DOI:
10.1039/D1TB02318G
(Paper)
J. Mater. Chem. B, 2022,
10, 2584-2596
Bio-inspired antibacterial coatings on urinary stents for encrustation prevention†
Received
22nd October 2021
, Accepted 20th December 2021
First published on 22nd December 2021
Abstract
Urinary tract infection (UTI) represents one of the most common nosocomial infections, which is mainly related to indwelling catheters or stents. In addition to the formation of biofilms to reduce antibiotic sensitivity, the urease-producing bacteria can also increase urine pH, causing Ca2+ and Mg2+ deposition and finally catheter obstruction. The prevention of UTIs and its complication (i.e., encrustation) thus is a great challenge in design of catheters and ureteral stents. In this work, a metal-catechol-assisted mussel chemistry (i.e., dopamine self-polymerization) was employed for surface functionalization of commercially available catheters with antimicrobial peptides (AMP), for the purpose of long-term anti-infection and encrustation prevention. To improve the stability of the polydopamine coating on polymeric stents, we used Cu2+-coordinated dopamine self-polymerization. Then, a cysteine-terminated AMP was introduced on the polydopamine coating through Michael addition. We found that the Cu2+-coordinated polydopamine coating showed improved stability and antibacterial effect. The cytotoxicity test confirmed that the bioinspired antibacterial coating showed good biocompatibility and no obvious toxicity. The results confirmed that the stents with AMP could in situ inhibit bacterial growth and biofilm formation, and finally reduce the deposition of struvite and hydroxyapatite crystals both in vitro and in vivo. We anticipate that this bioinspired strategy would develop a safe, stable and effective antibacterial coating on urinary tract medical devices for long-term bacterial inhibition and encrustation prevention.
1. Introduction
Catheters and ureteral stents are among the most commonly used medical devices in patients with urologic disorders.1,2 Currently, various polymeric materials including polyurethane (PU), silicone, polytetrafluoroethylene (PTFE), polyvinylchloride (PVC), and latex rubber have been widely employed to produce catheters/stents.3,4 In clinics, the stents can be used for the treatment of typical urinary diseases like urinary tract obstruction, or for renal and ureteral surgery,5 and the catheters are commonly used as an adjunct to relieve urinary incontinence and urinary retention as well as urinary diversion in bladder, prostate and urethral surgery. These devices have proven to be very effective in urinary drainage and supporting the urethra and ureters, but can also cause side effects for patients, such as discomfort, pain, haematuria, and in particular, chronic infection and subsequently encrustation.6–9
Long-term indwelling of the catheters or stents will inevitably lead to catheter associated urinary tract infections (UTIs).10–13 When the catheter or stent is surgically inserted, it inevitably destroys the protective barrier of the urinary system, and the bacteria can follow the catheter/stent retrograde into the urinary system and cause infections.14 In addition to the immediate harm of pathogens to patients, the infection can also induce chronic disease. Upon the surface attachment of bacteria, they begin to secrete an extracellular matrix, which enables the formation of a layer of biofilm on the surface of catheters/stents.15,16 Biofilms can reduce the antibiotic sensitivity. In addition, several typical pathogens in the urinary system, including Proteus mirabilis (P. mirabilis), Klebsiella pneumoniae (K. pneumoniae) and Pseudomonas aeruginosa (P. aeruginosa), produce ureases that can decompose urea with a faster rate (6 to 25 times higher) than that of other enzymes.17,18 Once excess urea was decomposed into ammonia, the pH value of urine would rapidly raise up to 8.5. In an alkaline environment, calcium phosphate and magnesium would deposit and form insoluble struvite and hydroxyapatite.4,6,19,20 Such infection-caused depositions on catheters or stents will eventually block the flow channels and cause obstruction. The depositions can gradually grow and lead to the failure of the catheters or stents. More seriously, endoscopic or open surgery may require removing the devices. Conceivably, UTIs and the complications have brought enormous burdens to patients. Current clinical treatments to ameliorate UTI mainly rely on antibiotics and regular catheter replacement. Leaving the pain caused by regular replacements aside, long-term administration of antibiotics will cause bacterial resistance, which may bring another trouble to the patients. In this context, the development of catheters/stents with antibiotic-free bactericidal ability and capability of in situ prevention of chronic UTIs and urinary encrustation is highly anticipated.
Antimicrobial peptides (AMPs) are a set of natural host defence peptides, first discovered in the study of “why the moist surface of amphibians did not permanently infect”.21 AMPs have been revealed with various functions, including behaving as immunomodulators to stimulate the accumulation of immune cells at the site of infection, controlling immune responses against a particular microorganism, showing anti-inflammatory properties, neutralizing endotoxins produced by bacteria, stimulating angiogenesis, and accelerating wound repair. Different from antibiotics with specific target molecules (e.g., peptides, proteins and DNAs), AMPs target the lipopolysaccharide layer of the cell membrane due to their unique structure of cationic and amphipathic residues. The membrane permeabilization of AMPs contributes greatly to the disruption of the bacterial cell wall, finally producing bactericidal ability.22–27 In addition, AMPs enter into the bacteria and interact with the lipid bilayer to form a transmembrane pore, which can increase the susceptibility of the antimicrobial drug.28 AMPs were also found to kill bacteria by inhibiting the intracellular functions, extracellular polymeric organisms, intracellular translocation, and inhibition of DNA/RNA/protein synthesis.29,30 Due to the wide spectrum bactericidal ability, AMPs can even inhibit the formation of biofilms that are resistant to most antibiotics.29,31 As one of the most promising alternatives to antibiotics, AMPs have shown promise to improve the outcome of medical devices with high risk of infections.
For the purpose of in situ anti-infection, surface modification of catheters or ureteral stents with AMPs represents a feasible and relatively safe strategy. The problem is that polymeric catheters or ureteral stents are relatively inert and hard to be stably modified with AMPs through traditional chemistry. In 2007, Lee et al developed a bio-inspired chemistry for surface modification based on the oxidative self-polymerization of dopamine (DPA).32 The inspiration of dopamine for surface modification derived from the coexistence of catechol and amine in mussel foot proteins to form a synergistic interface adhesion.33–36 The oxidation of dopamine results in a synergistic salt displacement of catechol and amine at the solid–liquid interface. The deposition of polydopamine (PDPA) can occur on almost all types of materials through catechol–metal coordination, electrostatic interaction, π–π interaction, hydrogen bonding and covalent reaction.37–39 Since a further step conjugation can be easily achieved through amino- or thiol-mediated Michael addition, this mussel-inspired chemistry allows functionalization of biomolecules on a variety of biomaterials.40–43 Recent studies also revealed that metal ion-assisted polydopamine adhesion can increase the coating stability and density, probably due to the metal–catechol-coordination-enhanced crosslinking network.44 In this context, we consider to use metal–catechol-assisted mussel chemistry for AMP functionalization on commercially available catheters. Specifically, a typical AMP (RWRWRWC–NH2)45,46 with a thiol group in the residue of cysteine was introduced on a Cu2+-coordinated polydopamine coating on PU ureteral stents (Scheme 1A). The stents with the AMP coating would in situ inhibit bacterial growth and biofilm formation, and finally reduce the deposition of struvite and hydroxyapatite crystals (Scheme 1B). In this work, we first optimized the bio-inspired coating and then evaluated its bactericidal and anti-crusting ability both in vitro and in vivo. We anticipate that this study would develop a safe, stable and effective antibacterial coating on urinary tract medical devices for long-term bacterial inhibition and encrustation prevention.
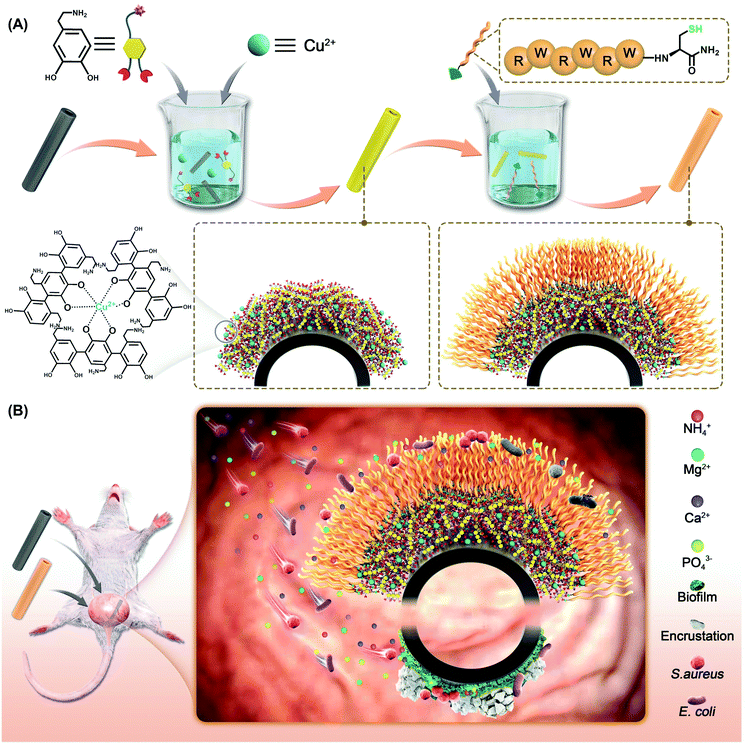 |
| Scheme 1 Schematic illustration of the bio-inspired antibacterial and anti-encrustation strategy. (A) The molecular structures of DOPA and AMPs and the synthesis process of the bio-inspired antibacterial coating. (B) The bio-inspired antibacterial coating shows anti encrustation effect. | |
2. Materials and methods
2.1 Materials
Polyurethane (PU) ureteral stents were purchased from Jingxin Medical Supplies Company (Suzhou, China). The Spring laboratory pure water system based on RO-DI step-by-step purification technology was used to produce deionized water (dH2O, 18.2 MΩ cm). Trypsin/EDTA (ethylenediaminetetraacetic acid) solution (0.25%), streptomycin, penicillin, RPMI (Roswell Park Memorial Institute) 1640 medium, MEM (minimum essential medium) and FBS (fetal bovine serum) were purchased from Gibco BRL (USA). Phosphate-buffered saline solution (PBS, 10 mmol L−1, pH = 7.4) was purchased from HyClone (USA). Cell Counting Kit-8 (CCK-8) was purchased from Beyotime Biotechnology (Shanghai, China) and was used as received. Acridine Orange (AO) and propidium iodide (PI) were purchased from Shanghai Toscience Biotechnology (Shanghai, China) and were used as received. Dopamine hydrochloride (DPA·HCl) was obtained from HEOWNS, and creatinine (99%), urea (99%), ammonium chloride (NH4Cl), sodium sulphate (Na2SO4), calcium chloride (CaCl2), monobasic potassium phosphate (KH2PO4), sodium chloride (NaCl), potassium chloride (KCl), sodium oxalate (C2Na2O4), trisodium citrate dihydrate (C6H5Na3O7·2H2O), magnesium chloride hexahydrate (MgCl2·6H2O), copper sulfate pentahydrate (CuSO4·5H2O) and tryptone were purchased from Hushi, Shanghai, China. Agar and yeast extract powder were purchased from Aladdin. LB liquid medium (1 L) containing 10 g sodium chloride, 5 g yeast extract, and 10 g tryptone and LB solid medium (1 L) containing 10 g sodium chloride, 5 g yeast extract, 10 g tryptone and 15 g aga. E. coli (ATCC 25922), S. aureus [CMCC(B) 26003] and P. mirabilis [CMCC(B)49005] were purchased from Shanghai Luwei Microbial Biotechnology (Shanghai, China). The brands and sources of all other biochemical reagents will be mentioned in the experimental methods as described below.
2.2 Preparation of a Cu-coordinated polydopamine AMP coating (PDPA@Cu–AMP)
(a) Pre-treatment of polystyrene (PS) 24-well plates.
24-Well plates were first treated with plasma (Fangrui Technology Shenzhen GD-5) to improve the wetting performance of the sample surface. The power was set at 180 W, and the treatment lasted for 300 s and repeated three times.
(b) Preparation of PDPA@Cu coatings.
Five different ratios of DPA·HCl to Cu2+ (1000
:
0, 1000
:
25, 1000
:
75, 1000
:
125, and 1000
:
175) were used for deposition of PDPA@Cu coatings on the pre-treated 24-well plates. The PDPA@Cu coated wells were named PDPA@Cu-X (X = 0, 25, 75, 125, or 175). Typically, the plasma-treated 24-well plates were first added with 1 mL of pH 8.5 PBS and 0.1 mg of DPA·HCl, and then different volumes of CuCl2 (1 mg mL−1, 0 μL, 2.5 μL 7.5 μL, 12.5 μL and 17.5 μL) were added immediately. The reaction lasted at room temperature for 48 hours. The above solution was observed to appear brown after 2 min. After 48 h, the solution became dark brown with black solid precipitation. The PDPA@Cu-coated wells were then washed with deionized water 3 times, and dried with nitrogen. For coating on PU ureteral stents, the same procedures were applied.
(c) Preparation of PDPA@Cu-X–AMP coatings.
The PDPA@Cu-coated wells were added with 0.1 mg of AMP (RWRWRWC–NH2) and 1 mL of PBS (pH 8.5). After incubation at room temperature for 24 h, the wells were gently washed with 2 mL deionized water three times, and dried with nitrogen. Finally, different antibacterial coatings (named PDPA@Cu-X–AMP, X = 0, 25, 75, 125, or 175) were obtained.
For coating on SiO2 slides, PU sheets or PU ureteral stents, the same procedures were applied according to the above three steps.
2.3 Characterization
Mass Spectrometry (MS) (Bruker solan X 70 FT-MS, Swiss) analysis was used to confirm the chemical structure of the AMP. X-ray photoelectron spectroscopy (XPS) (K-alpha, Thermo Fisher, USA), with a monochromatic Al Kα excitation source (1486.6 eV), was used to detect the surface elemental compositions of PDPA coatings and the elemental compositions of encrustations. Scanning electron microscopy (SEM) (ZEISS EVO 18) was used to characterize the surface morphology of the coating, the encrustation on stents, and the morphology of bacteria. An atomic force microscope (AFM) (Bruker Multimode 8) was used to characterize the roughness of the coating. A water contact angle meter (Dataphysics OCA20) was used to characterize the hydrophilicity of the surfaces.
2.4
In vitro antibacterial assays
(a) The effect of Cu2+ on the antibacterial ability of PDPA@Cu-X–AMP.
Bacteria (E. coli) were cultured in LB liquid medium at 37 °C. The bacterial solution was taken out, and centrifuged, the supernatant was removed, and then E. coli was suspended with normal saline and diluted to an OD value of 0.1 (UV spectrophotometer at 625 nm). According to the absorbance and approximate bacterial concentration table given by Shanghai Luwei Microbial Biotechnology, when the OD value of E. coli solution at 625 nm is 0.1, the concentration is about 1.5 × 108 CFU mL−1.
PDPA@Cu-X and PDPA@Cu-X–AMP (X = 0, 25, 75, 125, or 175) were coated on 24 well plates as described in Section 2.2. Bare 24 wells were selected as a control. Then the wells were disinfected with 75% alcohol for 5 mins, then were gently washed three times with 1 mL sterile normal saline and dried with nitrogen. E. coli (30 μL, concentration 1.5 × 105 CFU mL−1) was dropped in each well, and the wells were covered with sterilized clingfilm seals for preserving moisture. The well plate was then put in a bacterial incubator at 37 °C for 24 h. After that, each well was added with 1 mL sterilized normal saline with sufficient mixing. 100 μL of the solution from each well was taken out and put into 9.9 mL sterile normal saline. 100 μL diluted solution was put into LB solid medium and incubated at 37 °C for 24 h.
(b) Broad-spectrum antibacterial effect of PDPA@Cu-75–AMP coatings.
The coatings were grafted on to 10 mm double J stents and divided into three groups: control group, PDPA@Cu-75 coating and PDPA@Cu-75–AMP coating. Each group contained three wells. The sterilization method was the same as before. 30 μL E. coli, S. aureus and P. mirabilis (concentration 1.5 × 105 CFU mL−1) were added into three wells of each group, respectively, and then the well plates were cultured and incubated according to the above method.
(c) Stability of PDPA@Cu-75–AMP coatings.
The coating was grafted onto a 24-well plate and divided into two groups: control group and PDPA@Cu-75–AMP coating. Each group contained 10 wells. Each well was added into 1 mL sterile PBS (pH 8.5), and stored at 4 °C. One well of each group was taken out every week for a total of 10 weeks. The wells were taken out and sterilized by soaking in 0.5 mL 75% alcohol for 5 minutes. Then the wells were cleaned with 1.5 mL sterile PBS three times and dried with nitrogen. After that, E. coli (30 μL, concentration 1.5 × 105 CFU mL−1) was dropped onto the surface of the control group and PDPA@Cu-75–AMP coating group according to the method above, and incubated at 37 °C for 24 h. After 24 h the well plates were taken out and diluted 1000 times with sterile normal saline. 100 μL of diluted solution was taken onto LB solid medium for 24 h.
(d) Bacterial inhibition of PDPA@Cu-75–AMP coatings on PU stents in artificial urine.
In order to simulate the antibacterial effect of double J stents in vivo, an AMP was grafted onto 10 mm F8 (circumference 8 mm) stents and uncoated stents and PDPA@Cu-75 coating stents were added as a control. The stents were disinfected with 75% alcohol and placed into a sterile 24-well plate. One stent was put into one well and each well was put into 1 mL sterilized artificial urine (1 L contained 0.65 g CaCl2·2H2O, 0.65 g MgCl2·6H2O, 4.6 g NaCl, 2.3 g Na2SO4, 1.6 g KCl, 2.8 g KH2PO4, 1.0 g NH4Cl, 20 mg sodium oxalate, 0.65 g sodium citrate·2 H2O, 1.1 g creatinine and 25 g urea,47 sterilized with a 0.45 μm pore size filter17,48,49). 10 μL E. coli (concentration 1.5 × 107 CFU mL−1) was added to the artificial urine and cultured at 250 rpm and 37 °C. After 24 hours, half of the artificial urine was taken out and half of the fresh sterile artificial urine was added for further culture. After 1, 3, 7, and 14 days, the samples were taken out, rinsed with 2 mL sterile PBS 3 times and then placed in a 1 mL sterile normal saline centrifuge tube for 5 minutes in a 40 kHz ultrasonic bath. Then the solution was diluted 100 times, and 100 μL solution was taken for LB solid medium culture.
2.5
In vitro anti-encrustation
A total of 12 F8 stents with a length of 10 mm were selected, of which 4 were control group, 4 were PDPA@Cu-75 coating group, and the remaining 4 were PDPA@Cu-75–AMP coating group. They were weighed and placed in an in vitro bladder model; the model was composed of a 500 mL conical flask as a bladder in vitro; a rubber plug with three 5 mm holes can plug the taper bottle, two of the holes were put L type glass tube, one for artificial urine input port, the pipe orifice was 3 cm from the bottom, another tube for artificial urine output, and the orifice level was at 400 mL horizontal line. The thermometer was put in the last hole. Then a 0.5 mm diameter guide wire was used to suspend the stents in the in vitro bladder. One magnetic bead was taken into the conical flask. The in vitro bladder was placed flat on a constant temperature magnetic agitator at 37 °C and stirred at 60 rpm. Artificial urine containing 1 × 105 CFU mL−1P. mirabilis, which was stored in an ice water mixture to keep it fresh, was injected into the bladder at 0.5 mL min−1 with a peristaltic pump. In every 24 hours 10 mL artificial urine was removed from the bladder in vitro and measured with a pH meter (PHS-2F LEICI China). After 2 weeks, the stent was taken out, the loose crystallite was washed out with ultra-pure water, and then the stents were put into an oven to dry at 55 °C for 12 h. The stents were weighted after being dried. Two stents from each group were fixed with conductive adhesive and sputter-coated with gold for 20 min, and then were taken for SEM, and the other two for XPS measurements of crystal composition.
2.6 Cytotoxicity
(a) Live/dead cell staining.
Due to the small surface area of the stents, it was difficult to have sufficient area for the cytotoxicity test (followed by standard ISO 10993-5:2009). The coating was grafted onto the wells of a 24-well plate, infected with 75% alcohol and dried with nitrogen. L929 cells were cultured in 1640 + 10%FPS medium. The wells in the plate were divided into three groups, which contained control, PDPA@Cu-75 coating and PDPA@Cu-75–AMP coating, with two wells in each group. The cell counting method was used to add 1 mL of 5 × 104 L929 cells to each well. After 24 h culture, the medium was sucked out, 1 mL fresh 1640 medium +15 μl AO + 10 μl PI was added, and the wells were incubated at 37 °C for 15 min in the dark. Then the medium was sucked out, and the wells were cleaned with 1 mL sterile PBS three times, and observed under a fluorescence microscope.
(b) CCK8 (Cell-Counting Kit 8) assay.
Due to the small area of stents and the dark color of the PDPA coating, there will be a large error of OD value between the PDPA coating and the control group. The coating was grafted onto PU sheets. Therefore, 10 × 10 mm PU sheets were prepared and divided into 11 groups, which contained a control group, PDPA@Cu-X (X = 0, 25, 75, 125, and 175) group and PDPA@Cu-X–AMP, (X = 0, 25, 75, 125, and 175) group. Each PU sheet was sterile and washed with 2 mL PBS. PU sheets were then placed into sterile 24-well plates and added with 1 mL 1640 medium and 5 × 104 L929 cells for incubation in a 5% CO2 cell incubator at 37 °C for 24 hours. Each sample was removed, and the surface was cleaned with 2 mL sterile PBS three times. The unattached cells were washed off, and then put PU sheets were taken into empty well plates. 300 μL EDTA was added to each well for 3 minutes, and 600 μL 1640 medium was added to terminate the digestion of the culture. The cells were washed off the PU sheet and then the cell suspension was centrifuged at 1000 rpm for 5 minutes. The supernatant was removed and the 600 μL 1640 medium was added. Each group of the cell suspension was divided into 6 parts on average, and every part was put into one well of the 96 well plate. 10 μL CCK8 solution was added into each well of the 96 well plate. The 96 well plates were incubated in the dark for 4 hours and measured with a microplate reader for OD value at 450 nm. The CCK8 assay was taken for the control group, PDPA@Cu-75 group and PDPA@Cu-75–AMP group for 48 and 72 h with the method mentioned above.
2.7
In vivo anti-encrustation
The animal experiments were approved by the Animal Ethics Committee of the First Affiliated Hospital of Soochow University for animal experiment (202103A211) and all experiments were performed in compliance with the authors’ institute's policy on animal use and ethics. A total of 15 male Wistar/SD rats weighing 280–320 g were selected. It has been shown that rat urine is similar to human urine in terms of urine composition and crystallization behavior.48,49 And most of the encrustation is formed at both ends of the stents, that is, the renal pelvis and bladder. Therefore, the rat bladder was used as an in vivo encrustation model. The PDPA@Cu-75 and PDPA@Cu-75–AMP group coatings were grafted onto 5 mm F4 PU ureteral stents. Unmodified ureteral stents were used as a negative control. Therefore, bare stents, PDPA@Cu-75 coating and PDPA@Cu-75–AMP coating stents were examined in in vivo experiments in a rat model (n = 5). The anesthetic solution (10% chloral hydrate, 0.3 mL/100 g live weight) was injected intraperitoneally for general anesthesia, and then stents (5 mm in length) were placed into the bladder of rats and stored for 14 days (Fig. 5A). After that, 1 mL of P. mirabilis (1.5 × 105 CFU mL−1) was injected into bladders by the urethra at days 1, 4, 7 and 10. In 14 days, the rats were weighed and sacrificed. The abdomen was cut through a midline incision. The bladder was exposed and 100 μL of urine was extracted with a 1 mL sterile syringe. The urine in the bladder of the rats contained P. mirabilis that we injected before, so CLED agar plates were used for bacterial culture. The kidneys, ureter and bladder were removed completely. After macro assessment, bladder and kidney samples were preserved in 10% formalin solution (3.7% formaldehyde and 1.1% methanol) for 6 h, and then dehydrated with ethanol. After that, ethanol was cleared with xylene, and specimen was infiltrated with paraffin wax to support the tissue for thin sectioning. After the specimen was sliced and fixed on a glass slide, wax was removed by xylene and xylene substitutes. Then hematoxylin and eosin were used for hematoxylin–eosin staining (H&E), and after H&E staining, the specimen was histopathologically evaluated. After the stents were removed, cleaned and dried, SEM was used to observe the crusting of the stents.
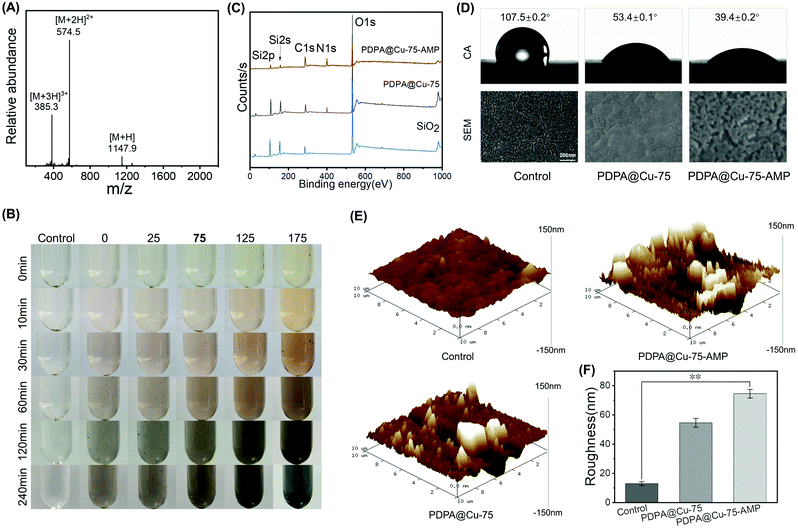 |
| Fig. 1 (A) ESI mass spectrum of AMP(RW)3. (B) The colours of PDPA with different Cu concentrations in different times. (C) XPS of bare SiO2 slides, PDPA@Cu-75 coating and PDPA@Cu-75–AMP coating. (D) Contact angle and SEM of bare PU sheets, PDPA@Cu-75 coating and PDPA@Cu-75–AMP coating on PU sheets. (E) AFM and (F) roughness of bare PU sheets, PDPA@Cu-75 coating and PDPA@Cu-75–AMP coating on PU sheets. The error bars represent SD. Statistically significant differences are indicated by *p < 0.05 or **p < 0.005 compared with the control group. | |
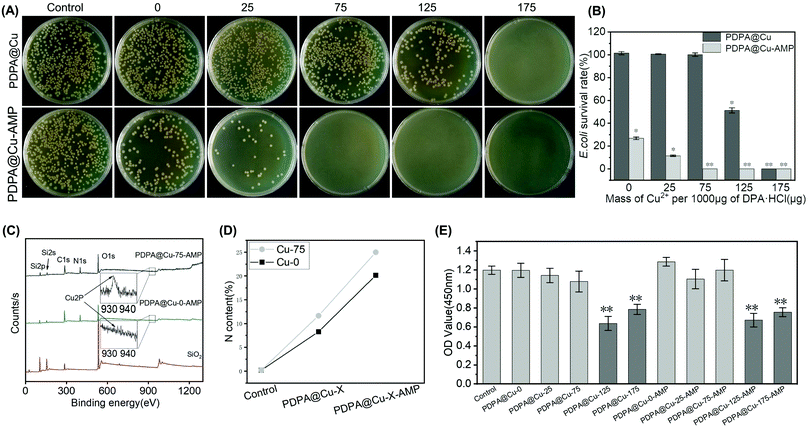 |
| Fig. 2 (A) Bacterial growth on LB solid medium and (B) E. coli survival rate after contact with the control group, PDPA@Cu with different mass ratios of DPA to Cu2+ group and PDPA@Cu–AMP coating with different mass ratios of DPA to Cu2+ group for 24 h. (C) XPS of SiO2 slides, PDPA@Cu-0–AMP and PDPA@Cu-75–AMP coatings. (D) Different N contents of control, PDPA@Cu-0/75, and PDPA@Cu-0/75–AMP coatings. (E) CCK8 assay of control, different rate of DPA to Cu2+ PDPA@Cu and PDPA@Cu–AMP coating groups. The error bars represent SD, and statistically significant differences are indicated by *p < 0.05 or **p < 0.005 compared with the control group. | |
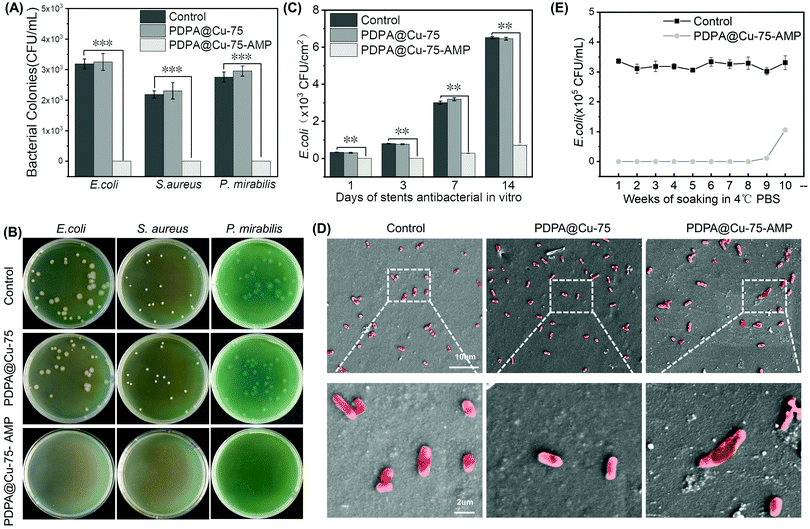 |
| Fig. 3 (A) and (B) antibacterial effect of bare stents (control), PDPA@Cu-75 and PDPA@Cu-75–AMP coatings against E. coli, S. aureus and P. mirabilis. (C) Comparison of bare stents (control), PDPA@Cu-75 and PDPA@Cu-75–AMP coating DJ stents in 2 weeks of continuous in vitro antibacterial experiment. (D) SEM of E. coli after stay on bare PU sheets, PDPA@Cu-75 and PDPA@Cu-75–AMP coating PU sheets for 24 hours. (E) Time of decomposition failure of the PDPA@Cu-75–AMP coating after soaking in PBS at 4 °C. The error bars represent SD. Statistically significant differences are indicated by **p < 0.005 or ***p < 0.001 compared with the control group. | |
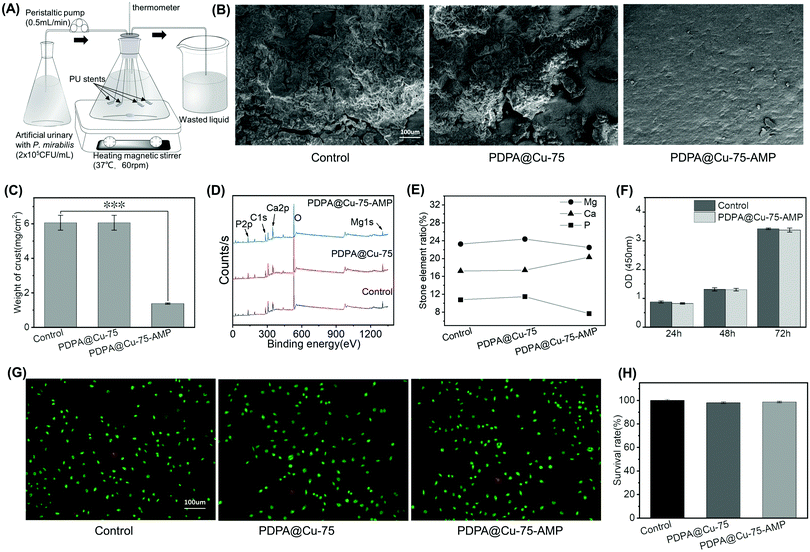 |
| Fig. 4 (A) Schematic of the in vitro bladder model. (B) SEM of bare stents, PDPA@Cu-75 and PDPA@Cu-75–AMP coating surfaces after 2 weeks of encrustation experiment in vitro. (C) Mass of crystal deposit on bare stents, PDPA@Cu-75 and PDPA@Cu-75–AMP coating surfaces after 2 weeks of encrustation experiment in vitro. (D) XPS of crystal deposit and (E) changes in the proportion of P, Ca, and Mg in bare stents, PDPA@Cu-75 and PDPA@Cu-75–AMP coating stents. (F) 24, 48, and 72 h of CCK8 assay of control and PDPA@Cu–AMP group. (G) Living and dead cell staining and (H) the survival rate of L929 cells after being cultured on bare PU sheets, PDPA@Cu-75 and PDPA@Cu-75–AMP coating PU sheets for 24 h. The error bars represent SD. Statistically significant differences are indicated by ***p < 0.001 compared with the control group. | |
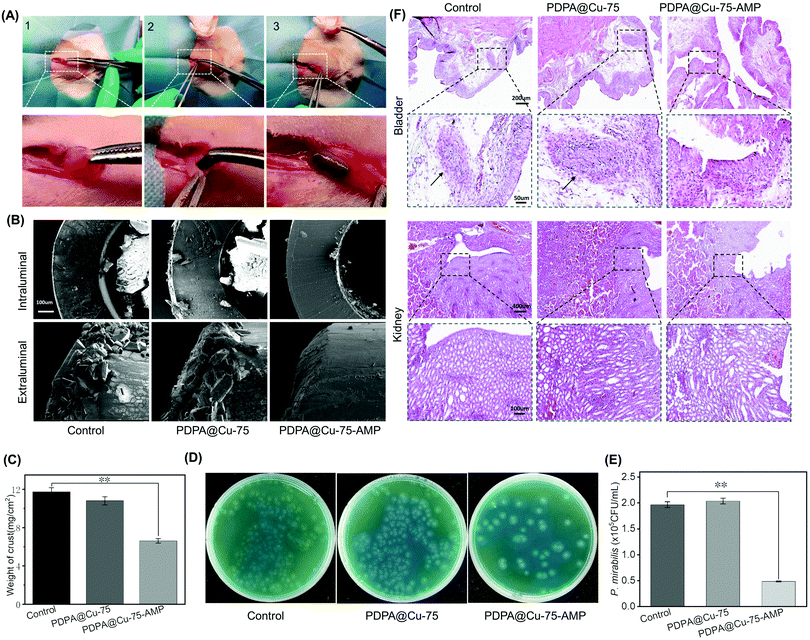 |
| Fig. 5 (A) 1 Rat bladder, 2 the stent entered the bladder from the bottom and 3 stent in the bladder. (B) SEM of rats’ intravesical stents lumen and surface and (C) the mass of deposit in control, PDPA@Cu-75 and PDPA@Cu-75–AMP coating groups. (D) Bare stent, PDPA@Cu-75 and PDPA@Cu-75–AMP coating stent group of rats’ urines cultured in CLED agar plate medium and (E) the colony counting of P. mirabilis. (F) H&E staining of pathological sections of bladder and kidneys of rats in control, PDPA@Cu-75 and PDPA@Cu-75–AMP coating groups; the arrow indicates neutrophil infiltration. The error bars represent SD. Statistically significant differences are indicated by **p < 0.005 compared with the control group. | |
2.8 Statistical analysis
The one way ANOVA test was used to evaluate the difference between sets of data in microbiology and animal experiments. Differences of statistical examinations were determined by the Tukey test. Microsoft Excel 2019 and IBM SPSS Statistics 22 were used to analyze statistics. The p value < 0.05 was considered as statistically significant.
3. Results and discussion
3.1 Preparation of PDPA@Cu–AMP coatings
To facilitate the conjugation of the AMP on the PDPA coating, here we employed a typical trivalent antibacterial dipeptide (RW)3,45,46 which was further capped with a cysteine at the C-terminal. The thiol group could be used to react with PDPA through Michael addition. Electrospray ionization mass spectrometry (ESI-MS) analysis was used to confirm the success of peptide molecular synthesis. As shown in Fig. 1A, the monoisotopic mass [M + H]+ of AMP (RWRWRWC–NH2) was found at 1147.9 Da, which was in line with its theoretical molecular weight (1147.39 Da), according to the chemical structure. The preparation of the PDPA@Cu-75–AMP coating was divided into two steps. The first step was to self-polymerize DPA into PDPA under an alkaline condition. SiO2 slides, PU sheets or stents were used to deposit polydopamine on the surface. In this work, we considered to employ Cu2+ for an improved PDPA coating. This is due to the strong coordination between Cu2+ and the catechol of DPA, which would enhance the coating density and stability. As shown in Fig. 1B, the self-polymerization of DPA and the resultant PDPA coating thickness were highly dependent on the Cu2+ concentration. At the same reaction time, the higher the Cu2+ concentration, the darker the colour of the solution observed, while with the same Cu2+ concentration, a longer reaction time led to darker colour of the solution. This result indicated the importance of the reaction time and Cu2+ content during DPA self-polymerization, which would determine the grafting thickness and stability of a PDPA@Cu-X coating. Thus, an optimization of the Cu-assisted DPA polymerization is needed.
When the first step PDPA coating was completed, the quinone bonds formed during the self-polymerization process were exposed to the surface of the coating. Upon the addition of the cysteine-capped AMP (even at room temperature), it could be grafted onto the PDPA surface to form an antibacterial coating through quinone–thiol Michael addition reaction. In order to verify that the PDPA@Cu coating and AMP could be steadily grafted onto the surface of the above materials (e.g., SiO2 slides, PU substrate or stents), we then chose a typical Cu-coordinated PDPA coating (PDPA@Cu) for AMP conjugation (PDPA@Cu–AMP). Then, XPS analysis of the untreated (control), PDPA@Cu and PDPA@Cu–AMP was performed to examine the surface chemical compositions. As shown in Fig. 1C, the N 1s signal (399.8 eV) from the surfaces of PDPA@Cu and PDPA@Cu–AMP was obviously observed, while the Si 2p and Si 2s signals from SiO2 were also shielded. In contrast, the control group showed no N 1s signal. Furthermore, due to catechol–metal coordination, the XPS spectrum also showed a peak of Cu 2p signal (will be discussed in the next section). Quantitative analysis of PDPA@Cu revealed that the contents of C, N and Cu elements were 91.84%, 7.21% and 0.95%, respectively. The next step reaction with the AMP also led to an increase of the N content (22.7%), and the C/N ratio was calculated to be 77
:
23, which was between that of a pure PDPA coating (90
:
10) and that of the AMP (73
:
27), implying the success of peptide conjugation.
The surface wettability of different surfaces was also characterized with a water contact angle meter. As shown in Fig. 1D, the PDPA@Cu coating could significantly increase the surface hydrophilicity, which was in line with previous studies.45,50,51 For example, the contact angle of a bare PU sheet was 107.5 ± 0.2°. After PDPA@Cu coating, the surface contact angle was significantly reduced to 53.4 ± 0.1°. In addition, a next step AMP conjugation further resulted in the decrease of contact angle (39.4 ± 0.2°), probably due to the high hydrophilicity of trivalent RW sequences. SEM and AFM were also used to study the surface morphology of substrates with different treatments (Fig. 1E). SEM showed that the surfaces with coatings were significantly different from a bare PU surface (Fig. 1D). It was also found that PDPA@Cu and PDPA@Cu–APM coatings showed a rougher surface than the bare PU substrate. Quantitative analysis of the AFM images confirmed the increased surface roughness of PU substrates with coatings (Fig. 1F), further confirming the success of PDPA coating. Taken together, these results jointly demonstrated that the Cu2+-assisted DPA polymerization could be efficiently used for surface modification of PU-based materials, indicating the potential for antibacterial functionalization of commercially available PU catheters.
3.2 Optimization of the PDPA@Cu–AMP coatings
In this work, Cu2+ was used to accelerate DPA self-polymerization, in order to increase the thickness of the coating, the density of AMP graft sites, and also the stability of the whole layer. Although Cu2+ possesses inherent bactericidal activity, it will also elicit significant cytotoxicity when the concentration reaches a relatively high value. Thus, it is necessary to select an appropriate Cu2+ concentration capable of not only improving the antibacterial performance of the PDPA coating but also exhibiting low or no cytotoxicity. To this end, five groups of PDAP@Cu and PDAP@Cu–AMP coatings on PU substrates with different Cu2+ to DPA ratios were prepared, and their biocompatibility and antibacterial activity were comprehensively studied. E. coli was used as the bacteria model for antibacterial assays. As shown in Fig. 2A, the PDPA coating without Cu coordination had no significant antibacterial effect. When Cu2+ was cooperated into PDPA, the E. coli colony was gradually decreased on the LB solid plate. When the Cu2+/DPA ratio increased to 175
:
1000, no E. coli could be observed on the plate, indicating a Cu2+-derived antibacterial activity of the PDPA@Cu-175 coating. Quantitative analysis further revealed that the PDPA@Cu-25 and PDPA@Cu-75 coatings had no significant antibacterial ability (Fig. 2B), implying the negligible influence of Cu2+ on bacterial survival when the Cu2+/DPA ratio was lower than 75
:
1000.
The AMP-conjugated coatings were also applied for antibacterial assays. We could find that the number of bacterial colonies was significantly reduced on the PDPA@Cu-0–AMP coating compared with the control group (PDPA@Cu-0). Unfortunately, there was still considerable bacterial growth observed. This result indicated that although the AMP molecules were grafted on the PU surface, the AMP grafting density was not enough to show a desirable antibacterial effect. In contrast, Cu2+ coordinated PDPA coatings all showed higher antibacterial activity compared to that of PDPA@Cu-0–AMP. As shown in Fig. 2A, there were only a few E. coli colonies on the surface of the PDPA@Cu-25–AMP coating, and no bacteria existed on the other groups with higher concentration of Cu coordination (i.e., the PDPA@Cu-75–AMP, PDPA@Cu-125–AMP and PDPA@Cu-175–AMP coatings). Quantitative analysis of the E. coli survival rate indicated that the groups introduced with higher concentrations of Cu2+ could thoroughly eliminate surface bacteria (Fig. 2B), implying that the AMP densities on a Cu2+-coordinated PDPA coating were significantly increased, and it could bring potent bacterial killing ability compared with the Cu2+ free control group (PDPA@Cu-0–AMP). These results verified our hypothesis that Cu2+ could enhance the self-polymerization of DPA and subsequently improve the AMP grafting efficiency.
We further analyzed the surface Cu elements using XPS. Fig. 2C showed that the Cu 2P peak (932.4 eV) was obvious in the spectrum of the PDPA@Cu-75–AMP coating, but not in the PDPA@Cu-0–AMP coating. Moreover, the N content of PDPA@Cu-75–AMP was significantly higher than that of PDPA@Cu-0–AMP (Fig. 2D), suggesting that Cu2+ participation in the self-polymerization of DPA could increase the grafting amount of the PDPA coating, and this then improved the AMP grafting density and subsequently enhanced the surface antibacterial properties. Since we have figured out the lowest Cu2+ concentration for an effective AMP conjugation, the Cu2+-derived cytotoxicity was further evaluated by a CCK8 assay. CCK8 assay is based on an electron carrier 1-methoxy 5-methylphenazine dimethyl sulfate (1-methoxy PMS), which could be reduced to a highly water-soluble yellow haze (formazan) by dehydrogenase in the cell mitochondria. The amount of haze generated was proportional to the number of living cells. Enzyme linked immunoassay (ELISA) was used to measure the light absorption value at 450 nm, which could indirectly reflect the number of living cells. The results of CCK8 assay showed that, at lower Cu2+ concentrations (e.g., PDPA@Cu-0–AMP, PDPA@Cu-25–AMP, and PDPA@Cu-75–AMP), no significant cytotoxicity was observed. In contrast, the high Cu coatings including PDPA@Cu-125, PDPA@Cu-175–AMP, PDPA@Cu-125–AMP, and PDPA@Cu-175–AMP showed an obvious inhibition of cell proliferation (L929 cells) (Fig. 2E). The results indicated that a potentially safe PDPA@Cu coating for in vivo use should be prepared using the Cu2+/DPA ratio lower than 75
:
1000.52 Since the PDPA@Cu-0–AMP and PDPA@Cu-25–AMP coatings both exhibited relatively lower antibacterial activity, in our following experiments, the PDPA@Cu-75–AMP was used as the optimal coating, because of its double advantages in biocompatibility and antibacterial ability.
3.3 Antimicrobial activity of the PDPA@Cu-75–AMP coating in vitro
The AMP (RW)3 used in this study possesses a broad spectrum of antibacterial properties. It has been revealed that the main pathogenic bacteria in urinary system infections are Gram-negative bacteria such as E. coli and P. mirabilis and Gram-positive S. aureus. Since the ultimate application of our coating is for surface modification of ureteral stents or ureter catheters, E. coli, P. mirabilis and S. aureus thus were all chosen to test the in vitro antibacterial activity of our coating. The PDPA@Cu-75–AMP coating was first prepared onto F8 ureteral stents, and then their bacterial inhibition (24 h) was tested against the three Gram-positive and Gram-negative bacteria. Compared with the bare and the AMP free PDPA@Cu-75 coated stents, the PDPA@Cu-75–AMP coated stents could significantly inhibit all kinds of bacterial growth (including E. coli, P. mirabilis and S. aureus) on the surface (Fig. 3A and B), indicating the broad spectrum of antibacterial activity. In addition, the long-term antibacterial effect of the PDPA@Cu-75–AMP coating was further tested. Our results showed that the PDPA@Cu-75–AMP coating on ureteral stents could continuously inhibit bacterial growth for two weeks (Fig. 3C). In contrast, bacteria on the bare and AMP free PDPA@Cu-75 surface showed rapid proliferation in one week. Although the antibacterial efficiency of the PDPA@Cu-75–AMP coating showed a slight decrease, it still had significantly better antibacterial activity compared to the others. The results demonstrated that our PDPA@Cu-75–AMP coating could not only immediately kill the surface bound bacteria but also enable long-term bacterial inhibition.
The morphology and membrane integrity of the surface bound bacteria (E. coli) were characterized by SEM. On the surfaces of the control group and the PDPA@Cu-75 coating group, bacteria could maintain the integrity of the cell wall (Fig. 3D). On the contrary, the bacteria on PDPA@Cu-75–AMP coated surfaces showed an incomplete cell membrane, indicating that surface conjugated antibacterial peptides could efficiently kill the bacteria contacted. The result demonstrated that the conjugated AMP on PU ureteral stents did not lose its antibacterial activity. Further in this study, ten groups of PDPA@Cu-75–AMP coatings were soaked in PBS. The antibacterial activity for each group at different weeks was tested. It was found that the PDPA@Cu-75–AMP coating could maintain excellent antibacterial properties until the 9th week (Fig. 3E), indicating the long-term stability of the AMP and the PDPA coating. This result suggested that the PDPA@Cu-75–AMP coating is very suitable for indwelling stents in the urinary system, which are always implanted in the bladder for months.
3.4 Anti-encrustation of the PDPA@Cu-75–AMP coating in vitro
The formation of encrustation on the ureteral stent surface can lead to increased infection, obstruction, and also the difficulty to remove stents. The reason for encrustation formation is mainly due to bacterial infection and biofilm formation, especially P. mirabilis, which can decompose urea, increase urine pH, and result in a microenvironment that is inclined to form a crust on ureteral stent surfaces. Since our PDPA@Cu-75–AMP-coating possesses broad-spectrum antibacterial and anti-biofilm properties, we designed an in vitro bladder model (Fig. 4A) to simulate the crusting process of ureteral stents, and evaluated the effect of our coating on encrustation prevention.
After 2 weeks of incubation in artificial urine with P. mirabilis, the thermometers, magnetic beads, glass tubes, and conical bottles were covered with a layer of white crusts; moreover, the artificial urine was turbid. The pH of artificial urine also increased from less than 5.5 to 8.5 in two weeks, and balanced to a value between 8.3 and 8.5 in the later period. The phenomenon implied the decomposition of urea by P. mirabilis, and the increased pH value would provide a favourable condition for the deposition of Mg2+ and Ca2+. On the surfaces of bare and the PDPA@Cu-75-coated stents, there were not only relatively firm attachments, but also loose stones. The stents were placed into ultra-pure water to wash away the loose stones, and then dried with nitrogen. The weight of the crust on each stent was obtained. SEM images of the stent surfaces showed that the amount of crust of the PDPA@Cu-75–AMP-coated stents was significantly less compared with that on the control and the PDPA@Cu-75-coated ones (Fig. 4B). We can clearly observe that the groups of control and the PDPA@Cu-75 were covered with a mass of encrustation, and the real surface of the polymer stents could hardly be observed. In contrast, the PDPA@Cu-75–AMP stent showed a clean surface, and only sporadic and small encrustation could be found. The average crusting weight in the control and the PDPA@Cu-75 group showed no statistical significance, while there was statistical significance for the group of PDPA@Cu-75–AMP (Fig. 4C). The crust on the PDPA@Cu-75–AMP surface was only 23% of the other two groups. Since the components of these stones contain mainly magnesium ammonium phosphate encrustation (struvite) produced by urease-producing bacteria, the contents of Ca, Mg and P in these stones were then semi-quantitatively characterized by XPS (Fig. 4D and E). XPS analysis showed that there was no significant difference in the components of these stones in control, PDPA@Cu-75, and PDPA@Cu-75–AMP groups. We found that these stones were mainly struvite. Moreover, the contents of P and Mg in the PDPA@Cu-75–AMP group were significantly less than those of the other two groups (Fig. 4F). The result implied that the PDPA@Cu-75–AMP coating had a long-term (2 week) and efficient inhibitory effect on encrustation formation induced by urease-producing bacteria, and this property is highly desired for indwelling catheters or stents.
3.5 Cytotoxicity of the PDPA@Cu-75–AMP coating
We have verified that the PDPA@Cu-75–AMP coating showed the best antibacterial and anti-encrustation performance. We then further investigated the biocompatibility of this coating prior to applying in vivo studies on its ability to inhibit bacteria and prevent encrustation. CCK8 and live/death staining experiments were performed to evaluate the cytotoxicity of the PDPA@Cu-75–AMP coating. CCK8 assay was performed on L929 cells after 24, 48 and72 hours of culture on the PDPA@Cu-75-AMP and the control surfaces. It was found that there was no significant difference in cell proliferation between the two groups in 3 days (Fig. 4F), suggesting the negligible influence of the AMP- and Cu2+-containing coating on cell growth.
Live/Dead staining was further applied to provide an intuitive cytotoxicity of the coating. Live/Dead staining is a very efficient tool to analyze the viability and adherence of cells on surfaces. The assay is based on two fluorescent dyes green AO and red PI. AO has membrane permeability and can penetrate the live cell membrane to stain nuclear DNA and RNA. PI is a DNA-binding dye with excitation and emission wavelengths of 488 nm and 630 nm, respectively. It produces red fluorescence, but has no membrane permeability, and cannot pass through living cell membranes, thus enabling the staining of only dead cells. After culture on the PDPA@Cu-75–AMP and the control surfaces for 24 h, L929 cells were stained with AO and PI. According to the staining mechanism, normal cells could be coloured with green, early apoptotic cells showed weak red fluorescence, late apoptotic cells showed enhanced red, and necrotic cells showed strong red fluorescence. It was observed that cells adhered well on the surfaces, and almost all the cells were green stained (Fig. 4G). Cell counting showed that the number of dead cells is very small, less than 1% (Fig. 4H). Moreover, the PDPA@Cu-75–AMP group showed no significant difference as compared to the bare (control) group. The result indicated that the mussel-inspired, Cu-coordinated and APM-modified coating had negligible cytotoxicity.
3.6
In vivo anti-encrustation of the PDPA@Cu-75–AMP coating
With this biocompatible, antibacterial and anti-encrustation coating in hand, we then applied it on commercially available PU stents to investigate the in vivo properties. In this study, Wistar/SD rats were used for in vivo tests because their urine and encrustation are similar to those of humans. Stents with or without the PDPA@Cu-75–AMP coating were surgically inserted into the bladder of rats followed by injection of P. mirabilis into the bladders (Fig. 5A). After 2 weeks, all the stents were removed from the bladder, and cleaned with ultrapure water. SEM was first used to check the surface crusting morphology after drying with nitrogen. As rats are living creatures, and the bladder can contract involuntarily and produce friction between the stent surface and the bladder, it is difficult to form more stones on the stent surface as the surfaces in the in vitro bladder model. Nevertheless, the stent orifice and lumen are difficult to rub and touch, which is conducive to the formation of stone in a bacteria-containing environment. SEM images showed that, in the control and the PDPA@Cu-75 group, there was plenty of encrustation in both the orifice and the lumen. Moreover, the encrustation had already blocked the stents (Fig. 5B). In contrast, in the PDPA@Cu-75–AMP group, only a few calculi formed in the orifice and the lumen, and the lumen was still unimpeded. The encrustation was then carefully removed and quantitatively analyzed. As shown in Fig. 5C, the weight of stones in the PDPA@Cu-75–AMP group was significantly reduced compared with the control and PDPA@Cu-75 group (Fig. 5C). This result was in line with that of the in vitro bladder model, in which the PDPA@Cu-75–AMP coating showed superior anti-crusting abilities because of the antibacterial activity of AMP.
To confirm the in vivo antibacterial ability of our coating, urine from the bladders of rats was also taken out for bacterial culture (Fig. 5D). It was found that bacteria on the PDPA@Cu-75–AMP coating, PDPA@Cu-75 and control groups were mainly P. mirabilis. As expected, the bacterial colony in the group of PDPA@Cu-75–AMP was significantly reduced compared with control groups (Fig. 5E), indicating the efficient antibacterial ability in vivo. The result also verified that the anti-encrustation of PDPA@Cu-75–AMP was directly related to the antibacterial ability. Although the PDPA@Cu-75–AMP coating could not completely kill bacteria in urine, it could still effectively inhibit bacterial reproduction, inhibit the development of UTIs and reduce the degree of infection.
Further in animal experiments, the bladders and kidneys from all groups of rats were examined with H&E staining (Fig. 5F). It was found that the bladder tissues in the control and PDPA@Cu-75 coating groups showed inflammation. H&E images of the control and PDPA@Cu-75 groups both had obvious infiltration of neutrophils, while in the PDPA@Cu-75–AMP group, infiltration of neutrophils in bladder tissues was significantly reduced. Moreover, no obvious abnormality was found in the renal pathological sections of all three groups. This may be because the anti-reflux function of the ureter was not damaged, and the infected urine could not retrograde into the kidney. We also inferred that the inflammatory inhibition of the PDPA@Cu-75–AMP group was due to the antibacterial ability, which reduced the intrusion of bacteria into bladder tissues and also the whole urinary system.
4. Conclusions
In summary, here we reported a Cu2+-assisted mussel-inspired strategy for surface antibacterial modification of catheters with AMP. We optimized the bio-inspired coating and then evaluated its bactericidal and anti-crusting ability. The results confirmed that Cu2+-coordinated antibacterial coating showed improved stability, antibacterial effect, and good biocompatibility both in vitro and in vivo. More importantly, the mussel-inspired, Cu2+-coordinated, AMP-modified stents could in situ inhibit bacterial growth and biofilm formation, showing long-term anti-infection and encrustation prevention in vivo. The developed bioinspired strategy in this work may show promise for fabrication of efficient antibacterial surfaces on urinary tract medical devices with long-term bacterial inhibitory effects and encrustation prevention ability.
Author contributions
Qin Yao: methodology, investigation, data curation and writing original draft. Binghai Chen: conceptualization, methodology, investigation, data curation, and writing original draft. Jiaxiang Bai: conceptualization, resources, and supervision. Wenbo He: formal analysis and visualization. Xu Chen: visualization and investigation. Dechun Geng: conceptualization, resources, and supervision. Guoqing Pan: conceptualization, resources, supervision, writing, review & editing and project administration.
Conflicts of interest
There are no conflicts to declare.
Acknowledgements
We greatly acknowledge the financial support from the National Key Research and Development Program of China (2019YFA0112000), the National Natural Science Foundation of China (21875092 and 82072425), the Natural Science Foundation of Jiangsu Province (BK20211123), the Social Development Foundation of Zhenjiang (SH2021033), the Innovation and Entrepreneurship Program of Jiangsu Province, and the “Six Talent Peaks” program of Jiangsu Province (2018-XCL-013), and the Special Project of Diagnosis and Treatment for Clinical Diseases of Suzhou (LCZX202003).
Notes and references
- F. Gong, X. Cheng, S. Wang, Y. Zhao, Y. Gao and H. Cai, Acta Biomater., 2010, 6, 534–546 CrossRef CAS PubMed.
- J. Zhao, Z. Cao, H. Lin, H. Yang, J. Li, X. Li, B. Zhang and K. Yang, J. Mater. Sci.: Mater. Med., 2019, 30, 83 CrossRef PubMed.
- P. Singha, J. Locklin and H. Handa, Acta Biomater., 2017, 50, 20–40 CrossRef CAS PubMed.
- A. Mosayyebi, C. Manes, D. Carugo and B. K. Somani, Curr. Urol. Rep., 2018, 19, 35 CrossRef PubMed.
- B. Saltzman, Urol. Clin. North Am., 1988, 15, 481–491 CrossRef CAS.
- D. J. Stickler, J. Intern. Med., 2014, 276, 120–129 CrossRef CAS PubMed.
- I. Scarneciu, S. Lupu, C. Pricop and C. Scarneciu, Pak. J. Med. Sci., 2015, 31, 522–526 Search PubMed.
- G. Giannarini, F. X. Keeley, Jr., F. Valent, F. Manassero, A. Mogorovich, R. Autorino and C. Selli, BJU Int., 2011, 107, 648–654 CrossRef PubMed.
- I. Singh, N. P. Gupta, A. K. Hemal, M. Aron, A. Seth and P. N. Dogra, Urology, 2001, 58, 526–531 CrossRef CAS PubMed.
- C. V. Gould, C. A. Umscheid, R. K. Agarwal, G. Kuntz, D. A. Pegues and C. Healthcare Infection Control Practices Advisory, Infect. Control Hosp. Epidemiol., 2010, 31, 319–326 CrossRef PubMed.
- D. G. Maki and P. A. Tambyah, Emerging Infect. Dis., 2001, 7, 342–347 CrossRef CAS PubMed.
- J. W. Warren, L. Steinberg, J. R. Hebel and J. H. Tenney, Arch. Intern. Med., 1989, 149, 1535–1537 CrossRef CAS PubMed.
- M. Wang and T. Tang, J. Orthop. Translat., 2019, 17, 42–54 CrossRef PubMed.
- S. M. Jacobsen, D. J. Stickler, H. L. Mobley and M. E. Shirtliff, Clin. Microbiol. Rev., 2008, 21, 26–59 CrossRef CAS PubMed.
- M. Ramstedt, I. A. C. Ribeiro, H. Bujdakova, F. J. M. Mergulhao, L. Jordao, P. Thomsen, M. Alm, M. Burmolle, T. Vladkova, F. Can, M. Reches, M. Riool, A. Barros, R. L. Reis, E. Meaurio, J. Kikhney, A. Moter, S. A. J. Zaat and J. Sjollema, Macromol. Biosci., 2019, 19, e1800384 CrossRef PubMed.
- R. Wasfi, S. M. Hamed, M. A. Amer and L. I. Fahmy, Front. Cell. Infect. Microbiol., 2020, 10, 414 CrossRef CAS PubMed.
- K. Belfield, X. Chen, E. F. Smith, W. Ashraf and R. Bayston, Acta Biomater., 2019, 90, 157–168 CrossRef CAS PubMed.
- B. D. Jones and H. L. Mobley, Infect. Immun., 1987, 55, 2198–2203 CrossRef CAS PubMed.
- V. Zumstein, P. Betschart, W. C. Albrich, M. T. Buhmann, Q. Ren, H. P. Schmid and D. Abt, Swiss Med. Wkly., 2017, 147, w14408 Search PubMed.
- A. Torzewska and A. Rozalski, Microbiol. Res., 2014, 169, 579–584 CrossRef CAS PubMed.
- J. M. Ageitos, A. Sánchez-Pérez, P. Calo-Mata and T. G. Villa, Biochem. Pharmacol., 2017, 133, 117–138 CrossRef CAS PubMed.
- C. Monteiro, F. Costa, A. M. Pirttila, M. V. Tejesvi and M. C. L. Martins, Sci. Rep., 2019, 9, 10753 CrossRef PubMed.
- M. Zasloff, Nature, 2002, 415, 389–395 CrossRef CAS PubMed.
- S. A. Baltzer and M. H. Brown, J. Mol. Microbiol. Biotechnol., 2011, 20, 228–235 CrossRef CAS PubMed.
- J. Fernebro, Drug Resist. Updates, 2011, 14, 125–139 CrossRef PubMed.
- G. Laverty, S. P. Gorman and B. F. Gilmore, Int. J. Mol. Sci., 2011, 12, 6566–6596 CrossRef CAS PubMed.
- M. D. Seo, H. S. Won, J. H. Kim, T. Mishig-Ochir and B. J. Lee, Molecules, 2012, 17, 12276–12286 CrossRef CAS PubMed.
- K. A. Brogden, Nat. Rev. Microbiol., 2005, 3, 238–250 CrossRef CAS PubMed.
- A. C. Rios, C. G. Moutinho, F. C. Pinto, F. S. Del Fiol, A. Jozala, M. V. Chaud, M. M. Vila, J. A. Teixeira and V. M. Balcao, Microbiol. Res., 2016, 191, 51–80 CrossRef CAS PubMed.
- M. R. Yeaman and N. Y. Yount, Pharmacol. Rev., 2003, 55, 27–55 CrossRef CAS PubMed.
- J. M. Sierra, E. Fusté, F. Rabanal, T. Vinuesa and M. Viñas, Expert Opin. Biol. Ther., 2017, 17, 663–676 CrossRef PubMed.
- H. Lee, B. P. Lee and P. B. Messersmith, Nature, 2007, 448, 338–341 CrossRef CAS PubMed.
- G. P. Maier, M. V. Rapp, J. H. Waite, J. N. Israelachvili and A. Butler, Science, 2015, 349, 628–632 CrossRef CAS PubMed.
- J. H. Ryu, P. B. Messersmith and H. Lee, ACS Appl. Mater. Interfaces, 2018, 10, 7523–7540 CrossRef CAS PubMed.
- J. Liebscher, R. Mrowczynski, H. A. Scheidt, C. Filip, N. D. Hadade, R. Turcu, A. Bende and S. Beck, Langmuir, 2013, 29, 10539–10548 CrossRef CAS PubMed.
- M. d'Ischia, A. Napolitano, V. Ball, C. T. Chen and M. J. Buehler, Acc. Chem. Res., 2014, 47, 3541–3550 CrossRef PubMed.
- J. Yang, M. A. Cohen Stuart and M. Kamperman, Chem. Soc. Rev., 2014, 43, 8271–8298 RSC.
- J. Yu, Y. Kan, M. Rapp, E. Danner, W. Wei, S. Das, D. R. Miller, Y. Chen, J. H. Waite and J. N. Israelachvili, Proc. Natl. Acad. Sci. U. S. A., 2013, 110, 15680–15685 CrossRef CAS PubMed.
- S. A. Mian, L. M. Yang, L. C. Saha, E. Ahmed, M. Ajmal and E. Ganz, Langmuir, 2014, 30, 6906–6914 CrossRef CAS PubMed.
- D. Song, L. Chen, T. Li and Z. R. Xu, Colloids Interface Sci. Commun., 2021, 40, 100340 CrossRef CAS.
- X. Chen, Y. Gao, Y. Wang and G. Pan, Smart Mater. Med., 2021, 2, 26–37 CrossRef.
- Y. Xiao, W. Wang, X. Tian, X. Tan and Z. Yang, Research, 2020, 2020, 1–12 Search PubMed.
- J. Bai, H. Wang, H. Chen, G. Ge and D. Geng, Biomaterials, 2020, 255, 120197 CrossRef CAS PubMed.
- Y. Yang, P. Gao, J. Wang, Q. Tu, L. Bai, K. Xiong, H. Qiu, X. Zhao, M. F. Maitz, H. Wang, X. Li, Q. Zhao, Y. Xiao, N. Huang and Z. Yang, Research, 2020, 2020, 9203906 CAS.
- Y. Liu, K. Ai and L. Lu, Chem. Rev., 2014, 114, 5057–5115 CrossRef CAS PubMed.
- T. K. Das, S. Ganguly, S. Ghosh, S. Remanan and N. C. Das, Colloids Interface Sci. Commun., 2019, 33, 100218 CrossRef CAS.
- D. P. Griffith, D. M. Musher and C. Itin, Invest. Urol., 1976, 13, 346–350 CAS.
- F. Atmani and S. R. Khan, Urol. Res., 1995, 23, 95–101 CrossRef CAS PubMed.
- O. Miyake, T. Yoshioka, K. Yoshimura, M. Honda, S. Yamaguchi, T. Koide and A. Okuyama, Br. J. Urol., 1998, 81, 14–19 CAS.
- N. Shahkaramipour, T. N. Tran, S. Ramanan and H. Lin, Membranes, 2017, 7, 13 CrossRef PubMed.
- D. J. Miller, D. R. Dreyer, C. W. Bielawski, D. R. Paul and B. D. Freeman, Angew. Chem., Int. Ed., 2017, 56, 4662–4711 CrossRef CAS PubMed.
- J. Long, B. Teng, W. Zhang, L. Li, M. Zhang, Y. Chen, Z. Yao, X. Meng, X. Wang, L. Qin and Y. Lai, Biomater. Transl., 2021, 272–284 Search PubMed.
Footnotes |
† Electronic supplementary information (ESI) available. See DOI: 10.1039/d1tb02318g |
‡ These authors contributed equally to this work. |
|
This journal is © The Royal Society of Chemistry 2022 |
Click here to see how this site uses Cookies. View our privacy policy here.