Pu, J. Li, Y. Huang and M. Chu, Acta Biomater., 2022, 146, 341–356 CrossRef CAS PubMed.
- S. Wang, G. Yu, W. Yang, Z. Wang, O. Jacobson, R. Tian, H. Deng, L. Lin and X. Chen, Adv. Sci., 2021, 8, 2002927 CrossRef CAS PubMed.
- X. Huang, L. Tang, L. Xu, Y. Zhang, G. Li, W. Peng, X. Guo, L. Zhou, C. Liu and X.-C. Shen, J. Mater. Chem. B, 2022, 10, 7717–7731 RSC.
- Y. Zhu, H. Shi, T. Li, J. Yu, Z. Guo, J. Cheng and Y. Liu, ACS Appl. Mater. Interfaces, 2020, 12, 18309–18318 CrossRef CAS PubMed.
- L. M. Chong, D. J. H. Tng, L. L. Y. Tan, M. L. K. Chua and Y. Zhang, Appl. Phys. Rev., 2021, 8, 041322 CAS.
- Z. Liu, Z. Xie, W. Li, X. Wu, X. Jiang, G. Li, L. Cao, D. Zhang, Q. Wang, P. Xue and H. Zhang, J. Nanobiotechnol., 2021, 19, 160 CrossRef CAS PubMed.
- M. Zhang, X. Liu, Y. Mao, Y. He, J. Xu, F. Zheng, W. Tan, S. Rong, Y. Chen, X. Jia and H. Li, ACS Appl. Mater. Interfaces, 2022, 14, 27551–27563 CrossRef CAS PubMed.
- F. Zhang, Y. Liu, B. Yang, P. Guan, J. Chai, G. Wen and B. Liu, J. Mater. Chem. B, 2021, 9, 2417–2427 RSC.
- C. Xu, J. Nam, H. Hong, Y. Xu and J. J. Moon, ACS Nano, 2019, 13, 12148–12161 CrossRef CAS PubMed.
- Z. Feng, T. Zhu, L. Wang, T. Yuan, Y. Jiang, X. Tian, Y. Tian and Q. Zhang, Inorg. Chem., 2022, 61, 12652–12661 CrossRef CAS PubMed.
- Y. Jiang, W. Meng, L. Wu, K. Shao, L. Wang, M. Ding, J. Shi and X. Kong, Adv. Healthcare Mater., 2021, 10, 2100789 CrossRef CAS PubMed.
- Y. Shi, D. Zhu, D. Wang, B. Liu, X. Du, G. Wei and X. Zhou, Coord. Chem. Rev., 2022, 471, 214725 CrossRef CAS.
- W. Guo, F. Wang, D. Ding, C. Song, C. Guo and S. Liu, Chem. Mater., 2017, 29, 9262–9274 CrossRef CAS.
- J. Sun, S. Kormakov, Y. Liu, Y. Huang, D. Wu and Z. Yang, Molecules, 2018, 23, 1704 CrossRef PubMed.
- F. Wei, S. Kuang, T. W. Rees, X. Liao, J. Liu, D. Luo, J. Wang, X. Zhang, L. Ji and H. Chao, Biomaterials, 2021, 276, 121064 CrossRef CAS PubMed.
- F. Wei, J. Karges, J. Shen, L. Xie, K. Xiong, X. Zhang, L. Ji and H. Chao, Nano Today, 2022, 44, 101509 CrossRef CAS.
- J. Shen, J. Karges, K. Xiong, Y. Chen, L. Ji and H. Chao, Biomaterials, 2021, 275, 120979 CrossRef CAS PubMed.
- L. Wang, X. Qu, Y. Zhao, Y. Weng, G. I. N. Waterhouse, H. Yan, S. Guan and S. Zhou, ACS Appl. Mater. Interfaces, 2019, 11, 35228–35237 CrossRef CAS PubMed.
- L. Jiao, H. Yan, Y. Wu, W. Gu, C. Zhu, D. Du and Y. Lin, Angew. Chem., Int. Ed., 2020, 59, 2565–2576 CrossRef CAS PubMed.
- X. Lu, S. Gao, H. Lin and J. Shi, Small, 2021, 17, 2004467 CrossRef CAS PubMed.
- E. Ju, K. Dong, Z. Chen, Z. Liu, C. Liu, Y. Huang, Z. Wang, F. Pu, J. Ren and X. Qu, Angew. Chem., Int. Ed., 2016, 55, 11467–11471 CrossRef CAS PubMed.
- C. Wang, F. Cao, Y. Ruan, X. Jia, W. Zhen and X. Jiang, Angew. Chem., Int. Ed., 2019, 58, 9846–9850 CrossRef CAS PubMed.
- M. Huo, L. Wang, Y. Wang, Y. Chen and J. Shi, ACS Nano, 2019, 13, 2643–2653 CAS.
- D. Wang, H. Wu, S. Z. F. Phua, G. Yang, W. Qi Lim, L. Gu, C. Qian, H. Wang, Z. Guo, H. Chen and Y. Zhao, Nat. Commun., 2020, 11, 357 CrossRef CAS PubMed.
- M. Lismont, L. Dreesen and S. Wuttke, Adv. Funct. Mater., 2017, 27, 1606314 CrossRef.
- P. Gao, Y. Chen, W. Pan, N. Li, Z. Liu and B. Tang, Angew. Chem., Int. Ed., 2021, 60, 16763–16776 CrossRef CAS PubMed.
- T. Luo, G. T. Nash, Z. Xu, X. Jiang, J. Liu and W. Lin, J. Am. Chem. Soc., 2021, 143, 13519–13524 CrossRef CAS PubMed.
- K. Lu, C. He and W. Lin, J. Am. Chem. Soc., 2014, 136, 16712–16715 CrossRef CAS PubMed.
- D. Jin, J. Zhang, Y. Huang, X. Qin, J. Zhuang, W. Yin, S. Chen, Y. Wang, P. Hua and Y. Yao, Dalton Trans., 2021, 50, 1189–1196 RSC.
- L. He, Q. Ni, J. Mu, W. Fan, L. Liu, Z. Wang, L. Li, W. Tang, Y. Liu, Y. Cheng, L. Tang, Z. Yang, Y. Liu, J. Zou, W. Yang, O. Jacobson, F. Zhang, P. Huang and X. Chen, J. Am. Chem. Soc., 2020, 142, 6822–6832 CrossRef CAS PubMed.
- N. Ma, Y. Li, H. Xu, Z. Wang and X. Zhang, J. Am. Chem. Soc., 2010, 132, 442–443 CrossRef CAS PubMed.
- F. Fan, S. Ji, C. Sun, C. Liu, Y. Yu, Y. Fu and H. Xu, Angew. Chem., Int. Ed., 2018, 57, 16426–16430 CrossRef CAS PubMed.
- Y. Liu, N. Wen, K. Li, M. Li, S. Qian, S. Li, T. Jiang, T. Wang, Y. Wu and Z. Liu, Mol. Pharmaceutics, 2022, 19, 805–818 CrossRef CAS PubMed.
- L. Ke, F. Wei, X. Liao, T. W. Rees, S. Kuang, Z. Liu, Y. Chen, L. Ji and H. Chao, Nanoscale, 2021, 13, 7590–7599 RSC.
- D. Wei, Y. Yu, X. Zhang, Y. Wang, H. Chen, Y. Zhao, F. Wang, G. Rong, W. Wang, X. Kang, J. Cai, Z. Wang, J.-Y. Yin, M. Hanif, Y. Sun, G. Zha, L. Li, G. Nie and H. Xiao, ACS Nano, 2020, 14, 16984–16996 CrossRef CAS PubMed.
- C.-N. Lok, T. Zou, J.-J. Zhang, I. W.-S. Lin and C.-M. Che, Adv. Mater., 2014, 26, 5550–5557 CrossRef CAS PubMed.
- J.-J. Zhang, W. Lu, R. W.-Y. Sun and C.-M. Che, Angew. Chem., Int. Ed., 2012, 51, 4882–4886 CrossRef CAS PubMed.
- J. L.-L. Tsai, T. Zou, J. Liu, T. Chen, A. O.-Y. Chan, C. Yang, C.-N. Lok and C.-M. Che, Chem. Sci., 2015, 6, 3823–3830 RSC.
- J. Li, L. Zeng, Z. Wang, H. Chen, S. Fang, J. Wang, C.-Y. Cai, E. Xing, X. Liao, Z.-W. Li, C. R. Ashby Jr, Z.-S. Chen, H. Chao and Y. Pan, Adv. Mater., 2022, 34, 2100245 CrossRef CAS PubMed.
- M. Fornasier, S. Biffi, B. Bortot, P. Macor, A. Manhart, F. R. Wurm and S. Murgia, J. Colloid Interface Sci., 2020, 580, 286–297 CrossRef CAS PubMed.
- J. Chen, A. C. Sedgwick, S. Sen, Y. Ren, Q. Sun, C. Chau, J. F. Arambula, T. Sarma, L. Song, J. L. Sessler and C. Liu, Chem. Sci., 2021, 12, 9916–9921 RSC.
- J. Karges, M. Tharaud and G. Gasser, J. Med. Chem., 2021, 64, 4612–4622 CrossRef CAS PubMed.
- D. Wei, Y. Huang, B. Wang, L. Ma, J. Karges and H. Xiao, Angew. Chem., Int. Ed., 2022, 61, e202201486 CAS.
- E. R. Johnson, S. Keinan, P. Mori-Sánchez, J. Contreras-García, A. J. Cohen and W. Yang, J. Am. Chem. Soc., 2010, 132, 6498–6506 CrossRef CAS PubMed.
- M.-S. Yuan, D.-E. Wang, P. Xue, W. Wang, J.-C. Wang, Q. Tu, Z. Liu, Y. Liu, Y. Zhang and J. Wang, Chem. Mater., 2014, 26, 2467–2477 CrossRef CAS.
- M. O. Sinnokrot, E. F. Valeev and C. D. Sherrill, J. Am. Chem. Soc., 2002, 124, 10887–10893 CrossRef CAS PubMed.
- X. Li, D. Lee, J.-D. Huang and J. Yoon, Angew. Chem., Int. Ed., 2018, 57, 9885–9890 CrossRef CAS PubMed.
- Y.-Y. Zhao, L. Zhang, Z. Chen, B.-Y. Zheng, M. Ke, X. Li and J.-D. Huang, J. Am. Chem. Soc., 2021, 143, 13980–13989 CrossRef CAS PubMed.
- T. X.-Q. Zhou, M. Xiao, V. Ramu, J. Hilgendorf, X. Li, P. Papadopoulou, M. A. Siegler, A. Kros, W. Sun and S. Bonnet, J. Am. Chem. Soc., 2020, 142, 10383–10399 CrossRef PubMed.
- W. Yu, L. Sisi, Y. Haiyan and L. Jie, RSC Adv., 2020, 10, 15328–15345 RSC.
- V. Negri, J. Pacheco-Torres, D. Calle and P. López-Larrubia, Top. Curr. Chem., 2020, 378, 15 CrossRef CAS PubMed.
- R.-Q. Li, C. Zhang, B.-R. Xie, W.-Y. Yu, W.-X. Qiu, H. Cheng and X.-Z. Zhang, Biomaterials, 2019, 194, 84–93 CrossRef CAS PubMed.
- J.-Y. Zhou, W.-J. Wang, C.-Y. Zhang, Y.-Y. Ling, X.-J. Hong, Q. Su, W.-G. Li, Z.-W. Mao, B. Cheng, C.-P. Tan and T. Wu, Biomaterials, 2022, 289, 121757 CrossRef CAS PubMed.
- W. Lv, T. Yang, Q. Yu, Q. Zhao, K. Y. Zhang, H. Liang, S. Liu, F. Li and W. Huang, Adv. Sci., 2015, 2, 1500107 CrossRef PubMed.
- J. Wang, Q. Ni, Y. Wang, Y. Zhang, H. He, D. Gao, X. Ma and X. J. Liang, J. Controlled Release, 2021, 331, 282–295 CrossRef CAS PubMed.
- Y. Zhang, H. Fu, S. Chen, B. Liu, W. Sun and H. Gao, Chem. Commun., 2020, 56, 762–765 RSC.
- Z. Dong, L. Feng, W. Zhu, X. Sun, M. Gao, H. Zhao, Y. Chao and Z. Liu, Biomaterials, 2016, 110, 60–70 CrossRef CAS PubMed.
- X. Wei, M. Song, G. Jiang, M. Liang, C. Chen, Z. Yang and L. Zou, Theranostics, 2022, 12, 5272–5298 CrossRef CAS PubMed.
|
This journal is © The Royal Society of Chemistry 2023 |
Click here to see how this site uses Cookies. View our privacy policy here.