DOI:
10.1039/D1TB02323C
(Review Article)
J. Mater. Chem. B, 2022,
10, 2338-2356
Recent advances of zwitterionic-based topological polymers for biomedical applications
Received
22nd October 2021
, Accepted 29th January 2022
First published on 2nd February 2022
Abstract
Zwitterionic polymers, comprising hydrophilic anionic and cationic groups with the same total number of positive and negative charges on the same monomer residue, have received increasing attention due to their distinctive physico-chemical properties, including high hydration, good biocompatibility, good antifouling and good antibacterial properties, and thus show great potential as a novel type of biomedical material. Unique topological polymers with block, star, dendritic, comb, brush and cyclic structures not only meet the requirements of material structure accuracy but also provide a theoretical research template for the development of materials science. Topological structures can facilitate polymers with precise and adjustable structures, hydrodynamic size, lower viscosity, more active sites, less intermolecular entanglement, and increased colloidal stability, which can effectively achieve drug encapsulation, improve the drug-loading efficiency, achieve faster internalization, and reduce the in vitro cytotoxicity. Therefore, the synthesis of topological polymers with precise structures has become an inevitable requirement for the development of materials and their biomedical applications. The article mainly reviews the properties and application of zwitterionic polymers and their derivatives with different topological structures. In particular, the recent progress of these polymers in drug delivery, antitumor properties, biomedical diagnosis and antifouling coatings are described and introduced. Finally, the current problems in these applications are discussed, and the potential research prospects of zwitterionic-based topological polymers in current research are put forward.
1. Introduction
In recent years, zwitterionic polymers have become a hot subject of research in the field of biomaterials because of their unique structure, easy functionalization and ultra-low contamination. Zwitterionic materials are charge-neutral ionic systems, which possess both anionic and cationic components with equal charge on the same monomer residue.1–4Fig. 1 illustrates the most commonly used zwitterionic moieties, including quaternary ammonium,5 phosphate,6,7 carboxylate or sulfonate.8–10 Compared with non-ionic hydrophilic polymers (such as polyethylene glycol, polyvinyl alcohol and poly(2-methyl-2-oxazoline)), zwitterionic polymers can bind a large number of water molecules via strong electrostatic force-induced hydration, endowing the material surface with superhydrophilicity. As for zwitterionic species, charge neutrality and high surface hydration are generally considered to be the key to resisting non-specific protein adsorption, thus effectively preventing contamination from proteins.11
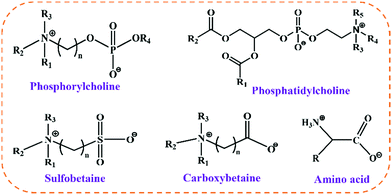 |
| Fig. 1 Zwitterionic moieties and their chemical structures. | |
Polyethylene glycol (PEG) is a kind of non-toxic and biocompatible polymer. PEG can reduce protein immunogenicity and improve pharmacokinetics, becoming the gold standard of antifouling materials and being widely used in biomedical research.12–15 However, it is reported that PEG is easily oxidized and decomposed. Besides, it has poor long-term stability and difficult natural degradability. Unfortunately, these drawbacks greatly limit the practical application of PEG.16–18 Moreover, PEGylated drugs can induce the production of anti-PEG antibodies and lead to the rapid clearance of drugs in vivo.19,20 Several research groups have proved that anti-PEG antibodies are closely related to the accelerated blood clearance (ABC) of the second injection of PEG liposomes.21,22 In addition, several animal experiments have shown that PEG liposomes can activate the complement system of animals and may cause allergic reactions.23,24 Therefore, specific attention is given to the immunogenicity of PEG. In recent decades, the emerging zwitterionic polymers have been considered to be the most meaningful substitutes to PEG. Compared with the amphiphilic nature of PEG, zwitterionic polymers have the advantages of superhydrophilicity, good thermal stability and biocompatibility. At present, the most studied zwitterionic polymers including phosphorylcholine (PC), carboxybetaine (CB), sulfobetaine (SB), etc.,25–28 which can maintain their stability, prolong the circulation time in vivo, avoid immunogenicity and produce antibodies, demonstrate good application prospects in the fields of drug delivery, antitumor therapy and antifouling coating.29–31
Traditional linear zwitterionic polymers can be prepared through the direct polymerization of zwitterionic monomers. Although its synthesis is simple and straightforward, the function of linear zwitterionic polymers is specific. They may self-assemble to form micelles, vesicles and nanoparticles with a large size, poor stability, and challenging controllability; thus, it is difficult to obtain more defined structures. With the development of polymerization technology, in order to meet the ever-increasing performance requirements of polymer materials, a variety of zwitterionic-based topological polymers, such as block-based, star-shaped, hyperbranched, dendritic, brush and cyclic polymers have sparked the attention of researchers’. This type of polymer, with the merits of controllable synthesis, good stability, small size, and convenient and efficient multi-functionalization, has been widely used in biomedicine. However, the existing zwitterionic polymer-based materials can no longer meet the complex and changeable microenvironment, which limits their applications in some specific fields. Thereby, how to further elegantly integrate zwitterionic materials with other stimuli-responsive materials to create novel materials with virtues from both moieties has become the focus of scientific research, and zwitterions with other functional monomer-based topological polymers could be a good choice. At present, although many reviews have summarized zwitterionic polymers for biomedical applications, to the best our knowledge, there has been no systematic review of the research progress in zwitterionic-based topological polymers. In this review, we introduce the zwitterionic-based topological polymers with different topological structures, describe their preparation process, and clarify their biomedical applications. In addition, the problems existing in the current research are also discussed, and their prospects are considered.
2. Zwitterionic-based topological polymers
Traditional linear polymers do not have branch points and their molecular chains are easy to entangle, the viscosity of the system increases rapidly with the increase in relative molecular weight, the stability of self-assembled micelles is poor, and they are easy to disassemble after intravenous injection because the concentration is below the critical micelle concentration (CMC), resulting in the early release of encapsulated drugs, which great limits their functional application. Topological polymers, including but not limited to linear block,32,33 star-shaped,34 dendritic35 and brush structures,36 have more precise structures, a lower viscosity, and smaller size (such as dendrimers), for which it is easy to achieve cell internalization and they can be used as drug-delivery carriers. In addition, their abundant terminal functional groups increase the active sites, making it easy to achieve targeting. Moreover, topological polymers have an increased colloidal stability (such as cyclic brush polymers), which can achieve good antitumor effects. With the development of biomaterials science, the synthesis of zwitterionic polymers with different topological structures has attracted extensive attention, which has had an important influence on the polymer performance, regulated the topological structure in polymer synthetic chemistry, and further promoted the development of the biomedical field. In the following, we will focus on the characteristics and synthesis of each topological structure in the remainder of Section 2 and clarify their biomedical applications in Section 3.
2.1 Zwitterionic-based block polymers
As the demand for material properties increases, polymers are gradually moving towards the direction of refined structure synthesis, and the preparation of polymers with precise structures through controllable synthesis has become the development trend. In the past few decades, the self-assembly of zwitterionic block copolymers has attracted great attention in the scientific community. For example, Ye et al.37 synthesized the controlled architecture of a dual-functional block polymer by sequentially grafting a quaternary ammonium salt polymer and a zwitterionic polymer via surface-initiated atom transfer radical polymerization (SI-ATRP). Quaternary ammonium salt polymers reduce the excessive propagation of bacteria, thus exerting antibacterial activity to prevent the formation of biofilms as well as the adhesion of biofouling, so that a comprehensive “defense” and “attack” against biofouling can be conducted. Fu et al.38–40 designed and constructed two polymer brushes with opposite hierarchical structures (SiPQAs-b-PSBMA and Si-PSBMA-b-PQAs) via the grafting of poly(sulfobetaine methacrylate) (PSBMA) and cationic bactericidal poly(quaternary ammonium salts) (PQAs). They found there were synergistic antibacterial effects between different alkyl chain lengths and different hierarchies. PSBMA also has good blood compatibility, especially anticoagulant properties, and thus plays a pivotal role as an anticoagulant material. Based on this, Zhao et al.41 prepared block and random zwitterionic polymers (P(SBMA-b-NaSS)) and (P(SBMA-co-NaSS)) for the first time by grafting PSBMA and sodium phenylethylsulfonate (NaSS) from a polysulfone (PSf) membrane through SI-ATRP (Fig. 2a). The results showed that, compared with PSf and PSBMA membranes, the modified zwitterion membranes had significantly enhanced anticoagulant properties and lower protein adsorption properties. This study has laid a foundation for the development of zwitterionic copolymer-modified membranes and has potential biomedical applications.
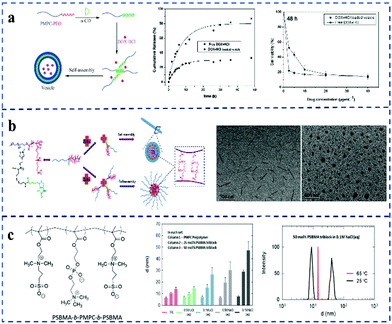 |
| Fig. 2 Synthesis of zwitterionic-based block polymers. (a) Study on blood compatibility of the zwitterion-modified membrane.41 Reproduced from ref. 41 with permission from Elsevier. (b) Zwitterionic diblock bioconjugates.49 Reproduced from ref. 49 with permission from American Chemical Society. (c) Average particle size of ZI-ABA in different solvents and 50 mol% PSBMA triblock copolymer in 0.1 M NaCl solution at different temperatures.50 Reproduced from ref. 50 with permission from American Chemical Society. | |
2-Methacryloyloxyethylphosphocholine (MPC) is currently one of the most widely used zwitterions, and the synthesis of biocompatible and non-toxic gene-delivery vectors is the key to the treatment of genetic diseases. For this purpose, Narain et al.42 copolymerized MPC with cations to synthesize di-block copolymers and random copolymers, which were then combined with DNA. Due to the different morphologies of DNA vectors formed via different polymerization methods, the gene-delivery efficiency was further affected. The results confirmed that the prepared block-based zwitterionic copolymer could deliver a gene vector more effectively in the presence or absence of serum in vitro. Therefore, polymer vesicles prepared from MPC-based materials have potential prospects as drug-delivery vehicles. Based on this, Ji et al.43,44 prepared a novel biocompatible polymer vesicle. Specifically, they first prepared PEO-PMPC diblock copolymers via ATRP and loaded doxorubicin (DOX) after reacting with α-cyclodextrin (α-CD). The in vitro results confirmed that the drug-loaded polymer vesicles can significantly reduce the cytotoxicity of the original drug and effectively release DOX at the tumor site.
Owing to the fact that phosphonic acid/ester can form a stable coordination with the metal/metal oxide surface, which provides a highly stable anchoring ability on various substrates and demonstrates excellent antifouling properties, phosphorus-group-based zwitterionic polymers are also of great significance in drug delivery, dental biomaterials and tissue engineering. Shen et al. developed a series of zwitterionic-phosphonate block polymers via controlled RAFT polymerization for antifouling coatings on metal substrates.45 The zwitterionic polymers not only have good synthesis controllability and use mild reaction conditions but can be stably coated on biomedical metals through PO–metal coordination bonds due to the existence of pDEMMP. They further systematically studied the relationship between the antifouling performance of the polymer-coated TC4 substrate and the chain lengths of pSBMA and pDEMMP in zwitterionic block polymers. The research results found that pDEMMP with only 5 chain lengths could form a stable zwitterionic polymer coating on the TC4 substrate, while pSBMA with a chain length of more than 40 could effectively inhibit protein adsorption, and cell and bacterial adhesion. Therefore, the biocompatible zwitterionic/phosphate block copolymer constructed by selecting the polymer with the optimal number of segments has excellent antifouling abilities and can be widely used in biomedical implants.
Zwitterionic poly(sulfobetaine) (PSB) is one of the most typical thermoresponsive polymer materials. The upper critical solution temperature (UCST) behavior of PSB is opposite to the lower critical solution temperature (LCST) behavior of many non-ionic polymers in aqueous solution. Moreover, the phase-transition temperature of PSB (CPPSB) is very sensitive to the addition of salt to the polymer solution, while CPPNIPMAM is relatively insensitive to the addition of salt. Orthogonal dual thermoresponsive diblock copolymers (DBCs) made from LCST-type PNIPAM or PNIPMAM blocks and UCST-type PSB blocks have attracted attention due to their self-assembly behavior. In solution, they can form core–shell micelles, whose structure can be effectively reversed when the temperature is changed.46,47 Based on this dual stimulus-sensitive behavior, Kreuzer et al.48 prepared a PSB-based DBC thin film (PSBP245-b-PNIPMAM105) from saline solutions. Biological conjugates prepared by combining amphiphilic polymers and natural proteins have both temperature-dependent changes and enzymatic activity, and have been widely used in biological separation, drug delivery and enzyme biological engineering. However, the self-assembly behavior of bioconjugates has not been studied. Based on this, Zhao et al.49 synthesized a zwitterionic block copolymer with biotin groups at the junction point through the combination of click chemistry, the thiol–disulfide exchange reaction and ATRP (Fig. 2b). The bioconjugates exhibit UCST-type thermosensitivity and can further self-assemble when induced by temperature. At temperatures lower than the UCST, the bioconjugates self-assembled in aqueous solution to form rod-like structures and spherical micelles, depending on the number of block copolymers grafted onto the protein molecules. When the conjugate contains 1.3 block copolymer chains, rod-like structures can be formed, while spherical micelles can be formed when the conjugate contains 2.9 copolymer chains. Therefore, this work provided a platform for the synthesis of conjugates with various topological structures. Panzer and co-workers synthesized a distinctive fully zwitterionic triblock copolymer (ZI-ABA) for the first time (Fig. 2c).50 The fully ZI-ABA triblock polymers were synthesized through free radical polymerization with MPC as the middle block, and SBMA as the symmetrical end blocks. The ZI-ABA copolymers exhibit self-assembly behavior, and thermal- and salt-responsive behavior in solution due to the strong aggregation of SBMA.
Poly(amino acid)s, with both cations and anions, belong to zwitterionic polymers, and also demonstrate wide biological applications. Wu et al. reported a series of dimethylaminopropyl aminated poly(α,β-L-aspartic acid) (DMPA-g-PAsp) materials with anti-biofouling ability and good biocompatibility.51 Liu et al. developed a serine-based zwitterionic polymer (pSerMA), which was further grafted onto a gold surface via surface-initiated photopolymerization.52 The pSerMA-grafted surface can not only strongly inhibit the adsorption of proteins but also inhibit the adsorption from whole human serum and plasma. In addition, Gitsov and Schutz reported the “green” synthesis of unnatural poly(amino acid)s catalyzed by enzymes.53 Specifically, they formed DL-tyrosine by polymerizing linear-dendritic laccase complexes with water. Studies have confirmed that the formed materials are completely water-soluble and exhibit typical polyzwitterionic pH-responsive behavior.
2.2 Star-shaped zwitterionic polymers
Star polymers are complex macromolecular polymers that have more than three linear connections radially from one branch point. Compared with linear analogues of the same molecular weight, star-shaped polymers possess unique hydrodynamic properties and encapsulation capabilities due to their three-dimensional spherical structure. In addition, star-shaped polymers exhibit a lower solution viscosity and smaller dynamic mechanical size in dilute solution due to less arm entanglement. Moreover, their internal and peripheral structures provide a convenient way to introduce different active functional groups, which has gradually become a research hotspot in the field of polymer science.54 Up until now, star polymers have been widely used in the design of biomedical materials for drug delivery and imaging diagnosis.55 For example, poly(amidoamine) (PAMAM) dendrimers have been widely studied in biomedical applications due to their good biocompatibility.56 However, owing to the lack of any shielding effect on the positive charge of the dendrimer, the amino-terminated cationic PAMAM dendrimers exhibited concentration-dependent cytotoxicity. The cytotoxicity can be effectively reduced by modification with polymer, such as PEG, acryloxyethyl phosphorylcholine (APC) and carboxybetaine acrylamide (CBAA).57–62 Based on this, Li et al.63 synthesized a novel, non-toxic, high-anticancer-activity star polymer anticancer drug-delivery vehicle (PAMAM-g-PDMAPS) by grafting PAMAM with poly[3-dimethyl(methacryloyloxyethyl)ammonium propanesulfonate] (PDMAPS).
High molecular weight polycations such as poly(2-dimethylamino)ethyl methacrylate have been used to construct gene-transfer vectors due to their strong DNA aggregation ability.64 However, the cytotoxicity of the polycation limits its application. Compared with linear polycations, star-shaped polycations have a lower in vitro cytotoxicity but a higher transfection efficiency. For example, star-shaped polycations grafted with polyhedral oligomeric silsesquioxanes (POSS) have good biocompatibility and a well-defined structure.65,66 Moreover, the hydrophobic part promotes gene aggregation, and improves drug loading and endocytosis. It has been reported that zwitterionic materials (such as PC, SB, CB, etc.) and nanoparticles based on these materials can stabilize gene vectors in complex-environment media (such as serum, blood and body fluids) to achieve ‘zero’ pollution.67,68 In addition, the superhydrophilic properties of zwitterions form a strong polar contrast with the hydrophobic core of POSS, and possess excellent stability, significantly enhancing the drug-loading efficiency. At the same time, nanoparticles coated with zwitterionic materials can effectively avoid rapid blood clearance during repeated injections by inhibiting the production of polymer-specific antibodies. Based on this, Li et al.69 synthesized star-shaped zwitterionic cationic copolymers via the ATRP copolymerization of zwitterionic poly[N-(3-(methacryloylamino)propyl)-N,N-dimethyl-N-(3-sulfopropyl)ammonium hydroxide] (PMPDSAH) and cationic poly[2-(dimethylamino)ethyl methacrylate] (PDMAEMA) (Fig. 3a). These star-shaped copolymers can self-assemble to form core–shell corona micelles in aqueous solution, and further encapsulate the tumor-suppressor gene p53 and DOX to evaluate their synergistic tumor suppression effect in vivo. The results showed that the POSS-based star-shaped polymer can form stable micelles in aqueous solution, even in the presence of serum. In addition, the DOX and p53-gene-loaded complex has excellent biocompatibility and can effectively deliver DOX and the p53 gene. In vivo results further confirmed that the complex not only has an enhanced antitumor effect but can also minimize the toxic side effects. Therefore, this work provides a potential clinical application for cancer treatment. Polymethacrylates are another cationic disinfectant that have been widely studied for their good antibacterial activity. However, they usually cause toxicity to serum, and various strategies to improve their non-contaminating properties or biocompatibility often result in reduced bactericidal activity. So far, the antibacterial work of PDMA has been reported, but star-shaped polymers are rarely used as antibacterial compounds. Based on the designability of the above three-dimensional (POSS) structure, Pu et al.70 synthesized star polymers (POSS-g-PDMA) via ATRP with non-toxic POSS as the core and poly[2-(dimethylamino)ethyl methacrylate] (PDMA) as the arm (Fig. 3b). SB was further copolymerized with POSS-g-PDMA to obtain random (POSS-g-(PDMA-r-PSB)) and block (POSS-g-(PDMA-b-PSB)) arm structures. Furthermore, they compared the hemolytic and antibacterial activity of block and random zwitterionic cationic polymers. The results confirmed that both random and block zwitterionic polymers could effectively kill Escherichia coli within 30 min. More importantly, compared with random zwitterionic polymers, block zwitterionic polymers not only significantly enhanced the serum stability but also reduced the aggregation of red blood cells in the blood.
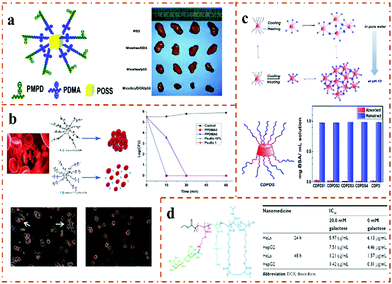 |
| Fig. 3 Synthesis of zwitterionic star-shaped polymers. (a) Antitumor effect of POSS-based star-shaped polymer in vivo.69 Reproduced from ref. 69 with permission from Elsevier. (b) Antibacterial study of star-shaped POSS-g-(PDMA-r-PSB) copolymers.70 Reproduced from ref. 70 with permission from American Chemical Society. (c) Thermo-responsive and BSA adsorption behavior of CDPD and CDPDS star polymer.74 Reproduced from ref. 74 with permission from The Royal Society of Chemistry. (d) In vitro cytotoxicity of dual cored hepatoma-specific star-shaped nanogel.78 Reproduced from ref. 78 with permission from Dovepress. | |
PSBs are the most popular UCST polymers in aqueous solution and have been widely applied in smart materials such as thermoresponsive gels, multilayer microspheres and nanoparticles.71–73 In addition, PSB is also used to construct antifouling surfaces under broad pH ranges. Based on the advantages of zwitterionic PSB, Zhang et al.74 synthesized a star polymer (CDPDS) with polyzwitterionic blocks and hyper-branched polycationic (PDMAEMA) blocks (Fig. 3c). The star-shaped polymers exhibit adjustable UCST behavior and good biocompatibility. The research results showed that the PSBMA blocks have a good shielding effect on the cationic PDMAEMA blocks, so that the CDPDS star polymer only showed UCST behavior. In addition, the higher density of PDMAEMA significantly enhanced the solubility of the star polymers. Moreover, star polymers have enhanced thermal responsiveness, low toxicity and could effectively reduce the adsorption of BSA. Therefore, CDPDS star polymers can be well applied in the field of biomedicine.
In addition to the star-shaped zwitterionic polymers studied above, the recently reported star-shaped polymer nanogels, also known as the intelligent drug-delivery systems, have shown themselves to be potential tools for the efficient diagnosis and treatment of cancer or neurodegenerative disorders.75 In fact, it has been reported that star-shaped polymer nanogels prepared via controlled/living free radical polymerization are in principle suitable for delivering multifunctional molecular cargoes, controlling cellular uptake, and triggering intracellular drug release. In addition, the star polymer nanogels also exhibit stable physicochemical properties, which is good for their prolonged systemic retention.76 Moreover, the hydrophobic interaction between the drug and the core of the star polymer nanogel can achieve a high drug-carrying capacity, and the “arm” can be modified using chemically and biologically active ligands to achieve active and targeted delivery. Glycosylation is a promising method of active targeted drug delivery.77 The combination of glycosylation targeting ability and the tumor microenvironment has potential prospects for targeted drug delivery. Lou et al.78 prepared a star-shaped thermoresponsive and reductase-cleavable polymer nanogel via an “arm-first” ATRP method for targeted liver cancer drug delivery (Fig. 3d). The thermo- and redox-sensitive core, composed of poly(N-isopropylacrylamide) (PNIPAM) cross-linked by disulfide bonds (–S–S–), has amphiphilic and anti-adsorption properties with PSBMA. The arm conjugate (PSBMA) formed by the ionic copolymer block is used to reduce the interaction of the polymer nanogel with plasma proteins and cells. Poly(2-lactose diaminoethyl methacrylamide) (PLAMA) is used as a targeted fragment for recognizing ASGP-Rs in hepatocytes. Compared with HeLa cells, star-shaped nanogels have lower IC50 values in human hepatoma cells (HepG2).
2.3 Dendritic zwitterionic polymers
Dendrimers are polymers that have hyperbranched three-dimensional structures, are nano-sized and have high geometric symmetry.79–82 Owing to their internal cavities, surface functionalities, small size (<20 nm), and easy removability, they have been considered as ideal nanocarriers for drug or gene delivery. Therefore, massive studies have proposed the use of dendrimers as nanomaterial carriers. Due to steric hindrance, the PEG crown of PEGylated dendritic nanocarrier hinders its interaction with the cell membrane, resulting in a decrease in the endocytosis efficiency.83 The zwitterionic PSB can not only resist protein absorption through electrostatic repulsion but can also avoid steric hindrance accumulation through the formed hydration shell. Therefore, PSB has been widely used in the preparation of dendritic macromolecules instead of PEG and its derivatives.84 Based on the advantages of zwitterionic PSB in drug-delivery systems for cancer or inflammation treatment, Wu et al.85 reported a novel dendrimer containing pH-degradable acetal bonds and dendritic polyacetal, where the end of the dendritic polyacetal is connected with an SB-type zwitterion. The self-assembly behavior, pH responsiveness and drug-release behavior of the modified zwitterionic dendritic polyacetal in water were studied, and it was confirmed that zwitterion-modified dendritic polyacetal has the function of charge reversal and an excellent drug-release performance. Furthermore, by combining the advantages of the polyacetal and the dendrimer, Wu et al.86 designed and synthesized a dendritic zwitterionic polymer with CD as the host molecules and adamantane-terminated poly(sulfobetaine) (Ada-PSB) as the guest molecules (Fig. 4a). Then, the amphiphilic supramolecular LDBCs were successfully synthesized via the host–guest recognition of β-CD and Ada. Micelles and vesicles were obtained by adjusting the hydrophilic/hydrophobic ratio of the LDBCs. In vitro drug release and cytotoxicity results showed that drug-loaded dendritic nanoparticles could achieve over 90% drug release under intracellular acidic conditions, and their cytotoxicity is comparable to free DOX. Therefore, dendritic zwitterionic polymers could be used as potential anticancer drug-delivery carriers.
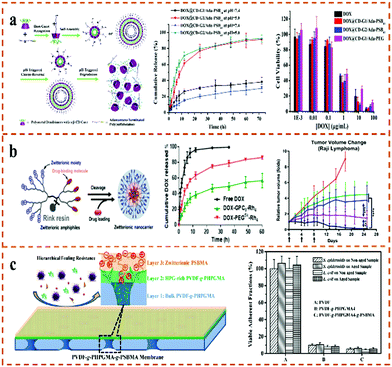 |
| Fig. 4 Synthesis of dendrimer zwitterionic polymers. (a) The pH-triggered charge reversal, degradation behavior and in vitro drug-release profile of dendritic supramolecular micelles and vesicles.86 Reproduced from ref. 86 with permission from the Royal Society of Chemistry. (b) In vivo tumor treatment of amphiphilic dendritic zwitterionic polymer.90 Reproduced from ref. 90 with permission from American Chemical Society. (c) Viable adherent fractions of dendritic zwitterionic (PVDF-g-PHPGMA-g-PSBMA) hierarchical antifouling membranes.91 Reproduced from ref. 91 with permission from American Chemical Society. | |
Dendritic nanocarriers with a definite structure are promising for tumor targeting and anticancer therapy due to their enhanced drug-carrying capacity and stability.87 Zwitterionic materials, such as phosphorylcholine (PC), not only reduce protein adsorption, but also show more effective antifouling properties by providing stealth properties to the nanoparticles.88,89 Luo et al.90 developed a novel Janus dendrimer (JD) amphiphilic zwitterionic polymer based on their previously designed terminal dendrimer nanocarriers to enhance the delivery of anticancer drugs (Fig. 4b). In order to avoid the tedious synthesis and purification of liquid-phase dendrimers, they used solid-phase peptide chemistry (SPPC) to construct JD amphiphilic polymers with precise chemical structures. Janus dendrimers self-assemble to form zwitterionic JD nanoparticles, and then DOX is loaded through hydrophobic interactions. Compared with linear PEGylated nanoformulations, drug-loaded zwitterionic JD nanoparticles show higher stability, sustained drug release in vitro, prolonged blood circulation and better anti-tumor properties.
The practical applications of fluoropolymers are greatly restricted due to their inherent inertness. To develop environmentally friendly antifouling coatings that do not release pollutants, Li et al.91 used polyvinylidene fluoride (PVDF) as a model fluoropolymer to construct a layered antifouling surface polymer (Fig. 4c). Specifically, they synthesized the PVDF-g-PHPGMA copolymer of pentafluorophenyl methacrylate (PFMA) with the ozone-preactivated PVDF via RAFT polymerization. PVDF-g-PHPGMA membranes and zwitterionic SBMA were further functionalized via SI-ATRP to obtain the PVDF-g-PHPGMA-g-PSBMA membranes. The overall antibacterial adhesion and antifouling performance of the PVDF-g-PHPGMA-g-PSBMA membrane was obtained through the good synergistic effect between the dendritic architecture of PHPGMA and the superhydrophilic PSBMA brush.
2.4 Brush zwitterionic polymers
Brush polymers are a kind of polymer with a nanometer-sized and hyperbranched structure, and have a simpler preparation procedure than dendritic polymers. Over the past few decades, polymer brushes have become lubricants with excellent stability, and antifouling and lubrication abilities due to their dense segment grafted structure and resistance to interpenetration under compression, which provide enhanced wear protection.92 With the increased demand for functional surface coatings, zwitterionic polymer brushes with excellent lubricity and low-pollution coatings have attracted considerable attention. Recent studies have reported that the low interpenetration of bottle brush (BB) polymers, zwitterionic brush (PZWs) and surface grafted polymer brush can provide good surface lubrication.93–95 Among them, the most lubricious brush formulations belong to PZWs. PZW brushes have been widely used on various surfaces due to their high lubricating properties and inherent inertness to biological contaminants.96–98 Yan et al.99 prepared brush-like PZW brushes with different structures, such as linear PMPC brushes, graft copolymer brushes and bottlebrushes. Exposing the PZW molecular brush to the interface showed good lubricity and resistance to protein and cell contamination. PMPC is an attractive alternative to PE brushes.100 Based on this, Faivre et al.101 designed lubricating and wear-protection fluids via the synergistic mixture of the natural linear polyelectrolyte hyaluronic acid (HA) and a water-soluble BB polymer (Fig. 5a). The results show that, compared with salt solution, the strong cohesion of the topological polymer BB and HA mixed solution can significantly improve the wear protection of polymer surfaces under wide range of shear conditions. PMPC zwitterionic brushes, whether grafted on the surface or physically adsorbed on the surface in the form of bottle-brush polymers, have excellent lubrication and wear-resistance properties. The zwitterionic bottlebrush polymer film shows unprecedented antifouling properties to charged proteins by adsorbing charged substances. Inspired by the excellent lubrication and wear-protection properties of zwitterionic bottlebrush polymers, Adibnia et al.102 reported that zwitterionic bottle-brush polymers can be physically adsorbed in the presence of multivalent cations, and further found that the zwitterionic bottle-brush polymer still had great lubricating and anti-wear properties under the concentration of multivalent ions owing to the unique hydration properties of the zwitterionic part (Fig. 5b). In addition, these polymers are generally biocompatible, making them potential candidates for clinical applications such as artificial joint interfaces. However, these brush polymers will cleave from the surface when they are immersed in aqueous or acidic and alkaline solutions for several days, which severely limits their applications. To improve the long-term stability of the brush-like zwitterionic polymer in water and salt solutions, Yu et al.103 proposed a handy way for manufacturing robust and homogeneous PMPC brushes. Specifically, they coupled a 2-bromoisobutyl bromide (BIBB) initiator to a polyglycidyl methacrylate (PGMA)-modified substrate and used SI-ATRP to polymerize PMPC brushes. The prepared PGMA-PMPC brush shows excellent stability and lubricity in water and salt solutions, indicating their potential applications in low-fouling membrane coatings and biomedical engineering.
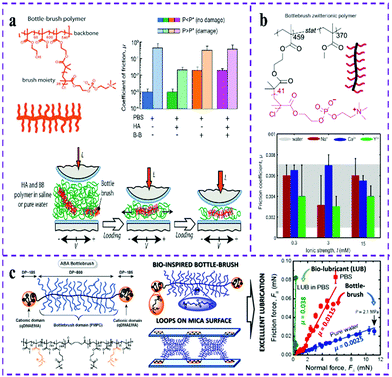 |
| Fig. 5 Schematic representation of the zwitterionic bottlebrush (BB) polymer. (a) Friction coefficient measurement and wear protection mechanism of HA and BB polymer mixtures.101 Reproduced from ref. 101 with permission from American Chemical Society. (b) Super-lubricating behavior of BB polymer in the presence of multivalent ions.102 Reproduced from ref. 102 with permission from American Chemical Society. (c) Low friction behavior of bioinspired BB polymer PDMEMA-g-PMPC.108 Reproduced from ref. 108 with permission from American Chemical Society. | |
Manufacturing hierarchical polymer brushes with antifouling and bactericidal functions can effectively improve the antibacterial effect of biological materials, which is a promising way to resist infections associated with biological materials.104,105 Since the antifouling and bactericidal graft segments of the hierarchical polymer brush are constructed in different spatial layers, this not only greatly reduces the mutual interference of the same-level grafted segments, but also promotes the synergistic effects between the layered grafted segments. Although Fu et al.106 have reported a dual-function antibacterial surface that prevents bacterial adhesion and kills bacteria, they have not studied in depth how the surface functional hierarchical structures affect the anti-adhesion and antibacterial properties of the surface. In order to construct a more effective antibacterial surface, this team successfully constructed three hierarchical polymer brushes (Si-PQAs-b-PSBMA, Si-PSBMA-b-PQAs and Si-PQAs-r-PSBMA) via the SI-ATRP polymerization of different alkyl chain lengths and zwitterionic antifouling PSBMA polymer blocks and cationic bactericidal poly(quaternary ammonium salt) (PQA) polymers. This work confirmed that the construction of hierarchical structure polymer brushes with different PQA alkyl chain lengths can effectively improve the antifouling and bactericidal properties.107
Studies have shown that, due to the highly complex architecture of proteins, the natural glycoprotein lubricin (LUB) is key to the lubrication, antiadhesion and wear protection of articular cartilage surfaces. Inspired from the brush-like structure of LUB, Israelachvili et al.108 prepared two bottle-brush polymer brushes (PDMEMA-g-PMPC and PMMA-g-PMPC) via the combination of ATRP and different post-modification techniques (Fig. 5c). The polymer block is composed of a hydrophobic (methyl methacrylate, MMA) monomer and a positively charged amine monomer (qDMAEMA). The two components are strongly adsorbed on the surface of mica through electrostatic and hydrophobic interactions. The polymer bottle-brush block consists of a flexible MMA main chain decorated with PMPC, and possesses the excellent biocompatibility and lubricity of the zwitterionic branch. Studies show that the ABA bottle-brush polymer has excellent lubrication properties.
2.5 Cyclic zwitterionic polymers
In recent decades, owing to the absence of polymer chain termini, cyclic polymers have been shown to have unique physical and chemical properties, such as a lower intrinsic viscosity, a smaller hydrodynamic volume and mean square radius of gyration, a higher glass transition temperature as well as a higher thermal stability compared with linear analogues; thus, they have attracted widespread attention from scientists. In addition, the unique steric hindrance structure of cyclic polymers can effectively resist entanglement between chains, such that the cyclic polymers show advantages in nanomedicine delivery that include but are not limited to enhanced colloidal stability and drug loading, slower degradation behavior, prolonged circulation time, lower in vitro cytotoxicity, and improved cell uptake efficiency and therapeutic effects.109–111 However, the current preparation methods for cyclic polymers are still very limited, and the synthesis and purification processes are very complicated. Therefore, it is necessary to develop a straightforward and efficient strategy for the preparation of cyclic polymers. Although there have been related reports on synthesizing cyclic polymers, these have been limited to the preparation of low-to-medium molecular weight (<10.0 kDa) cyclic polymers, and the cyclization of high molecular weight linear precursors usually produces topological by-products, such as knots or chains that arise from chain coupling. In order to alleviate the entropy challenge of coupling high molecular weight linear polymer end groups, innovative methods such as the cyclization of polymer carriers and zwitterionic ring-opening polymerization (ZROP) have emerged. N-Heterocyclic carbenes (NHC) are an effective nucleophilic reagent and a relatively poor leaving group,112 and these two characteristics are vital for the preparation of high molecular weight polymers (>1200 kDa).113 Tezuka first proposed a new strategy for the synthesis of high molecular weight cyclic polymers via ZROP (Fig. 6a).114 Zhang et al.115 synthesized cyclic poly(N-substituted glycine) via NHC-mediated N-substituted N-carboxyanhydride ZROP (Fig. 6b). The excellent biocompatibility and enhanced proteolytic stability of these polymers could be used in various biotechnologies such as drug delivery and bioactive coatings. At the same time, they synthesized a cyclic polymer backbone composed of poly(N-propargyl glycine) (c-PNPG) through NHC-mediated N-propargyl N-carboxyanhydride ZROP. However, up to now, scientists have only proposed ZROP, and the cyclic or cyclic brush polymer materials of zwitterionic have not been reported. Based on the super-lubricity of zwitterionic polymers and the enhanced steric stability of the cyclic topology, facilitating zwitterionic polymers with cyclic topology can be a promising research direction. The higher dimensional stability of the cyclic brush zwitterionic polymer material endows its super-lubricating behavior, which can provide potential application advantages for antifouling materials and joint-lubrication treatment.
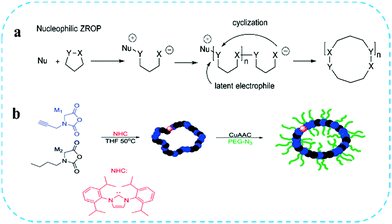 |
| Fig. 6 (a) Zwitterionic ring-opening polymerization (ZROP) to synthesize cyclic polymers and (b) cyclic brush polymers.114,115 Reproduced from ref. 114 with permission from American Chemical Society. | |
2.6 Other polymers
In addition to the topological zwitterionic polymers mentioned above, there are other types of polymer, such as spherical brushes, granular polymers, nano-microspheres, block-combs and so on.116–119 For example, Jiang and co-workers have done a lot of work on initiating the polymerization of zwitterions on a variety of planes and nanoparticle surfaces (Fig. 7a).120 They modified the ATRP initiator with siloxane groups on silicon nanoparticles, and then added different concentrations of the carboxylic betaine monomer (CBAA) to initiate polymerization. Then, two kinds of silicon nanoparticle with different particle sizes were obtained, which could be stable in protein solution for more than 72 hours. Similarly, they prepared ultra-stable PCBMA-modified gold nanoparticles. Under mild conditions, Xu and co-workers introduced the PCB copolymer on the surface of silica nanoparticles for the first time and prepared a spherical mixed charged silica brush (MCB), which could regulate the adsorption and release of protein without inactivation.116 Because the zwitterionic CBMA component has adjustable adsorption for acidic and basic proteins, the spherical charged brushes have great potential for drug loading, controlled release and immobilized enzymes. Zhou et al.121 prepared temperature-sensitive spherical polymer microgels (SB-g-NBrMGs) by grafting PSPMK onto poly(N-isopropylacrylamide) (P-NIPAM) microgels (Fig. 7b). The microgel showed an ultra-low coefficient of friction and temperature-triggered drug-release behavior. This microgel with both lubrication and controlled-drug release capabilities can be used as a joint synovial fluid for the treatment of arthritis.
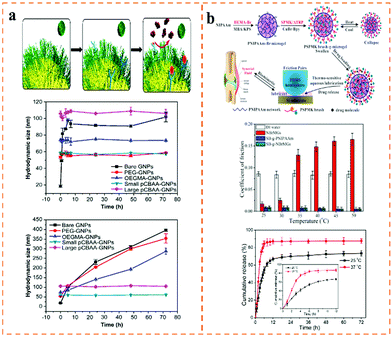 |
| Fig. 7 (a) Non-specific protein adsorption of PCBAA-GNPs.120 Reproduced from ref. 120 with permission from Elsevier. (b) Temperature-sensitive hairy spherical microgel and release profiles of aspirin-SB-g-NBrMGs in PBS.121 Reproduced from ref. 121 with permission from American Chemical Society. | |
In this section, we mainly introduced the various topological structures based on zwitterionic polymers, which can also generate materials with different morphologies, such as micelles, nanoparticles, films, and hydrogels. Among them, zwitterionic hydrogels are widely used in biomedicine, gel electrolytes, electronic sensing and other fields because of their high ionic group density, and strong hydrophilicity, affinity, and unique anti-polyelectrolyte effects. For example, Wei et al. reported two zwitterionic dextran hydrogels (CB-Dex and SB-Dex).122 Their research work confirmed that zwitterionic modified dextran can minimize bacterial adhesion, prevent wound infection, and accelerate wound healing. In addition, Lv and Wei et al.123 recently developed an anti-swelling conductive hydrogel (DN-FT-HCl) with high toughness, high compressive modulus, and high ionic conductivity, and developed an underwater strain sensor based on this hydrogel. The hydrogel sensor can accurately and reliably identify underwater motion signals. Moreover, based on the potential biomedical advantages of hydrogels, Saha et al.124 reviewed the synthesis and applications of zwitterionic microgels or nanogels developed in recent years. In the next section, we will focus on various biological applications of zwitterionic-based topological polymers.
3. Potential biomedical applications of zwitterionic-based topological polymers
Topological zwitterionic polymers have been widely used in the biomedical field due to their controllable structure, high stability, various functions, high drug loading and encapsulation efficiency, strong hydration ability, good biocompatibility and excellent anti-biological pollution characteristics. In the following, we will discuss the applications of topological zwitterionic polymers in drug delivery, antitumor therapy, biomedical diagnosis and antifouling coatings in detail.
3.1 Drug-delivery carriers
The intake of traditional drugs may have great toxicity and side effects on patients, and the effectiveness of drugs can also decrease with prolonged taking times. Therefore, how to reduce the times of administration and effectively reduce the toxic side effects become the key to drug delivery. In recent years, the developed sustained-release drug-delivery method can effectively reduce the injection frequency of administration and play a sustained and stable role in specific parts. The superhydrophilicity and anti-protein adsorption properties of zwitterionic polymers make them an important drug-delivery material. In addition, zwitterionic polymers can not only avoid rapid recognition by the immune system but can also prolong the circulation time of nanoparticles in vivo.125 Generally, drugs can be encapsulated in polymer micelles and nanogels, or covalently linked to polymer chains for drug delivery. Because the pH of tumor tissue is different from that of normal tissue, and the intracellular matrix of tumor cells contains a higher concentration of GSH, the preparation of zwitterionic polymers with pH/redox responsiveness has potential application prospects in the delivery of anticancer drugs. For example, Liu et al.126 synthesized the PLA-b-PMPC block copolymer with a controllable structure and loaded the anticancer drug doxorubicin (DOX). The copolymer can form spherical micelles with small diameters (<50 nm) to load DOX with an efficiency of 40–67%. Due to the phosphorylcholine groups, the micelles have good biocompatibility, and can effectively be delivered to cancer cells and release the drugs locally. Ni et al.127 prepared pH/redox-responsive zwitterionic polymer nanoparticles (Dex-SA NPs) using succinic acid (SA)-modified dextran (Dex) and further crosslinked them with cystine. Due to the reversible charge-conversion characteristics of the zwitterionic nanoparticles, DOX-loaded NPs showed obvious pH/redox-specific drug-release behavior to the tumor microenvironment. In addition, the NPs possessed excellent serum stability, a prolonged extracorporeal circulation time and an enhanced cellular uptake capacity. They can be used as a potential anti-cancer drug-delivery carrier for the controlled release and enhanced uptake of intracellular anti-cancer drugs. In addition to controlled drug release, the development of polymers with long circulation times and anti-immune responses is of great significance for effective drug delivery. Based on this, Lin et al.128 first synthesized four star-shaped zwitterionic polymers (CD-CBMA) with different molecular weights, and further studied the various biological properties of CD-CBMA (Fig. 8a). The results showed that the star polymer systems can resist non-specific protein adsorption more effectively than linear polymers. In addition, the star polymer with the largest molecular weight (123 kDa) has a long-circulation time (40 h), low cytotoxicity, and almost no damage and inflammation in major organ tissues, even after repeated injections. This work proved the great potential of star-shaped CD-CBMA for drug delivery. Chen et al.129 synthesized a biocompatible, novel polymer–drug conjugate containing the zwitterionic polymethacryloxyethyl (PMPC) component and camptothecin via a one-pot ATRP–click coupling strategy. The drug loading can be as high as 14% while maintaining good water solubility (>250 mg mL−1). The authors believe that the chemical bond between the PMPC backbone and the drug is the key to the controlled drug release. Luo et al.130 constructed a micellar structure with dual pH and redox response using zwitterionic copolymer materials containing multiple functional blocks for directional delivery and DOX release in the tumor microenvironment. Zwitterionic polymer-based drug-delivery systems can also be applied to the delivery of nucleic acid drugs. For example, Li et al.131 designed and synthesized a multifunctional zwitterionic block copolymer with redox responsiveness for efficient DNA delivery. Li et al.63 synthesized pH-sensitive dendritic star-shaped zwitterionic nanoparticles, PAMAM-g-PDMAPS, where these nanoparticles can effectively control the drug release and inhibit the growth of HepG2 cells. Therefore, pH-sensitive micelles of PAMAM-g-PDMAPS star-shaped zwitterionic copolymers are a promising anticancer drug-delivery carrier. In addition, Jia et al.132 modified the surface of the fifth generation polyamidoamine (PAMAM) dendrimer with acryloyloxyethyl phosphorylcholine (APC) to obtain a structure with a zwitterionic shell and a hydrophobic core. The zwitterionic shell reduces the toxicity of the G5 PAMAM dendrimer. The anticancer drug Adriamycin (ADR) is loaded into the hydrophobic core of G5-PC and can be released continuously. Cell morphology and survival experiments showed that the G5-PC drug-loaded conjugate can be effectively endocytosis, reduce toxicity and inhibit the growth of tumor cells.
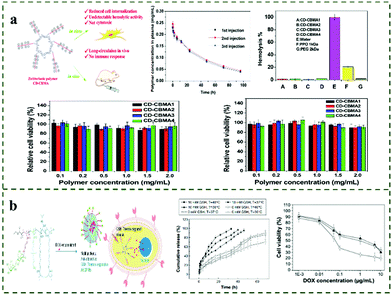 |
| Fig. 8 (a) Study on the biocompatibility of star-shaped zwitterionic polymer CD-CBMA.128 Reproduced from ref. 128 with permission from Elsevier. (b) Thermo- and GSH-triggered star nanogel for drug release.135 Reproduced from ref. 135 with permission from Dovepress. | |
In addition to the micelle structure, the recently emerging zwitterionic polymer hydrogel drug-carrier system has been widely used by researchers. Based on the low disulfide (–S–S–) bond energy, Dong and Pang et al.133 developed a reduction-responsive biodegradable PSBMA zwitterionic polymer nanogel with a long circulation time for the effective delivery of DOX at tumor sites. Based on the ultra-low contamination and functionalization properties of amino acid-derived zwitterionic polymers (PAAZ), the zwitterionic polymer nanogel can prolong the blood-circulation time in vivo. Compared with the non-degradable PSBMA nanogel, the zwitterionic nanogel showed faster DOX release under a reductive environment, indicating that the degradation of the nanogel greatly accelerates the drug release. In addition, Peng and co-workers first studied hypoxia-responsive degradable poly(phosphobetaine)-based zwitterionic nanogels.134 The nanogels showed controlled DOX-release characteristics and a negligible accelerated blood clearance (ABC) phenomenon. Moreover, Lou et al.135 synthesized thermo- and redox-sensitive star-shaped nanogels with disulfide cross-linked PNIPAM with zwitterion PSBMA as the core and PLAMA as the arm (Fig. 8b). In vitro release studies showed that the star-shaped drug-loaded nanogels had a significantly increased DOX release under high GSH and high temperature conditions, as well as increased cytotoxicity to human hepatoma cells (HepG2). Therefore, the dual-response star-shaped nanogels modified by lactose have a hepatoma-targeting ability and can be used as a specific anti-cancer drug-delivery carrier for cancer treatment. Topological zwitterionic polymers are an ideal drug-delivery material, which can effectively enhance the solubility of drugs, improve the encapsulation efficiency, prolong the drug circulation time in vivo, and reduce the toxic side effects. Furthermore, rapid release at the lesion site and ideal therapeutic effects are also pursued in practical applications.
3.2 Antitumor effects
A tumor is a medical problem that can seriously endanger human life. Tumors show great heterogeneity, genetic instability, and can have a complex pathogenesis. Tumor-treatment methods include surgery, chemotherapy, radiotherapy, targeted drug therapy, gene therapy, immunotherapy, and so on.136 In addition, differentiation-inducing agents are widely used in the treatment of various precancerous lesions and can effectively prevent the occurrence of tumors. However, this differentiation-inducing therapy is only suitable for patients with early cancerous transformation, and the preparation of the differentiation-inducing agent is difficult; the stability and safety of this therapy therefore need to be confirmed further. Moreover, is difficult to resist the complex and variable tumor environment using a single therapy, and the development of combined anti-tumor therapies is inevitable. At present, although tumor surgery has prolonged the lives of some patients to a certain extent and has improved the quality of life with a limited life span, surgery still has great limitations. Based on the shortcomings of tumor treatment mentioned above, the developed zwitterionic materials have broad application prospects in anti-tumor therapy. Based on this, Gu et al.137 synthesized zwitterionic triblock multi-acid-sensitive antitumor drug-loaded “stealth” micelles [pCBMA-b-p(ADA-TMDP)], which consist of pCBMA as the hydrophilic shell and p(ADA-TMDP) components as the hydrophobic core. The drug-loaded micelles could significantly accelerate the drug release (3.26-fold) under intracellular acidic conditions (pH 5.0) compared with the neutral environment (pH 7.4), which can effectively improve the anti-tumor effects of chemotherapy. Liu et al.69 constructed star zwitterionic polymers for the co-delivery of DOX and p53 tumor-suppressor genes (Fig. 9a). The star-shaped gene carrier had a strong DNA binding ability and an increased drug-encapsulation ability. Zwitterionic PMPD can effectively improve the serum stability and biocompatibility of the star complex. In addition, the integrated delivery vector was effectively delivered to MCF-7 cells, which significantly increased tumor cell apoptosis and an improved anti-tumor efficacy in vivo. Based on the multiple anti-tumor effects of stimulus-responsive polymeric drug carriers, Wu and co-workers developed a pH/redox dual-responsive zwitterionic polymer.138 The functional self-assembled spherical micelles can effectively encapsulate DOX, reduce the toxic and side effects, and enhance the antitumor efficacy in vivo. Moreover, Luo et al.139 synthesized a four-arm star zwitterionic polymer (4sPCLDEAS) loaded with DOX, which can induce the apoptosis of tumor spheroids and effectively inhibit their growth, where the toxicity is even equivalent to that of free DOX. This work provided a reasonable design idea for the manufacture of star-shaped drug-loaded micelle systems with high anti-tumor activities. Moreover, they further developed a pH-sensitive four-arm star zwitterionic polymer (4s-PCL-PDEASB) with lower cytotoxicity compared with linear polymers.140 The star copolymer loaded with curcumin can prolong the retention time of curcumin circulating in the blood, to effectively reduce the damage of curcumin to the liver and lungs, and showing excellent antitumor effects.
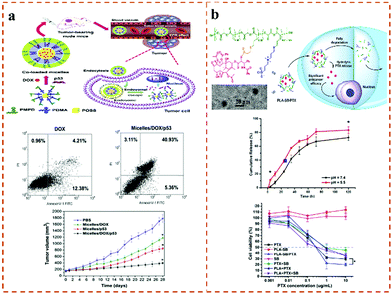 |
| Fig. 9 (a) Study on drug delivery and tumor suppression of star-shaped amphiphilic copolymer micelles.69 (b) The drug release and cytotoxicity of the biodegradable zwitterionic conjugate PLA-SB/PTX.141 Reproduced from ref. 69 and 141 with permission from Elsevier. | |
For tumor treatment, whether drug-loaded micelles can successfully enter tumor cells is the key. Tu et al.141 developed a hydrophilic star-shaped six-arm poly-ε-propiolactone-b-poly(2-methacryloxyethyl phosphorylcholine) (6sPCL-b-PMPC) block polymer, loaded with the anticancer drug paclitaxel (PTX) after self-assembly (Fig. 9b). Compared with ordinary PCL-b-PEG micelles, tumor cells can adsorb the star 6sPCL-b-PMPC micelles more effectively, and this carrier has a higher toxicity to human cervical cancer cells. Cheng et al.142 synthesized a biodegradable zwitterionic polymer with the corresponding paclitaxel (PTX) coupled with PLA-SB/PTX with biodegradable polylactide (PLA) as the main chain. This polymer drug conjugate can release PTX through biodegradation, thereby killing tumor cells and achieving the purpose of an anticancer drug.
The adsorption of serum proteins on the surface of nanoparticles will seriously affect the stability of the complex physiological and pathological microenvironment in vivo after intravenous injection. Therefore, drug-delivery systems require superhydrophilic surfaces to effectively reduce the formation of serum proteins, prolong long-term circulation in vivo, and improve the treatment of diseases via nano-formulations. Zwitterionic polymer materials have been proved to have excellent “stealth” functionality. For example, Luo and co-workers developed a Janus dendritic multifunctional zwitterionic polymer drug-delivery carrier through solid-phase peptide chemistry (SPPC).90 The high hydrophilicity of zwitterionic GPC provides DOX nano-formulations with excellent stability both in vitro and in vivo, significantly increases tumor accumulation and improves the anticancer effects. Jiang and Wu et al.143 prepared two water-soluble worm-like brush polymers with different structures using poly-3-phenylthiophene as the main chain and PEG and PCB as the side chains. The worm-like brush polymer not only effectively improved the water solubility of the conjugated polymer poly-3-phenylthiophene and prevent fluorescence aggregation-induced quenching in the aqueous phase, but also its luminescent properties endow the brush polymer with inherent biological functionality. In addition, the high-density of PEG and PCB hydrophilic side chains on the brush-shaped polymers can effectively prevent the non-specific adsorption of proteins, extend the blood circulation time, and improve the passive targeting of tumors. Aiming at the problem that it is difficult for drugs to penetrate in solid tumors, Mao et al.144 proposed a zero-waste multifunctional cooperative active drug-delivery system, which is a nanomotor PSBMA/L-Cys/a-CHCA(PCA) that is driven by zwitterion H2S (Fig. 10). Zwitterionic nanomaterials endow the nanomotor with a long cycle time through anti-protein adhesion properties. Overexpression of H2S can cause tumor-cell acidosis. At the same time, a-CHCA can further aggravate acidosis. Therefore, the synergistic effect of H2S and a-CHCA-loaded drugs induces multiple acidosis and apoptosis of tumor cells and can achieve the good inhibition of tumor growth by the zwitterionic H2S-driven nanomotor. Early and correct diagnosis and the targeted treatment of malignant tumors are the key to successful tumor treatment. Zwitterionic polymers play a vital role in the early diagnosis and treatment of malignant tumors because of their unique structures and properties. Zwitterionic polymers are functionally bonded with targeting ligands to improve the specific recognition of tumor cells, enable the early diagnosis and treatment of tumors, inhibit tumor growth, control the rapid deterioration of diseases, and greatly reduce mortality. Zwitterionic polymer materials have made great contributions to human health in the treatment of tumors.
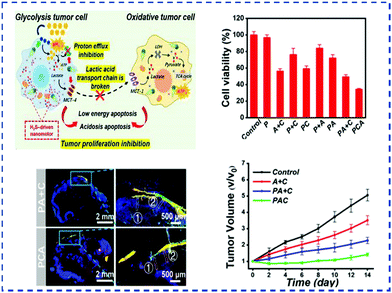 |
| Fig. 10 Synthesis process of PCA nanomotor and the treatment strategy of tumor metabolism symbiosis.114 Reproduced from ref. 114 with permission from John Wiley and Sons. | |
3.3 Biomedical diagnostics
Biomedical diagnosis and treatment are the foundation of ensuring human health and improving people's quality of life. However, the existing biomedical diagnostic and therapeutic preparations have high costs, complex preparation processes, and lack any guarantee of biological safety, which greatly restrict the development of biomedical diagnostics and therapeutics. Zwitterionic polymer materials are low cost, require mild reaction conditions and have easy surface modification. In particular, topological polymers have stable chemical structures, which is expected to solve the current problems of unguaranteed biosafety and the single limitation of existing diagnosis and treatment. At the same time, they have huge scientific research and application value in the field of biomedical diagnosis and treatment. Zhang et al.145 proved for the first time that pCBMA has both anti-biological pollution properties and easy modification based on carboxylic acid groups to fix other functional groups or biological ligands, so that pCBMA-based materials have good application prospects in medical diagnosis. Iwasaki et al.146 prepared a copolymer of thiol-terminated methacrylic acid and MPC, then modified it on a gold-coated surface plasmon resonance (SPR) chip (Fig. 11a). Biotin was introduced into the carboxyl group of the copolymer, which not only provided a good biocompatible surface but also meant that antibiotic proteins could be detected at concentrations as low as 100 mg mL−1 in plasma. Mitsubayashi et al.147 copolymerized MPC and 2-ethylhexyl methacrylate (EHMA), and then immobilized glucose oxidase (GOD) on a porous polytetrafluoroethylene (PTFE) membrane to form an enzyme membrane. They further fixed the enzyme membrane on a hydrogen peroxide electrode to prepare a flexible biosensor to achieve the characteristics of the glucose sensor. This study showed that the glucose sensor has good glucose responsiveness, where the current output range (of 0.05–1.00 mmol L−1) and the calibration range (0.14–0.23 mmol L−1) are equivalent to the glucose level in normal tears. Therefore, this work is expected to produce a non-invasive contact lens biological detector for the continuous monitoring of glucose content in tear fluid.
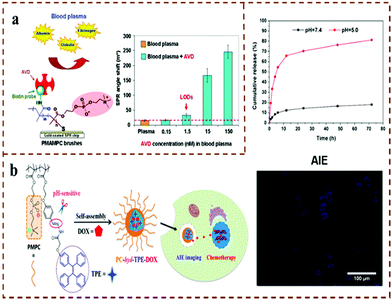 |
| Fig. 11 (a) PMAMPC brush probe detects complex matrices (such as blood plasma and clinical samples).146 Reproduced from ref. 146 with permission from American Chemical Society. (b) PMPC-hyd-TPE conjugate for AIE imaging and pH-responsive drug delivery.151 Reproduced from ref. 151 with permission from American Chemical Society. | |
Cancer is one of the diseases that endangers human life and health. Advanced cancer not only consumes a lot of financial resources, but also the adaptability of patients is poor. Therefore, the early diagnosis of cancer is extremely important.148 Studies have shown that the level of the activated leukocyte adhesion molecule (ALCAM) in cancer patients is higher than the normal level of ALCAM in serum.149 The movement of ALCAM onto a surface makes the material-specific detection of ALCAM possible. Jiang et al.150 grafted PCBAA onto silica nanoparticles to prepare PCBAA-SiP via ATRP. The results showed that the PCBAA-modified nanoparticles could be stably stored in negative/positive protein solution for 72 hours, and the particle size was almost unchanged. More importantly, PCBAA-modified silica nanoparticles can easily be functionalized with ALCAM to diagnose cancer cells in complex media. The synergistic effect of AIE fluorescence imaging and drug delivery is of great significance for the diagnosis and treatment of cancer. Based on this, Jin et al.151 synthesized a new pH-sensitive zwitterionic polymer PMPC-hyd-TPE with the AIE-active molecule TPE (Fig. 11b). Due to the “stealth” performance of PC, zwitterionic self-assembled micelles showed excellent stability and low protein adsorption. In vitro results showed that TPE is cleaved under acidic conditions, which accelerated drug release in vitro, and the PMPC-hyd-TPE drug-loaded micelles were effectively internalized and had an AIE imaging ability in HepG2 cells; moreover, in vitro imaging confirmed that the PC-hyd-TPE-DOX micelles could effectively accumulate in the tumor site and specifically release DOX. Therefore, the multifunctional micelles developed in this study with excellent antifouling, pH-sensitive drug delivery and in vitro AIE imaging properties have potential significance for the diagnosis and treatment of cancer in the future.
Zwitterionic polymer-modified magnetic nanoparticles have become potential materials for magnetic resonance imaging (MRI) diagnosis. Because zwitterionic pCBMA is rich in carboxyl groups, they can easily chemically immobilize antibodies and other biomolecules on the targeting ligand. Based on this, Zhang et al.152,153 developed the zwitterionic polymer pCBMA-coated magnetic nanoparticles pCBMA-MNPs. The pCBMA-MNPs can be stored stably in phosphate buffered saline (PBS) and undiluted serum for at least six months and can prolong the half-life of blood circulation in the body. Because MRI diagnosis can determine the location of the disease, timely and accurate diagnosis and treatment are possible. When the nanogel enters the reducing intracellular environment, Fe3O4 nanoparticles are released spontaneously, and the MRI properties are enhanced. These characteristics make zwitterionic polymer gels an ideal carrier for drug delivery and MRI diagnosis. Shen et al.154 prepared a zwitterionic polyglycerol dendrimer (PGD), which was used as an extracellular dendritic MRI contrast agent (CA) for blood pool imaging. Xie et al.155 modified the sensor of a dynamic blood glucose detector (CGMS) with a zwitterionic polymer, which effectively reduced the measurement noise and avoided frequent calibration. In addition, zwitterionic polymers can also be applied to sensors. Wiarachai et al.156 designed the Au–PPgMA65MPC35–PNA zwitterionic polymer material, which can be used as a sensor for DNA detection and can detect the most DNA sequences. Takeuchi et al.157 synthesized poly(2-methacryloxyethyl phosphorylcholine)-grafted gold nanoparticles (AuNPs). This nano-sensing system can effectively detect the concentration of CRP in human serum (as low as 1% w/w, with a detection limit of ∼50 ng mL−1). The high cost and low biological safety of existing biomedical diagnostic materials have greatly restricted the development of biomedical diagnostics. The novel zwitterionic biomedical diagnostic materials developed are not only low in price, have high biosafety and require a simple preparation process, but also have the function of magnetic imaging. Through molecular-targeted or antibody-targeted biological modification of zwitterions, the early localization, accuracy and integration of diagnosis and treatment are realized, which can promote the development of existing medical diagnosis and provide a greater guarantee for humans.
3.4 Antifouling
Biocontamination from proteins and bacteria has a great impact on biosensors, contact lenses, medical transplantation equipment and marine ships. Therefore, the inhibition of biofouling and the development of new functional antifouling materials are vital to real life. PEG and its derivatives are the most widely used anti-biological pollution materials at present.158 However, PEG materials are easily oxidized in the biological environment and have poor stability. Zwitterionic polymers have become an ideal substitute for PEG. The anti-biological pollution mechanism of zwitterionic polymer materials has been widely studied and recognized. Zwitterionic polymers have large dipole moments and abundant hydrophilic groups (such as carboxyl, sulfonic acid, amine, etc.). A large number of water molecules can be gathered around these groups, that is, a tightly bonded hydration layer will be formed on the surface of the material, which can make the material superhydrophilic, and effectively prevent the adsorption of proteins and other biomolecules on the surface of the material.159,160 Moreover, zwitterionic polymers have been used to construct various biomedical materials, such as polyurethanes, poly(vinylidene fluoride), metal materials and so on, which endows the biological substrates with excellent antifouling properties. Therefore, zwitterionic polymers have become the representative of a new generation of antifouling materials. Wang et al.161 prepared a zwitterionic macromolecular cross-linked polyurethane antifouling coating with long-lasting tolerance and long-term antifouling performance. The hydrophilicity of the PCBMA-based macromolecular cross-linking agent coating can be maintained in flowing PBS for over two weeks, and the PU coating surface treated in PBS solution after one week could still effectively resist the adsorption of proteins and bacteria. Cai et al.162 developed a PCB-based hydrogel coating that can improve the blood compatibility of activated carbon (PAC). Furthermore, an antifouling ability is essential for the development of medical metal implants. Zwitterionic block copolymers can be anchored on different metal surfaces and impart good antifouling properties. This efficient antifouling strategy is used widely in biomedical metal implants and devices. Takai et al.163 synthesized a series of poly(MPC-b-(MPTSSi-r-MPTMSi)) block copolymers, which were successfully applied to glass substrates as uniform polymer coatings. Zwitterionic block copolymers show excellent protein tolerance and a wide pH durability (pH = 2–9). Besides, the hydrophilic surface designed by the zwitterionic block copolymer coating does not require pretreatment, which indicates that it is suitable for medical and environmental purification equipment, such as biosensors, sanitary ware, and water purification. Based on the serious pollution problem of fluoropolymer membranes, Cai et al.164 developed a PVDF-g-PHPGMA-g-PSBMA hierarchical antifouling and antibacterial membrane on a polyvinylidene fluoride (PVDF) membrane through the synergistic effect of the dendritic structure of PHPGMA branches and the “superhydrophilic” properties of PSBMA brushes. The PVDF-g-PHPGMA-g-PSBMA membranes have potential value in biomedical applications because of their excellent anti-protein and bacterial adhesion ability. This research is a universal and effective method for manufacturing antifouling fluoropolymer membranes in a controlled and green manner. Moreover, Fu and co-workers et al.107 designed and constructed three different structures of hierarchical polymer brushes with antifouling and sterilization functions (Si-PQAs-b-PSBMA, Si-PSBMA-b-PQAs and Si-PQAs-r-PSBMA). The comprehensive antibacterial ability of biomaterials can be effectively improved by the polymer hierarchical brush. Kwon et al.165 successfully grafted (sultaine-co-tyramine) (PSBTA) on the surface of polyurethane via a tyrosinase-catalyzed reaction (Fig. 12a). The PSBTA-modified coating surface can effectively prevent the adsorption of fibrinogen, fibroblasts and Staphylococcus aureus. The PSBTA polyurethane coating imparts good biological inertness to the material, which can greatly reduce the probability of implantation failure for artificial medical devices. Mi et al.166 synthesized a thermosensitive multifunctional ABA triblock copolymer wound-dressing hydrogel. The middle block B contains the positively charged and hydrolyzable betaine ester as well as antibacterial drugs. The copolymer solution was applied to the wound and rapidly turned into gel at the body temperature. The release of antibacterial drugs can reduce the risk of wound infection, and the positive charge can promote cell adhesion during tissue regeneration.
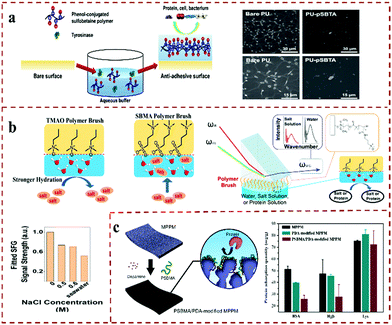 |
| Fig. 12 Zwitterionic antifouling applications. (a) Fluorescence microscope image of in vitro platelet adhesion on bare and pSBTA-grafted PU substrate.165 Reproduced from ref. 165 with permission from American Chemical Society. (b) Study on salt resistance of new type non-polluting zwitterionic polymer.168 Reproduced from ref. 168 with permission from Elsevier. (c) PSBMA/PDA co-deposition preparation of anti-fouling film surface and protein adsorption quantity.169 Reproduced from ref. 169 with permission from Elsevier. | |
With the increasing demand for biomedical antifouling, there have been many reports on the preparation of antifouling coatings based on zwitterions. For example, Zhou et al.167 prepared an imidazole-based zwitterionic polymer antifouling coating via electrochemical surface atom transfer radical polymerization (e-SIATRP). Jiang's research group designed and synthesized various types of zwitterionic antifouling coating. For example, they developed a novel zwitterionic polymer brush oxide (TMAO) antifouling material using the advantages of zwitterionic polymer hydration antifouling (Fig. 12b).168 Compared with other zwitterionic polymer antifouling materials, the pTMAO antifouling material can effectively improve the adverse effect of the addition of salt molecules on the hydration surface of the zwitterionic polymer. Even if exposed to high salt solutions, such as in seawater, the surface hydration is only slightly reduced. In addition, the pTMAO material can completely resist the adhesion of proteins in seawater. Therefore, the pTMAO material with excellent antifouling properties developed by this work can effectively resist the damage of salt and proteins on the hydrated surface. In addition, Zhou et al.169 prepared a zwitterionic polymer-modified polypropylene antifouling microporous membrane (MPPM) material via the one-step co-deposition of PSBMA and polydopamine (PDA) (Fig. 12c). Compared with the UV-induced surface-grafting method, the MPPM microporous membranes showed significantly enhanced antifouling and permeability. Based on the continuous development of zwitterionic polymer coatings, Lu and Yu et al. have also done a lot of research on this, confirming that zwitterionic coatings can be used as potential antifouling materials.170
Zwitterionic polymers also play an important role in marine biological antifouling materials. For example, Zhang et al.171 successfully developed an environmentally friendly fouling-resistance marine coating based on PSBMA for the first time. Rajan et al.172 prepared the ABA triblock copolymer PSBMA-b-PDMS-b-PSBMA through the three-step polymerization process of ring-opening polymerization (ROP), Michael addition and ATRP, and then directly incorporated it into a polyurethane coating and evaluated the stain-release performance of the coating. Studies found that the modified polyurethane coating shows an excellent fouling-release effect on bacteria and diatoms, which further proved that the migration of the PSBMA segment on the surface has an important impact on the adhesion of marine fouling organisms. Therefore, in future work, it is necessary to further adjust the composition of PSBMA to obtain a coating with wide decontamination properties. Cheng et al.173 proposed a scheme that integrates antifouling, antibacterial and biocompatibility properties, that is, they added the mild cationic antibacterial agent salicylate (SA) into the antifouling pCBMA hydrogel to prepare the poly(N,N-dimethyl-N-(ethylcarbonylmethyl)-N-[2-(methacryloyloxy)-ethyl]ammonium salicylate) (pCBMA-1 C2 SA) hydrogel. This hydrogel can achieve the long-term release of SA through ester hydrolysis, thereby inhibiting the growth of planktonic bacteria. The CBMA ester becomes an antifouling surface after complete hydrolysis, which can prevent protein adsorption or bacterial aggregation. In addition, the pCBMA-1 C2 SA hydrogel can inhibit 99% of the growth of E. coli and Staphylococcus epidermidis. Moreover, SA is a widely used anti-inflammatory drug, so the hydrogel developed in this research may also reduce skin wounds caused by inflammatory tissue and promote wound healing.
In recent years, some multifunctional zwitterionic polymer antifouling materials with stimuli responsiveness have become research hotspots. Zheng et al.174 synthesized zwitterionic polymers with salt ion responsiveness and modified it on a silicon wafer substrate to obtain a surface with adjustable friction and antifouling properties. Zuilhof et al.175,176 not only developed a new kind of zwitterionic polymer crosslinked network (ZPN) coating with self-healing and antifouling properties, but also obtained a zwitterionic coating with anti-protein and dual self-healing properties through coating zwitterionic polymer colloidal particles on different surfaces for the first time. In addition, they further systematically reviewed the recent progress of self-healing antifouling materials.177 In short, his research group has made outstanding contributions to the development of zwitterionic self-healing coatings. As a new generation of antifouling materials, zwitterionic-based topological polymers are favored for their efficient antifouling properties and broad application prospects. Through the development of functional zwitterionic antifouling coatings, the aging time of a material can be delayed, and thus the durability of the material is effectively improved and the antifouling time is prolonged. In a word, these new materials have great potential to create benefits for human life and society.
3.5 Other polymers
In addition to the above scope of applications, zwitterionic polymers are also widely used in many research fields such as protein modification,178,179 gene carriers,180–182 anticoagulant materials,183,184 hemodialyzers,185,186 cardiovascular stents,187 orthopedic materials,188,189 tissue engineering and so on.190 For example, Gao et al.191 used a site-specific in situ growth (SIG) strategy to efficiently link the zwitterionic polymer PMPC to the protein interferon-α (IFN-α) to prepare a new type of site-specific and highly active conjugate (INF-PMPC). Compared with PEG-modified interferon-alpha (PEGASYS), INF-PMPC showed a prolonged circulation half-life and excellent therapeutic efficacy. In addition, PMPC conjugates can completely inhibit the growth of tumors. Therefore, this method of synthesizing PMPC protein conjugates has provided a general platform for the development of next-generation protein therapeutic agents. Lam et al.192 synthesized the 2-(dimethylaminoethyl methacrylate and 2-methacryloyloxyethyl phosphorylcholine) (DMAEMA-MPC) diblock copolymer in which MPC blocks were used as a biocompatible steric stabilizer to maintain the stability of the complex, thus further effectively compressing DNA via a cationic block. Kim et al.187 successfully prepared a phospholipid/poly(L-lactic acid) mixture (PLLA/PMB30W) by mixing polylactic acid (PLLA) and a water-soluble amphiphilic phospholipid polymer (PMB30W). The results of the study showed that even if the (PLLA/PMB30W) blend was implanted into rat subcutaneous tissue for 6 months, it still had a high density of phosphocholine groups on the surface of the tissue without significant bio-absorption. In addition, the enzyme-linked immunosorbent assay of PLLA/PMB30W determined that this mixture can quantitatively reduce pro-inflammatory cytokines. Since the selected phosphorylcholine group could significantly reduce tissue response in vivo and in vitro, the PLLA/PMB30W blend becomes a promising material for temporary cardiovascular stent devices. Ishihara et al.189 prepared PMPC-g-(Co–Cr–Mo) copolymers on the surface of a metallic cobalt–chromium–molybdenum alloy (Co–Cr–Mo) by introducing biocompatible MPC and silane through surface grafting and surface initiation, respectively. The biocompatibility, lubricity and graft stability of the surface before and after modification were studied. The results showed that the silanized surface was soaked in PBS for 12 weeks without hydrolysis, and the lubricity was similar to that of the original joint. Therefore, the metal modified using the above method is expected to become an artificial bone-replacement material.
4. Conclusion and perspective
In this review, we have introduced the design and synthesis of zwitterionic-based polymer materials with different topologies, as well as their biomedical applications in drug delivery, antitumor therapy, biomedical diagnosis and antifouling coatings. In terms of drug delivery, block and star zwitterionic polymer drug carriers have high drug loadings and encapsulation efficiencies, low clearance rates and long circulation times. In addition, the responsive drug release of the polymer can be realized through functional modification combined with other materials that have pH, GSH, salt and other stimulus responsiveness. For antitumor activity, the smaller size and higher stability of star and dendritic zwitterionic polymers enable the rapid internalization of cells, resulting in a higher cell uptake and better antitumor effects. For biomedical diagnosis, the introduction of imaging, carbon dots and magnetic materials into zwitterionic topological polymer structures can be used for the early diagnosis and treatment of diseases. In terms of antifouling coatings, the nanoscale size and high graft density show excellent stability and lubricity, promoting the development of antifouling coatings and wear-resistant materials. To summarize, zwitterionic-based topological polymer materials have played a non-negligible role in biomedical research.
Although considerable progress in topological zwitterionic materials for biomedical applications has been well demonstrated, substantial challenges remain to be addressed in the future. First, only a few of these strategies have achieved clinical success, indicating that there are still potential limitations, such as complex synthesis processes, unclear mechanisms of drug delivery, and so on. Second, as a drug-delivery system, unexpected disintegration of drug carriers may cause an explosive release of drugs. In order to solve this problem, it is a great concern to construct a controlled-release drug system with multiple binding forces. For tumor treatment, how to achieve more accurate targeted regulation without damaging normal tissues remains a big challenge. For biomedical diagnosis, improving the sensitivity and accuracy of imaging diagnosis is still being explored. Based on the advantages of molecular brushes, brush-shaped zwitterionic polymers have made great progress in anti-fouling applications. However, their anti-fouling times are limited, and their industrial development has not yet been implemented. Hence, it is urgent to develop a sustainable antifouling coatings in complex media. Last but not least, although zwitterionic-based topological polymer materials have been greatly developed, the synthesis of cyclic and cyclic brush zwitterionic polymers is still a blank. Based on the unique advantages of cyclic polymers and zwitterionic materials, we envisage combining the cyclic topology with zwitterions and designing stimulus-responsive cyclic brush zwitterionic polymer materials, which could promote the potential development of topological zwitterionic polymer materials in the field of biomedicine. In the future, the development of zwitterion functionalization is unstoppable. With the goal of improving the multifunctional application of zwitterionic materials, reducing the costs, simplifying the processes, and promoting the clinical transformation of zwitterionic-based topological polymer systems are expected to reduce human suffering and create unlimited benefits for the economy and society.
Abbreviations
PEG | Polyethylene glycol |
PC | Phosphorylcholine |
CB | Carboxybetaine |
SB | Sulfobetaine |
PSBMA | Poly(sulfobetaine methacrylate) |
MPC | 2-Methacryloyloxyethylphosphocholine |
CBAA | Carboxybetaine acrylamide |
ATRP | Atom transfer radical polymerization |
SI-ATRP | Surface-initiated atom transfer radical polymerization |
RAFT | Reversible addition–fragmentation chain transfer |
ROP | Ring-opening polymerization |
UCST | Upper critical solution temperature |
LCST | Lower critical solution temperature |
POSS | Polyhedral oligomeric silsesquioxanes |
PDMAEMA | Poly[2(dimethylamino)ethyl methacrylate] |
PNIPAM | Poly(N-isopropylacrylamide) |
CD | Cyclodextrin |
Ada | Adamantane |
DOX | Doxorubicin |
PDA | Polydopamine |
PAMAM | Poly(amidoamine) |
HA | Hyaluronic acid |
BB | Bottle brush |
PU | Polyurethane |
GSH | Glutathione |
ROS | Reactive oxygen species |
DTT | Dithiothreitol |
Dex | Dextran |
SA | Succinic acid |
Author contributions
The manuscript was written through contributions of all authors. All authors have given approval to the final version of the manuscript.
Conflicts of interest
The authors declare that they have no known competing financial interests or personal relationships that could have appeared to influence the work reported in this paper.
Acknowledgements
Financial support from National Natural Science Foundation of China (no. 52073191, and 51925304) is gratefully acknowledged. The components in the Table of Contents image are reproduced from ref. 49, 90, 102, 115 and 151 with permission from the American Chemical Society, reproduced from ref. 74 and 116 with permission from the Royal Society of Chemistry, reproduced from ref. 169, 191 and 193 with permission from Elsevier, reproduced from ref. 135 with permission from Dove press, and reproduced from ref. 144 with permission from John Wiley and Sons.
Notes and references
- S. Chen, L. Li, C. Zhao and J. Zhen, Polymer, 2010, 51, 5283–5293 CrossRef CAS.
- A. B. Lowe and C. L. McCormick, Chem. Rev., 2002, 102, 4177–4190 CrossRef CAS PubMed.
- C. Leng, S. Sun, K. Zhang, S. Jiang and Z. Chen, Acta Biomater., 2016, 40, 6–15 CrossRef CAS PubMed.
- A. Laschewsky and A. Rosenhahn, Langmuir, 2019, 35, 1056–1071 CrossRef CAS PubMed.
- J. Meng, Z. Cao, L. Ni, Y. Zhang, X. Wang and E. Liu, J. Membr. Sci., 2014, 461, 123–129 CrossRef CAS.
- S. Chen, J. Zheng, L. Li and S. Jiang, J. Am. Chem. Soc., 2005, 127, 14473–14478 CrossRef CAS PubMed.
- V. Gaberc-Porekar and I. Zore, Curr. Opin. Drug Discovery, 2008, 11, 242–250 CAS.
- Z. Cao, L. Zhang and S. Jiang, Langmuir, 2012, 28, 11625–11632 CrossRef CAS PubMed.
- W. Lee and Y. Chen, J. Appl. Polym. Sci., 2003, 89, 1884–1889 CrossRef CAS.
- Y. Terayama, M. Kikuchi, M. Kobayashi and A. Takahara, Macromolecules, 2010, 44, 104–111 CrossRef.
- X. Zhu, M. Fryd and B. Wayland, Polym. Degrad. Stab., 2013, 98, 1173–1181 CrossRef CAS.
- E. Ostuni, R. G. Chapman, R. E. Holmlin, S. Takayama and G. M. Whitesides, Langmuir, 2001, 17, 5605–5620 CrossRef CAS.
- K. Avgoustakis, Curr. Drug Delivery, 2004, 1, 321–333 CrossRef CAS PubMed.
- J. Cheng, B. A. Teply, I. Sherifi, J. Sung, G. Luther, F. Gu, E. LevyNissenbaum, A. F. Radovic-Moreno, R. Langer and O. C. Farokhzad, Biomaterials, 2007, 28, 869–876 CrossRef CAS PubMed.
- Z. Cao and S. Jiang, Nano Today, 2012, 7, 404–413 CrossRef CAS.
- S. N. S. Alconcel, A. S. Baas and H. D. Maynard, Polym. Chem., 2011, 2, 1442–1448 RSC.
- A. J. Keefe and S. Jiang, Nature Chem., 2012, 4, 60–64 CrossRef PubMed.
- Y. Li, M. Gerth and H. Zuilhof, Langmuir, 2012, 28, 12509–12517 CrossRef CAS PubMed.
- P. R. Garay, R. E. Gewely and J. Armstrong, Expert. Opin. Drug. Delivery, 2012, 9, 1319–1323 CrossRef PubMed.
- T. Shida and H. Kiwada, Biol. Pharm. Bull., 2013, 36, 889–891 CrossRef PubMed.
- E. T. M. Dams, P. Laverman, W. J. G. Oyen, G. Storm, G. L. Scherphof, J. W. M. Van der Meer, F. H. M. Corstens and O. C. Boerman, J. Pharmacol. Exp. Ther., 2001, 292, 1071–1079 Search PubMed.
- T. Shida, S. Kashima and H. Kiwada, J. Controlled Release, 2008, 126, 162–165 CrossRef PubMed.
- I. Hamad, A. C. Hunter and J. Szebeni, Mol. Immunol., 2008, 46, 225–232 CrossRef CAS PubMed.
- M. Steiner, A. Attarbaschi and U. Kastner, Pediatr. Blood. Cancer, 2007, 49, 640–642 CrossRef CAS PubMed.
- L. Zheng, H. S. Sundaram, Z. Wei, C. Li and Z. Yuan, React. Funct. Polym., 2017, 118, 5161 CrossRef.
- T. Hasegawa, Y. Iwasaki and K. Ishihara, J. Biomed. Mater. Res., 2002, 63, 333–341 CrossRef CAS PubMed.
- L. R. Carr, H. Xue and S. Jiang, Biomaterials, 2011, 32, 961–968 CrossRef CAS PubMed.
- L. R. Carr, G. Cheng, H. Xue and S. Jiang, Langmuir, 2010, 26, 14793–14798 CrossRef CAS PubMed.
- R. S. Kane, P. Deschatelets and G. M. Whitesides, Langmuir, 2003, 19, 2388–2391 CrossRef CAS.
- J. Ladd, Z. Zhang, S. Chen, J. C. Hower and S. Jiang, Biomacromolecules, 2008, 9, 1357–1361 CrossRef CAS PubMed.
- Y. Wang, Y. Luo and O. Zhao, ACS Appl. Mater. Interfaces, 2016, 8, 19899 CrossRef CAS PubMed.
- K. H. Jung, H. J. Kim, M. H. Kim and J. C. Lee, J. Membr. Sci., 2021, 618, 118677 CrossRef CAS.
- A. Noguchi, T. Masuda, C. Chen, S. Yoshizawa, N. Isu and M. Takai, Mater. Adv., 2020, 1, 2737–2744 RSC.
- C. Cao and X. Luo, Biomaterials, 2014, 35, 4517–4524 CrossRef PubMed.
- Y. Han, Y. Qian, X. Zhou, H. Hu, X. Liu, Z. Zhou, J. Tang and Y. Shen, Polym. Chem., 2016, 7, 6354–6362 RSC.
- L. Liu, D. Zhang, B. P. Ren, X. Gong, L. Xu, Z. Feng, Y. Chang, Y. He and J. Zheng, J. Mater. Chem. B, 2020, 8, 3814–3828 RSC.
- G. Ye, J. Lee, F. Perreault and M. Elimelech, ACS Appl. Mater. Interfaces, 2015, 7, 23069–23079 CrossRef CAS PubMed.
- Y. He, X. Wan, W. Lin, J. Li, Z. Li, F. Luo, J. Li, H. Tan and Q. Fu, Biomater. Sci., 2020, 8, 6890 RSC.
- Y. He, X. Wan, K. Xiao, W. Lin, J. Li, Z. Li, F. Luo, H. Tan, J. Li and Q. Fu, Biomater. Sci., 2019, 7, 5369–5382 RSC.
- X. Wan, Y. Zhang, Y. Deng, Q. Zhang and Q. Fu, Soft Matter, 2015, 11, 4197–4207 RSC.
- T. Xiang, L. Zhang, R. Wang, Y. Xia, B. Su and C. Zhao, J. Colloid Interface Sci., 2014, 432, 47–56 CrossRef CAS PubMed.
- R. Narain, M. Ahmed, N. Bhuchar and K. Ishihara, Bioconjugate Chem., 2011, 22, 1228–1238 CrossRef PubMed.
- G. Liu, Q. Jin, X. Liu, L. Lv, C. Chen and J. Ji, Soft Matter, 2011, 7, 662–669 RSC.
- G. Liu, C. Chen and J. Ji, Soft Matter, 2012, 8, 8811–8821 RSC.
- Y. Liu, W. Peng, X. Zhang, J. Peng, P. Liu and J. Shen, J. Mater. Sci. Technol., 2021, 62, 96–106 CrossRef.
- V. Vasantha, S. Jana, A. Parthiban and J. G. Vancso, Chem. Commun., 2014, 50, 46–48 RSC.
- V. Hildebrand, A. Laschewsky, M. Päch, P. Müller-Buschbaum and C. M. Papadakis, Polym. Chem., 2017, 8, 310–322 RSC.
- L. P. Kreuzer, T. Widmann, C. Geiger, P. Wang, A. Vagias, J. E. Heger, M. Haese, V. Hildebrand, A. Laschewsky and C. M. Papadakis, Langmuir, 2021, 37, 9179–9191 CrossRef CAS PubMed.
- J. Wang, L. Wang, X. Ji, L. Liu and H. Zhao, Macromolecules, 2017, 50, 2284–2295 CrossRef CAS.
- M. E. Taylor, S. J. Lounder, A. Asatekin and M. J. Panzer, ACS Mater. Lett., 2020, 2, 261–265 CrossRef CAS.
- X. Wang, H. Gu and G. Wu, J. Dispers. Sci. Technol., 2020, 1805331 Search PubMed.
- Q. Liu, S. Anuradha and L. Liu, Biomacromolecules, 2013, 14, 226–231 CrossRef CAS PubMed.
- I. Gitsov, L. Wang, N. Vladimirov, A. Simonyan, D. J. Kiemle and A. Schutz, Biomacromolecules, 2014, 15, 4082–4095 CrossRef CAS PubMed.
- X. Pang, L. Zhao, M. Akinc, J. Kim and Z. Lin, Macromolecules, 2011, 44, 3746–3752 CrossRef CAS.
- W. Lin, G. Ma, F. Ji, J. Zhang, L. Wang, H. Sun and S. Chen, J. Mater. Chem. B, 2015, 3, 440–448 RSC.
- Y. Li, H. Guo, J. Zheng, J. Gan, Y. Zhang, X. Guan, K. Wu and M. Lu, RSC Adv., 2014, 4, 54268–54281 RSC.
- W. Cao and L. Zhu, Macromolecules, 2011, 44, 1500–1512 CrossRef CAS.
- H. Hu, X. Fan and Z. Cao, Polymer, 2005, 46, 9514–9522 CrossRef CAS.
- N. Malik, R. Wiwattanapatapee, R. Klopsch, K. Lorenz, H. Frey, J. Weener, E. Meijer, W. Paulus and R. Duncan, J. Controlled Release, 2000, 65, 133–148 CrossRef CAS PubMed.
- C. Kojima, K. Kono, K. Maruyama and T. Takagishi, Bioconjugate Chem., 2000, 11, 910–917 CrossRef CAS PubMed.
- L. Jia, J. Xu, H. Wang and J. Ji, Colloids Surf., B, 2011, 84, 49–54 CrossRef CAS PubMed.
- L. Wang, Z. Wang, G. Ma, W. Lin and S. Chen, Langmuir, 2013, 29, 8914–8921 CrossRef CAS PubMed.
- L. Li, Y. Wang, F. Ji, Y. Wen, J. Li, B. Yang and F. Yao, J. Biomater. Sci.: Polym., 2014, 25, 1641–1657 CrossRef CAS PubMed.
- L. Qiu, R. Wang, C. Zheng, Y. Jin and L. Jin, Nanomedicine, 2010, 5, 193–208 CrossRef CAS PubMed.
- D. Cordes, P. Lickiss and F. Rataboul, Chem. Rev., 2010, 110, 2081–2173 CrossRef CAS PubMed.
- Y. Yang, X. Wang, Y. Hu, H. Hu, D. Wu and F. Xu, ACS Appl. Mater. Interfaces, 2014, 6, 1044–1052 CrossRef CAS PubMed.
- K. Knop, R. Hoogenboom, D. Fischer and U. S. Schubert, Angew. Chem., Int. Ed., 2010, 49, 6288–6308 CrossRef CAS PubMed.
- S. Jiang and Z. Cao, Adv. Mater., 2010, 22, 920–932 CrossRef CAS PubMed.
- Y. Li, B. Xu, T. Bai and W. Liu, Biomaterials, 2015, 55, 12–23 CrossRef PubMed.
- Y. Pu, Z. Hou, M. Khin, R. Zamudio-Vazquez, K. Poon, H. Duan and M. B. Chan-Park, Biomacromolecules, 2017, 18, 44–55 CrossRef CAS PubMed.
- J. Seuring and S. Agarwal, ACS Macro Lett., 2013, 2, 597–600 CrossRef CAS.
- N. Morimoto, K. Muramatsu, T. Wazawa, Y. Inoue and M. Suzuki, Macromol. Rapid Commun., 2014, 35, 103–108 CrossRef CAS PubMed.
- S. Chen and S. Jiang, Adv. Mater., 2008, 20, 335–338 CrossRef CAS.
- M. Zhang, W. Shen, Q. Xiong, H. Wang, Z. Zhou, W. Chen and Q. Zhang, RSC Adv., 2015, 5, 28133–28140 RSC.
- J. A. Syrett, D. M. Haddleton, M. R. Whittake, T. P. Davis and C. Boyer, Chem. Commun., 2011, 47, 1449–1451 RSC.
- J. K. OhD, J. Siegwart and H. I. Lee, J. Am. Chem. Soc., 2007, 129, 5939–5945 CrossRef PubMed.
- Y. Zhang, W. Poon, A. J. Tavaresa, I. D. McGilvray and W. Chan, J. Controlled Release, 2016, 240, 332–348 CrossRef CAS PubMed.
- S. Lou, X. Zhang, M. Zhang, S. Ji, W. Wang, J. Zhang, C. Li and D. Kong, Int. J. Nanomed., 2017, 12, 3653–3664 CrossRef CAS PubMed.
- S. Mignani, S. El Kazzouli, M. M. Bousmina and J. P. Majoral, Chem. Rev., 2014, 114, 1327–1342 CrossRef CAS PubMed.
- J. Bugno, H. J. Hsu and S. Hong, Biomater. Sci., 2015, 3, 1025–1034 RSC.
- S. Svenson, Chem. Soc. Rev., 2015, 44, 4131–4144 RSC.
- Q. Sun, M. Radosz and Y. Shen, J. Controlled Release, 2012, 164, 156–169 CrossRef CAS PubMed.
- C. Stoffelen and J. Huskens, Nanoscale, 2015, 7, 7915–7919 RSC.
- X. Pang, Y. Jiang, Q. Xiao, A. W. Leung, H. Hua and C. Xu, J. Controlled Release, 2016, 222, 116–129 CrossRef CAS PubMed.
- D. Huang, F. Yang, X. Wang, H. Shen, Y. You and D. Wu, Polym. Chem., 2016, 7, 6154–6158 RSC.
- D. Huang, Y. Wang, F. Yang, H. Shen, Z. Weng and D. Wu, Polym. Chem., 2017, 8, 6675–6687 RSC.
- Y. Yuan, C. Mao, X. Du, J. Du, F. Wang and J. Wang, Adv. Mater., 2012, 24, 5476–5480 CrossRef CAS PubMed.
- Z. Cao and S. Jiang, Nano Today, 2012, 7, 404–413 CrossRef CAS.
- L. Wang, C. Shi, X. Wang, D. Guo, T. M. Duncan and J. Luo, Biomaterials, 2019, 215, 119233 CrossRef CAS PubMed.
- L. Wang, X. Ji, D. Guo, C. Shi and J. Luo, Mol. Pharmaceutics, 2021, 18, 2349–2359 CrossRef CAS PubMed.
- X. Li, X. Hu and T. Cai, Langmuir, 2017, 33, 4477–4489 CrossRef CAS PubMed.
- S. Lee and N. D. Spencer, Science, 2008, 319, 575 CrossRef CAS PubMed.
- U. Raviv, S. Giasson, N. Kampf, J. F. Gohy, R. Jerome and J. Klein, Nature, 2003, 425, 163–165 CrossRef CAS PubMed.
- J. Klein, E. Kumacheva, D. Mahalu, D. Perahia and L. J. Fetters, Nature, 1994, 370, 634–636 CrossRef CAS.
- M. Chen, W. H. Briscoe, S. P. Armes and J. Klein, Science, 2009, 323, 1698–1701 CrossRef CAS PubMed.
- M. Kobayashi, Y. Terayama, N. Hosaka, M. Kaido, A. Suzuki, N. Yamada, N. Torikai, K. Ishihara and A. Takahara, Soft Matter, 2007, 3, 740–746 RSC.
- W. Feng, J. L. Brash and S. Zhu, Biomaterials, 2006, 27, 847–855 CrossRef CAS PubMed.
- M. Kobayashi, Y. Terayama, M. Kikuchi and A. Takahara, Soft Matter, 2013, 9, 5138–5148 RSC.
- W. Yan, S. N. Ramakrishna, N. D. Spence and E. M. Benetti, Langmuir, 2019, 35, 11255–11264 CrossRef CAS PubMed.
- X. Banquy, J. Burdynska, D. W. Lee, K. Matyjaszewski and J. Israelachvili, J. Am. Chem. Soc., 2014, 136, 6199–6202 CrossRef CAS PubMed.
- J. Faivre, B. R. Shrestha, J. Burdynska, G. Xie, F. Moldovan, T. Delair, S. Benayoun, L. David, K. Matyjaszewski and X. Banquy, ACS Nano, 2017, 11, 1762–1769 CrossRef CAS PubMed.
- V. Adibnia, M. Olszewski, G. D. Crescenzo, K. Matyjaszewski and X. Banquy, J. Am. Chem. Soc., 2020, 142, 14843–14847 CrossRef CAS PubMed.
- Y. Yu, G. J. Vancso and S. Beer, Eur. Polym. J., 2017, 89, 221–229 CrossRef CAS.
- X. Ding, S. Duan, X. Ding, R. Liu and F. Xu, Adv. Funct. Mater., 2018, 28, 1802140 CrossRef.
- J. Zhang, W. Zhu, B. Xin, S. Lin, L. Jin and H. Wang, Biomater. Sci., 2019, 7, 3795–3800 RSC.
- W. He, Y. Zhang, J. Li, Y. Gao, F. Luo, H. Tan, K. Wang and Q. Fu, Sci. Rep., 2016, 6, 32140 CrossRef CAS PubMed.
- Y. He, X. Wan, W. Lin, J. Li, Z. Li, F. Luo, J. Li, H. Tan and Q. Fu, Biomater. Sci., 2020, 8, 6890–6902 RSC.
- X. Banquy, J. Burdynska, D. W. Lee, K. Matyjaszewski and J. Israelachvili, J. Am. Chem. Soc., 2014, 136, 6199–6202 CrossRef CAS PubMed.
- X. Qiu, F. Tanaka and F. M. Winnik, Macromolecules, 2007, 40, 7069–7071 CrossRef CAS.
- M. Zhang, Y. Liu, J. Peng, Y. Liu, F. Liu, W. Ma, L. Ma, C. Yu and H. Wei, Polym. Chem., 2020, 11, 6139–6148 RSC.
- H. Wei, L. R. Volpatti, D. L. Sellers, D. O. Maris, I. W. Andrews, A. S. Hemphill, L. W. Chan, D. S. H. Chu, P. J. Horner and S. H. Pun, Angew. Chem., Int. Ed., 2013, 52, 5377–5381 CrossRef CAS PubMed.
- T. Yamamoto and Y. Tezuka, Polym. Chem., 2011, 2, 1930–1941 RSC.
- W. Jeong, E. J. Shin, D. A. Culkin, J. L. Hedrick and R. M. Waymouth, J. Am. Chem. Soc., 2009, 131, 4884–4891 CrossRef CAS PubMed.
- Y. Tezuka, Acc. Chem. Res., 2017, 50, 2661–2672 CrossRef CAS PubMed.
- S. H. Lahasky, W. K. Serem, L. Guo, J. C. Garno and D. Zhang, Macromolecules, 2011, 44, 9063–9074 CrossRef CAS.
- K. Chen, F. Hu, H. Gu and H. Xu, J. Mater. Chem. B, 2017, 5, 435–443 RSC.
- P. Saha, R. Ganguly, X. Li, R. Das, N. K. Singha and A. Pich, Macromol. Rapid Commun., 2021, 42, 2100112 CrossRef CAS PubMed.
- M. Ajmal, S. Demirci, M. Siddiq, N. Aktas and N. Sahiner, Colloids Surf., 2015, 486, 29 CrossRef CAS.
- Z. Ding, W. He, J. Tao, W. Jiang, L. Li and T. Pan, J. Polym. Sci. Pol. Chem., 2011, 49, 2783–2789 CrossRef CAS.
- W. Yang, L. Zhang, S. Wang, A. D. White and S. Jiang, Biomaterials, 2009, 30, 5617–5621 CrossRef CAS PubMed.
- G. Liu, Z. Liu, N. Li, X. Wang, F. Zhou and W. Liu, ACS Appl. Mater. Interfaces, 2014, 6, 20452–20463 CrossRef CAS PubMed.
- X. Qiu, J. Zhang, L. Cao, Q. Jiao, J. Zhou, L. Yang, H. Zhang and Y. Wei, ACS Appl. Mater. Interfaces, 2021, 13, 7060–7069 CrossRef CAS PubMed.
- J. Ren, Y. Liu, Z. Wang, S. Chen, Y. Ma, H. Wei and S. Lv, Adv. Funct. Mater., 2021, 2107404 CrossRef.
- P. Saha, R. Ganguly, X. Li, R. Das, N. K. Singha and A. Pich, Macromol. Rapid Commun., 2021, 42, 2100112 CrossRef CAS PubMed.
- J. Fang, H. Nakamura and H. Maeda, Adv. Drug Delivery Rev., 2011, 63, 136–151 CrossRef CAS PubMed.
- G. Liu, P. Lv, C. Chen, X. Hu and J. Ji, Macromol. Chem. Phys., 2011, 212, 643–651 CrossRef CAS.
- L. Wu, L. Zhang, G. Shi and C. Ni, Mater. Sci. Eng., C, 2016, 61, 278–285 CrossRef CAS PubMed.
- W. Lin, G. Ma, F. Ji, J. Zhang, L. Wang, H. Sun and S. Chen, J. Mater. Chem. B, 2015, 3, 440–448 RSC.
- X. Chen, S. McRae, S. Parelkar and T. Emrick, Bioconjugate Chem., 2009, 20, 2331–2341 CrossRef CAS PubMed.
- M. Cai, M. Leng, A. Lu, L. He, X. Xie, L. Huang, Y. Ma, Y. Chen and X. Luo, Colloids Surf., B, 2015, 126, 1–9 CrossRef CAS PubMed.
- Y. Wen, Z. Zhang and J. Li, Adv. Funct. Mater., 2014, 24, 3874–3884 CrossRef CAS.
- L. Jia, J. Xu, H. Wang and J. Ji, Colloids Surf., B, 2011, 84, 49–54 CrossRef CAS PubMed.
- Y. Men, S. Peng, P. Yang, Q. Jiang, Y. Zhang, B. Shen, P. Dong and Z. Pang, ACS Appl. Mater. Interfaces, 2018, 10, 23509–23521 CrossRef CAS PubMed.
- S. Peng, Y. Men, R. Xie, Y. Tian and W. Yang, J. Colloid Interface Sci., 2019, 539, 19–29 CrossRef CAS PubMed.
- S. Lou, X. Zhang, W. Wang, J. Zhang, C. Li and D. Kong, Int. J. Nanomed., 2017, 12, 3653–3664 CrossRef CAS PubMed.
- J. Chen, Z. Jiao, L. Lin, Z. Guo, C. Xu, Y. Li, H. Tian and X. Chen, J. Polym. Sci., 2015, 33, 830–837 CAS.
- J. Ma, K. Kang, Q. Yi, Z. Zhang and Z. Gu, RSC Adv., 2016, 6, 64778–64790 RSC.
- Z. Wu, Z. Gan, B. Chen, F. Chen, J. Cao and X. Luo, Biomater. Sci., 2019, 7, 3190–3203 RSC.
- J. Cao, X. Xie, A. Lu, B. He, Y. Chen, Z. Gu and X. Luo, Biomaterials, 2014, 35, 4517–4524 CrossRef CAS PubMed.
- Z. Wu, M. Cai, X. Xie, L. He, L. Huang, Y. Chen and X. Luo, RSC Adv., 2015, 5, 106989–107000 RSC.
- S. Tu, Y. Chen, Y. Qiu, K. Zhu and X. Luo, Macromol. Biosci., 2011, 11, 1416–1425 CrossRef CAS PubMed.
- H. Sun, M. Y. Z. Chang, W. Cheng, Q. Wang, A. Commisso, M. Capeling and C. Cheng, Acta Biomater., 2017, 64, 290–300 CrossRef CAS PubMed.
- Y. Zhang, Z. Zhang, C. Liu, W. Chen, C. Li, W. Wu and X. Jiang, Polym Chem., 2017, 8, 1672–1679 RSC.
- M. Wan, Z. Liu, T. Li, H. Chen, Q. Wang, T. Chen, Y. Tao and C. Mao, Angew. Chem., Int. Ed., 2021, 60, 16139–16148 CrossRef CAS PubMed.
- Z. Zhang, S. Chen and S. Jiang, Biomacromolecules, 2006, 7, 3311–3315 CrossRef CAS PubMed.
- P. Akkahat, S. Kiatkamjornwong, S. I. Yusa, V. P. Hoven and Y. Iwasaki, Langmuir, 2012, 28, 5872–5881 CrossRef CAS PubMed.
- M. Chu, H. Kudo, T. Shirai, K. Miyajima, H. Saito, N. Morimoto, K. Yano, Y. Iwasaki, K. Akiyoshi and K. Mitsubayashi, Biomed. Microdevices, 2009, 11, 837–842 CrossRef CAS PubMed.
- J. L. Jameson and D. L. Longo, Obstet. Gynecol.
Surv., 2015, 70, 612–614 CrossRef.
- H. Vaisocherova, W. Yang, Z. Zhang, Z. Cao, G. Cheng, M. Piliarik, J. Homola and S. Jiang, Anal. Chem., 2008, 80, 7894–7901 CrossRef CAS PubMed.
- G. Jia, Z. Cao, H. Xue, Y. Xu and S. Jiang, Langmuir, 2009, 25, 3196–3199 CrossRef CAS PubMed.
- Y. Chen, H. Han, H. Tong, T. Chen, H. Wang, J. Ji and Q. Jin, ACS Appl. Mater. Interfaces, 2016, 8, 21185–21192 CrossRef CAS PubMed.
- L. Zhang, H. Xue, C. Gao, L. Carr, J. Wang, B. Chu and S. Jiang, Biomaterials, 2010, 31, 6582–6588 CrossRef CAS PubMed.
- L. Zhang, H. Xue, C. Gao, A. Keefe, J. Wang and S. Jiang, Biomaterials, 2011, 32, 4604–4608 CrossRef CAS PubMed.
- Y. Han, Y. Qian, X. Zhou, H. Hu, X. Liu, Z. Zhou, J. Tang and Y. Shen, Polym. Chem., 2016, 7, 6354–6362 RSC.
- X. Xie, J. C. Doloff, V. Yesilyurt, A. Sadraei, J. J. McGarrigle, M. Commis, O. Veiseh, S. Farah, D. Isa and S. Ghanis, Nat. Biomed. Eng., 2018, 2, 894–906 CrossRef CAS PubMed.
- O. Wiarachai, T. Vilaivan, Y. Iwasaki and V. P. Hoven, Langmuir, 2016, 32, 184–1194 CrossRef PubMed.
- Y. Kitayama and T. Takeuchi, Anal. Chem., 2014, 86, 5587–5594 CrossRef CAS PubMed.
- Y. Xie, M. Liu and J. Zhou, Appl. Surf. Sci., 2012, 258, 153–8159 Search PubMed.
- S. Chen, J. Zheng, L. Li and S. Jiang, J. Am. Chem. Soc., 2005, 127, 14473–14478 CrossRef CAS PubMed.
- Y. Xie, M. Liu and J. Zhou, Appl. Surf. Sci., 2012, 258, 8153–8159 CrossRef CAS.
- W. Wang, Y. Lu, J. Xie, H. Zhu and Z. Cao, Chem. Commun., 2016, 52, 4671–4674 RSC.
- N. Cai, Q. Li, J. Zhang, T. Xu, W. Zhao, J. Yang and L. Zhang, J. Colloid Interface Sci., 2017, 503, 168–177 CrossRef CAS PubMed.
- A. Noguchi, T. Masuda, C. Chen, S. Yoshizawa, N. Isu and M. Takai, Mater. Adv., 2020, 1, 2737–2744 RSC.
- X. Li, X. Hu and T. Cai, Langmuir, 2017, 33, 4477–4489 CrossRef CAS PubMed.
- H. J. Kwon, Y. Lee, L. T. Phuong, G. M. Seon, E. Kim, J. K. Park, H. Yoon and K. D. Park, Acta Biomater., 2017, 61, 169–179 CrossRef CAS PubMed.
- L. Mi, H. Xue, Y. Li and S. Jiang, Adv. Funct. Mater., 2011, 21, 4028–4034 CrossRef CAS.
- W. Zhao, Q. Ye, H. Hu, X. Wang and F. Zhou, J. Mater. Chem. B, 2014, 2, 5352–5357 RSC.
- H. Huang, C. Zhang, R. Crisci, T. Lu, H. C. Hung, M. S. J. Sajib, P. Sarker, J. Ma, T. Wei, S. Jiang and Z. Chen, J. Am. Chem. Soc., 2021, 143, 16786–16795 CrossRef CAS PubMed.
- R. Zhou, P. Ren, H. Yang and Z. Xu, J. Membr. Sci., 2014, 466, 18–25 CrossRef CAS.
- C. Zhang, J. Lu, Y. Hou, W. Xiong, K. Sheng and H. Lu, ACS Appl. Mater. Interfaces, 2018, 10, 17463–17470 CrossRef CAS PubMed.
- Z. Zhang, J. A. Finlay, L. Wang, Y. Gao, J. A. Callow, M. E. Callow and S. Jiang, Langmuir, 2009, 25, 13516–13521 CrossRef CAS PubMed.
- R. B. Bodkhe, S. J. Stafslien, J. Daniels, N. Cilz, A. J. Muelhberg, S. E. M. Thompson, M. E. Callow, J. A. Callow and D. C. Rajan, Prog. Org. Coat., 2015, 78, 369–380 CrossRef CAS.
- G. Cheng, H. Xue, G. Li and S. Jiang, Langmuir, 2010, 26, 10425–10428 CrossRef CAS PubMed.
- J. Yang, H. Chen, S. Xiao, M. Shen, F. Chen, P. Zhong and J. Zheng, Langmuir, 2015, 31, 9125–9133 CrossRef CAS PubMed.
- Z. Wang, A. E. Van, S. P. Pujari, H. Feng, J. A. Dijksman, M. M. J. Smulders and H. Zuihof, J. Mater. Chem. B, 2017, 5, 6728–6733 RSC.
- Z. Wang, G. Fei, H. Xia and H. Zuilhof, J. Mater. Chem. B, 2018, 6, 6930–6935 RSC.
- Z. Wang, L. Scheres, H. Xia and H. Zuilhof, Adv. Funct. Mater., 2020, 30, 1908098 CrossRef CAS.
- S. Liang, Y. Liu, X. Jin, G. Liu, J. Wen, L. Zhang, J. Li, X. Yuan, S. Irvin, Y. Chen and W. Chen, Nano Res., 2016, 9, 1022–1031 CrossRef CAS.
- L. Zhang, Y. Liu, G. Liu, D. Xu, S. Liang, X. Zhu, Y. Lu and H. Wang, Nano Res., 2016, 9, 2424–2432 CrossRef CAS.
- M. Ukawa, H. Akita, T. Masuda, Y. Hayashi, T. Konno, K. Ishihara and H. Harashima, Biomaterials, 2010, 31, 6355–6362 CrossRef CAS PubMed.
- F. Dai and W. Liu, Biomaterials, 2011, 32, 628–638 CrossRef CAS PubMed.
- F. Dai, P. Wang, Y. Wang, L. Tang, J. Yang, W. Liu, H. Li and G. Wang, Polymer, 2008, 49, 5322–5328 CrossRef CAS.
- W. Feng, S. Zhu, K. Ishihara and J. L. Brash, Biointerphases, 2006, 1(1), 50–60 CrossRef CAS PubMed.
- Y. J. Shih and Y. Chang, Langmuir, 2010, 26, 17286–17294 CrossRef CAS PubMed.
- T. Hasegawa, Y. Iwasaki and K. Ishihara, J. Biomed. Mater. Res., 2002, 63, 333–341 CrossRef CAS PubMed.
- Y. Iwasak, N. Nakabayash and K. Ishihara, J. Artif. Organs, 2003, 6, 260–266 CrossRef PubMed.
- H. I. Kim, K. Ishihara, S. Lee, J. H. Seo, H. Y. Kim, D. Suh, M. U. Kim, T. Konno, M. Takai and J. S. Seo, Biomaterials, 2011, 32, 2241–2247 CrossRef CAS PubMed.
- M. Kyomoto, T. Moro, K. Saiga, M. Hashimoto, H. Ito, H. Kawaguchi, Y. Takatori and K. Ishihara, Biomaterials, 2012, 33, 4451–4459 CrossRef CAS PubMed.
- M. Kyomoto, T. Moro, K. Saiga, F. Miyaji, H. Kawaguchi, Y. Takatori, K. Nakamura and K. Ishihara, Biomaterials, 2010, 31, 658–668 CrossRef CAS PubMed.
- Y. Hong, S. H. Ye, A. Nieponice, L. Soletti, D. A. Vorp and W. R. Wagner, Biomaterials, 2009, 30, 2457–2467 CrossRef CAS PubMed.
- J. Hu, G. Wang, W. Zhao and W. Gao, J. Controlled Release, 2016, 237, 71–77 CrossRef CAS PubMed.
- J. K. W. Lam, Y. M. S. P. Armes, A. L. Lewis, T. Baldwin and S. Stolnik, J. Controlled Release, 2004, 100, 293–312 CrossRef CAS PubMed.
- Z. Xiong, C. S. Alves, J. Wang, A. Li, J. Liu, M. Shen, J. Rodrigues, H. Tomas and X. Shi, Acta Biomater., 2019, 99, 320–329 CrossRef CAS PubMed.
|
This journal is © The Royal Society of Chemistry 2022 |
Click here to see how this site uses Cookies. View our privacy policy here.