DOI:
10.1039/C9NJ06433H
(Paper)
New J. Chem., 2020,
44, 10102-10110
Chitosan and pectin core–shell beads encapsulating metformin–clay intercalation compounds for controlled delivery†
Received
29th December 2019
, Accepted 3rd March 2020
First published on 4th March 2020
Abstract
This work explores the development of bionanocomposite systems for controlled drug delivery of metformin, the most extensively used oral drug for treatment of type 2 diabetes, also now proposed for treatment of various types of cancer. These systems involve the incorporation of metformin–clay intercalation compounds into a biopolymer matrix, chitosan or pectin, that it is further covered with one or two more biopolymer coatings to ensure stability in the stomach (pectin coating) as well as mucoadhesive properties (chitosan) to attain controlled release of metformin in the intestinal tract. Thus, intercalation compounds involving the ion-exchange of interlayer cations of a natural Wyoming montmorillonite (Cloisite®Na) and a synthetic hectorite (LAPONITE® XLG) were entrapped in chitosan or pectin matrices, producing bionanocomposite beads that were coated with a pectin layer in the first case, or with a chitosan layer and a second external layer of pectin in the second case, to produce core–shell beads that were tested in the controlled release of metformin. The produced beads were submitted to water stability and in vitro release tests simulating the changes of pH along the gastro-intestinal tract with the aim to establish the performance of each type of core–shell bead as a delivery system. It is expected to profit from the stability provided by pectin to reduce the delivery in the stomach, to improve the residence time of the system in the intestinal tract using the mucoadhesive properties of the chitosan, and to use the drug intercalated in the clay as a reservoir from which it can be slowly released in the intestine, and so the combined action of the three components affords a controlled delivery system for the oral administration of metformin.
Introduction
Metformin (MF) is a biguanide-type drug (Fig. 1) for the treatment of type 2 diabetes, being the most used oral medication for this chronic disease.1,2 However, this drug has certain inconveniences due to its low absolute bioavailability (50–60%) and short half-life (6.2 h), which make necessary the administration of large amounts of drug, which can cause gastrointestinal problems.3,4 For this reason, the design of controlled drug delivery systems (CDDS) is of great interest in pharmaceutical dosing, in order to provide an optimal therapeutic level of the drug and maintain it throughout the treatment. To achieve this objective, the design of the carrier is necessary to provide control in the drug release and, if possible, to afford targeted release of the drug in comparison with conventional release systems.5,6 For example, in a conventional oral administration, the drug is released almost immediately in the stomach, often requiring several daily doses, where high concentrations can reach toxic levels and increase the possibility of side effects such as gastrointestinal problems.6
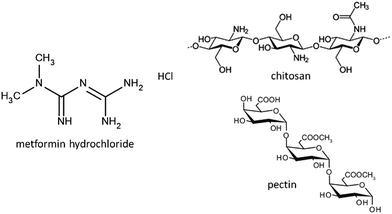 |
| Fig. 1 Structures of metformin hydrochloride, chitosan and pectin. | |
In the last few years, several designs presenting biocompatibility, biodegradability, nontoxicity, etc.5 have been proposed for the release of active substances. Currently, the most commonly used materials are liposomes, nanoparticles, nanoemulsions, natural and synthetic polymers, various inorganic solids (carbon based, metals, silicates, clays, and layered double hydroxides), etc.7–17 Among inorganic carriers, clay minerals have proved to be very efficient to develop controlled release systems, due to properties like adsorption and ion exchange ability.8,10,11,16,18 The systems designed for controlled drug release in oral administration should consider the different environments that the drug will find throughout its passage through the gastrointestinal tract. An important factor is the pH change that occurs along the gastrointestinal tract, which can affect the stability of the DDS. For this reason, clay minerals and their intercalation compounds can be encapsulated in polymer or biopolymer matrixes forming (bio)nanocomposite materials, in order to provide additional control in the drug release.15,19–24 Thus, several approaches to the use of bionanocomposite systems have been recently reported in which biopolymers of different characteristics (carboxymethylcellulose, alginate, chitosan, pectin, zein, cellulose nanofibers,…) are used as matrices in which the drug is further dispersed either, intercalated into the layered solid (smectite clays, layered double hydroxides,…), loaded into halloysite nanotubes or directly adsorbed on sepiolite or modified sepiolite, to procure materials than can be processed as films, tablets or beads for easier and more controlled drug release.21,25–32
In this context, it is here reported the development of new CDDS formulations for the release of metformin based on the encapsulation of clay–metformin hybrids in a core–shell system involving chitosan and pectin biopolymers. The intercalation compounds of metformin in two lamellar clays, a Wyoming-type natural Na-montmorillonite marketed as Cloisite® (Mt) and the commercial synthetic hectorite known as LAPONITE® XLG (Lap), commonly employed in cosmetics and pharmaceutics, were reported in previous work.29,33 Both biopolymers, chitosan and pectin, are widely used in the food industry and in the area of biomedicine as scaffolding, wound dressings or DDS.34–36 Chitosan is a polysaccharide extracted mainly from the exoskeleton of crustaceans, which consists of poly[β-(1-4)-2-amino-2-deoxy-D-glucopyranose] (Fig. 1). It is a cationic polymer at pH below 6 with protonated amino groups in the C2 positions of the glucose rings. Given the known mucoadhesive properties of chitosan,37,38 it is used in DDS in which the drug must have a residence time in a specific mucosa, such as buccal or intestinal. In order to overcome the disadvantage of the low stability of chitosan at acid pH, many modifications or complex formation with other polymers have been proposed to improve the stability of chitosan in the stomach, its mucoadhesion and other properties.39,40 Unlike chitosan, pectin is an anionic polysaccharide extracted from citrus fruits, consisting of residues of (1,4)-α-D-galacturonic acid41 (Fig. 1). It is resistant to low pH conditions and digestion by the proteases and amylases present in the stomach, but at the same time it is sensitive to the pectinases present in the colon, facilitating its complete digestion in the intestinal tract.42 In this way, we have previously reported the use of chitosan–pectin combinations to afford the targeted release in the colon tract of 5-aminosalicylic (5ASA), a drug prescribed for treatment of ulcerative colitis and Crohn's disease.19 Hence, the combination of chitosan and pectin in a core–shell system of clay–MF hybrids appears as a great opportunity to simultaneously benefit from the properties of both biopolymers, and increase the residence time of the drug in the intestine, maintaining a controlled release rate.
Results and discussion
Bionanocomposite systems based on clay–metformin hybrids
The preliminary results on the intercalation of MF in charged layered silicates, such as Na-montmorillonite and LAPONITE® XLG clay minerals, have been reported in previous works.29,33 Computational modeling studies were conducted to understand the behavior of these silicates in the process of intercalation and release of MF hosted in between the silicate layers. As these MF-clay hybrid systems were not satisfactory formulations for application as controlled release systems in oral administration of metformin,29,33 the aim of the current work was the development of new formulations by encapsulation of these clay–MF hybrids within suitable biopolymer matrixes (pectin and chitosan) in different core–shell configurations, in order to improve their delivery properties. For comparison, three hybrids have been selected in the current study: (i) Mt–MF, which corresponds to an intercalation compound prepared in the presence of a large excess of MF in solution to assure the system is in the plateau of the adsorption isotherm, where it contains around three times the CEC of the used Mt (243.6 mEq./100 g),33 (ii) Mt–MFw, the same intercalation compound but recovered after thoroughly washing with water, in order to remove the excess MF adsorbed on the external surface of the clay, to get an amount of MF equivalent to the cation exchange capacity (CEC) of the pristine Mt (93 mEq./100 g),33 and (iii) Lap–MF, which incorporates an amount of MF equivalent to the CEC of LAPONITE® XLG (63 mEq./100 g). The XRD patterns of the Mt–MF and Mt–MFw hybrids (Fig. 2) confirm the intercalation of MF from the X-ray diffraction patterns as they showed the displacement of the (001) reflection to lower 2 theta angles. Thus, the basal spacing changes from 1.20 nm in the pristine Mt to 1.36 nm in the corresponding intercalation compounds, resulting in a basal spacing increase of 0.40 nm. In the Lap clay, the (001) reflection is observed at a distance of 1.44 nm due to the greater content of water in this clay,29 whereas in the Lap–MF hybrid this reflection peak appears at 1.32 nm, from which it is possible to calculate a basal spacing increase of 0.36 nm. In all the diffraction patterns of these hybrid materials, the appearance of the (002) reflection peak at 0.65 and 0.63 nm for the Mt and the Lap based hybrids confirms the formation of clay–MF intercalation compounds.29 Molecular modeling quantum mechanical calculations performed using the Castep code43 based on plane wave DFT (Density Functional Theory) confirmed that the intercalation of MF cations in both types of clay follows an ion-exchange mechanism, stabilizing a monolayer of MF ions in the interlayer region, where the drug molecule is disposed more parallel to the mineral surface in Lap than in Mt (Fig. 2).29
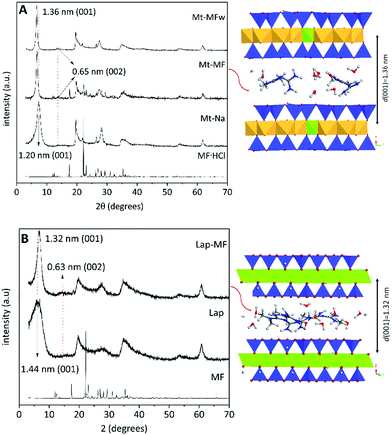 |
| Fig. 2 XRD patterns of pure MF·HCl, pristine Mt and Lap, and their intercalation compounds. On the right there is also shown a model of the intercalation compound determined by applying the Castep code within the Materials Studio package.29,44 | |
Two configurations based on the encapsulation of the selected clay–MF hybrids in chitosan or pectin were prepared and evaluated in the present work. One of them is based on the incorporation of the clay–MF hybrids in chitosan, forming beads that were later coated with a pectin layer in a core–shell configuration similar to that previously explored in LDH-5ASA bionanocomposite bead systems.19 The pectin@chitosan/clay–MF bionanocomposite systems were prepared by dispersing the Mt–MFw intercalation compound in the chitosan matrix, and the beads were coated with pectin from 0.5, 1 and 1.5% (w/v) aqueous solutions of the biopolymer. Additionally, core–shell beads incorporating the Mt–MF hybrid were coated with the 0.5% (w/v) pectin dispersion. For comparison, analogous core–shell beads were prepared incorporating pure MF·HCl instead of the clay–MF hybrids. The second configuration is based on the encapsulation of the clay–MF hybrids in pectin beads that were later coated with a first layer of chitosan and a second layer of pectin. The pectin@chitosan@pectin/clay–MF beads were developed by encapsulation of the Mt–MFw and Lap–MF hybrids in the pectin core, and coated with a chitosan layer and an external coating of pectin, as detailed in the Experimental section, using 0.5% w/w solutions of each biopolymer. In both configurations pectin was selected for the external coating, as it is very resistant to acidic pH, while chitosan was chosen due to its mucoadhesive properties, which can be beneficial to increase the permanence of the DDS in the intestinal tract.
Table 1 shows the amount of MF loaded in all the prepared formulations as well as the encapsulation efficiency for each system. The systems incorporating the pure drug in chitosan (CHT/MF) have lower MF loading and encapsulation efficiency compared to those loaded with the Mt–MF hybrids and to the systems based on beads with a pectin core, showing encapsulation efficiency values of 25.0, 29.5 and 31.8%, for the systems prepared with starting amounts of 0.1, 0.3 and 0.5 g of metformin, respectively. In contrast, an almost 70% encapsulation efficiency was reached in the pectin formulations (PEC/MF). This result may be related to the fact that MF·HCl in solution is dissociated, giving the protonated MF species, which may show electrostatic repulsion with the protonated chitosan chains, making it difficult to increase the loading of drug into the chitosan matrix. In fact, the increase of the starting amount of MF added to chitosan does not result in an increase of the drug loading. Therefore, 0.1 g of MF was used as a reference to prepare the other bionanocomposite systems. In the case of incorporating MF stabilized in the Mt–MFw hybrid, it is possible to load a larger amount of drug with encapsulation efficiencies close to 60% independently of whether the bead contains just chitosan or it was later coated with different amounts of pectin. The system prepared using the non-washed hybrid (Mt–MF), with an MF content about three times higher than that of the Mt–MFw hybrid, showed a very close encapsulation efficiency (60.9%), which suggests that the use of clay-based hybrids with a large excess of drug adsorbed on the external surface of the clay is not necessary.
Table 1 Encapsulation efficiency and amount of loaded MF in different formulations
Formulation |
MF loading (%) |
Encapsulation efficiency (%) |
CHT/MF0.1 |
0.6 ± 0.2 |
25.0 ± 0.8 |
CHT/MF0.3 |
2.2 ± 0.5 |
29.5 ± 1.0 |
CHT/MF0.5 |
2.7 ± 0.3 |
31.7 ± 0.6 |
CHT/Mt–MFw |
5.8 ± 0.2 |
58.2 ± 0.8 |
PEC0.5%@CHT/Mt–MFw |
5.7 ± 0.1 |
56.8 ± 1.0 |
PEC0.5%@CHT/Mt–MF |
6.1 ± 0.3 |
60.9 ± 1.0 |
PEC1%@CHT/Mt–MFw |
5.7 ± 0.2 |
57.3 ± 0.9 |
PEC1.5%@CHT/Mt–MFw |
5.7 ± 0.2 |
57.5 ± 1.2 |
PEC/MF |
6.9 ± 0.4 |
69.3 ± 1.1 |
PEC/Mt–MFw |
8.7 ± 0.3 |
87.3 ± 0.9 |
PEC@CHT@PEC/Mt–MFw |
8.6 ± 0.2 |
86.2 ± 0.8 |
PEC/Lap–MF |
9.1 ± 0.9 |
91.1 ± 1.1 |
PEC@CHT@PEC/Lap–MF |
9.0 ± 1.2 |
90.0 ± 1.2 |
All the prepared pectin-core based bead materials show higher encapsulation efficiency than the systems prepared with chitosan. The PEC/Mt–MFw and PEC/Lap–MF beads exhibit very good encapsulation efficiency, reaching values of 87 and 91%, respectively, clearly higher than those formulations encapsulating directly MF·HCl (69.3%). As observed in the chitosan-core based systems, this result may be associated with the protective effect exerted by the clay minerals, where the drug is protected within the sheets of the layered silicates. Here again, the encapsulation efficiency of the pectin/clay–MF beads and that of the core–shell systems is practically the same, confirming that there is no loss of drug during the coating of the beads to produce the shell. Comparing the encapsulation efficiencies obtained for all the formulations, the best results were obtained when pectin was used in the core of the beads. The reduced values obtained in the chitosan systems can be attributed to a competing effect between the intercalated MF species and the protonated chitosan chains, which would be avoided using the negatively charged pectin. Thus, the pectin-based systems seem more appropriate for MF delivery purposes.
FE-SEM images of the cross-section of the PCT@CHT/Mt–MFw and PEC@CHT@PEC/Mt–MFw bionanocomposite beads are shown in Fig. 3. The pectin coating covering the CHT/Mt–MF bionanocomposite core can be observed in Fig. 3A. The external surface of the bead is homogeneous and smooth (Fig. S1A and B, ESI†), while the cross-section presents a quite homogeneous and porous pectin layer over a more compact and bulky material in the bionanocomposite core, without segregation of phases, confirming the good dispersion of the hybrid in the polymer matrix (Fig. S2, ESI†). The two biopolymer phases seem to have a good interaction, confirming the adherence between the two biopolymers as already reported in other works.19,45Fig. 3B shows the cross-section of the pectin bead encapsulating the Mt–MFw hybrid with the double coating of chitosan and pectin again at the external surface. In this FE-SEM image it is possible to distinguish the two layers of the coating, with chitosan sandwiched between the external pectin coating and the internal pectin bionanocomposite core. The three phases are highly homogenous and show porosity created during the lyophilization process used to dry the final beads. The surface morphology of the beads encapsulating MF·HCl and the Mt–MFw hybrid in pectin, without an external coating, observed by FE-SEM (Fig. S1C and D, ESI†) seems also quite smooth and does not present polymer agglomerates. The FE-SEM image of the PEC/Mt–MFw beads (Fig. S1D, ESI†) shows that the Mt–MFw hybrid is homogeneously dispersed in the pectin matrix, and the external surface of the bead practically does not show any roughness or cracking, confirming excellent integration of the hybrid within the biopolymer matrix.
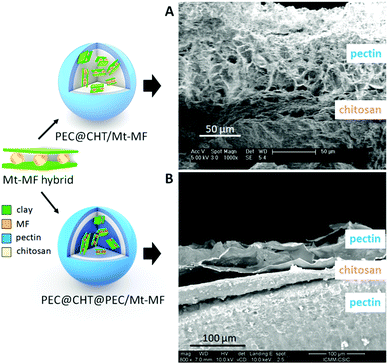 |
| Fig. 3 Schematic models of the MF-clay hybrid and the chitosan (CHT)/pectin (PEC) bionanocomposite beads (on the left) and FE-SEM images (on the right) of cross-sections of the PEC@CHT@PEC/Mt–MFw (A) and PEC@CHT@PEC/Mt–MFw (B) bionanocomposite beads. | |
Water absorption properties of the beads
For controlled drug release applications, it is important to study the water absorption capacity and swelling properties of the beads. Thus, these parameters were evaluated for the different systems in HCl solution (pH 1.2) and phosphate buffer (pH 6.8). Fig. 4 shows the evolution in the content of water absorbed by the beads as a function of the time in contact with the aqueous media. It is observed that the water absorption of the different formulations strongly depends on the pH of the medium. This behavior is attributed to the protonation of the amino groups in chitosan at acid pH, provoking the gradual dissolution of the biopolymer until complete disintegration of the beads.19 When the chitosan beads are covered with a pectin layer, the stability in the presence of a large amount of H+ increases due to the insolubility of pectin at this pH, and all the beads show lower absorption of water and do not disintegrate during the time set for the experiment (Fig. 4A). In the pectin@chitosan core–shell beads, the water uptake is reduced in those systems incorporating the clay–MF hybrid in the core instead of MF·HCl, probably due to a physical crosslinking effect between the hybrid component and the chitosan matrix, showing no relevant differences between PEC@CHT/Mt–MF beads with different content of pectin in the external coating at pH 1.2.
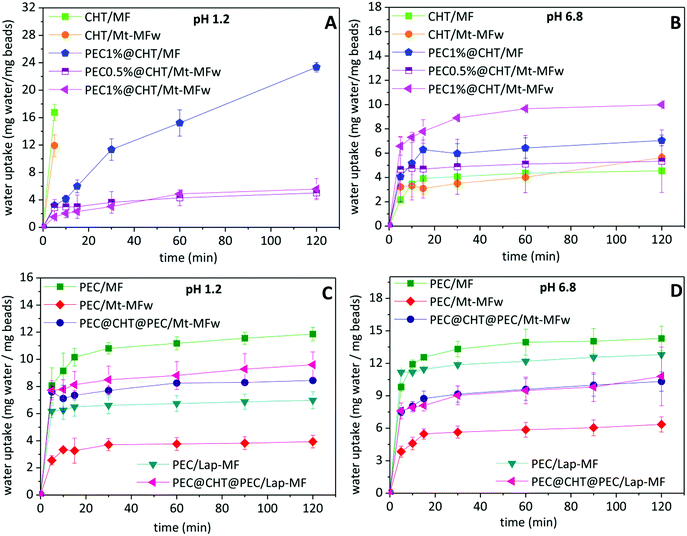 |
| Fig. 4 Water uptake of pectin@chitosan (A and B) and pectin@chitosan@pectin beads (C and D) immersed in water at pH 1.2 (0.06 M HCl) and pH 6.8 (phosphate buffer), graphics on the left and the right, respectively. Each value is the mean ± S.D. n = 3. | |
In contrast, the CHT/MF and CHT/Mt–MF beads exhibit the greatest stability, with very similar water uptake profiles, when immersed in phosphate buffer at pH 6.8 (Fig. 4B), which is due to the insolubility of chitosan at this pH. Under these conditions, the pectin-coated chitosan beads show higher water uptake as the amount of pectin in the external coating increases, which can be attributed to the solubility of pectin at this pH and to the presence of phosphate ions in the medium, which capture the calcium ions acting as bridges between the pectin chains, facilitating the increase of water uptake.46 On the other hand, the pectin beads prepared with MF·HCl show the maximum uptake at pH 1.2 (Fig. 4C), reaching a constant value around 10% after 5 min, although in the present case the beads do not completely disintegrate. This result was expected because of the insolubility of pectin at acidic pH as mentioned above. The lower uptake of water at pH 6.8 (Fig. 4D) corresponds to pectin beads incorporating the clay–MF hybrids, being lower in the bionanocomposite based on montmorillonite. As mentioned above, this effect may be associated with the physical crosslinking established between the Mt–MF hybrid and the biopolymer chains, resulting in a greater resistance to swelling of these beads. However, the water uptake results indicate that the interaction between the Lap–MF hybrid and pectin is less strong and cannot counteract the effect of pH and the phosphate ions. The beads containing the pectin–chitosan core–shell structure show similar water uptake, with lower values than those of PEC/MF or PEC/Lap–MF. In view of the oral administration of these formulations, the water uptake results confirmed that the coating of pectin on the beads is crucial to protect chitosan from the low pH conditions of the stomach. Given that those systems based on a pectin core show higher MF loading (Table 1), the ternary core–shell beads seem to be a very promising system for the release of metformin.
In vitro tests of metformin release
The in vitro release tests of metformin from the prepared formulations were carried out simulating the gastrointestinal tract, as detailed in the Experimental section. Fig. 5A shows that the drug was completely released from the CHT/MF beads in the first two hours at acid pH simulating the gastric conditions. This result was already expected because chitosan is not resistant to acid pH, as shown in the previous water uptake study. The core–shell beads in which the chitosan bead was further coated with pectin showed a higher resistance at low pH, obtaining more controlled release over the 8 h of the experiment simulating the gastrointestinal tract. When comparing core–shell systems incorporating clay–MF hybrids in the chitosan core, it is observed that the MF release depends on the core composition and also on the thickness of the pectin coating. Thus, the system incorporating the non-washed hybrid (PEC0.5@CHT/Mt–MF300) presents a fast initial release at pH 1.2 of about 40% MF, which may be associated with the presence of drug dispersed within the biopolymer matrix coming from MF adsorbed at the external surface of the clay in the Mt–MF hybrid during the bead preparation. Although this PEC0.5%@CHT/Mt–MF system exhibits an initial release greater than the washed hybrid, the release is then gradual in comparison with the Mt–MF hybrid alone, but it only reaches a total release of 80% after 8 h. In contrast, the PEC0.5@CHT/Mt–MFw system shows more controlled release with about 20% MF liberated at the pH of the stomach, and progressive and continuous release at pH conditions simulating the intestinal tract, reaching complete MF release upon the 8 h of the experiment. When the beads present a thicker coating of pectin, i.e., those prepared using pectin solutions of concentration 1 and 1.5% (PEC1@CHT/Mt–MFw and PEC1.5@CHT/Mt–MFw beads, respectively), it is possible to reduce further the release of metformin at the pH conditions of the stomach. However, the release in the conditions of the intestine also slows down, reaching in both cases up to 60% liberated MF after 8 h. These results confirm the efficiency of the pectin coating on the chitosan beads and suggest that the core–shell beads could be used for the controlled release of metformin. However, these systems have the disadvantage of a low encapsulation efficiency (around 60%) compared to those based on a pectin core (around 90%), as explained in the previous section. For this reason, the MF release from pectin@chitosan@pectin/clay–MF systems was also investigated.
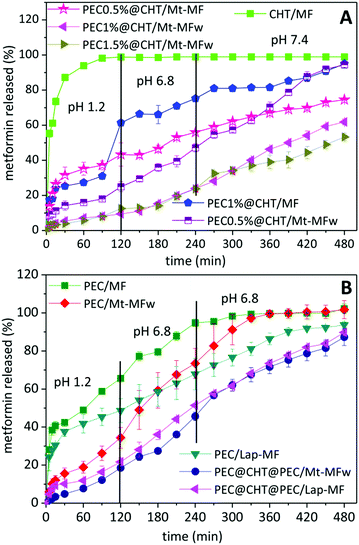 |
| Fig. 5 Profiles of the in vitro release of metformin from various formulations based on chitosan with and without a pectin coating (A) or a pectin core protected or not with a double coating of chitosan and pectin (B). The experiment simulates the pH conditions that occur in the passage through the gastrointestinal tract (pH and residence time) and was carried out at 37 °C. Each value is the mean ± S.D. n = 3. | |
Fig. 5B shows the release profiles from the beads prepared from pectin incorporating MF·HCl, exhibiting an initial release of about 40% at pH 1.2 and releasing 100% MF at pH 6.8 in the conditions that simulate the first zone of the gastrointestinal tract. Thus, the PEC/MF system is much more satisfactory than the CHT/MF system, due to the higher stability of pectin at this pH as mentioned above. For the systems incorporating the clay–MF hybrids, i.e., PEC/Mt–MFw and Lap–MFw, the MF release at pH 1.2 is considerably reduced, especially for that based on the montmorillonite hybrid, due to the protection afforded by the inorganic host in the intercalation compounds, although different release rates are observed in this medium depending on the clay nature. At pH 6.8 phosphate anions capture the calcium ions that crosslink the biopolymer chains, and the drug is released at a quite constant rate, reaching 100% MF released in approximately 6 hours. In the case of the double-coated core–shell beads, PEC@CHT@PEC/Mt–MF and PEC@CHT@PEC/Lap–MF, both systems show a quite similar behavior with initial slow release kinetics in the acidic medium, which corroborates the protective effect exerted by the external pectin coating. In these systems, a significant slow release rate is kept at higher pH values, 6.8 and 7.4, providing controlled release of MF along the whole gastrointestinal tract.
Conclusions
The present work reports the development of biopolymer-based drug delivery systems for the controlled release of metformin, the drug most commonly used for the treatment of type 2 diabetes. The DDS were produced by encapsulation in the biopolymers chitosan and pectin of clay–metformin hybrids reported in previous studies, based on the intercalation of MF in two lamellar clay minerals, a synthetic hectorite (LAPONITE® XLG) and a natural Wyoming montmorillonite, by an ion-exchange mechanism. The prepared DDS were designed to combine the advantages of the three components, pectin, chitosan, and clays: (i) the outer pectin coating protects the DDS in acidic media, in the first zone of the gastrointestinal tract (stomach); (ii) the chitosan matrix, used as an encapsulating matrix or as a protective coating on pectin beads, provides the DDS with mucoadhesive properties of interest for specific adsorption in the intestinal tract; and (iii) the incorporation of the drug intercalated into the layered clay results in a reservoir that offers the possibility of controlling the kinetics of drug release when the biopolymer matrix is degrading. The arrangement of these components and the final structure of the beads can influence the release process and its kinetics mainly by two effects, the swelling and diffusion processes.
The first core–shell system studied in the current work consists of the encapsulation of the clay–MF intercalation compound in a chitosan matrix, further coated with a pectin layer. A second configuration consists of pectin beads incorporating the clay–MF hybrid, which were coated first with a chitosan layer and then with an external pectin coating. This last system has proven to be more efficient as it is able to encapsulate around 90% of metformin in contrast to the 60% reached in the chitosan-based formulations, which can be attributed to the competing effect of the protonated chitosan chains with the intercalated MF.
In vitro tests of MF release in fluids simulating the changes in pH and residence time that occur during the in vivo passage of the drug through the gastrointestinal tract demonstrated higher efficacy of both types of core–shell bionanocomposite systems compared to those prepared without protective coatings or incorporating directly the drug without the clay host. Another important factor is that the release kinetics can be controlled by adjusting the concentration of the pectin solution, which can lead to coatings of different thickness. Thus, several proposed formulations are able to provide very slow release at low pH, allowing controlled delivery of most of the metformin in medium simulating the intestinal tract. These results may be of special relevance to prepare appropriate formulations for the controlled oral administration of metformin, allowing the administration of lower amounts than those usually indicated to obtain an optimal therapeutic effect. With the proposed bionanocomposite CDDS, the residence time of MF in the intestinal region could be increased and, consequently, its efficiency may be higher, potentially avoiding the common side effects caused by MF. In addition, the possibility of including more specific functionalities in chitosan and/or pectin could be exploited to make the release location even more specific depending on the disease to be treated with metformin. The advantages of these bionanocomposites for application as CDDS are their availability, biocompatibility, biodegradability and low cost, which make them a very promising approach for the treatment of type 2 diabetes and other diseases.
Experimental
Starting materials and reagents
Metformin (MF) was purchased from Sigma-Aldrich as 1,1-dimethylbiguanide hydrochloride (C4H12ClN5), with a MW of 165.62 g mol−1 and purity 97%. LAPONITE® XLG (Lap) was kindly provided by BYK Additives & Instruments, and Cloisite®Na by Southern Clay Products. Deionized water (a resistivity of 18.2 MΩ cm) was obtained using Elga Maxima Ultrapure Water equipment. Chitosan (CHT) of medium molecular weight (250 kDa) and 75–85% deacetylation and pectin (PEC) from citrus fruit were purchased from Sigma-Aldrich. Aqueous solutions were prepared from chemicals of analytical reagent grade furnished by Sigma-Aldrich: HCl (37%, P.A.), acetic acid (glacial), NaOH (98%), NaCl (>99%), NaH2PO4·H2O (>99%) and CaCl2·2H2O (>99%).
Methods
Intercalation of metformin in the layered silicates
The intercalation of metformin (MF) in montmorillonite (Mt) and LAPONITE® XLG (Lap) was carried out as reported in previous studies.29,33 Briefly, 300 mg of metformin was added to 20 ml of a Mt (0.15 g) dispersion for the preparation of Mt–MF hybrids, while 62.58 mg of metformin was added to a suspension of 0.2 g of Lap in 20 ml of water to obtain the Lap–MF hybrids. After homogenization of each mixture under magnetic stirring for 48 h, the solids were recovered by centrifugation, and then dried at 60 °C directly (Mt–MF and Lap–MF) or after washing to remove any excess MF weakly adsorbed on the clay surface.
Preparation of the core–shell bionanocomposite beads
Preparation of pectin@chitosan/clay–MF beads.
Chitosan–pectin bionanocomposite beads encapsulating the clay–MF hybrids were prepared following the procedure described by Ribeiro et al.19 and schematized in Fig. S3 (ESI†). Thus, 0.1 g of pure drug (in the form of MF·HCl) or the amount of clay–MF hybrid containing exactly the same amount of metformin was added to 1% (w/v) chitosan solution prepared in 1% acetic acid, whose pH was previously raised to approximately 5 to avoid possible alterations in the clays. After homogenization by magnetic stirring, the resulting gel was introduced into a burette and small drops were poured slowly into 2 M NaOH solution. The rigid gel spheres formed due to the insolubility of chitosan at alkaline pH values were collected after approximately 20 min and rinsed thoroughly until neutral pH. The materials were labelled as CHT/MF, CHT/clay–MFw or CHT/clay–MF. Subsequently, the beads were coated by pectin using a dispersion of this biopolymer at a concentration of 0.5, 1 or 1.5% (v/w). The chitosan spheres remained in contact with the pectin gel for approximately 10 min, and then were collected using a Büchner funnel and immersed in a 10% solution of CaCl2 for approximately 15 min. The coated spheres were collected and rinsed thoroughly with an excess of water (approx. 300 ml) to remove the CaCl2 excess. The samples were labelled as PEC0.5%@CHT/clay–MFXw, PEC0.5%@CHT/clay–MF, PEC1%@CHT/clay–MFXw, and PEC1.5%@CHT/clay–MFXw. The prepared microspheres were frozen at −20 °C with liquid nitrogen and lyophilized (Cryodos −80, Telstar) to produce the final beads tested in the controlled release application.
Preparation of pectin@chitosan@pectin/clay–MF beads.
Pectin spheres incorporating MF, as a pure drug or in the clay–MF hybrid materials, and then double-coated with a layer of chitosan and another layer of pectin were prepared through the following procedure. The pure drug (0.1 g) or a suitable amount of clay–MF hybrid containing the same amount of MF, i.e., 0.1 g, was added to a 5% (v/w) pectin solution. After complete homogenization, the resulting gel was slowly dripped from a burette into 10% (v/w) CaCl2 solution. After about 20 min, the formed spheres were collected and rinsed thoroughly (approx. 300 ml of water) to remove residual Ca2+ ions, the products being denoted as PEC/MF, PEC/clay–MFw, and PEC/clay–MF. Next, the pectin spheres were coated with a layer of chitosan by immersing them in a 0.5% aqueous dispersion of chitosan for 10 min, removed by filtration and then immersed into 2 M NaOH solution. After approximately 10 min, the beads were collected by filtration and washed with abundant water until neutral pH. Subsequently, these spheres went through an additional coating step by immersing them in a 0.5% (v/w) pectin solution. After 10 min, they were transferred to a 10% CaCl2 solution to consolidate the coating, followed by washing with abundant water (approx. 300 ml) to remove residual Ca2+ ions. The bionanocomposite beads were denoted as PEC@CHT@PEC/MF, PEC@CHT@PEC/clay–MFw, and PEC@CHT@PEC/clay–MF. All the prepared materials were frozen at −20 °C with liquid nitrogen and lyophilized (Cryodos −80, Telstar) to produce the consolidated beads for later application as drug delivery systems.
Characterization
Powder X-ray diffraction (XRD) patterns were obtained using a BRUKER D8-ADVANCE diffractometer with a Cu-Kα source, with a goniometer speed of 0.3 s per step between 3 and 70 degree 2θ angle values. The organic content was determined using a LECO CHNS-932 elemental analyser. The morphology of the materials was studied with a FEI NOVA NANO 230 high resolution scanning electron microscope with an EDAX-Ametek detector. The measurement of MF released from the prepared systems was carried out measuring the drug concentration in solution with the help of a UV-1201 spectrophotometer from Shimadzu, using quartz cuvettes with 1 cm path length. Thus, the absorbance of the solutions at 233 nm was used to determine the drug concentration by applying the Lambert–Beer law.
Water absorption properties of the MF-loaded bionanocomposite beads
The water uptake of the pectin–chitosan bionanocomposite beads was evaluated at room temperature by immersing the beads (≈10 mg) in 25 ml of water or in a phosphate buffer solution simulating the pH of the first intestinal zone (around 6.8). At predetermined times, the beads were removed from the liquid, the excess water removed with absorbent paper, and then weighed on an analytical balance. The percentage of adsorbed water was determined from eqn (1) | 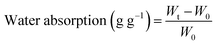 | (1) |
where Wt and W0 are the wet and initial mass of the beads, respectively.
Determination of the encapsulation efficiency of metformin in the pectin–chitosan bionanocomposite beads
After encapsulation of pure MF or the clay–MF hybrids in the biopolymer matrix, the amount of drug incorporated and the encapsulation efficiency were determined in each one of the prepared systems. For this purpose, 0.2 g of beads were immersed in a buffer solution of pH 6.8 prepared with 0.030 g of NaOH, 0.40 g of NaH2PO4·H2O and 0.62 g of NaCl to completely extract the drugs from the beads. The system was maintained under magnetic stirring at 37 °C for 24 h. The supernatant was recovered by centrifugation at 9000 rpm for 10 min, and then the MF content was determined using UV-spectroscopy. The percentage of incorporated metformin and the encapsulation efficiency were calculated using eqn (2) and (3), respectively. |  | (2) |
|  | (3) |
In vitro metformin release studies
The study of the amount of MF released from the prepared systems was carried out in vitro for 8 h, in solutions that simulate the gastrointestinal tract. For this purpose, the materials selected for this study (approximately 5 mg of the clay–MF hybrids or around 50 mg of bionanocomposite beads) were first added to 100 ml of a 0.06 M HCl solution (pH 1.2) containing 0.1% (w/v) NaCl to simulate the fluid in the gastric tract. This medium was kept in a thermostatic bath at 37 °C for 2 h. Then, the pH was raised to 6.8 by adding 0.03 g of NaOH, 0.40 g of NaH2PO4·H2O and 0.62 g of NaCl to the previous solution of pH 1.2, in order to simulate the pH of the first zone of the intestinal fluid (small intestine), and the studied systems were kept in this medium for 2 h. Finally, the system was kept at pH 7.4 for 4 h, by adding 1 M NaOH to the solution of pH 6.8, in order to simulate the pH of the intestinal colon area. At appropriate time intervals, an aliquot of 3 ml was withdrawn, and the amount of MF released from the drug-loaded beads was determined by UV spectrophotometry (λ = 233 nm), applying the Lambert–Beer law with a molar absorption coefficient of 13
424 l mol−1 cm−1. After the measurement, the aliquot was added back into the solution in order to keep the volume constant. All the experiments were carried out in triplicate. For the measurement of MF released at pH 1.2, the pH was raised to 7 by adding a buffer solution, so that the MF band at λ = 233 nm can be observed. In this case, the collected aliquot (1 ml) is not returned to the main solution, and 1 ml of fresh pH 1.2 solution is replenished to keep the volume constant. All the experiments were performed at least in triplicate.
Conflicts of interest
There are no conflicts to declare.
Acknowledgements
This work was supported by the MINECO (Spain) and FEDER (EU) funds (project MAT2015-71117-R). EPR acknowledges the CNPq (Brazil) for the fellowship 204360/2014-5. We acknowledge support of the publication fee by the CSIC Open Access Publication Support Initiative through its Unit of Information Resources for Research (URICI).
Notes and references
- R. Turner, Lancet, 1998, 352, 854–865 CrossRef.
- S. M. Setter, L. J. Iltz, J. Thams and K. R. Campbell, Clin. Ther., 2003, 25, 2991–3026 CrossRef CAS PubMed.
- M. Foretz, B. Guigas, L. Bertrand, M. Pollak and B. Viollet, Cell Metab., 2014, 20, 953–966 CrossRef CAS PubMed.
- P. A. Marmwar and M. C. Singh, Asian J. Pharm., 2016, 10, 7–15 CAS.
- H. M. Mansour, M. Sohn, A. Al-Ghananeem and P. P. DeLuca, Int. J. Mol. Sci., 2010, 11, 3298–3322 CrossRef CAS PubMed.
- K. Modi, M. Modi, D. Mishra, P. Mittal, U. Sorathiya and P. Shelat, Int. Res. J. Pharm., 2013, 4, 70–76 CrossRef CAS.
- C. da Costa Fernandes Jr., T. S. Pinto, H. R. Kang, P. Magalhães Padilha, I. H. J. Koh, V. R. L. Constantino and W. F. Zambuzzi, Adv. Biosyst., 2019, 3, 1–14 Search PubMed.
- C. Aguzzi, P. Cerezo, C. Viseras and C. Caramella, Appl. Clay Sci., 2007, 36, 22–36 CrossRef CAS.
- J.-M. Oh, D.-H. Park, S.-J. Choi and J.-H. Choy, Recent Pat. Nanotechnol., 2012, 6, 200–217 CrossRef CAS PubMed.
- C. Viseras, P. Cerezo, R. Sanchez, I. Salcedo and C. Aguzzi, Appl. Clay Sci., 2010, 48, 291–295 CrossRef CAS.
- M. Hun Kim, G. Choi, A. Elzatahry, A. Vinu, Y. Bin Choy and J.-H. Choy, Clay Clay Miner., 2016, 64, 115–130 CrossRef.
- J. Choy, S. Choi, J. Oh and T. Park, Appl. Clay Sci., 2007, 36, 122–132 CrossRef CAS.
- J.-H. Yang, J.-H. Lee, H.-J. Ryu, A. A. Elzatahry, Z. A. Alothman and J.-H. Choy, Appl. Clay Sci., 2016, 130, 20–32 CrossRef CAS.
- A. George, P. A. Shah and P. S. Shrivastav, Int. J. Pharm., 2019, 561, 244–264 CrossRef CAS PubMed.
- E. Ruiz-Hitzky, M. Darder, B. Wicklein, F. A. Castro-Smirnov and P. Aranda, Clay Clay Miner., 2019, 67, 44–58 CrossRef.
-
M. I. Carretero, C. S. F. Gomes and F. Tateo, Developments in Clay Science, Elsevier Ltd., 2013, vol. 5, pp. 711–764 Search PubMed.
- R. H. Mueller, K. Maeder and S. Gohla, Eur. J. Pharm. Biopharm., 2000, 50, 161–177 CrossRef.
- C. Viseras, C. Aguzzi, P. Cerezo and A. Lopez-Galindo, Appl. Clay Sci., 2007, 36, 37–50 CrossRef CAS.
- L. N. M. Ribeiro, A. C. S. Alcântara, M. Darder, P. Aranda, F. M. Araújo-Moreira and E. Ruiz-Hitzky, Int. J. Pharm., 2014, 463, 1–9 CrossRef CAS PubMed.
- A. C. S. Alcântara, P. Aranda, M. Darder and E. Ruiz-Hitzky, J. Mater. Chem., 2010, 20, 9495–9504 RSC.
- L. Lisuzzo, G. Cavallaro, S. Milioto and G. Lazzara, New J. Chem., 2019, 43, 10887–10893 RSC.
- J. K. Park, Y. B. Choy, J.-M. Oh, J. Y. Kim, S.-J. Hwang and J.-H. Choy, Int. J. Pharm., 2008, 359, 198–204 CrossRef CAS PubMed.
- A. C. S. Alcântara and M. Darder, Chem. Rec., 2018, 18, 696–712 CrossRef PubMed.
- D.-H. Park, S.-J. Hwang, J.-M. Oh, J.-H. Yang and J.-H. Choy, Prog. Polym. Sci., 2013, 38, 1442–1486 CrossRef CAS.
- E. P. Rebitski, G. P. Souza, S. A. A. Santana, S. B. C. Pergher and A. C. S. Alcântara, Appl. Clay Sci., 2019, 173, 35–45 CrossRef CAS.
- E. P. Rebitski, A. C. S. Alcântara, M. Darder, R. L. Cansian, L. Gómez-Hortigüela and S. B. C. Pergher, ACS Omega, 2018, 3, 13538–13550 CrossRef CAS PubMed.
- A. S. Oliveira, A. C. S. Alcântara and S. B. C. Pergher, Mater. Sci. Eng., C, 2017, 75, 1250–1258 CrossRef CAS PubMed.
- L. Lisuzzo, G. Cavallaro, F. Parisi, S. Milioto, R. Fakhrullin and G. Lazzara, Coatings, 2019, 9, 1–8 CrossRef.
- E. P. Rebitski, M. Darder, C. I. Sainz-Díaz, R. Carraro, P. Aranda and E. Ruiz-Hitzky, Appl. Clay Sci., 2020, 186, 105418 CrossRef CAS.
- R. García-Vázquez, E. P. Rebitski, L. Viejo, C. de Los Ríos, M. Darder and E. M. García-Frutos, Appl. Clay Sci., 2020, 189, 105541 CrossRef.
- Y. Yahia, F. García-Villén, A. Djelad, L. S. Belaroui, R. Sanchez-Espejo, M. Sassi, A. López-Galindo and C. Viseras, Appl. Clay Sci., 2019, 180, 105169 CrossRef CAS.
- L. Lisuzzo, B. Wicklein, G. Lo Dico, G. Lazzara, G. del Real, P. Aranda and E. Ruiz-Hitzky, Dalton Trans., 2020 10.1039/C9DT03804C.
- E. P. Rebitski, P. Aranda, M. Darder, R. Carraro and E. Ruiz-Hitzky, Dalton Trans., 2018, 47, 3185–3192 RSC.
- F. Shahidi, J. K. V. Arachchi and Y.-J. Jeon, Trends Food Sci. Technol., 1999, 10, 37–51 CrossRef CAS.
- P. Sriamornsak, Expert Opin. Drug Delivery, 2011, 8, 1009–1023 CrossRef CAS PubMed.
- N. V. K. Yadu, K. M. Raghvendra, V. Aswathy, P. Parvathy, S. Sunija, M. S. Neelakandan, S. Nitheesha and K. A. Vishnu, Res. Dev. Mater. Sci., 2017, 2, 170–185 Search PubMed.
- J. Shimoda, H. Onishi and Y. Machida, Drug Dev. Ind. Pharm., 2001, 27, 567–576 CrossRef CAS PubMed.
- T. M. M. Ways, W. M. Lau and V. V. Khutoryanskiy, Polymers, 2018, 10, 267 CrossRef PubMed.
- V. B. V. Maciel, C. M. P. Yoshida and T. T. Franco, Carbohydr. Polym., 2015, 132, 537–545 CrossRef CAS PubMed.
- D. Cheikh, F. García-Villén, H. Majdoub, M. B. Zayani and C. Viseras, Appl. Clay Sci., 2019, 172, 155–164 CrossRef CAS.
- A. Synytsya, Carbohydr. Polym., 2003, 54, 97–106 CrossRef CAS.
- K. Itoh, T. Hirayama, A. Takahashi, W. Kubo, S. Miyazaki, M. Dairaku, M. Togashi, R. Mikami and D. Attwood, Int. J. Pharm., 2007, 335, 90–96 CrossRef CAS PubMed.
- S. J. Clark, M. D. Segall, C. J. Pickard, P. J. Hasnip, M. I. J. Probert, K. Refson and M. C. Payne, Z. Kristallogr. – Cryst. Mater., 2005, 220, 567–570 CAS.
-
Biovia, Materials Studio, San Diego, CA, USA, 2018.
- S. K. Chinnaiyan, K. Deivasigamani and V. R. Gadela, Int. J. Biol. Macromol., 2019, 125, 278–289 CrossRef CAS PubMed.
- C. Remuñán-López and R. Bodmeier, J. Controlled Release, 1997, 44, 215–225 CrossRef.
Footnote |
† Electronic supplementary information (ESI) available: FESEM images of the bead surface, XRD patterns of hybrid and bionanocomposite film samples and scheme of the general procedure for the preparation of the bionanocomposite beads. See DOI: 10.1039/c9nj06433h |
|
This journal is © The Royal Society of Chemistry and the Centre National de la Recherche Scientifique 2020 |
Click here to see how this site uses Cookies. View our privacy policy here.