The fate of plastic in the ocean environment – a minireview
Received
22nd October 2020
, Accepted 13th January 2021
First published on 14th January 2021
Abstract
The presence of plastics in the marine environment poses a threat to ocean life and has received much scientific and public attention in recent years. Plastics were introduced to the market in the 1950s and since then, global production figures and ocean plastic littering have increased exponentially. Of the 359 million tonnes (Mt) produced in 2018, an estimated 14.5 Mt has entered the ocean. In particular smaller plastic particles can be ingested by marine biota causing hazardous effects. Plastic marine debris (PMD) is exposed to physical, chemical and biological stressors. These cause macro and microplastic to break down into smaller fragments, including sub micrometre sized nanoplastic particles, which may account for an important but so far unevaluated fraction of the ocean plastic budget. Physicochemical and biological deterioration of PMD also leads to the release of more volatile compounds and the terminal oxidation of PMD, which most likely accounts for an important but also unevaluated fraction in the ocean plastic budget. This minireview provides an overview on (1) the quantity of plastic production and waste, pathways for plastics to enter the marine realm, the inventory of PMD and the negative effects of PMD to ocean life. (2) We discuss plastic degradation mechanisms in the ocean, expanding on the processes of photodegradation and biodegradation. (3) This review also highlights the emerging topic of nanoplastics in the sea and provides an overview on their specific physical and chemical properties, potential harm to ocean life, and nanoplastic detection techniques.
Environmental significance
Large quantities of plastics are released from the terrestrial to the marine realm, and the impacts of plastics to the marine environment are problematic. The severity of this problem is gaining momentum because the demand for plastic, and coupled to this, the release of plastic debris to the ocean is probably increasing. In this mini review, we provide an overview on plastic production figures, and pathways for plastic waste to enter the ocean. We furthermore provide an overview on the fate of plastic marine debris, which is strongly influenced by fragmentation. While this leads to the generation of micro and nanoplastics, it also increases the surface to volume ratio of the fragments. This likely increases rates of photooxidation and biodegradation, which may constitute important but unevaluated sinks for plastic in the ocean. Finally, we discuss the emerging topic of ocean nanoplastics, which are characterised by different physicochemical properties than micro/macroplastics.
|
Introduction
The vast majority of plastics are of a petrochemical origin and derived from the polymerisation of monomers resulting in synthetic organic polymers.1–3 Their versatile properties have boosted mass production to meet the rapidly developing demand for plastics for a broad variety of applications.4,5 Nowadays, almost all aspects of daily life involve plastics: transport, clothing, construction and packaging materials.3 As a result of waste mismanagement and littering, accumulation of plastic debris in the natural environment is widespread.2,6,7
The most common plastic types [polyethylene (PE), polypropylene (PP)] are characterised by a density lower than water. These represent 36% and 21%, respectively of nonfibrous plastic produced until now.2 Other polymer types are often expended/foamed [polystyrene (PS), polyurethane (PU)], or build to enclose larger volumes (e.g. polyethylene terephthalate (PET) bottles) or used for ropes and nets [nylon (polyamide – PA)]. PS, PU, PET and PA account for ≲10% of the global plastic production until now.2 Plastic litter is transported by wind, surface runoff and rivers from land to the ocean or is directly released via shoreline littering and offshore activities to the sea where it accumulates.3,8,9 As a result, scientific concern and public awareness related to the contamination of the natural environment with plastic waste are growing.
In the last decades, investigations have focused on microplastics (particle size: 1 μm to 5 mm) and, more recently, nanoplastics (particle size: <1 μm) and their potential impact on the ocean environment.4,10–13 Micro and nanoplastics can originate from primary industrial sources or from the degradation of macroplastics (particle size: >5 mm).1,4 The latter is facilitated by different types of physical, chemical and biological processes, which can lead to the fragmentation and degradation of plastics.14 Because of their small size, microplastics and nanoplastics become bioavailable and can bioaccumulate, while current evidence on biomagnification across marine food webs is ambiguous.15–18 Micro and nanoplastic can have negative effects on biota (e.g. by causing inflammation, oxidative stress and disruption of hormone signalling).16,19–21 In addition, plastics often contain additives (e.g. softeners, flame retardants) that may be incorporated and can lead to potentially negative effects for the host organism, while effects for other organism (including humans) higher up in the food chain is not well constrained. Finally, smaller particles have a tendency to sorb hydrophobic compounds, including toxic pollutants.22 If these are in equilibrium with the surrounding seawater, the risk of sorbed chemicals for ocean life is considered relatively low, but toxicity might become relevant under some exposure conditions.23–27
It is unknown how long plastic waste remains in the environment. The durability of plastic entails slow degradation and it was believed that plastics may persists in the environment for an extended period of time, possibly exceeding centuries or even millennia.4 Yet, floating plastics not only fragment in the marine environment but are also degraded through photooxidation, which, in combination with microbial degradation, can severely shorten the lifetime of PMD.14,28–31 Furthermore, several microbes were found that seemingly degrade some plastic polymers directly,32,33 though it is at present debated if and in how far such metabolic traits exist in the marine environment and/or substantially contribute to plastic removal from the ocean environment.34–36
The objectives of this review are to provide an overview on (1) the production of plastics and their use, management and pathways for plastic litter to enter the ocean. (2) We also summarize the current knowledge on plastic degradation, expanding on the processes of photodegradation and biodegradation. (3) This review will furthermore address the emerging topic of nanoplastics in the ocean environment.
Plastic production and plastic waste
Plastic manufacturing is inexpensive, and the global production has increased exponentially ever since mass production began in the 1950s,2,6 reaching ∼359 million tonnes (Mt) in 2018.37 Almost half of all plastics are produced in Asia, while NAFTA and EU countries each account for about a 20%.10 Plastics can be categorized into two groups: fossil-based (petrochemical origin) and bio-based plastics (from renewable organic matter), each of which is classified as non-degradable or bio-degradable.38 Most of the traditional plastics such as PE are fossil-based and classified as non-biodegradable, but they can also be manufactured from renewable base materials yielding a bio-based but still non-biodegradable form of traditional plastic (see discussion on biodegradability of conventional plastics in section ‘Biodegradation’). Traditional plastics can further be separated into those with a carbon–carbon based backbone (e.g. PE, PP, PS) and those with heteroatoms in their main chain (e.g. PET, PU). Biodegradable plastics such as poly lactic acid (PLA) are typically made from renewable base materials, e.g. starch or cellulose, but some biodegradable plastics (e.g. polycaprolactone – PCL) can also be of a petrochemical origin. Biodegradable plastics were developed as an alternative to traditional plastics because they bring new perspectives to waste management options such as composting.39,40 When produced from renewable carbon sources, these materials also have a reduced carbon footprint and do not necessitate fossil hydrocarbon exploitation and other environmentally undesired aspects of petrochemistry.39,40 The global market share of bio-based plastics has been growing but is relatively low with 2% when compared to fossil-based plastics.41
The success of plastics as a base material has been substantial; the inexpensive production and their versatility has made plastics part of almost all sectors of daily life.42 In the last decades, there has been a shift from reusable plastics to single-use plastics, mostly for packaging.2 Single-use plastics are typically consumed and thrown away within the year they are produced; whereas plastics produced for other uses (e.g. construction) can have longer lifespans, sometimes reaching decades.2 Based on data of waste generation reported by the World Bank for 217 countries in 2016,43 the percentage of plastic in municipal solid waste was 10% in 20106 and increased to 12% in 2016.44 Additionally, the total amount of plastic waste was found to roughly proportion global production figures.2,6 Plastic litter is highest at locations of production and urban centres of high population density.6,10
The ∼7800 Mt plastics produced since the 1950s2,37 are distributed between three fractions:2
• Plastics in use (∼30%)
• Post-consumer managed plastic waste: recycled (∼9%) and incinerated plastic (∼12%)
• Discarded plastics in managed systems such as landfills and mismanaged plastic waste (plastics that are littered or inadequately disposed), make up to ∼60% of all the plastics ever produced.2
A substantial part of municipal plastic waste becomes mismanaged waste,2,21,45 when stored in open dumps, which leak plastic litter as a result of runoff and wind. Cities situated at the coats or near rivers consequently become more probable sources of plastic marine litter, and population size and the quality of waste management systems largely determine how much plastic enters the ocean from land.6,10,46 In 2010, 83% of the global mismanaged plastic waste originated from 20 countries.6 Sixteen of these are middle-income countries, characterised by fast economic growth but where waste management infrastructure is insufficient or totally lacking, leaving an average of 68% of waste mismanaged. Countries with a lower economic status and less waste management infrastructures are thus more likely to have a higher percentage of mismanaged waste.6
Sources of plastics in the ocean
Plastic debris became the most commonly detected litter type in the ocean.47 PMD results from the indiscriminate disposal of waste, either directly, i.e. from littering at sea (fishnets, paint or dumping from boats) or indirectly, i.e. waste originating from land (Fig. 1).1,2,6,8,21 Land based, mostly coastal sources account for 80% of PMD, which is transported via natural waterways, sewage/drainage systems, wind or human neglect.48–50 The fishing industry and coastal tourism contribute 18% of PMD, and the remaining 2% are from accidental losses during ocean transport or runoffs from processing facilities.4 The fraction of plastic waste reaching the oceans in 2010 was estimated with 4.8 to 12.7 Mt.6 This is equivalent to 1.8 to 4.7% when considering global plastic resin production37 (1.5 to 4.1% when also accounting for the production of fibrous plastics)2 or 1.7 to 4.6% of plastic waste produced in coastal countries (from where most of the PMD originates).6 Earlier estimates concluded that ∼0.1% of the global production of plastic enters the ocean as PMD.51 With respect to the increase in global plastic resin production reaching 359 Mt in 2018,37 5.5 to 14.5 Mt of plastic waste could thus have been released to the ocean in 2018. According to a recent study by Lau et al. (2020),52 the predicted amount of plastics entering the ocean will increase 2.6-fold until 2040 (assuming that the development of plastic production and plastic waste generation and management continues at the current pace).
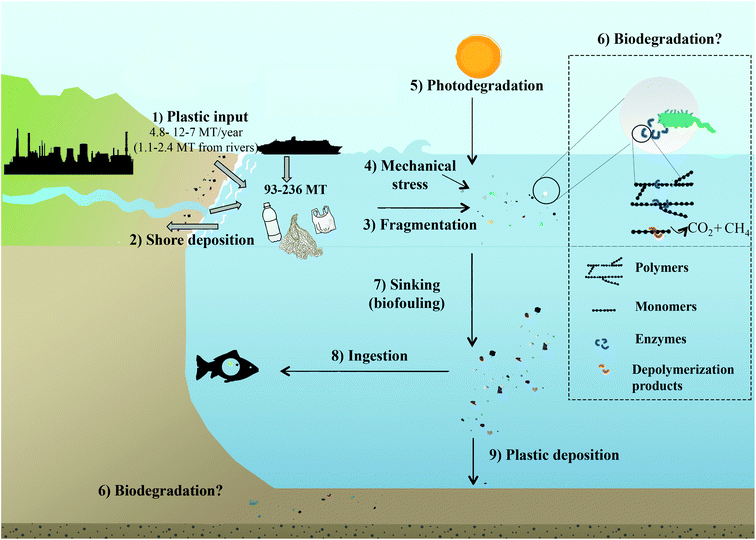 |
| Fig. 1 The fate of plastics in the ocean. (1) Input of plastic to the ocean. (2) Shore deposition. (3) Floating plastics fragment and breakdown into microplastics and nanoplastics through physicochemical factors e.g. (4) mechanical stress, (5) photodegradation and (6) possibly biodegradation. Photooxidation also leads to the production of smaller daughter products, of which at least some can be utilised by microbes. Photooxidation also introduces functional groups into the plastic polymer, which may then become more available for microbial degradation. Biodegradation of intact and partially degraded plastic fragments is facilitated by microbial enzymes breaking down polymers into smaller units, e.g. oligo and monomers, which are then taken up by the organism and used for growth and/or as an energy source, so that the plastics are ultimately degraded to CO2 or CH4. (7) Biofouling lead to a loss of buoyancy of the plastic–biofilm aggregates, which then sink. (8) Plastics are ingested by marine organisms. (9) Plastics sediment and are deposited on the ocean floor. Note that only the plastic input has been parametrised: a total of 4.8 to 12.7 Mt6 (1.15–2.41 Mt from rivers)49 of mismanaged plastics waste has entered the ocean in 2010, 80% from land-based sources, 20% from fishing industry or accidental losses at sea.4 | |
Missing plastic in the ocean
About ∼7800 Mt of plastic have been produced until 2015.2,15 Consequently, ∼117 to 320 Mt of PMD should be present in the sea when assuming that 1.5 to 4.1% of the global plastic production enters the ocean.6 Considering that only ∼50% of the global plastic production is comprised of polymers with a density lower than sea water,2 57–160 Mt of PMD should thus be floating at the sea surface. However, recent inventories (based on datasets of surface trawl observations, ocean models and ocean input estimates) only revealed a total mass of floating PMD of 0.09–0.25 Mt.53,54 Surface trawl observations may be underestimated by a factor of 4–16.55 The amount of floating PMD might hence be in the order of 0.4 to 4 Mt, yet this still stands in stark contrast to the ∼67–160 Mt of positively buoyant PMD released since the 1950s. The missing plastic paradox has been riddling scientists since 2004.56 Furthermore, despite an increase in global plastic production rates, concentrations of floating microplastics in the ocean were not found to increase at a similar rate, further pointing towards a ‘missing’ fraction of microplastic in the oceans.7,10,51,54,57,58
Several explanations were brought forward to explain the missing plastic paradox: (i) the input of plastic into the ocean is overestimated or (ii) the inventory of PMD is underestimated. Perhaps more importantly are mechanisms that (iii) remove PMD from the ocean surface, and (iv) degrade PMD at the ocean surface, the water column or sediments. However, the further fate of plastic in the ocean is not well understood and parameterisation of sinks for PMD is lacking.59,60
(i) Plastic input scenarios were evaluated based on statistics of municipal waste generation,6 but with the exception of river plastic discharge,45,46,50,61 the parameterisation of plastic transfer from land to sea is not well constrained. However, river plastic discharge alone has been estimated with 0.4 to 4 Mt per year and >⅔rd of the global total of riverine plastic in the ocean stems from the top 10–20 polluting rivers.49,50 These figures indicate that plastic input scenarios of 4.8 to 12.7 Mt are reasonable.6
(ii) Global PMD inventories rely on a limited amount of data points of actual PMD concentration measurements, which are then upscaled in model simulations.51,53,54 Measurements and model simulations revealed an uneven distribution of PMD, which complicates assessing global PMD inventories: highest PMD concentrations were found in the subtropical gyres (which have been termed ‘trash vortexes’ or ‘garbage patches’), while PMD concentrations are much lower in the in the tropical and Arctic open ocean.51,53,54,62–64 Furthermore, coastal PMD undergoes cycles of beaching and remobilisation45 adding a temporal dimension to the geographical unevenness.
Also, no standard exists for measuring PMD concentrations, leading to a discrepancy of (micro)plastic counts in the oceans.10 For example, different nets and mesh sizes are used for capturing floating PMD, which complicates comparability. The relatively large mesh size (typically >300 μm) does also not account for small micro plastic particles which are probably more abundant51,65 and it has been suggested that this may explain at least some of the discrepancy.21 Furthermore, net sampling does not account for nanoplastics at all. These have been found in the ocean,66 but virtually nothing is known about ocean nanoplastic abundance and distribution due to limiting methodologies to quantify them.67
(iii) The surface of floating plastics is colonized by organisms that form a biofilm.68–73 This process, biofouling, accelerates aggregation of particles and leads to an increment in density to the point that the particles may sink, transporting microplastics vertically to deeper water layers or the ocean floor.50,67,68,74,75 In some marine regions, relatively high amounts of plastic debris were indeed found in sediments,7,47,57,76 which provides evidence that at least some of the floating plastic is exported from the sea surface and deposited on the ocean floor. It has even been suggested that plastic could be stored in the geological record and may then become a marker horizon for the Anthropocene.77,78 However, PMD sinking fluxes are largely understudied and the deposition mechanisms by which the microplastics reach the sediments is not yet fully understood.79–86 It is also unclear if sunken (but previously floating) PMD remains at the seafloor or if sedimented plastics could become afloat again once the coating biofilm is (partially) degraded.4 Indeed, the findings of an abundance of suspended PMD in the mid water column79,86 begs the question if plastics may not only float or sink but might also oscillate in the water column.74 However, suspended PMD abundances can be highly variable and the vertical resolution for sampling suspended PMD is usually limited, which complicates interpolation between data points. Also, data from high-volume sampling (10 m3) suggest that the typical low-volume samples (<1 m3) might be insufficient to estimate suspended PMD.84 Further data on suspended and sedimented PMD and a better understanding on underlying processes determining vertical PMD fluxes are clearly needed for well-balanced PMD budgets.
PMD is also ingested by several marine organisms, including commercially important species.18,19,87–90 PMD is thus removed from the water column through ingestion and at least temporarily stored in marine organism. Though PMD is thus incorporated in marine food web structures, it is not clear how efficiently it is transferred from prey to predator.91,92 Plastics in marine organisms might be excreted and either become afloat again or, encapsulated in faecal pellets, sinking down to the ocean floor.93 Just as for overgrown plastic particles, it needs to be tested if sinking aggregates of faecal pellets and PMD provides a permanent or temporary sink.
(iv) Plastic degradation includes fragmentation (i.e. breakage into smaller pieces) as well as physicochemical and biological degradation that act on the molecular level (e.g. chain scission of the polymer as well as its oxidation or reduction to CO2 and CH4, respectively). Degradation may also lead to the formation of nanoplastics,67,94–97 which are not accounted for in global plastic estimates due to a lack of detection and/or quantification techniques.66,98–100 The principal mechanisms of key plastic degradation pathways are known (see further details on PMD degradation in the following section), but none of these pathways have been parametrised so far, precluding to better constrain global PMD budgets.
Pathways of plastic degradation in the ocean
Fragmentation
Structural weaknesses and loss of mechanical integrity leads to the breakdown of parent into smaller daughter plastic particles.4,82,94 As such, fragmentation leads to a change in PMD size distribution, and while fragmentation does not remove PMD from the environment, it can accelerate physicochemical and biochemical reactions at the PMD surface because the surface to volume ratio of smaller particles is higher82 (see the following section on ‘Photooxidation’ and ‘Biodegradation’). In the marine environment, plastic fragmentation is induced by mechanical stress, e.g. due to wave action.48,101 Furthermore, fragmentation is accelerated through weathering and potential biological degradation, as these make plastics more brittle.9,10,82,102 A recent study by Gerritse et al. (2020)103 investigated PMD fragmentation in a larger microcosm experiment with continuous water circulation (but not waves). The study found that gravimetric weight loss of PE, PP and PS was ≤1% per year, while PET, PU (3–5% per year) and biodegradable plastics (>7% per year) were characterised by higher rates of weight loss, and that microplastic generation was responsible for (at least some) of the detected weight loss. For PE, the microcosm results were similar to an experiment where PE sheets were exposed to the marine environment.104 Fragmentation rates increase with reduction of particle size.82,105 PMD particles >2 mm tend to have a flake/sheet like morphology so that one face is more likely exposed to sunlight,82 which accelerates fragmentation. The particles then fragment along cracks/fissures at the particle surface, so that the particles become more cubic in shape once the length and width of the daughter fragment approach the thickness of the parent particle, which seems at ∼1 mm. The cubic particles then degrade faster because they tend to roll at the surface. Accelerated fragmentation would also explain the comparably low masses of floating PMD particles <2 mm.51,82 PMD fragmentation also leads to the formation of particle sizes <1 μm, i.e. nanoplastics. This has been documented as a result of shear forces in the digestive tracts of krill and perhaps other crustaceans,96 due to abrasion in a mill106 or blender,94 and even as a result of turbulence95 (see section on ‘Nanoplastics’ for further discussion).
Photooxidation
Photooxidation changes the physical properties of plastics at the molecular level. Visual effects include yellowing of transparent plastics, and the loss of the polymer's mechanical properties make it brittle.14 The presence of additives and stabilizers in the polymer matrix modify the efficiency and rate of photooxidation.42 Photooxidation of plastics comprises free-radical reactions and chain scission initiated by (solar) UV radiation.4,14,48,105 The basic mechanisms and photochemical reactions are well known including differences in the degradation pathway of different polymers (see Gewert et al. (2015)14 for a more comprehensive review). The main reactions involved in the sequence of photooxidation are as follows:14,105,107
(1) Initiation: impurities and structural abnormalities (PE, PP, PS) or unsaturated chromophoric groups (PS, PET) in the polymer structure absorb UV light. This causes breakage of chemical bonds in the polymer chain leading to the formation of free radicals:
Once initiated, the degradation can also proceed thermooxidatively without the need for further UV radiation exposure.
(2) Propagation: the polymer radical reacts with oxygen and forms a peroxy radical:
The peroxy radical can then react with the next substrate leading to the formation of further radicals:
The autocatalytic degradation reaction sequence can progress as long as oxygen is available. Further radical reactions lead to chain branching and ultimately to chain scission.
(3) Termination: propagation reactions are terminated when inert products are formed from the combination of two radicals, which, for example, can lead to cross linking of polymer chains:
or the formation of oxygen-containing functional groups:
As a result of chain scission, crosslinking and oxidation, the polymer's molecular weight and molecular weight distribution is changed and it loses mechanical properties (e.g. extensibility, tensile modulus, or impact strength). This weakens the material, making it more susceptible to fragmentation, which in return increases the available surface area for additional reactions, including further photooxidation and, potentially, biodegradation.14,105 Photooxidation can be complete leading to the terminal oxidation end product CO2 and in conjunction with fragmentation, it can then also lead to the formation of smaller molecules, ranging from hydrocarbon gases to shorter-chain carbonyl compounds (e.g. straight chain or with aromaticity, depending on the parent polymer)14,29,108 as well as nanoplastics.13,67,109 Because of the involved radical reactions, the latter will likely include partially oxidised fragments containing carbonyl groups.
It is commonly believed that photooxidation constitutes an important sink for PMD.14,105 However, literature reports on photooxidation rates are scarce and challenging to compare because of differences in the experimental setups with respect to polymer types/geometry, and UV source characteristics.29–31,110,111 To the best of our knowledge, no study exists that reports on all classes of daughter products that may be generated from photooxidation, i.e. the terminal oxidation end product CO2 as well as gaseous and dissolved volatile and larger chain scission products (e.g. long chain hydrocarbons, carbonyl compounds, oligomers and nanoplastic fragments that can be collectively analysed as dissolved organic carbon – DOC). Ward and colleagues (2019)30 reported on photooxidation-induced CO2 and DOC- and Zhu et al. (2020)31 on DOC production rates. Not accounting for the production of volatile compounds during photooxidation,111 the results from Ward et al. (2019)30 indicate that DOC production accounts for the majority of photooxidation-induced carbon loss from the polymer. For solar radiation conditions as encountered at 30°N, and only considering complete oxidation to CO2, Ward et al. (2019)30 estimated the half-lives of mm-sized microplastic particles of two PS types with 220 (PS without additives) and 380 years (PS with black rubber particles as additives). When additionally considering DOC production,30 half-lives of the two PS types were substantially shorter with 10 and 40 years, respectively. Zhu et al. (2020)31 measured DOC production from plastic particles (mostly PE) collected from the North Pacific Subtropical Gyre and from microplastic sized postconsumer microplastic particles (PE, PP and expanded PS). During the ∼2 month of exposure to UV light as encountered at 30°N, DOC production accounted for 1.6% (gyre PMD), 0.1% (PE) 3.9% (PP) and 6.8% (PS) of the plastic's carbon contents. Assuming first order reaction kinetics31 and only accounting for DOC production rates, PMD particle degradation would follow exponential decay according Nt = N0e−λ/t (where Nt is the fraction remaining after time t, N0 is the initial amount, and λ is the decay constant). Hence, the reported DOC production rates translate to half-lives of ∼8 (gyre PMD), 93 (PE), 2.6 (PP) and 1.5 years (expanded PS).31 Assuming that the residence time of PMD at the ocean surface could be in the order of years, and/or that PMD might oscillate in the water column and periodically re-surfaces, the preliminary results of Ward et al. (2019) and Zhu et al. (2020) show that photooxidation may indeed account for a substantial transformation of PMD into smaller chain scission products and nanoplastics.29–31 The longevity of plastic in the ocean as a function UV radiation thus needs to be constrained further in future research efforts and should be included in budget estimates and model simulations.
Biodegradation
Plastic biodegradation entails the assimilation and mineralization of plastic-derived carbon mediated by (micro) organisms, leading to its eventual removal from the natural environment. As such, plastic biodegradation can proceed as a two-step process where physicochemical processes initially break down the polymer matrix into more labile daughter products that may be degraded further through microbes. Both mechanical forces, and probably more importantly, photooxidation are known to degrade polymers leading to a huge diversity of daughter products such as long and short-chain alkanes and carbonyl compounds, oligomers and nanoplastics.14,29,30,94–96,106,108 Incubation experiments with natural surface water microbial communities from the Mediterranean Sea29 and an estuary of the subtropical NW Atlantic31 showed that microbes were able to utilise at least some of the water-soluble fraction of daughter products generated during photooxidation of PE, PP, and PS. Furthermore, these compounds stimulated microbial growth, yet it seems that PE derived daughter products are less bioavailable and might even inhibit microbial growth.31 At present, the efficiency of microbially-mediated degradation of plastic-derived photooxidation products remains unclear. We consider it likely that different factors such temperature or nutrient levels influence biodegradation rates in marine environments. This needs to be tested for different ocean provinces including the subtropical ocean gyres where PMD accumulates, but also remote habitats such as the deep sea in order to assess the longevity of these compounds in the ocean. It appears logical that some of the known microbes that are, for example, involved in short and long chain hydrocarbon or fatty acid degradation profit from the release of plastic-photooxidation daughter products. However, the identity of the relevant microbes and associated metabolic pathways in the ocean are totally unknown. Also, the chemical identity of bioavailable, recalcitrant and potentially toxic plastic-derived photooxidation products needs to be constrained in future research efforts.
Plastic biodegradation can also be mediated by microbes alone, i.e. microbes mediate initial degradation/depolymerisation (releasing smaller daughter products and potentially nanoplastics) and further degradation/oxidation. There are two principally different pathways how biodegradation of the polymer might proceed: an indirect action, in which metabolic products of microorganisms affect the plastic structure; and a direct action, in which the deterioration of plastics provides a trophic resource for microbial growth.38,112 From a thermodynamic standpoint, plastics are typically rich in chemical energy making polymer oxidation reactions exergonic.113 Plastic degradation is thus, in principal, a worthwhile strategy for microorganisms to obtain energy and/or carbon. Earlier works could show that a diversity of microbes colonise PMD and some evidence exists that these not only comprise opportunistic communities, but that the different polymers might select for a specific, plastic-related community.36,70,73 Other studies found weight loss of plastics exposed to microbial communities in the laboratory or the environment.60
Some bacteria and a few fungi were identified that can make use of plastics as a carbon and energy source (see reviews by Krueger et al. (2015)114 and Jacquin et al. (2019)115). Important groups of bacteria are Arthrobacter, Corynebacterium, Micrococcus, Pseudomonas, Rhodococcus, while fungi belonging to Aspergillus and Penicillium were found. Only some of these groups were isolated from marine systems. The mechanism of degradation, i.e. reactions and enzymes involved in plastic degradation are typically unknown. However, it seems likely that these are similar to enzymes that facilitate degradation of other compounds, which have some chemical similarity to plastics (e.g. crude oil and lignin). For PE-oxidation, oxygenases were suggested;114 these enzymes are known to mediate key steps during (long chain) alkane oxidation. The alkB gene coding for a monooxygenase was identified to play a central role in PE degradation mediated by a Pseudomonas strain.116 This enzyme introduces a hydroxyl group to the carbon chain, which is then further oxidised to fatty acids and metabolised.117 For PS degradation, enzymes similar to those utilised by lignin-oxidising microbes were suggested,114,118 and recent work identified a serine hydrolase as a key enzyme during PS degradation mediated by Pseudomonas.119 However, the exact functioning of this enzyme is not known. In contrast to plastics with a carbon–carbon backbone (such as PE and PS), insights into the biodegradation of polymers with heteroatoms in their backbone (such as PET and PA) seems further. The pathway of PET degradation catalysed by specific cutinases (ester-hydrolysing enzymes) in the bacterium Ideonella sakaiensis is well known120 and has been further optimised for industrial recycling applications.121 Polyurethane-degrading enzymes have already been reviewed two decades ago,122 and PA degrading microbes have been described decades ago, too (though the functioning/enzymes of PA-degradation are still unconstrained).123,124 Heteroatom-containing plastics are most probably easier to degrade, because enzymes can attack the functional groups that are formed by the heteroatoms (see also the study by Min et al. (2020)60 on predictors for PMD surface erosion).
Despite the growing body of evidence that microbial degradation of conventional plastics exists, it is still a matter of debate whether this proceeds in the ocean, and to what extend it provides a sink for PMD.36,70,114,115 This is related to the following:
(i) Many plastic degraders were found in terrestrial rather than marine environments.
(ii) Microbes can metabolise very recalcitrant material in the environment (e.g. lignin and oil) but these have existed in nature since geological time scales, while plastics have been introduced to the environment about 70 years ago. That begs the question if this time period has been long enough for microbes to adapt to these substrates.
(iii) Plastic material was found in the ocean that has apparently stayed intact in the marine environment for decades.125
(iv) Methods to measure degradation in situ can be unreliable. Co-exposure of plastics to biological and physicochemical forcing (e.g. wave action), makes it difficult to distinguish between real degradation and mechanical stress induced fragmentation (see section ‘Fragmentation’), which biases gravimetric methods.
While we cannot rule out that plastic degradation in the marine environment might indeed be low, the opposite can neither be ruled out. (i) Most terrestrial microbes have closely related sister groups in the marine environment, and it appears likely that this is also true for physiological traits. (ii) Plastic degradation in the environment could indeed be the result of (unspecific) co-metabolism. For example, different types of cutinases of non-PET degrading organisms can depolymerise PET, albeit at lower rates than the PET-ase of Ideonella sakaiensis.120 (iii) Intact plastic material was often found in environments that are conducive for organic matter preservation, e.g. cold125 or anoxic habitats126 (and to a lesser degree in warm and oxic environments). Just as for the degradation of smaller compounds generated through photooxidation and/or fragmentation, we consider it very likely that microbial degradation rates of plastic in the ocean are controlled by environmental factors. The van t'Hoff and Arrhenius equations describe the positive effect of elevated temperature on (bio)chemical reaction rates. Though microbes have adapted their physiology to ambient temperature conditions127 (and some potential plastic degraders were found in cold habitats),128 organic matter in cold habitats is generally better preserved than in warmer settings. Similarly, the higher energy yield associated with organic matter degradation with oxygen as well as the ability of aerobic microbes to utilise non-specific hydrolysing/depolymerising enzymes and reactive oxygen species makes complex organic carbon compounds generally more labile in oxic marine environments.127,129–133 We therefore hypothesize that potential plastic degradation proceeds at higher rates in the warm and well-oxygenated surface ocean, in particular at lower latitudes, when compared to deep water and high latitude habitats as well as anoxic (sediment) habitats. The availability of nutrients, the surface to volume ratio of PMD particles, and the molecular formulation of the PMD and additive admixture are likely to influence PMD degradability, too.60,114,134
(iv) Microbial degradation of PMD might indeed be slow but nevertheless important on time scales of decades: assuming that microbial degradation rates follow first order reactions then a low degradation rate of 1% per year would already translates to a particle half life of 70 years. However, just as for photooxidation, it seems very likely that a reduction in particle size leads to an increase in microbial degradation velocity because of the increase in surface to volume ratio when the particle becomes smaller.82 We thus argue that methods need to be (further) developed to facilitate measuring low biodegradation rates quantitatively in a more direct fashion in order to better constrain the role of microbes in PMD degradation. The basis for such methods could be, for example, infrared spectroscopy, which allows measuring plastic oxidation states,82 or isotopically labelled plastics that can be used in isotope probing assays for tracing matter from the polymer into microbial reaction products and biomass.135,136
Nanoplastics
Nanoplastics are defined as plastic particles <1 μm that originate from fragmentation, photooxidation and, possibly, biodegradation (see sections ‘Fragmentation’, ‘Photooxidation’ and ‘Biodegradation’). In additions, primary nanoplastics are probably released to the environment, too.137 Chemically, nanoplastics might thus comprise ‘virgin’ polymers (e.g. pure PE), but may also contain additives just as the parent plastic, or may comprise functional groups (e.g. carbonyl moieties) added to the primary polymer in the process of macro/microplastic degradation.
As macro/microplastic degradation product, nanoplastics might explain some parts of the ‘missing plastic paradox’. However, nanoplastics were for the first time discovered in 2017 in the marine environment,66 because methods for the detection and quantification of environmental nanoplastics were only developed recently.67,98,99,138 For this reason, knowledge on abundance/concentration and distribution of nanoplastics in the marine environment are completely unknown. At the nanoscale, optical and chemical methods conventionally used for microplastic analysis are not suitable because of sensitivity issues as well as physical and optical limits.13,40,67,68,96 Furthermore, many of the currently applied methods for nanoplastic analysis (dynamic light scattering, electron microscopy and pyrolysis GC-MS) require substantial sample pre-concentration and pre-treatment. Only a recent development-using thermal desorption proton transfer mass spectrometry can be used for higher-throughput measurements.98 In addition to challenges for nanoplastic analyses, nanoplastic investigations are further complicated because commonly applied plastic sampling techniques are not suitable for nanoplastic sampling: i.e. plankton nets have mesh sizes of several μm. In order to gain any insights into environmental nanoplastic dynamics, it is thus paramount to utilise and further develop analytical methods that are suitable to characterised nanoplastic at environmental levels and to combine these with appropriate sampling strategies.
Possible fate of nano plastics in the marine environment
The lack of natural analogues for nanoplastics complicates the prediction of their fate, transport, reactivity and toxicity in environmental systems.12,20,23 Nanoplastics can undergo photooxidation,100 which might constitute an important sink for marine nanoplastics, at least in the surface ocean. Other sinks for marine NP could be biodegradation; because of the increased surface to volume ratios,12,139 microbial degradation might be faster (however, this has not been investigated yet). Microbial degradation of molecules released during photooxidation has been shown previously, but the identity of the degraded (or remaining) compounds has not been analysed.29,31 Nanoparticles possess chemical, mechanical and physical properties that differ from larger plastics fragments. Most strikingly, dispersal of nanoplastics is very different when compared to micro/macro plastics composed of the same polymer. Larger macro/micro plastic particles (≥μm scale) are controlled by buoyancy properties, which in turn are determined by density (and shape). As a consequence, macro/microplastics float, sink or, in rare cases, are neutrally buoyant. The dispersion of nanoparticles, on the other hand, is not governed by buoyancy properties, but with decreasing particle size, it is more dominantly controlled by the collision of nanoplastics with water molecules and Brownian motion.140 Nanoplastics consequently exhibit a colloidal behaviour and they may thus be dispersed throughout the ocean water column and sediments. Consequently, any ecological niche in the ocean and the whole spectrum of marine life might be impacted by nanoplastics. On the other hand, nanoplastics may also aggregate with particles of the same type (homoaggregation) or with other (nano)particles (heteroaggregation).100,139,141,142 Heteroaggregation appears to occur more frequently as a result of the ubiquitous presence of other colloids in the water column.141 Most studies on nanoplastic aggregation and stability in aqueous environments were conducted with manufactured/engineered PS nanoparticles,142–145 which complicates extrapolation of these results to other polymer types. Nevertheless, it could be shown that heteroaggregation is determined by nanoplastic surface charge modifications, electrostatic interactions with ions and organic matter in the surrounding aqueous medium and bridging processes. Heteroaggregates are porous, spongy or mesh-like structures where nanoplastic particles can be surrounded by organic and inorganic molecules forming a so-called corona, and bridging effects with ions and organic matter lead to the formation of clusters with nanoplastics and (other) organic and inorganic constituents.143,144 The formation of aggregates reduces the surface to volume ratios of the parent nanoplastics, ‘shields’ the parent nanoplastics from the surrounding water, and the presence of other non-plastic aggregate constituents ad chemical complexity.139 Heteroaggregation thus influences the surface chemistry of nanoplastics (e.g. sorption processes). The larger size of the aggregates will make buoyancy effects more important influencing their dispersal and potential deposition in the marine environment. PS nanoplastics were shown to be more stable in freshwater than marine environments.144 Rivers carry a huge load of plastic pollution,49 which might also include nanoplastics.142 We therefore expect that nanoplastic aggregates tend to form in estuaries so that estuaries and river plumes might be sites of enhanced nanoplastic deposition. Further research needs to be conducted addressing aggregation and deposition characteristics of relevant polymer types across different environmental conditions. Finally, the difference in physicochemical characteristics of nanoplastic aggregates compared to the parent nanoplastic will also affect interactions of nanoplastics and aquatic life.145,146
Impact of nanoplastics on marine life
At present, interactions of nanoplastics with organisms are not well constrained and investigations are limited to laboratory studies with manufactured/engineered nanoplastic particles because of the lack of techniques to measure nanoplastics in the environment. Nanoplastics might simply pass through organisms without further interactions but experimental studies rather suggest that the effects of nanoplastics are more negative when compared to their macro/micro plastic counterparts, at least under the tested exposure conditions,16,147 which might be higher than environmental levels. Furthermore, negative effects of nanoplastics might be dependent on size, and polymer type.16 Nanoplastics are characterised by high surface to volume ratios and thus better adsorb toxic compounds and potentially transfers them to organisms after ingestion.16,18,137,147–149 An experimental study could show that PS-nanoplastics can easily permeate into lipid bilayer membranes, alter the membrane structure and reduce molecular diffusion, so that cell functions are probably perturbed.150 The adsorption of nanoplastic particles by a freshwater algae lowered photosynthesis rates and promoted the formation of reactive oxygen species151 or inhibited growth.152Daphnia magna, a freshwater crustacean, has been exposed to nanoplastics during several experiments.16,153–155 The results of the studies have shown an increased toxicity, physical damage and higher mortality rates of Daphnia as a function of nanoplastic exposure. Similar results were found for a marine rotifer, which accumulated nanoplastics, and oxidative stress-induced damages on its lipid membranes could be observed.147 The rotifer also showed inhibition of drug-resistance proteins, leading to an enhanced toxicity of organic pollutants. In a feeding experiment, polystyrene nanoparticles were ingested by scallops, and the ingested nanoplastics were translocated mostly to the digestive and circulatory system, before reaching the muscles, kidney and gonads.156 These results are in contrast to a similar study, where the scallops were exposed to radiolabelled silver nanoparticles (instead of plastic nanoplastics), which accumulated in the hepatopancreas.157 This could suggest that the chemical composition of the nanomaterials defines their behaviour in biological systems, perhaps due to differences in physicochemical surface properties.16,156 This is important when considering that nanoparticles in the environment are likely coated with larger molecules that build a corona around the particle (see above).139 A corona increases the likelihood of particle aggregation and also changes the particles surface chemistry, which may reduce toxicity.145,146 Furthermore, the size of nanoplastic seems to play an important role on their toxicity and residence time in an organism, too. The fitness of Daphnia magna was impaired by small nanoplastic particles of ∼52 nm but not by larger ones of >120 nm,16 and the depuration of 24 nm particles was faster than that of 250 nm particles.156 The transfer of nanoplastic through food web structures could be shown for an algae–crustacean–fish system16,155 providing evidence for potential negative effects of nanoplastic exposure on all trophic levels. Noteworthy is that at least for fish, nanoplastics can cross the blood brain barrier,16 accumulate in several vital organs,158,159 and disrupt metabolism and hormonal signalling.158
Humans are also exposed to nanoplastics and their added chemicals.160 There are three entryways: via the lungs, contact with the skin and ingestion (e.g. with marine food).18,160 Once taken up, they are translocated to different organs where they might be hazardous for human health.161 Their potential to activate the innate immune system, inducing inflammatory responses, or mediating oxidative stress has been documented.160 Additionally, the activation of ion channels in human lungs after the exposure to PS nanoplastics has also been observed,162 and PE nanoplastic were shown to enter human stem cells in vitro impacting gene expression at concentrations below cytotoxicity.163 Just as for marine organisms, human exposure levels to nanoplastics, tissue accumulation rates and dose-dependencies in relation to particle properties need to be constrained.161
Conclusions
An increasing global demand for plastics leading to increasing plastic production figures is a likely future scenario. In conjunction with a growing population in coastal zones, the risk for an elevated release of plastic debris to the marine environment is thus high. Macro and microplastics are the most commonly found litter types in the ocean and their negative effect to the ocean environment is well documented. Yet, plastics are also degraded in the ocean; most importantly through photooxidation, probably in tandem with microbial degradation, and it is likely that microbes can solely degrade plastics, too. We thus expect that plastic degradation in the ocean is highest in tropical and subtropical regions, i.e. where pollution and accumulation levels of PMD are highest, too. In a hypothetical, future scenario with strongly reduced influx of plastic into the ocean, degradation mechanisms may possibly remove plastic debris from the ocean surface at time scales relevant for human lifespans. Fragmentation and degradation mechanism also lead to the transformation of macro/microplastics into nanoplastics. It consequently seems probable that the generation and influx of nanoplastics into the ocean is coupled to the abundance of ocean macro/microplastic. While the effects of nanoplastics to ocean life seem more negative when compared to microplastics, it might be that nanoplastic degradation is faster because of the higher surface to volume ratio, which likely increases the rate of degradation reactions. Also, nanoplastics are potentially more bioavailable than microplastics, which probably increase their toxicity but may also increase the likelihood for biodegradation. However, nanoplastics are also subjected to aggregation mechanisms, which may reduce the stability of nanoplastics in marine environments. Our knowledge on marine plastic dynamics, in particular for nanoplastics, is very sketchy. In addition to strategies for mitigating ocean plastic littering, future research efforts should aim to determine the fate of plastic in the marine realm with a particular focus on nanoplastic.
Conflicts of interest
There are no conflicts to declare.
Acknowledgements
This study was financed through the European Research Council (ERC-CoG Grant No. 772923, project VORTEX).
Notes and references
- M. Cole, P. Lindeque, C. Halsband and T. S. Galloway, Microplastics as contaminants in the marine environment: a review, Mar. Pollut. Bull., 2011, 62, 2588–2597 CrossRef CAS.
- R. Geyer, J. R. Jambeck and K. L. Law, Production, use, and fate of all plastics ever made, Sci. Adv., 2017, 3 DOI:10.1126/sciadv.1700782.
- R. C. Thompson, S. H. Swan, C. J. Moore and F. S. vom Saal, Our plastic age, Philos. Trans. R. Soc., B, 2009, 364, 1973–1976 CrossRef.
- A. L. Andrady, Microplastics in the marine environment, Mar. Pollut. Bull., 2011, 62, 1596–1605 CrossRef CAS.
-
P. G. Ryan, in Marine anthropogenic litter, ed. M. Bergmann, L. Gutow and M. Klages, Springer, Cham, 2015, pp. 1–25 Search PubMed.
- J. R. Jambeck, R. Geyer, C. Wilcox, T. R. Siegler, M. Perryman, A. Andrady, R. Narayan and K. L. Law, Plastic waste inputs from land into the ocean, Science, 2015, 347, 768–771 CrossRef CAS.
- L. C. Woodall, A. Sanchez-Vidal, M. Canals, G. L. Paterson, R. Coppock, V. Sleight, A. Calafat, A. D. Rogers, B. E. Narayanaswamy and R. C. Thompson, The deep sea is a major sink for microplastic debris, R. Soc. Open Sci., 2014, 1 DOI:10.1098/rsos.140317.
- P. G. Ryan, C. J. Moore, J. A. van Franeker and C. L. Moloney, Monitoring the abundance of plastic debris in the marine environment, Philos. Trans. R. Soc., B, 2009, 364, 1999–2012 CrossRef CAS.
- D. K. A. Barnes, F. Galgani, R. C. Thompson and M. Barlaz, Accumulation and fragmentation of plastic debris in global environments, Philos. Trans. R. Soc., B, 2009, 364, 1985–1998 CrossRef CAS.
- A. L. Andrady, The plastic in microplastics: a review, Mar. Pollut. Bull., 2017, 119, 12–22 CrossRef CAS.
- H. S. Auta, C. U. Emenike and S. H. Fauziah, Distribution and importance of microplastics in the marine environment: a review of the sources, fate, effects, and potential solutions, Environ. Int., 2017, 102, 165–176 CrossRef CAS.
-
K. Mattsson, S. Jocic, I. Doverbratt and L.-A. Hansson, in Microplastic Contamination in Aquatic Environments, ed. Y. Zeng, Elsevier, 2018, pp. 379–399 Search PubMed.
- J. Gigault, A. Ter Halle, M. Baudrimont, P.-Y. Pascal, F. Gauffre, T.-L. Phi, H. El Hadri, B. Grassl and S. Reynaud, Current opinion: what is a nanoplastic?, Environ. Pollut., 2018, 235, 1030–1034 CrossRef CAS.
- B. Gewert, M. M. Plassmann and M. MacLeod, Pathways for degradation of plastic polymers floating in the marine environment, Environ. Sci.: Processes Impacts, 2015, 17, 1513–1521 RSC.
- M. Kooi and A. A. Koelmans, Simplifying microplastic via continuous probability distributions for size, shape, and density, Environ. Sci. Technol. Lett., 2019, 6, 551–557 CrossRef CAS.
- K. Mattsson, E. V. Johnson, A. Malmendal, S. Linse, L.-A. Hansson and T. Cedervall, Brain damage and behavioural disorders in fish induced by plastic nanoparticles delivered through the food chain, Sci. Rep., 2017, 7 DOI:10.1038/s41598-017-10813-0.
- M. E. Miller, M. Hamann and F. J. Kroon, Bioaccumulation and biomagnification of microplastics in marine organisms: a review and meta-analysis of current data, PLoS One, 2020, 15 DOI:10.1371/journal.pone.0240792.
- B. Toussaint, B. Raffael, A. Angers-Loustau, D. Gilliland, V. Kestens, M. Petrillo, I. M. Rio-Echevarria and G. Van den Eede, Review of micro- and nanoplastic contamination in the food chain, Food Addit. Contam., Part A, 2019, 36, 639–673 CrossRef CAS.
- L. G. A. Barboza, C. Lopes, P. Oliveira, F. Bessa, V. Otero, B. Henriques, J. Raimundo, M. Caetano, C. Vale and L. Guilhermino, Microplastics in wild fish from North East Atlantic Ocean and its potential for causing neurotoxic effects, lipid oxidative damage, and human health risks associated with ingestion exposure, Sci. Total Environ., 2020, 717 DOI:10.1016/j.scitotenv.2019.134625.
- T. Kögel, Ø. Bjorøy, B. Toto, A. M. Bienfait and M. Sanden, Micro- and nanoplastic toxicity on aquatic life: determining factors, Sci. Total Environ., 2020, 709 DOI:10.1016/j.scitotenv.2019.136050.
- K. L. Law, Plastics in the Marine Environment, Ann. Rev. Mar. Sci., 2017, 9, 205–229 CrossRef.
- F. Wang, M. Zhang, W. Sha, Y. Wang, H. Hao, Y. Dou and Y. Li, Sorption Behavior and Mechanisms of Organic Contaminants to Nano and Microplastics, Molecules, 2020, 25 DOI:10.3390/molecules25081827.
- E. Besseling, P. Redondo-Hasselerharm, E. M. Foekema and A. A. Koelmans, Quantifying ecological risks of aquatic micro- and nanoplastic, Crit. Rev. Environ. Sci. Technol., 2019, 49, 32–80 CrossRef.
- A. Menéndez-Pedriza and J. Jaumot, Interaction of Environmental Pollutants with Microplastics: A Critical Review of Sorption Factors, Bioaccumulation and Ecotoxicological Effects, Toxics, 2020, 8 DOI:10.3390/toxics8020040.
- N. H. Mohamed Nor and A. A. Koelmans, Transfer of PCBs from microplastics under simulated gut fluid conditions is biphasic and reversible, Environ. Sci. Technol., 2019, 53, 1874–1883 CrossRef CAS.
- J. P. Rodrigues, A. C. Duarte, J. Santos-Echeandía and T. Rocha-Santos, Significance of interactions between microplastics and POPs in the marine environment: a critical overview, TrAC, Trends Anal. Chem., 2019, 111, 252–260 CrossRef CAS.
-
R. Yamashita, K. Tanaka, B. G. Yeo, H. Takada, J. A. van Franeker, M. Dalton and E. Dale, in Hazardous chemicals associated with plastics in the marine environment, ed. H. Takada and H. K. Karapanagioti, Springer, 2019, pp. 163–183 Search PubMed.
- C. P. Ward and C. M. Reddy, Opinion: we need better data about the environmental persistence of plastic goods, Proc. Natl. Acad. Sci. U. S. A., 2020, 117(26), 14618–14621 CrossRef CAS.
- C. Romera-Castillo, M. Pinto, T. M. Langer, X. A. Álvarez-Salgado and G. J. Herndl, Dissolved organic carbon leaching from plastics stimulates microbial activity in the ocean, Nat. Commun., 2018, 9 DOI:10.1038/s41467-018-03798-5.
- C. P. Ward, C. J. Armstrong, A. N. Walsh, J. H. Jackson and C. M. Reddy, Sunlight Converts Polystyrene to Carbon Dioxide and Dissolved Organic Carbon, Environ. Sci. Technol. Lett., 2019, 6, 669–674 CrossRef CAS.
- L. Zhu, S. Zhao, T. B. Bittar, A. Stubbins and D. Li, Photochemical dissolution of buoyant microplastics to dissolved organic carbon: rates and microbial impacts, J. Hazard. Mater., 2020, 383 DOI:10.1016/j.jhazmat.2019.121065.
- T. Matjašič, T. Simčič, N. Medvešček, O. Bajt, T. Dreo and N. Mori, Critical evaluation of biodegradation studies on synthetic plastics through a systematic literature review, Sci. Total Environ., 2020 DOI:10.1016/j.scitotenv.2020.141959.
- J. Ru, Y. Huo and Y. Yang, Microbial degradation and valorization of plastic wastes, Front. Microbiol., 2020, 11 DOI:10.3389/fmicb.2020.00442.
- J. Jacquin, J. Cheng, C. Odobel, C. Pandin, P. Conan, M. Pujo-Pay, V. Barbe, A.-L. Meistertzheim and J.-F. Ghiglione, Microbial ecotoxicology of marine plastic debris: a review on colonization and biodegradation by the “plastisphere”, Front. Microbiol., 2019, 10 DOI:10.3389/fmicb.2019.00865.
- L. Roager and E. C. Sonnenschein, Bacterial candidates for colonization and degradation of marine plastic debris, Environ. Sci. Technol., 2019, 53, 11636–11643 CrossRef CAS.
- S. Oberbeckmann and M. Labrenz, Marine Microbial Assemblages on Microplastics: Diversity, Adaptation, and Role in Degradation, Ann. Rev. Mar. Sci., 2020, 12, 209–232 CrossRef.
-
PlasticsEurope, Plastics—The Facts 2019: An Analysis of European Plastics Production, Demand and Waste Data, PlasticsEurope, 2019 Search PubMed.
- S. K. Ghosh, S. Pal and S. Ray, Study of microbes having potentiality for biodegradation of plastics, Environ. Sci. Pollut. Res., 2013, 20, 4339–4355 CrossRef CAS.
- E. Kabir, R. Kaur, J. Lee, K.-H. Kim and E. E. Kwon, Prospects of biopolymer technology as an alternative option for non-degradable plastics and sustainable management of plastic wastes, J. Cleaner Prod., 2020, 258 DOI:10.1016/j.jclepro.2020.120536.
- S. Thakur, J. Chaudhary, B. Sharma, A. Verma, S. Tamulevicius and V. K. Thakur, Sustainability of bioplastics: opportunities and challenges, Curr. Opin. Green Sustain. Chem., 2018, 13, 68–75 CrossRef.
- R. Chinthapalli, P. Skoczinski, M. Carus, W. Baltus, D. de Guzman, H. Käb, A. Raschka and J. Ravenstijn, Biobased building blocks and polymers—global capacities, production and trends, 2018–2023, Ind. Biotechnol., 2019, 15, 237–241 CrossRef.
- A. L. Andrady and M. A. Neal, Applications and societal benefits of plastics, Philos. Trans. R. Soc., B, 2009, 364, 1977–1984 CrossRef CAS.
-
S. Kaza, L. Yao, P. Bhada-Tata and F. Van Woerden, What a waste 2.0: a global snapshot of solid waste management to 2050, The World Bank, 2018 Search PubMed.
- K. L. Law, N. Starr, T. R. Siegler, J. R. Jambeck, N. J. Mallos and G. H. Leonard, The United States’ contribution of plastic waste to land and ocean, Sci. Adv., 2020, 6 DOI:10.1126/sciadv.abd0288.
- L. Lebreton and A. Andrady, Future scenarios of global plastic waste generation and disposal, Palgrave Commun., 2019, 5 DOI:10.1057/s41599-018-0212-7.
- T. Emmerik and A. Schwarz, Plastic debris in rivers, Wiley Interdiscip. Rev.: Water, 2020, 7(1) DOI:10.1002/wat2.1398.
- G. Erni-Cassola, V. Zadjelovic, M. I. Gibson and J. A. Christie-Oleza, Distribution of plastic polymer types in the marine environment; a meta-analysis, J. Hazard. Mater., 2019, 369, 691–698 CrossRef CAS.
- D. A. Cooper and P. L. Corcoran, Effects of mechanical and chemical processes on the degradation of plastic beach debris on the island of Kauai, Hawaii, Mar. Pollut. Bull., 2010, 60, 650–654 CrossRef CAS.
- L. C. Lebreton, J. Van Der Zwet, J.-W. Damsteeg, B. Slat, A. Andrady and J. Reisser, River plastic emissions to the world’s oceans, Nat. Commun., 2017, 8 DOI:10.1038/ncomms15611.
- C. Schmidt, T. Krauth and S. Wagner, Export of Plastic Debris by Rivers into the Sea, Environ. Sci. Technol., 2017, 51, 12246–12253 CrossRef CAS.
- A. Cozar, F. Echevarria, J. I. Gonzalez-Gordillo, X. Irigoien, B. Ubeda, S. Hernandez-Leon, A. T. Palma, S. Navarro, J. Garcia-de-Lomas, A. Ruiz, M. L. Fernandez-de-Puelles and C. M. Duarte, Plastic debris in the open ocean, Proc. Natl. Acad. Sci. U. S. A., 2014, 111, 10239–10244 CrossRef CAS.
- W. W. Lau, Y. Shiran, R. M. Bailey, E. Cook, M. R. Stuchtey, J. Koskella, C. A. Velis, L. Godfrey, J. Boucher and M. B. Murphy, Evaluating scenarios toward zero plastic pollution, Science, 2020, 369(6510), 1455–1461 CrossRef CAS.
- M. Eriksen, L. C. M. Lebreton, H. S. Carson, M. Thiel, C. J. Moore, J. C. Borerro, F. Galgani, P. G. Ryan and J. Reisser, Plastic Pollution in the World’s Oceans: More than 5 Trillion Plastic Pieces Weighing over 250,000 Tons Afloat at Sea, PLoS One, 2014, 9 DOI:10.1371/journal.pone.0111913.
- E. Van Sebille, C. Wilcox, L. Lebreton, N. Maximenko, B. D. Hardesty, J. A. Van Franeker, M. Eriksen, D. Siegel, F. Galgani and K. L. Law, A global inventory of small floating plastic debris, Environ. Res. Lett., 2015, 10 DOI:10.1088/1748-9326/10/12/124006.
- L. Lebreton, B. Slat, F. Ferrari, B. Sainte-Rose, J. Aitken, R. Marthouse, S. Hajbane, S. Cunsolo, A. Schwarz, A. Levivier, K. Noble, P. Debeljak, H. Maral, R. Schoeneich-Argent, R. Brambini and J. Reisser, Evidence that the Great Pacific Garbage Patch is rapidly accumulating plastic, Sci. Rep., 2018, 8 DOI:10.1038/s41598-018-22939-w.
- R. C. Thompson, Y. Olsen, R. P. Mitchell, A. Davis, S. J. Rowland, A. W. John, D. McGonigle and A. E. Russell, Lost at sea: where is all the plastic?, Science, 2004, 304, 838 CrossRef CAS.
- I. A. Kane, M. A. Clare, E. Miramontes, R. Wogelius, J. J. Rothwell, P. Garreau and F. Pohl, Seafloor microplastic hotspots controlled by deep-sea circulation, Science, 2020, 368, 1140–1145 CrossRef CAS.
- A. E. Schwarz, T. N. Ligthart, E. Boukris and T. van Harmelen, Sources, transport, and accumulation of different types of plastic litter in aquatic environments: a review study, Mar. Pollut. Bull., 2019, 143, 92–100 CrossRef CAS.
- A. Chamas, H. Moon, J. Zheng, Y. Qiu, T. Tabassum, J. H. Jang, M. Abu-Omar, S. L. Scott and S. Suh, Degradation Rates of Plastics in the Environment, ACS Sustainable Chem. Eng., 2020, 8, 3494–3511 CrossRef CAS.
- K. Min, J. D. Cuiffi and R. T. Mathers, Ranking environmental degradation trends of plastic marine debris based on physical properties and molecular structure, Nat. Commun., 2020, 11 DOI:10.1038/s41467-020-14538-z.
- L. C. M. Lebreton, J. van der Zwet, J.-W. Damsteeg, B. Slat, A. Andrady and J. Reisser, River plastic emissions to the world’s oceans, Nat. Commun., 2017, 8 DOI:10.1038/ncomms15611.
-
C. Halsband and D. Herzke, Plastic litter in the European Arctic: what do we know?, Emerging Contaminants, 2019, vol. 5, pp. 308–318 Search PubMed.
- A. L. d. F. Lacerda, L. d. S. Rodrigues, E. Van Sebille, F. L. Rodrigues, L. Ribeiro, E. R. Secchi, F. Kessler and M. C. Proietti, Plastics in sea surface waters around the Antarctic Peninsula, Sci. Rep., 2019, 9 DOI:10.1038/s41598-019-40311-4.
- E. Van Sebille, S. Aliani, K. L. Law, N. Maximenko, J. M. Alsina, A. Bagaev, M. Bergmann, B. Chapron, I. Chubarenko and A. Cózar, The physical oceanography of the transport of floating marine debris, Environ. Res. Lett., 2020, 15 DOI:10.1088/1748-9326/ab6d7d.
- I. Peeken, S. Primpke, B. Beyer, J. Gütermann, C. Katlein, T. Krumpen, M. Bergmann, L. Hehemann and G. Gerdts, Arctic sea ice is an important temporal sink and means of transport for microplastic, Nat. Commun., 2018, 9 DOI:10.1038/s41467-018-03825-5.
- A. Ter Halle, L. Jeanneau, M. Martignac, E. Jardé, B. Pedrono, L. Brach and J. Gigault, Nanoplastic in the North Atlantic Subtropical Gyre, Environ. Sci.
Technol., 2017, 51, 13689–13697 CrossRef CAS.
- J. Gigault, B. Pedrono, B. Maxit and A. Ter Halle, Marine plastic litter: the unanalyzed nano-fraction, Environ. Sci.: Nano, 2016, 3, 346–350 RSC.
- M. R. Gregory, Environmental implications of plastic debris in marine settings—entanglement, ingestion, smothering, hangers-on, hitch-hiking and alien invasions, Philos. Trans. R. Soc., B, 2009, 364, 2013–2025 CrossRef.
- D. Lobelle and M. Cunliffe, Early microbial biofilm formation on marine plastic debris, Mar. Pollut. Bull., 2011, 62, 197–200 CrossRef CAS.
- E. R. Zettler, T. J. Mincer and L. A. Amaral-Zettler, Life in the “Plastisphere”: Microbial Communities on Plastic Marine Debris, Environ. Sci. Technol., 2013, 47, 7137–7146 CrossRef CAS.
- D. Debroas, A. Mone and A. Ter Halle, Plastics in the North Atlantic garbage patch: a boat-microbe for hitchhikers and plastic degraders, Sci. Total Environ., 2017, 599, 1222–1232 CrossRef.
- C. Dussud, C. Hudec, M. George, P. Fabre, P. Higgs, S. Bruzaud, A.-M. Delort, B. Eyheraguibel, A.-L. Meistertzheim and J. Jacquin, Colonization of non-biodegradable and biodegradable plastics by marine microorganisms, Front. Microbiol., 2018, 9 DOI:10.3389/fmicb.2018.01571.
- L. A. Amaral-Zettler, E. R. Zettler and T. J. Mincer, Ecology of the plastisphere, Nat. Rev. Microbiol., 2020, 18(3), 139–151 CrossRef CAS.
- M. Kooi, E. H. van Nes, M. Scheffer and A. A. Koelmans, Ups and Downs in the Ocean: Effects of Biofouling on Vertical Transport of Microplastics, Environ. Sci. Technol., 2017, 51, 7963–7971 CrossRef CAS.
- M. L. Kaandorp, H. A. Dijkstra and E. van Sebille, Closing the Mediterranean Marine Floating Plastic Mass Budget: Inverse Modeling of Sources and Sinks, Environ. Sci. Technol., 2020, 54, 11980–11989 CrossRef CAS.
- I. A. Kane and M. A. Clare, Dispersion, Accumulation, and the Ultimate Fate of Microplastics in Deep-Marine Environments: A Review and Future Directions, Front. Earth Sci., 2019, 7 DOI:10.3389/feart.2019.00080.
- P. L. Corcoran, C. J. Moore and K. Jazvac, An anthropogenic marker horizon in the future rock record, GSA Today, 2014, 24, 4–8 CrossRef.
- J. Zalasiewicz, C. N. Waters, J. A. I. do Sul, P. L. Corcoran, A. D. Barnosky, A. Cearreta, M. Edgeworth, A. Gałuszka, C. Jeandel and R. Leinfelder, The geological cycle of plastics and their use as a stratigraphic indicator of the Anthropocene, Anthropocene, 2016, 13, 4–17 CrossRef.
- C. A. Choy, B. H. Robison, T. O. Gagne, B. Erwin, E. Firl, R. U. Halden, J. A. Hamilton, K. Katija, S. E. Lisin and C. Rolsky, The vertical distribution and biological transport of marine microplastics across the epipelagic and mesopelagic water column, Sci. Rep., 2019, 9 DOI:10.1038/s41598-019-44117-2.
- F. M. C. Fazey and P. G. Ryan, Biofouling on buoyant marine plastics: an experimental study into the effect of size on surface longevity, Environ. Pollut., 2016, 210, 354–360 CrossRef CAS.
- M. Egger, F. Sulu-Gambari and L. Lebreton, First evidence of plastic fallout from the North Pacific Garbage Patch, Sci. Rep., 2020, 10 DOI:10.1038/s41598-020-64465-8.
- A. Ter Halle, L. Ladirat, X. Gendre, D. Goudouneche, C. Pusineri, C. Routaboul, C. Tenailleau, B. Duployer and E. Perez, Understanding the Fragmentation Pattern of Marine Plastic Debris, Environ. Sci. Technol., 2016, 50, 5668–5675 CrossRef CAS.
- K. L. Law, S. Morét-Ferguson, N. A. Maximenko, G. Proskurowski, E. E. Peacock, J. Hafner and C. M. Reddy, Plastic accumulation in the North Atlantic subtropical gyre, Science, 2010, 329, 1185–1188 CrossRef CAS.
- D. Li, K. Liu, C. Li, G. Peng, A. L. Andrady, T. Wu, Z. Zhang, X. Wang, Z. Song and C. Zong, Profiling the Vertical Transport of Microplastics in the West Pacific Ocean and the East Indian Ocean with a Novel in Situ Filtration Technique, Environ. Sci. Technol., 2020, 54, 12979–12988 CrossRef CAS.
- T. H. Nguyen, F. H. Tang and F. Maggi, Sinking of microbial-associated microplastics in natural waters, PLoS One, 2020, 15 DOI:10.1371/journal.pone.0228209.
- K. Pabortsava and R. S. Lampitt, High concentrations of plastic hidden beneath the surface of the Atlantic Ocean, Nat. Commun., 2020, 11(1) DOI:10.1038/s41467-020-17932-9.
- J.-P. W. Desforges, M. Galbraith and P. S. Ross, Ingestion of microplastics by zooplankton in the Northeast Pacific Ocean, Arch. Environ. Contam. Toxicol., 2015, 69, 320–330 CrossRef CAS.
- C. M. Rochman, M. A. Browne, A. J. Underwood, J. A. Van Franeker, R. C. Thompson and L. A. Amaral-Zettler, The ecological impacts of marine debris: unraveling the demonstrated evidence from what is perceived, Ecology, 2016, 97, 302–312 CrossRef.
- I. B. Jâms, F. M. Windsor, T. Poudevigne-Durance, S. J. Ormerod and I. Durance, Estimating the size distribution of plastics ingested by animals, Nat. Commun., 2020, 11(1) DOI:10.1038/s41467-020-15406-6.
- F. Ribeiro, E. D. Okoffo, J. W. O'Brien, S. Fraissinet-Tachet, S. O'Brien, M. Gallen, S. Samanipour, S. Kaserzon, J. F. Mueller, T. Galloway and K. V. Thomas, Quantitative Analysis of Selected Plastics in High-Commercial-Value Australian Seafood by Pyrolysis Gas Chromatography Mass Spectrometry, Environ. Sci. Technol., 2020, 54, 9408–9417 CrossRef CAS.
-
O. Setälä, M. Lehtiniemi, R. Coppock and M. Cole, in Microplastic contamination in aquatic environments, ed. E. Zeng, Elsevier, 2018, pp. 339–363 Search PubMed.
- M. F. M. Santana, F. T. Moreira and A. Turra, Trophic transference of microplastics under a low exposure scenario: insights on the likelihood of particle cascading along marine food-webs, Mar. Pollut. Bull., 2017, 121, 154–159 CrossRef CAS.
- M. Cole, P. K. Lindeque, E. Fileman, J. Clark, C. Lewis, C. Halsband and T. S. Galloway, Microplastics Alter the Properties and Sinking Rates of Zooplankton Faecal Pellets, Environ. Sci. Technol., 2016, 50, 3239–3246 CrossRef CAS.
- M. T. Ekvall, M. Lundqvist, E. Kelpsiene, E. Šileikis, S. B. Gunnarsson and T. Cedervall, Nanoplastics formed during the mechanical breakdown of daily-use polystyrene products, Nanoscale Adv., 2019, 1, 1055–1061 RSC.
- M. Enfrin, J. Lee, Y. Gibert, F. Basheer, L. Kong and L. F. Dumée, Release of hazardous nanoplastic contaminants due to microplastics fragmentation under shear stress forces, J. Hazard. Mater., 2020, 384 DOI:10.1016/j.jhazmat.2019.121393.
- A. L. Dawson, S. Kawaguchi, C. K. King, K. A. Townsend, R. King, W. M. Huston and S. M. Bengtson Nash, Turning microplastics into nanoplastics through digestive fragmentation by Antarctic krill, Nat. Commun., 2018, 9 DOI:10.1038/s41467-018-03465-9.
- M. Piccardo, M. Renzi and A. Terlizzi, Nanoplastics in the oceans: theory, experimental evidence and real world, Mar. Pollut. Bull., 2020, 157 DOI:10.1016/j.marpolbul.2020.111317.
- D. Materić, A. Kasper-Giebl, D. Kau, M. Anten, M. Greilinger, E. Ludewig, E. van Sebille, T. Röckmann and R. Holzinger, Micro- and Nanoplastics in Alpine Snow: A New Method for Chemical Identification and (Semi)Quantification in the Nanogram Range, Environ. Sci. Technol., 2020, 54, 2353–2359 CrossRef.
- C. Schwaferts, R. Niessner, M. Elsner and N. P. Ivleva, Methods for the analysis of submicrometer- and nanoplastic particles in the environment, TrAC, Trends Anal. Chem., 2019, 112, 52–65 CrossRef CAS.
- S. Wagner and T. Reemtsma, Things we know and don't know about nanoplastic in the environment, Nat. Nanotechnol., 2019, 14, 300–301 CrossRef CAS.
- J. E. Weinstein, B. K. Crocker and A. D. Gray, From macroplastic to microplastic: degradation of high-density polyethylene, polypropylene, and polystyrene in a salt marsh habitat: degradation of plastic in a salt marsh habitat, Environ. Toxicol. Chem., 2016, 35, 1632–1640 CrossRef CAS.
- N. Kalogerakis, K. Karkanorachaki, G. C. Kalogerakis, E. I. Triantafyllidi, A. D. Gotsis, P. Partsinevelos and F. Fava, Microplastics Generation: Onset of Fragmentation of Polyethylene Films in Marine Environment Mesocosms, Front. Mar. Sci., 2017, 4 DOI:10.3389/fmars.2017.00084.
- J. Gerritse, H. A. Leslie, C. A. de Tender, L. I. Devriese and A. D. Vethaak, Fragmentation of plastic objects in a laboratory seawater microcosm, Sci. Rep., 2020, 10 DOI:10.1038/s41598-020-67927-1.
- A. L. Andrady, J. E. Pegram and Y. Song, Studies on enhanced degradable plastics. II. Weathering of enhanced photodegradable polyethylenes under marine and freshwater floating exposure, J. Environ. Polym. Degrad., 1993, 1, 117–126 CrossRef CAS.
-
A. L. Andrady, in Marine anthropogenic litter, ed. M. Bergmann, L. Gutow and M. Klages, Springer, Cham, 2015, pp. 57–72 Search PubMed.
- H. El Hadri, J. Gigault, B. Maxit, B. Grassl and S. Reynaud, Nanoplastic from mechanically degraded primary and secondary microplastics for environmental assessments, NanoImpact, 2020, 17 DOI:10.1016/j.impact.2019.100206.
- G. Gryn'ova, J. L. Hodgson and M. L. Coote, Revising the mechanism of polymer autooxidation, Org. Biomol. Chem., 2011, 9, 480–490 RSC.
- B. Gewert, M. Plassmann, O. Sandblom and M. MacLeod, Identification of Chain Scission Products Released to Water by Plastic Exposed to Ultraviolet Light, Environ. Sci. Technol. Lett., 2018, 5, 272–276 CrossRef CAS.
- S. Lambert and M. Wagner, Characterisation of nanoplastics during the degradation of polystyrene, Chemosphere, 2016, 145, 265–268 CrossRef CAS.
- Y. K. Lee, K. R. Murphy and J. Hur, Fluorescence signatures of dissolved organic matter leached from microplastics: polymers and additives, Environ. Sci. Technol., 2020, 54, 11905–11914 CrossRef CAS.
- S.-J. Royer, S. Ferrón, S. T. Wilson and D. M. Karl, Production of methane and ethylene from plastic in the environment, PLoS One, 2018, 13 DOI:10.1371/journal.pone.0200574.
- G. Caruso, Plastic Degrading Microorganisms as a Tool for Bioremediation of Plastic Contamination in Aquatic Environments, Journal of Pollution Effects & Control, 2015, 3 DOI:10.4172/2375-4397.1000e112.
- R. N. Walters, S. M. Hackett and R. E. Lyon, Heats of combustion of high temperature polymers, Fire Mater., 2000, 24, 245–252 CrossRef CAS.
- M. C. Krueger, H. Harms and D. Schlosser, Prospects for microbiological solutions to environmental pollution with plastics, Appl. Microbiol. Biotechnol., 2015, 99, 8857–8874 CrossRef CAS.
- J. Jacquin, J. Cheng, C. Odobel, C. Pandin, P. Conan, M. Pujo-Pay, V. Barbe, A.-L. Meistertzheim and J.-F. Ghiglione, Microbial Ecotoxicology of Marine Plastic Debris: A Review on Colonization and Biodegradation by the “Plastisphere”, Front. Microbiol., 2019, 10 DOI:10.3389/fmicb.2019.00865.
- M. G. Yoon, H. J. Jeon and M. N. Kim, Biodegradation of polyethylene by a soil bacterium and AlkB cloned recombinant cell, J. Biorem. Biodegrad., 2012, 3 DOI:10.4172/2155-6199.1000145.
-
F. Rojo, Enzymes for aerobic degradation of alkanes, Handbook of hydrocarbon and lipid microbiology, 2010, vol. 2, pp. 781–797 Search PubMed.
- F. J. Ruiz-Dueñas and Á. T. Martínez, Microbial degradation
of lignin: how a bulky recalcitrant polymer is efficiently recycled in nature and how we can take advantage of this, Microb. Biotechnol., 2009, 2, 164–177 CrossRef.
- H. R. Kim, H. M. Lee, H. C. Yu, E. Jeon, S. Lee, J. Li and D.-H. Kim, Biodegradation of Polystyrene by Pseudomonas sp. Isolated from the Gut of Superworms (Larvae of Zophobas atratus), Environ. Sci. Technol., 2020, 54, 6987–6996 CrossRef CAS.
- S. Yoshida, K. Hiraga, T. Takehana, I. Taniguchi, H. Yamaji, Y. Maeda, K. Toyohara, K. Miyamoto, Y. Kimura and K. Oda, A bacterium that degrades and assimilates poly(ethylene terephthalate), Science, 2016, 351, 1196–1199 CrossRef CAS.
- V. Tournier, C. M. Topham, A. Gilles, B. David, C. Folgoas, E. Moya-Leclair, E. Kamionka, M.-L. Desrousseaux, H. Texier and S. Gavalda, An engineered PET depolymerase to break down and recycle plastic bottles, Nature, 2020, 580, 216–219 CrossRef CAS.
- T. Nakajima-Kambe, Y. Shigeno-Akutsu, N. Nomura, F. Onuma and T. Nakahara, Microbial degradation of polyurethane, polyester polyurethanes and polyether polyurethanes, Appl. Microbiol. Biotechnol., 1999, 51, 134–140 CrossRef CAS.
- D. Danso, J. Chow and W. R. Streit, Plastics: environmental and biotechnological perspectives on microbial degradation, Appl. Environ. Microbiol., 2019, 85 DOI:10.1128/aem.01095-19.
- T. Deguchi, Y. Kitaoka, M. Kakezawa and T. Nishida, Purification and characterization of a nylon-degrading enzyme, Appl. Environ. Microbiol., 1998, 64, 1366–1371 CrossRef CAS.
- S. Krause, M. Molari, E. V. Gorb, S. N. Gorb, E. Kossel and M. Haeckel, Persistence of plastic debris and its colonization by bacterial communities after two decades on the abyssal seafloor, Sci. Rep., 2020, 10 DOI:10.1038/s41598-020-66361-7.
- A. Nauendorf, S. Krause, N. K. Bigalke, E. V. Gorb, S. N. Gorb, M. Haeckel, M. Wahl and T. Treude, Microbial colonization and degradation of polyethylene and biodegradable plastic bags in temperate fine-grained organic-rich marine sediments, Mar. Pollut. Bull., 2016, 103, 168–178 CrossRef CAS.
- S. Arndt, B. B. Jørgensen, D. E. LaRowe, J. J. Middelburg, R. D. Pancost and P. Regnier, Quantifying the degradation of organic matter in marine sediments: a review and synthesis, Earth-Sci. Rev., 2013, 123, 53–86 CrossRef CAS.
- A. K. Urbanek, W. Rymowicz and A. M. Mirończuk, Degradation of plastics and plastic-degrading bacteria in cold marine habitats, Appl. Microbiol. Biotechnol., 2018, 102, 7669–7678 CrossRef CAS.
- N. E. Blair and R. C. Aller, The fate of terrestrial organic carbon in the marine environment, Ann. Rev. Mar. Sci., 2012, 4, 401–423 CrossRef.
- D. J. Burdige, Preservation of organic matter in marine sediments: controls, mechanisms, and an imbalance in sediment organic carbon budgets?, Chem. Rev., 2007, 107, 467–485 CrossRef CAS.
-
D. Canfield, E. Kristensen and B. Thamdrup, Aquatic geomicrobiology, Elsevier, 2005 Search PubMed.
- C. Laufkötter, J. G. John, C. A. Stock and J. P. Dunne, Temperature and oxygen dependence of the remineralization of organic matter, Global Biogeochem. Cycles, 2017, 31, 1038–1050 CrossRef.
- E. R. Zinser, The microbial contribution to reactive oxygen species dynamics in marine ecosystems, Environ. Microbiol. Rep., 2018, 10, 412–427 CrossRef CAS.
- A. A. Shah, F. Hasan, A. Hameed and S. Ahmed, Biological degradation of plastics: a comprehensive review, Biotechnol. Adv., 2008, 26, 246–265 CrossRef CAS.
- M. Sielicki, D. D. Focht and J. P. Martin, Microbial degradation of [14C] polystyrene and 1,3-diphenylbutane, Can. J. Microbiol., 1978, 24, 798–803 CrossRef CAS.
- M. T. Zumstein, A. Schintlmeister, T. F. Nelson, R. Baumgartner, D. Woebken, M. Wagner, H.-P. E. Kohler, K. McNeill and M. Sander, Biodegradation of synthetic polymers in soils: tracking carbon into CO2 and microbial biomass, Sci. Adv., 2018, 4(7), eaas9024 CrossRef CAS.
-
A. A. Koelmans, E. Besseling and W. J. Shim, in Marine anthropogenic litter, Springer, Cham, 2015, pp. 325–340 Search PubMed.
- S. M. Mintenig, P. S. Bäuerlein, A. A. Koelmans, S. C. Dekker and A. P. van Wezel, Closing the gap between small and smaller: towards a framework to analyse nano- and microplastics in aqueous environmental samples, Environ. Sci.: Nano, 2018, 5, 1640–1649 RSC.
- K. Mattsson, L.-A. Hansson and T. Cedervall, Nano-plastics in the aquatic environment, Environ. Sci.: Processes Impacts, 2015, 17, 1712–1721 RSC.
- P. A. Hassan, S. Rana and G. Verma, Making Sense of Brownian Motion: Colloid Characterization by Dynamic Light Scattering, Langmuir, 2015, 31, 3–12 CrossRef CAS.
- O. S. Alimi, J. Farner Budarz, L. M. Hernandez and N. Tufenkji, Microplastics and Nanoplastics in Aquatic Environments: Aggregation, Deposition, and Enhanced Contaminant Transport, Environ. Sci. Technol., 2018, 52, 1704–1724 CrossRef CAS.
- O. Oriekhova and S. Stoll, Heteroaggregation of nanoplastic particles in the presence of inorganic colloids and natural organic matter, Environ. Sci.: Nano, 2018, 5, 792–799 RSC.
- L. Cai, L. Hu, H. Shi, J. Ye, Y. Zhang and H. Kim, Effects of inorganic ions and natural organic matter on the aggregation of nanoplastics, Chemosphere, 2018, 197, 142–151 CrossRef CAS.
- N. Singh, E. Tiwari, N. Khandelwal and G. K. Darbha, Understanding the stability of nanoplastics in aqueous environments: effect of ionic strength, temperature, dissolved organic matter, clay, and heavy metals, Environ. Sci.: Nano, 2019, 6, 2968–2976 RSC.
- J. Wu, R. Jiang, W. Lin and G. Ouyang, Effect of salinity and humic acid on the aggregation and toxicity of polystyrene nanoplastics with different functional groups and charges, Environ. Pollut., 2019, 245, 836–843 CrossRef CAS.
- J. Saavedra, S. Stoll and V. I. Slaveykova, Influence of nanoplastic surface charge on eco-corona formation, aggregation and toxicity to freshwater zooplankton, Environ. Pollut., 2019, 252, 715–722 CrossRef CAS.
- C.-B. Jeong, H.-M. Kang, Y. H. Lee, M.-S. Kim, J.-S. Lee, J. S. Seo, M. Wang and J.-S. Lee, Nanoplastic Ingestion Enhances Toxicity of Persistent Organic Pollutants (POPs) in the Monogonont Rotifer Brachionus koreanus via Multixenobiotic Resistance (MXR) Disruption, Environ. Sci. Technol., 2018, 52, 11411–11418 CrossRef CAS.
- C.-B. Jeong, E.-J. Won, H.-M. Kang, M.-C. Lee, D.-S. Hwang, U.-K. Hwang, B. Zhou, S. Souissi, S.-J. Lee and J.-S. Lee, Microplastic size-dependent toxicity, oxidative stress induction, and p-JNK and p-p38 activation in the monogonont rotifer (Brachionus koreanus), Environ. Sci. Technol., 2016, 50, 8849–8857 CrossRef CAS.
- Y. Lu, Y. Zhang, Y. Deng, W. Jiang, Y. Zhao, J. Geng, L. Ding and H. Ren, Uptake and accumulation of polystyrene microplastics in zebrafish (Danio rerio) and toxic effects in liver, Environ. Sci. Technol., 2016, 50, 4054–4060 CrossRef CAS.
- G. Rossi, J. Barnoud and L. Monticelli, Polystyrene Nanoparticles Perturb Lipid Membranes, J. Phys. Chem. Lett., 2014, 5, 241–246 CrossRef CAS.
- P. Bhattacharya, S. Lin, J. P. Turner and P. C. Ke, Physical adsorption of charged plastic nanoparticles affects algal photosynthesis, J. Phys. Chem. C, 2010, 114, 16556–16561 CrossRef CAS.
- E. Besseling, B. Wang, M. Lürling and A. A. Koelmans, Nanoplastic affects growth of S. obliquus and reproduction of D. magna, Environ. Sci. Technol., 2014, 48, 12336–12343 CrossRef CAS.
- M. González-Pleiter, M. Tamayo-Belda, G. Pulido-Reyes, G. Amariei, F. Leganés, R. Rosal and F. Fernández-Piñas, Secondary nanoplastics released from a biodegradable microplastic severely impact freshwater environments, Environ. Sci.: Nano, 2019, 6, 1382–1392 RSC.
- Y. Ma, A. Huang, S. Cao, F. Sun, L. Wang, H. Guo and R. Ji, Effects of nanoplastics and microplastics on toxicity, bioaccumulation, and environmental fate of phenanthrene in fresh water, Environ. Pollut., 2016, 219, 166–173 CrossRef CAS.
- K. Mattsson, M. T. Ekvall, L.-A. Hansson, S. Linse, A. Malmendal and T. Cedervall, Altered Behavior, Physiology, and Metabolism in Fish Exposed to Polystyrene Nanoparticles, Environ. Sci. Technol., 2015, 49, 553–561 CrossRef CAS.
- M. Al-Sid-Cheikh, S. J. Rowland, K. Stevenson, C. Rouleau, T. B. Henry and R. C. Thompson, Uptake, whole-body distribution, and depuration of nanoplastics by the scallop Pecten maximus at environmentally realistic concentrations, Environ. Sci. Technol., 2018, 52, 14480–14486 CrossRef CAS.
- M. Al-Sid-Cheikh, C. Rouleau and E. Pelletier, Tissue distribution and kinetics of dissolved and nanoparticulate silver in Iceland scallop (Chlamys islandica), Mar. Environ. Res., 2013, 86, 21–28 CrossRef CAS.
- N. R. Brun, P. van Hage, E. R. Hunting, A.-P. G. Haramis, S. C. Vink, M. G. Vijver, M. J. Schaaf and C. Tudorache, Polystyrene nanoplastics disrupt glucose metabolism and cortisol levels with a possible link to behavioural changes in larval zebrafish, Commun. Biol., 2019, 2 DOI:10.1038/s42003-019-0629-6.
- J. A. Pitt, R. Trevisan, A. Massarsky, J. S. Kozal, E. D. Levin and R. T. Di Giulio, Maternal transfer of nanoplastics to offspring in zebrafish (Danio rerio): a case study with nanopolystyrene, Sci. Total Environ., 2018, 643, 324–334 CrossRef CAS.
- R. Lehner, C. Weder, A. Petri-Fink and B. Rothen-Rutishauser, Emergence of nanoplastic in the environment and possible impact on human health, Environ. Sci. Technol., 2019, 53, 1748–1765 CrossRef CAS.
- M. Prüst, J. Meijer and R. H. S. Westerink, The plastic brain: neurotoxicity of micro- and nanoplastics, Part. Fibre Toxicol., 2020, 17, 24, DOI:10.1186/s12989-020-00358-y.
- J. McCarthy, X. Gong, D. Nahirney, M. Duszyk and M. W. Radomski, Polystyrene nanoparticles activate ion transport in human airway epithelial cells, Int. J. Nanomed., 2011, 6, 1343–1356 CrossRef CAS.
- L. Hoelting, B. Scheinhardt, O. Bondarenko, S. Schildknecht, M. Kapitza, V. Tanavde, B. Tan, Q. Y. Lee, S. Mecking, M. Leist and S. Kadereit, A 3-dimensional human embryonic stem cell (hESC)-derived model to detect developmental neurotoxicity of nanoparticles, Arch. Toxicol., 2013, 87, 721–733 CrossRef CAS.
|
This journal is © The Royal Society of Chemistry 2021 |
Click here to see how this site uses Cookies. View our privacy policy here.