DOI:
10.1039/C9NH00291J
(Review Article)
Nanoscale Horiz., 2020,
5, 25-42
Biomimetic nanoparticle technology for cardiovascular disease detection and treatment
Received
5th May 2019
, Accepted 28th June 2019
First published on 28th June 2019
Abstract
Cardiovascular disease (CVD), which encompasses a number of conditions that can affect the heart and blood vessels, presents a major challenge for modern-day healthcare. Nearly one in three people has some form of CVD, with many suffering from multiple or intertwined conditions that can ultimately lead to traumatic events such as a heart attack or stroke. While the knowledge obtained in the past century regarding the cardiovascular system has paved the way for the development of life-prolonging drugs and treatment modalities, CVD remains one of the leading causes of death in developed countries. More recently, researchers have explored the application of nanotechnology to improve upon current clinical paradigms for the management of CVD. Nanoscale delivery systems have many advantages, including the ability to target diseased sites, improve drug bioavailability, and carry various functional payloads. In this review, we cover the different ways in which nanoparticle technology can be applied towards CVD diagnostics and treatments. The development of novel biomimetic platforms with enhanced functionalities is discussed in detail.
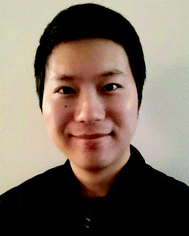
Joon Ho Park
| Joon Ho Park is a graduate student researcher in the Department of NanoEngineering at the University of California San Diego. He received his BS and MS in Chemical and Biological Engineering at Seoul National University. His research interests include engineering biomimetic nanoparticles for targeted drug delivery. |
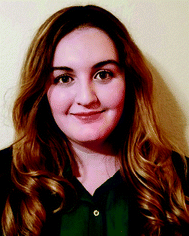
Diana Dehaini
| Diana Dehaini is a graduate student researcher in the department of NanoEngineering at the University of California San Diego. She received her BS in NanoEngineering at the University of California San Diego. Her research interests focus on the application of biomimetic nanoparticles for drug delivery and infectious disease treatment. |
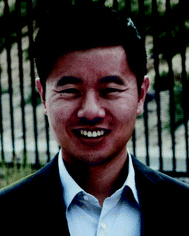
Ronnie H. Fang
| Ronnie H. Fang is an Assistant Project Scientist in the Department of NanoEngineering at the University of California San Diego. He received his PhD in NanoEngineering at the University of California San Diego. His research is focused on leveraging biomimetic nanoparticles for drug delivery and immunoengineering applications. |
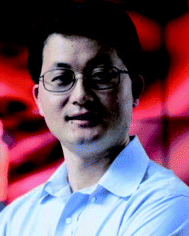
Liangfang Zhang
| Liangfang Zhang is a Professor in the Department of NanoEngineering and Moores Cancer Center at the University of California San Diego. He received his PhD in Chemical and Biomolecular Engineering at the University of Illinois at Urbana-Champaign. His research aims to create cutting-edge biomimetic nanotechnologies and exploit them for various biomedical applications with a particular focus on drug delivery, biodetoxification, and vaccination. |
1. Introduction
Cardiovascular disease (CVD) encompasses a number of conditions that afflict the heart and vasculature.1 These can include those that affect the blood vessels, such as coronary artery, peripheral artery, or cerebrovascular disease, as well as heart-related conditions that can lead to high blood pressure, irregular heartbeat, or chest pain caused by reduced blood flow, among many others. Oftentimes, the underlying cause is the buildup of atherosclerotic plaque, which results in the narrowing of blood vessels and restricts blood flow.2 Some risk factors for atherosclerosis include high cholesterol, high blood pressure, diabetes, smoking, and poor diet.3,4 If left untreated, chronic types of CVD can precipitate into a single traumatic event, such as a myocardial infarction or stroke, both of which are associated with high mortality rates.5–8 In developed countries, CVD represents the leading cause of death, and it is responsible for almost one third of all deaths worldwide.9 In the United States, more than 30% of the population suffers from some form of CVD.10 Despite advances in healthcare technology and reduced mortality rates, the total number of deaths is expected to increase into the future,11 highlighting the need for continued technological innovation with respect to the clinical management of CVD.
It is believed that a majority of the deaths resulting from CVD are completely preventable, oftentimes by basic modifications in lifestyle habits.12–14 While this underlines the importance of improved education and large-scale prevention initiatives, it also stresses the importance of having effective means for detecting signs of disease in the clinic, particularly at an early stage.15,16 Currently, simple functional and laboratory tests can be used to identify broad changes in physiological parameters and blood chemistry, which can oftentimes provide some rough indicators regarding the risk of CVD development.17 Patients exhibiting overt symptoms can be subjected to additional tests and various forms of imaging.18,19 More invasive techniques may be applied for definitive diagnosis, although they can carry significant risks that must be carefully weighed.20 Overall, there is a strong need for more reliable diagnostics and safe, noninvasive imaging modalities that can be used as screening tools to provide accurate information about CVD development over time.
The type of treatment for CVD largely depends on the stage of the disease, particularly for those conditions that have pathogenesis spanning across decades.21–24 As some forms of CVD progress, they can start to significantly affect quality of life, at which point invasive surgery is oftentimes indicated.25 During a traumatic event such as a heart attack or stroke, immediate treatment in the form of thrombolytics can be given to help dissolve the blood clots that are often the underlying cause.26,27 In some cases, endovascular removal of the clot may also be indicated.28 Despite advances in cardiovascular medicine, a majority of treatment options still seek to manage symptoms rather than address the underlying cause of disease.
Nanotechnology has been widely applied to the medical field, enabling researchers to design novel diagnostic and therapeutic platforms that can outperform traditional modalities.29–32 In particular, nanoparticle-based delivery systems have had a major impact on the clinical management of cancer, with a number of nanoformulations having been approved for use in human patients.33 Their advantages include the ability to enhance bioavailability by prolonging blood residence, deliver a wide range of payloads, and sustain release of therapeutic agents over time. Significant efforts have also been placed on developing actively targeted formulations, which can more accurately localize to disease sites.34–36 Many of these design principles are now being leveraged by researchers working in other fields, including CVD, where they also have the potential to bring about significant improvements.37,38 More recently, the application of biomimetic nanotechnology has become increasingly popular as a means of streamlining the creation of highly functional nanoparticle platforms.39,40 In particular, these nanoparticles excel at performing in complex biological environments.41 In this review, we first provide a brief overview of the current clinical management of CVD. Then, the various ways in which nanotechnology has been applied towards CVD diagnosis and treatment will be discussed. Finally, novel biomimetic platforms for CVD management will be covered (Fig. 1).
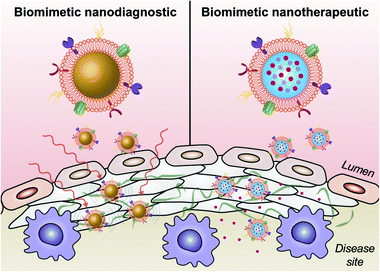 |
| Fig. 1 Biomimetic nanotechnology for cardiovascular disease (CVD) management. Nanoparticle delivery platforms designed based on biomimetic principles can more readily be targeted to disease sites within the cardiovascular system. When combined with the advantages of traditional nanodelivery systems, the enhanced localization of imaging and drug payloads afforded by biomimetic nanotechnology can ultimately enable the development of more effective diagnostic and therapeutic modalities for CVD, respectively. | |
2. Current clinical management
2.1 Diagnostics
2.1.1 Diagnostic assays.
Current clinically used analytical techniques allow for quantification of a wide range of different parameters, including routine blood cell counts and biochemistry, as well as levels of more specialized markers that can be used for disease diagnosis. The biomarkers and assays relevant to CVD detection can be categorized by tying them to their respective pathological processes.42 Especially, since levels of lipids and lipoproteins are well-established markers of an abnormal cardiovascular state, blood panels often look closely at levels of cholesterol, low-density lipoprotein (LDL), and high-density lipoprotein (HDL). Other biomarkers such as C-reactive protein, interleukin 6, fibrinogen, monocyte chemotactic protein-1, and tumor necrosis factor alpha are tied to inflammation in CVD. Autoantibodies have also been implicated in the atherogenesis process and have been proposed as potential biomarkers for CVD.43 Although numerous biomarkers have been researched and used for the diagnosis of CVD, current techniques often require costly and time-consuming bloodwork tests.44 Rather than relying on bloodwork, other noninvasive techniques such as electrocardiography can be used to detect certain conditions such as arrhythmias that can be highly related to deteriorating cardiovascular health.45
2.1.2 Imaging.
The ability to visualize disease development is oftentimes an essential tool employed by clinicians in the management of CVD. Ultrasound is one of the most widely used techniques for imaging various cardiovascular conditions. Echocardiograms and vascular ultrasounds can be used to identify the locations of clots or abnormal circulation.46 For more detailed imaging, methods such as computed tomography (CT), single-photon emission CT (SPECT), coronary angiography (CA), positron emission tomography (PET), and magnetic resonance imaging (MRI) may be required to provide sufficient information for diagnosis.15 SPECT is a technique whereby gamma rays are used to image and recreate three-dimensional images of organs.47 Radioisotopes, which can sometimes be used to label materials that exhibit specificity for CVD disease sites, are used in this imaging method.48 Diagnosis by coronary CT angiography is relatively safe compared with CA, which represents the current gold standard for diagnosing coronary artery disease.49 CA requires catheterization, whereby contrast dyes are directly injected into the coronary artery, while CT angiography only requires intravenous administration of the contrast agent; in both cases, imaging is done by an X-ray instrument. Although costly, PET can be used to image atherosclerotic plaque inflammation by detecting pairs of gamma rays emitted by tracers such as [18F]-fluorodeoxyglucose.50 In order to benefit from multiple modalities at once, techniques such as SPECT-CT and PET-CT are increasingly being employed.51 Depending on the type of CVD, there are also instances where MRI technology can be used for diagnosis and to determine the proper course of treatment.52 Delayed enhancement MRI, for example, can help distinguish between healthy and diseased heart tissue, which is helpful for evaluation after a myocardial infarction. However, many patients, particularly the elderly, cannot be subjected to MRI due to the presence implants such as hearing aids, pacemakers, and metal joint or bone replacements.53 Although there have been significant advancements in the imaging of CVD, patients still often must be administered a significant amount of contrast agent, and results are generally subject to interpretation, which can lead to misdiagnosis.54,55
2.2 Treatments
2.2.1 Therapeutic drugs.
Anticoagulants, also known as blood thinners, are used to decrease blood clotting.56 Although these drugs do not dissolve existing blood clots, they can inhibit formation of new clots or prevent existing clots from growing. Commonly used drugs include rivaroxaban, heparin, and warfarin.57–59 Antiplatelet agents such as aspirin and clopidogrel work by preventing platelets from aggregating and are commonly used for blood clot prevention.60 In contrast to anticoagulants, thrombolytics, also referred to as clot busters, work by directly dissolving a thrombus.61 These drugs are commonly indicated in the event of a traumatic event, such as myocardial infarction or ischemic stroke, and are used to help restore blood flow. Although they can be effective, thrombolytics may greatly effect hemostasis, resulting in some significant side effects.62
Instead of treating blood clots, drugs such as captopril and ramipril can reduce the load on blood vessels.63 These belong to the class of angiotensin-converting enzyme inhibitors that work by decreasing angiotensin II levels. Their use leads to expansion of blood vessels, reduced blood pressure, and easier blood flow. Another similar strategy involves blocking of the angiotensin II receptor rather than reducing the levels of angiotensin II.64 Drugs such as candesartan and losartan act in this way and prevent angiotensin II from interacting with blood vessels. Angiotensin-converting enzyme inhibitors and angiotensin II receptor blockers are generally used to manage CVD that are associated with high blood pressure. Vasodilators such as nitroglycerin, isosorbide dinitrate, nesiritide, and nitrates are currently being used in the clinic in various forms.65,66 Another way of lowering blood pressure is to induce diuresis,67 promoting the body to excrete fluids and sodium. Using diuretic drugs to reduce excess liquid can help lower blood pressure and relieve the heart's workload. Not only do they help to address high blood pressure, but these drugs can also help patients to reduce swelling in other parts of their bodies.
Sometimes, drugs are used to directly affect the heart. For example, calcium channel blockers interfere with the flux of calcium into cardiac myocytes, which can reduce heart rate or the strength at which the heart pumps.68 Drugs such as amlodipine, diltiazem, felodipine, nifedipine, nisoldipine, and verapamil are approved for treating hypertension and have proven to be effective for angina pectoris and cardiac arrhythmias. Beta-blockers, also known as beta-adrenergic blocking agents, can reduce heart rate as well as cardiac output.69 Calcium channel blockers and beta-blockers can be combined for chronic stable angina pectoris therapy.70 In conditions such as acute myocardial infarction or arrhythmia, digoxin, also known as digitalis, can help patients to restore their heart function by increasing the force of contraction.71 Digoxin acts by blocking atrioventricular conduction and can slow down certain types of arrhythmia, especially atrial fibrillation.72
Some other commonly used strategies for CVD include use of cholesterol-lowering drugs such as statins, nicotinic acids, and cholesterol absorption inhibitors.73 The latter two are mostly used when patients are not responding well to statins, including when potency is lacking or when side effects are present. High levels of LDL cholesterol are characteristic of hypercholesterolemia, a common cause of myocardial infarction. Therefore, statin drugs such as atorvastatin and rosuvastatin are often prescribed to lower the chance of heart attack and stroke.
2.2.2 Surgery.
No matter how many preventative drugs and strategies are used, physical interventions are often needed to treat the growth of a clot, development of atherosclerosis, or other cardiovascular conditions. Stents are commonly used to keep arteries open after angioplasty procedures, using a strong material often in combination with immunosuppressive and anti-clotting drugs.74 Their use can become necessary when there is significant arterial blockage, a specific region has had severe cardiovascular damage, or an aneurysm has likelihood to burst. Stents can be made of many materials, and those used clinically include metallic, plastic, and coated or drug-eluting variants. These devices have greatly improved survival rates from CVD events. Drug-eluting stents, which typically dispense sirolimus or paclitaxel, promote immunosuppression and acceptance of foreign material, while also reducing lumen diameter by preventing cell overgrowth.75 Patients with severe coronary heart disease may receive coronary artery bypass graft surgery to improve blood flow to the heart.76 The surgery involves grafting or connecting healthy arteries or veins to bypass the blocked area of the coronary artery, thus creating a new path for blood flow. Both angioplasty and bypass surgeries can be indicated in emergency situations, such as during myocardial infarction.77 Additionally, endovascular thrombectomy procedures that involve physical clot disruption and retrieval can lead to high rates of recanalization and good patient outcomes in many cases.78
3. Nanoparticles for CVD
3.1 Introduction
Nanotechnology has been increasingly used over the past several decades for medical applications.31 Currently, there are a number of clinically approved nanoformulations, such as Doxil, a liposomal version of doxorubicin, and Abraxane, an albumin-based version of paclitaxel.79,80 Nanoparticles, which range from a few nanometers to several hundred nanometers in size, can be fabricated using a variety of different materials, including polymers, lipids, metals, and inorganic compounds, which have been covered in-depth in previous reviews.32,81–83 A major reason for their appeal is that, at their nanoscale dimensions, these particles exhibit unique properties that are not observed at other size scales.84 Traditionally, nanoparticles have been employed as drug carriers for anticancer therapies due to their ability to be passively targeted via the enhanced permeation and retention effect, which takes advantage of the inherent disorder associated with tumor vasculature.85 Beyond their targeting capabilities, nanoparticles are also adept at loading payloads with varying properties, and this can be done by encapsulation within the nanomaterial matrix or by surface attachment.86,87 Depending on the specific platform, it is even possible to load both hydrophilic and hydrophobic payloads within the same nanocarrier.88 By modulating the material properties, sophisticated time-dependent release strategies can be established. Instead of relying on encapsulated cargoes, the nanomaterial itself can at times act as the functional component, which is the case for certain metallic or inorganic materials that have unique optical and photothermal conversion properties.89
Due to their small size, nanoparticles have a particularly high surface area to volume ratio. With regards to medical applications, this property provides a number of opportunities as well as challenges.90 One hurdle that must be overcome when introducing foreign nanomaterials into the body is immune clearance, whereby interactions with various proteins and cells can significantly compromise performance and efficacy.91 In order to prevent unwanted nonspecific interactions, different synthetic strategies have been developed in order to better shield nanoparticles from their surrounding environments. This includes the use of polyethylene glycol (PEG), which provides a hydration layer around the nanoparticles and sterically hinders protein adsorption and cellular engagement.92,93 PEGylation and coating with other synthetic materials, such as zwitterionic polymers,94 have been used as effective means of enhancing nanoparticle half-life in plasma. Besides stealth polymer coatings, other ligands can also be introduced onto the surface of nanoparticles to further enhance functionality.95 While surface functionalization can be used for therapeutic and imaging payloads, it is most commonly reserved for targeting moieties that can help to further improve localization to a site of interest.35 Examples of commonly used ligands include small molecules such as folate, or biomolecules such as small peptides, antibodies, and aptamers, all of which can be used to enhance nanoparticle specificity. Translational efforts for targeted nanoformulations are well underway, and some have recently been evaluated in clinical settings.96 In this section, we discuss the various CVD-related applications that can benefit from nanoparticle technology.
3.2 Diagnostics
3.2.1 Blood tests and sensors.
The cardiac troponin complex consists of three regulatory proteins, of which troponin I (cTnI) and troponin T (cTnT) are released into the bloodstream when cardiomyocytes are damaged.97 Since both cTnI and cTnT are undetectable in healthy patients, they represent good biomarkers for the diagnosis of acute myocardial infarction.98 In efforts to improve the detection of CVD biomarkers such as troponins, nanoparticle-based diagnostics are being developed. In particular, many of these new platforms have been based on electrochemical immunosensors, which are relatively simple and low-cost compared to other systems.99 Owing to their ease of miniaturization and their specificity, these sensors are well-suited for point-of-care applications. In terms of their construction, electrochemical immunosensors consist of a bioreceptor element, which can comprise of antibodies responsible for specifically detecting the target antigen, and a transducer that converts the binding events into electrical signals.100 Along these lines, an amperometric sensor, which detects changes in electrical currents caused by oxidation or reduction in biochemical reactions, was developed for the detection of cTnT using an ionic organic molecule, (E)-4-[(4-decyloxyphenyl)diazenyl]-1-methylpyridinium iodide, and chitosan-stabilized gold nanoparticles.101 In this case, the organic molecule acted as a redox probe, while monoclonal anti-cTnT antibodies were immobilized onto the gold nanoparticles to detect cTnT in the sample. The signal decrease was shown to be proportional to cTnT concentration, thus allowing for quantification of the analyte.
Other types of biosensors for CVD detection include those based on electrochemical impedance, where changes at the electrode caused by interactions with charged antigens are detected.100 In one study, gold nanoparticles were deposited onto a glassy carbon electrode, and a recognition peptide (CFYSHSFHENWPS), which has high affinity towards cTnI, was further assembled onto the nanoparticles.102 The charge transfer resistance of the biosensor was proportional to the concentration of cTnI, which allowed for detection of the analyte in a quantitative and label-free fashion, even with real serum samples. Potentiometric sensors, which detect analyte concentration by measuring electrical potential differences between a working electrode and a reference electrode,103 have also been developed for CVD diagnosis.104 Using a molecular imprinting technique, an artificial anti-cTnT antibody was synthesized and attached onto the surface of multiwalled carbon nanotubes for use as the recognition element. The resulting sensor was able to specifically detect cTnT in serum samples. Interaction of analytes with electrodes also cause changes in conductance, which can be detected by conductometric sensors.105 Various antibodies against biomarkers for CVD, including myoglobin, cTnI, creatine kinase-MB, and b-type natriuretic peptide, have been used to create single site-specific polyaniline nanowire biosensors.106 In each case, the measured signal from the sensor showed a linear correlation with biomarker concentration, enabling detection within a few minutes.
3.2.2 Imaging.
Traditionally, imaging technology utilizing gold nanoparticles has mostly been developed for cancer-related applications.107 Harnessing their favorable biocompatibility and versatility, gold nanoparticles have more recently been applied to the cardiovascular field. Photoacoustic imaging, in which gold nanoparticles can be employed, relies on the thermal expansion of contrast agents or tissues when pulsed laser beams are absorbed.108 This pulsed expansion generates acoustic signals, which are then picked up by an ultrasound transducer. This imaging modality has been applied to visualize atherosclerotic plaque using gold nanoshells designed to target vascular cell adhesion molecule 1, which enabled their accumulation in disease-prone regions of the vasculature.109 With a pulse width of 10 ns at a repetition rate of 20 Hz, sub-millimeter imaging resolution could be achieved. Photoacoustic imaging can also be combined with ultrasound imaging techniques to provide better assessments of atherosclerotic plaque. In an example, gold nanorods were used for combinatorial intravascular photoacoustic and ultrasound imaging of atherosclerotic plaques ex vivo.110 The photoacoustic modality could be used to detect lipid-rich regions, which are closely related to the progression of atherosclerosis, while the ultrasound imaging helped to provide structural information. Optical coherence tomography (OCT) is another cardiovascular imaging technique that has utilized gold nanoparticles. In the technique, infrared signal from a broadband coherent light source is directed to the target tissue, and an image is constructed based on the backscattered light.111 Gold nanoparticles can be used as a powerful signal enhancer since they exhibit a high backscattering coefficient.112 In particular, there have been reports demonstrating the potency of gold nanoshells as a contrast agent in cardiovascular OCT, whereby they could promote detection of individual cells in biocompatible fluids.113 CT imaging is a powerful noninvasive imaging technique which reconstructs cross-sectional images of an object from X-ray signals obtained at different angles.114 Because gold has a high atomic number and high mass absorption coefficient, gold nanoparticles are highly attractive for CT applications and could potentially outperform traditional iodine-based contrast agents.115 Along these lines, gold nanoparticles have been used to label monocytes, which were then recruited to atherosclerotic plaques in vivo.116 The delivery of the nanoparticles helped to greatly increase X-ray attenuation in the aorta, yielding better sensitivity in measuring monocyte accumulation in sites of atherosclerosis.
MRI is a noninvasive technique that can provide detailed information about vasculature that is crucial for effective diagnosis of CVD.117 Gadolinium (Gd) is used as a T1-weighted contrast agent in MRI to generate positive signal due to its high paramagnetism. Commercially, Gd diethylenetriaminepentaacetic acid (Gd-DTPA) has been approved by the United States Food and Drug Administration since 1988.118 In order to better image and diagnose atherosclerotic plaques using MRI, Gd-DTPA has been encapsulated within poly(lactic-co-glycolic acid) (PLGA) or polylactide–PEG nanoparticles to allow for longer circulation and better plaque accumulation by various targeting mechanisms.119 The payload was encapsulated inside of the polymeric nanoparticles without compromising its ability to create contrast in a manner comparable to free Gd-DTPA, and the resulting formulations exhibited minimal toxicity. In another study, a Gd-based contrast agent was loaded into an HDL nanocarrier.120 This platform exploited the ability of HDL with a methionine oxidation in apolipoprotein A-I to be taken up by macrophages, which are often concentrated in atherosclerotic plaques.
Superparamagnetic iron oxide nanoparticles have been widely used as MRI contrast agents.121 Unlike Gd, which is a T1 contrast agent, iron oxide nanoparticles act as T2-weighted contrast agents that generate negative signal. The nanoparticles also hold certain advantages over Gd, particularly with regards to safety. In order to capture the effect of both T1 and T2 contrast agents, dual-modal systems have been proposed. Core–shell Fe3O4/Gd2O3 nanocubes have been synthesized and reported to enhance the contrast between positive and negative signals to overcome the risk of generating pseudo-positive and pseudo-negative signals in vivo.122 It was revealed that the longitudinal relaxivity of the nanoparticles was about twofold that of Gd2O3 and their transverse relaxivity was also about twofold compared with Fe3O4. In another dual-modal imaging platform using iron oxide nanoparticles, the fluorescent dye Cy5.5 was conjugated onto the surface of dimercaptosuccinic acid-coated Fe3O4 nanoparticles for concurrent near-infrared fluorescence imaging and MRI.123 Further functionalization with antibody against profilin-1 resulted in effective targeting of atherosclerotic plaque in the carotid artery and enabled in vivo imaging. The coating of iron oxide with other materials such as gold is a strategy that can be used to protect against oxidation and provide ease of functionalization.124 In order to target CD163, which is upregulated in macrophages around atherosclerotic plaques, gold-coated iron oxide nanoparticles have been decorated with antibodies against the surface marker. In vivo testing revealed that the anti-CD163-functionalized nanoparticles accumulated in the site of atherosclerotic lesions, allowing for their detection by MRI. Another targeted nanoplatform took advantage of the fact that apoptotic cells at the site of atherosclerosis have phosphatidylserine exposed on their surfaces, unlike healthy cells in which the lipid is located in the inner leaflet of the plasma membrane (Fig. 2).125 Annexin V is a well-known protein that can specifically bind to phosphatidylserine, and decoration of 99mTc-labeled ultrasmall iron oxide nanoparticles with the ligand enabled the targeting of apoptotic macrophages for dual-modal SPECT/MRI detection of vulnerable plaques.
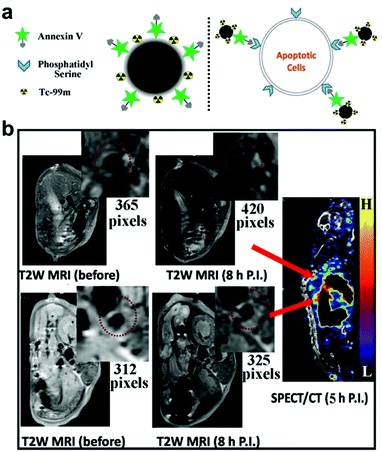 |
| Fig. 2 Nanoparticle-based diagnostic for CVD. (a) An ultrasmall iron oxide nanoparticle functionalized with a 99mTc label and annexin V can target apoptotic cells at the site of atherosclerosis, which present phosphatidylserine on their surfaces, for dual-modality imaging. (b) When the nanoimaging agent was administered into mice with atherosclerosis, plaques could readily be detected by both MRI and SPECT. Adapted with permission.125 Copyright 2015, American Chemical Society. | |
Aside from the more prominent materials described above, other types of nanomaterials have been employed for CVD diagnostics. Carbon nanotubes, which possess unique mechanical and electrical properties, have been widely applied to many other fields, including electronics and tissue engineering.126,127 For imaging purposes, it has been demonstrated that functionalized multiwalled carbon nanotubes can be used as contrast agents for ultrasonography. In an example, the nanotubes were oxidized and then functionalized by 1,3-dipolar cycloaddition of azomethine ylides for biocompatibility.128 The highly echogenic property of these nanostructures allowed them to generate ultrasound signal comparable to that of a commercially available contrast agent, sulfur hexafluoride, without causing any toxicity. In an ex vivo experiment, the platform could be used to image a pig heart, as well as the liver and bladder. While surface modification is the most common method for introducing targeting functionality onto nanoformulations, there are examples in which the nanoparticle itself can exhibit affinity towards specific cell populations. Other than HDL-based nanocarriers, polyglucose nanoparticles have been designed to act as glycogen biomimetics capable of targeting macrophages.129 In order to use this platform for PET, it was labeled with the isotope 18F. The size of the nanoparticles was optimized to accelerate renal excretion for rapid clearance from blood, thus improving target-to-background ratios. When administered in vivo, the particles were preferentially taken up by macrophages, leading to enrichment in inflamed cardiovascular tissue and better PET signal.
3.3 Therapeutics
3.3.1 Targeted drug delivery.
Chronic inflammation like what is found in advanced atherosclerotic lesions generally requires medical intervention in order to be resolved. The proresolving action of annexin A1, a glucocorticoid-regulated protein, is mediated through its receptor N-formyl peptide receptor 2, and a short peptide fragment from the amino-terminal end of the protein has been loaded into collagen IV-targeted nanoparticles.130 Administration of the formulation decreased lesional collagenase activity, oxidative stress, and plaque necrosis in an animal model of atherosclerosis. In a similar approach, the anti-inflammatory cytokine interleukin 10 was loaded into polymeric nanoparticles capable of controlled release and atherosclerotic plaque targeting.131 The formulation was fabricated by a nanoprecipitation process using a chip-based device that enabled the activity of the cytokine to be retained even after exposure to organic solvents. The nanoformulation proved to be more effective than the native cytokine, preventing formation of vulnerable plaque in advanced lesions by increasing the thickness of the fibrous cap while reducing the size of the necrotic cores.
Reactive oxygen species (ROS), along with the associated oxidative stress, are another key player in the pathogenesis of atherosclerosis.132 In an effort to develop an antioxidative nanotherapeutic, tempol and phenylboronic acid pinacol ester were covalently conjugated onto cyclic polysaccharide β-cyclodextrin and processed into nanoparticle form.133 Tempol acted as a superoxide dismutase mimetic agent and phenylboronic acid pinacol ester provided hydrogen peroxide-eliminating function. Intravenous administration of the nanoparticles resulted in their accumulation at atherosclerotic lesions of apolipoprotein E (ApoE)-deficient mice by passive targeting. This not only inhibited the development of atherosclerosis, but also helped to stabilize the plaques, which exhibited fewer cholesterol crystals, a smaller necrotic core, thicker fibrous caps, a lower number of macrophages, and a reduced amount of matrix metalloproteinase-9.
As discussed in previous sections, statins can be used to control a variety of CVD by lowering LDL cholesterol levels.134 In one study, pitavastatin was incorporated into PLGA nanoparticles that were then taken up by Ly-6G−CD11b+ monocytes after intravenous administration and subsequently delivered into atherosclerotic plaques.135 Adoptive transfer of CCR2+/+Ly-6Chigh inflammatory macrophages into ApoE knockout mice infused with angiotensin II was employed to promote plaque destabilization. It was found that administration of the pitavastatin-loaded nanoparticles reduced plaque destabilization and rupture by inhibiting the secretion of monocyte chemoattractant protein-1 and matrix metalloproteinase-9. In the same study, plasmids with the 7ND gene, a deletion mutant of monocyte chemoattractant protein-1, were loaded into the nanoparticles for gene therapy. 7ND transfection inhibited plaque destabilization and rupture, although it did not decrease the size of the plaque. In another study, simvastatin was encapsulated in reconstituted HDL nanoparticles, which enabled more specific delivery to atherosclerotic plaques (Fig. 3).136 The simvastatin-loaded HDL nanoparticles were used to treat ApoE knockout mice and showed accumulation in lesion sites, which led to progress inhibition of plaques at low dosages and decreased inflammation at higher dosages. In a follow-up work, it was shown that inhibition of macrophage proliferation using this formulation was a major contributor to the reduced inflammation observed in the atherosclerotic plaques.137
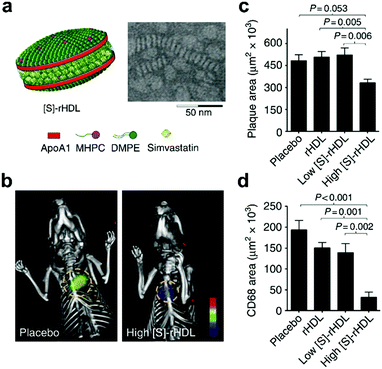 |
| Fig. 3 Nanoparticle-based therapeutic for CVD. (a) Schematic representation of a reconstituted high-density lipoprotein (HDL) nanoparticle loaded with simvastatin ([S]-rHDL), along with an accompanying transmission electron micrograph. (b) Fluorescence molecular tomography/CT imaging of protease activity demonstrated that atherosclerotic mice treated with a high dose of [S]-rHDL had significantly reduced inflammation levels. (c and d) Quantification of mean plaque area (c) and plaque macrophage content (d) demonstrated the benefits of treatment with [S]-rHDL. Adapted with permission.136 Copyright 2014, Springer Nature. | |
Because macrophages play a major role in the inflammatory response during atherogenesis,138 the cells represent an attractive target for atherosclerosis treatments. In order to lower macrophage levels in atherosclerotic lesions, the synthetic liver X receptor agonist GW3965 has been used.139 Liver X receptors are responsible for reverse cholesterol transport and their activation can also reduce the expression of proinflammatory genes. GW3965 was incorporated into collagen IV-targeting nanoparticles for atherosclerotic plaque homing. In vitro studies showed that the nanoparticles effectively upregulated liver X receptor target genes and downregulated proinflammatory mediators in macrophages. In vivo study in a mouse model of atherosclerosis showed that the nanoparticles could be successfully delivered to lesion sites, resulting in the reduction of macrophage levels without increasing hepatic lipid biosynthesis or hyperlipidemia. Macrophages can also be targeted using sugar-based amphiphilic macromolecules, which can be designed to stop macrophages from ingesting oxidized lipids by blocking the scavenger receptors on their surfaces.140 Nanoparticles with narrow size distributions were prepared with amphiphilic macromolecule shells and mucic acid-based cores by a flash nanoprecipitation method. When the lead formulation was injected intravenously into ApoE knockout mice, the nanoparticles accumulated in lipid-rich lesions and were able to reduce neointimal hyperplasia, lipid burden, and cholesterol clefts. Polarities of monocytes and macrophages can be shifted towards less inflammatory phenotypes if induced by peroxisome proliferator-activated receptor-γ.141 To utilize this trait, pioglitazone, an agonist of the receptor, was used to modulate the phenotypes of the relevant cells around atherosclerotic plaques in order to inhibit plaque ruptures.142 The molecule was loaded into PLGA nanoparticles, which were then intravenously injected into angiotensin II-infused ApoE knockout mice fed a high-fat diet. The pioglitazone-loaded nanoparticles not only regulated inflammatory cytokine expression, but also downregulated the production of extracellular matrix metalloproteinase inducer, inhibiting macrophage activation and ultimately decreasing atherosclerotic plaque rupture. Another factor that activates macrophages in atherosclerotic plaques is CD40 signaling mediated by CD4+ T lymphocytes.143 Blocking the interaction between CD40 and tumor necrosis factor receptor-associated factor 6 can reduce inflammatory responses in atherosclerosis. A lipophilic small-molecule SMI 6877002, which performs this inhibitory action, was encapsulated in reconstituted HDL, and the anti-inflammatory effect of the resulting formulation was evaluated by PET imaging in ApoE knockout mice and in nonhuman primates.144 It was shown that the nanoformulation successfully reduced inflammation in atherosclerotic plaques by hindering monocyte migration.
3.3.2 Clot dissolution.
In the case of acute thrombotic events that result in ischemia, tissue plasminogen activator (tPA) is often used for clot dissolution.61 However, without any targeting strategies, this treatment is susceptible to off-target drug action that can cause risk of hemorrhaging. Overall, there has been increasing interest in the targeted delivery of thrombolytic agents using nanoparticles, which can lead to the development of safer and less invasive treatments.145 Along these lines, thrombus targeting functionality has been added to polysaccharide-poly(isobutylcyanoacrylate) nanoparticles by attaching them with fucoidan, which shows high affinity towards the P-selectin expressed on activated platelets.146 The nanoparticles were loaded with tPA and delivered to the site of a thrombus by targeting the resident activated platelets. C57BL/6 mice with FeCl3-induced thrombosis were treated with the nanoparticles, and the status of the thrombus was monitored using fluorescence imaging. In the end, specific accumulation of the tPA-loaded nanoparticles was confirmed, as well as the thrombus-reducing ability of the formulation. Streptokinase, another thrombolytic drug, has been encapsulated in nanovesicles that allow protection against its off-target effects while in circulation.147 The nanovesicles specifically targeted thrombi by binding to integrin GPIIb–IIIa and P-selectin found on activated platelets. After binding, the vesicles were destabilized by phospholipase-A2 secreted from the clot, and the streptokinase was effectively released. In a murine carotid artery thrombosis model, the nanoparticle was able to target the thrombus without causing off-target hemorrhagic side effects. Apart from using thrombolytic drugs, other types of strategies have been explored for addressing thrombosis. For example, PLGA nanocarriers incorporated with small Fe3O4 nanoparticles and perfluorohexane were functionalized with the CREKA peptide for noninvasive thrombus imaging and physical thrombolysis.148 The Fe3O4 payload allowed for imaging using MRI or photoacoustic imaging, the perfluorohexane exhibited thrombolytic activity through a liquid-to-gas phase transition when triggered by ultrasound, and CREKA acted as a targeting moiety that binds specifically to fibrin.
3.3.3 Stent materials.
Nanoparticles have also been utilized in larger devices used in CVD treatment such as stents, which are employed to support or recanalize arteries narrowed by disease.149 Nanoparticle technology can be used to further improve upon current platforms by providing desirable features, such as enhanced biocompatibility and controlled drug elution. Sirolimus-loaded polylactide nanoparticles have been used for this purpose, and stents coated with the particles were proven to be effective at inhibiting proliferation of smooth muscle cells while promoting proliferation of endothelial cells in vitro.150 Inhibiting overgrowth of smooth muscle cells can help to prevent in-stent restenosis, and faster establishment of endothelial cells can decrease the risk of in-stent thrombosis. In order to closely monitor stents and the potential inflammation caused by them long-term, smart stents have been designed.151 A multifunctional bioresorbable electronic stent was shown to be capable of flow sensing, temperature monitoring, data storage, wireless power/data transmission, inflammation suppression, localized drug delivery, and hyperthermia therapy. Various nanomaterials, including ceria nanoparticles for catalytic ROS scavenging and mesoporous silica-coated gold nanorods for photothermal therapy, were integrated for their therapeutic effects, which were demonstrated under in vivo, ex vivo, and in vitro settings.
4. Biomimetic nanotechnologies for CVD
4.1 Introduction
Through the enhancement of bioavailability, better targeting, prolonged release, and other unique properties, nanoparticle technology has the potential to significantly improve CVD diagnosis and treatment. However, there are some challenges inherently associated with the use of purely synthetic platforms that may ultimately hinder their utility. For example, despite efforts to hide nanoparticles from immune detection, it has been demonstrated that antibodies directed against these nanocarriers can still be generated, which can greatly affect in vivo performance over multiple administrations.152,153 And while single ligand targeting approaches can be highly effective, they oftentimes do not accurately recapitulate the complex, multimodal interactions that naturally occur within the body.154 The identification of these ligands can also involve an extensive discovery phase that requires significant time and effort.155 It should be noted that achieving stoichiometric control of surface functionalization inherently becomes more difficult as an increasing number of unique ligands are introduced. To address these concerns, there has been a noticeable shift towards the use of biomimetic design principles when developing nanoformulations.40,156 In short, this type of approach takes inspiration from nature, whether it be from a functional perspective or through the direct use of naturally occurring biological material. By leveraging fine-tuned functions and designs that have been honed over the course of evolution, researchers are able to bypass some of the limitations facing traditional nanoparticle platforms and can identify novel ways to approach critical challenges in the field of nanomedicine.
Biomimetic nanotechnology can take on many different forms, but a significant portion of it centers around the mimicry of cells, which are one of the fundamental units of biology.157,158 The advantage of doing such is evident when one considers that many of the properties, including long circulation and specific targeting, that are highly desirable for nanoparticle platforms are inherent to certain types of living cells. One approach is physical mimicry, such as when nanodelivery vehicles have been designed to mimic the shape or stiffness characteristics of red blood cells (RBCs) to improve blood residence.159 More often, researchers take advantage of cell-derived ligands that perform specific functions, whether it be for targeting or signal transduction.156 These include proteins, lipids, and carbohydrates, as well as their derivatives, that are generally found on the membrane surface of cells. This approach bypasses the need to identify novel ligands for achieving functions that already exist in nature. It should be noted that some widely used ligands in traditional nanomedicine, including the RGD peptide motif,160 can be found in natural systems, further supporting the utility of biomimicry. Naturally occurring biomolecules have also been used as a means of decreasing nonspecific interactions. One example is glycolipids, which can be used to functionalize nanoparticle surfaces in a manner similar to PEGylation.161
A more recently developed biomimetic functionalization method directly uses cell membrane as a coating material around synthetic nanoparticulate cores.162 The result is a cell membrane-coated nanoparticle that has unique functionalities derived from the source cell. Cell membrane coating is a purely function-driven approach for nanoparticle modification, and design choices are made by identifying the desirable properties exhibited by specific cell populations found within the body. Unlike traditional surface coatings that work by hiding nanoparticles from the immune system, the cell membrane coating can act as a camouflaging layer that actively suppresses nonspecific clearance.163,164 The result is a nanoparticle that can circulate for extended periods of time within the bloodstream, making it highly useful for certain drug delivery applications.165,166 Several methods have also been developed to introduce additional functionality onto the surface of these membrane-coated nanoparticles in a nondestructive manner, including a lipid-insertion technique that anchors ligands using a polymer tether.167,168
While RBC membrane-coated nanoparticles (RBC-NPs) are well-suited for applications that require long circulation and RBC-specific interactions,169–172 nanoparticles derived from other cell types excel in different areas.173–176 One key advantage of membrane coating nanotechnology is that it provides a facile means of introducing multimodal functionality that would be difficult to replicate using traditional synthetic techniques.177,178 The characteristic of biomimetic nanoparticles that is perhaps the most relevant to CVD diagnostics and treatment is their biointerfacing capability.41,179 The natural interactions between cells and various disease substrates can be leveraged to create targeted nanoformulations that better localize to disease sites. For example, cancer cell membrane-coated nanoparticles have been demonstrated to homotypically bind with tumors of the same cellular origin.180–182 A number of other cell types, including white blood cells, platelets, and stem cells, have all been used to source membrane with tumor-targeting specificities.183–185 In many types of CVD, platelets represent an important cell type that is heavily implicated in disease pathogenesis.186 Platelets are responsible for hemostasis, and their aggregation at sites of injury can greatly exacerbate disease development or lead to acute traumatic events. They are also important modulators of immunity, propagating inflammatory responses that are a hallmark atherosclerosis.187 It should therefore come as no surprise that platelet mimicry has been a widely utilized strategy in the design of nanoformulations for CVD. In this section, we will discuss the development and applications of these and other biomimetic platforms (Table 1).
Table 1 Biomimetic approaches for the design of CVD nanomedicine
Design approach |
Description |
Representative example(s) |
Single-ligand modification |
Nanoparticles modified with biomimetic functionality through the inclusion of naturally occurring biomolecules. |
• PLGA nanoparticle with an HDL shell.188 |
• Sialyl Lewis X-conjugated polymersome.189 |
• Glycocalicin-conjugated PLGA nanoparticle.206 |
|
Natural carrier |
Naturally existing nanostructures or their derivatives. |
• Tobacco mosaic virus.202 |
|
|
• RBC membrane vesicle.191 |
|
Synthetic/natural hybrid |
Naturally occurring nanomaterials are combined with synthetic materials into a single hybrid nanostructure. |
• Platelet membrane-coated nanoparticle.179,211–213 |
|
|
• RBC membrane-coated nanoparticle.191,216 |
|
|
• Leukocyte-like liposome.190,208 |
|
Modified live cell |
Live cells modified with natural nanomaterials to provide enhanced functionality. |
• Stem cell modified with platelet-derived nanovesicles.209 |
|
Stress-sensitivity |
Nanoparticles designed to respond to physical cues such as shear stress. |
• PLGA nanoparticle microaggregate.199,200 |
|
|
• Nanoparticle-adsorbed carrier RBC.201 |
4.2 Diagnostics
Biomimetic diagnostic tools can facilitate identification of existing or impending inflammation due to CVD, as well as direct reperfusion efforts in response to a thrombotic event. Earlier biomimetic diagnostic tools focused on attaching naturally occurring targeting moieties onto nanoparticle platforms. For example, PLGA nanoparticle cores with a shell made up of HDL have been fabricated.188 Since HDL is known to target macrophages in atherosclerotic lesions, the nanoparticles were able to accumulate at the disease site and deliver a fluorescent dye payload for visualization. Furthermore, due to the biocompatible nature of both HDL and PLGA, the formulation demonstrated no cellular cytotoxicity, highlighting its safety. Another natural targeting moiety that has been utilized for nanoparticle-based detection of CVD-related inflammation is sialyl Lewis X, a target of P-selectin, which was conjugated to polymersomes along with an antibody targeted against intercellular adhesion molecule 1 (ICAM-1).189 Polymersomes, made up of block copolymers, are analogs of liposomes but benefit from thicker membranes and can better maintain structural integrity during ligand conjugation. Various immune cells, including leukocytes, leverage interactions with P-selectin and ICAM-1 to localize to sites of inflammation. It was demonstrated that the dual-functionalized nanoformulation exhibited cell-like properties that enabled preferential accumulation to inflamed tissue for delivery of a diagnostic imaging payload.
As the field has developed, more complex and multifunctional biomimetic platforms have taken advantage of cell membrane mimicry in order to improve circulation time and homing capabilities. In one example, rhodamine-labeled lipid films were rehydrated with mouse macrophage proteins capable of targeting activated endothelia through CD11a and CD18, which both bind to ICAM-1.190 These hybrid liposomal nanostructures demonstrated a 4.4-fold increase in accumulation at the target site as compared to bare liposomes. When the rhodamine on the lipid surface was replaced with a Gd-labeled lipid, the platform showed promise for use in MRI applications. In another example, RBC membranes were loaded with indocyanine green, a near-infrared imaging agent, as well as tPA for dissolving clots through the conversion of plasminogen to plasmin.191 Here, the cell membrane acted to prolong the circulation of the formulation through immunomodulatory markers on the surface, while the tPA targeted clots via favorable fibrin binding. In vitro blood clot localization studies demonstrated significantly higher accumulation of the tPA-conjugated formulation when compared to unconjugated formulations, suggesting future utility of the platform for ischemic stroke applications.
Another common biomimetic strategy used in disease detection is platelet membrane mimicry. Platelet-mimicking nanoparticles have already shown promise in other related fields, including cancer detection. For example, platelet membrane-coated magnetic nanoparticles have been fabricated that can preferentially accumulate in tumor tissue due to their ability to adhere to disrupted vasculature.192 The particles provided ample contrast during MRI, demonstrating potential for noninvasive and nontoxic tumor imaging. In another example, gold nanoparticles were biosynthesized in situ inside of living platelets.193 The final size of the nanoparticles was approximately 5 nm, and they were distributed within the cytoplasm of the cells. Due to the X-ray attenuating properties of the gold, the platform could have utility for use as a CT contrast agent. With its natural targeting functionality and excellent biocompatibility, platelet membrane can potentially be employed for the modification of a wide range of imaging modalities that have already been used with other types of cell membrane coatings.162
In the case of CVD imaging, nanoscale platelet mimics have been used to rapidly home payloads to thrombus sites. In an example, nanobubbles were fabricated using DiO-incorporated platelet membrane ghosts sonicated with sulfur hexafluoride gas (Fig. 4).194 Using multimodal optical fluorescence imaging, the membrane-functionalized nanobubbles showed significant accumulation at the site of brain ischemia within 10 minutes of injection, providing fast and accurate detection. In addition, accumulation after 40 minutes provided enough acoustic scattering for detection via ultrasound imaging. Besides responding to a thrombotic event, such as a heart attack or stroke, platelet mimicry has also been leveraged for the early detection of atherosclerosis. Platelet membrane-coated nanoparticles (PNPs) were fabricated with PLGA as the core material, which allowed the targeted delivery of various diagnostic payloads to atherosclerotic lesions.195 After demonstrating significant in vitro binding to foam cells, collagen, and activated endothelium, in vivo studies verified the accumulation of the nanoparticles to arterial plaque in regions staining positive for type IV collagen, ICAM-1, and CD68. The natural targeting property of the formulation enabled binding to not only preexisting plaque within the body, but also to regions susceptible to future plaque formation. When introducing a Gd-based payload into the particles for live MRI imaging, significant contrast was generated in the aortic arch of a mouse with atherosclerosis.
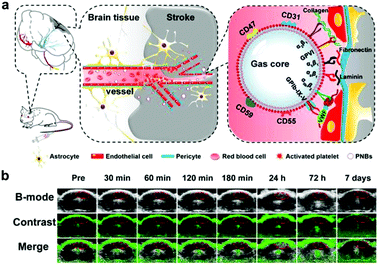 |
| Fig. 4 Biomimetic nanoparticle-based diagnostic for CVD. (a) Platelet-functionalized nanobubbles (PNBs) can be used to target stroke lesions via a number of natural biological interactions for imaging purposes. (b) Real-time contrast-enhanced ultrasound imaging in mice administered with the PNB formulation could be used to identify the region of a stroke. Adapted with permission.194 Copyright 2018, Ivyspring International Publisher. | |
4.3 Therapeutics
4.3.1 Fluid mechanics-enabled therapeutics.
A major underlying driver of many CVD is the buildup of atherosclerotic plaque in the vasculature. At these narrowed blood vessels, platelets can be activated locally due to high shear stress.196–198 This natural phenomenon has inspired researchers to engineer biomimetic nanotherapeutics that are capable of responding to high fluidic shear stress at obstructed blood vessels.199,200 A shear-activated nanotherapeutic was fabricated by spray-drying a solution of PLGA nanoparticles to produce microaggregates. Under normal physiological flow, the complexes were shown to be fairly stable, but when exposed to high shear stress, the microaggregates broke apart into individual nanoparticulates. The smaller nanoparticles experience a lower drag force that enables them to remain adhered to the blood vessel at the site of dispersion. Leveraging this property, it was demonstrated that the shear-activated formulation could preferentially target blood clots and sites of plaque buildup for drug delivery. When tested in an arterial thrombus mouse model, a tPA-conjugated formulation significantly delayed thrombosis. In a mouse pulmonary embolism model, the nanoformulation was able to rescue 85% of treated mice, whereas all of the control mice died within 45 minutes.
In a similar context, nanoparticles have been adsorbed onto carrier RBCs to extend drug circulation and for stimuli-responsive release.201 The adsorbed nanoparticles were also released through a high shear stress mechanism at the site of a thrombus. While the flexibility of the RBCs enabled them to stably migrate through narrow openings, the drug-loaded nanoparticles were desorbed and released during the associated deformations. Other biomimetic platforms that leverage the unique fluid mechanics at narrowed vessels have also been reported. Tobacco mosaic viruses have been shown to exhibit preferential thrombus homing abilities due to their unique elongated shape.202 Their rod-like structure experiences a lateral torque in linear laminar flow that favors accumulation at narrow vessel walls through hydrodynamic migration.203 This effect has been used to facilitate delivery of streptokinase204 and tPA205 for thrombolytic therapies.
4.3.2 Platelet mimetics.
Given the pivotal role of platelets in CVD and their natural interactions with the associated disease substrates, many researchers have attempted to mimic platelet binding mechanisms for targeted drug delivery. In one example, PLGA nanoparticles were conjugated via carbodiimide chemistry with glycocalicin, the external fraction of platelet GPIbα.206 The biomimetic platform was able to interact with damaged endothelial cells through P-selectin and the subendothelium through von Willebrand factor (vWF). Conjugated nanoparticles adhered significantly better on P-selection and vWF-coated surfaces when subjected to shear stress for 30 minutes when compared to unconjugated control particles. This effect was almost completely abrogated when the binding sites of the conjugated nanoparticles were blocked with anti-GPIb antibodies. Improving on single-ligand targeted platforms, multimodal platelet-like nanoparticles have also been reported.207 Through functionalizing a flexible nanoparticle with multiple binding peptides, it was shown that the biophysical structure and the biochemical interactions of natural platelets could be replicated. Along the same lines of multivalent functionality, entire fractions of leukocyte proteins have been purified and incorporated into liposomes for targeted delivery to inflamed tissues208 and for theranostic applications.190
Interestingly, live stem cells have been bestowed with platelet-like targeting capabilities through the modification of their cell membrane with platelet-derived nanovesicles.209 To accomplish this, platelet vesicles were first derived through a process involving freeze–thaw lysis and sonication. They were then fused onto the surface of cardiac stem cells in the presence of PEG, which enabled the modified cells to display the myocardial infarction homing ability of platelets due to the presence of the GPIbα glycoprotein. In a rat ischemia/reperfusion model, the platelet vesicle-modified stem cells were able to retain much better in the heart. The higher retention directly translated into increased cardiomyocyte growth, improved blood flow, and increased vessel density at the injury site. Similar results were obtained in a more clinically relevant porcine model of acute injury.
4.3.3 Platelet membrane-coated nanoparticles.
Another type of platelet-mimetic system utilizes the entire plasma membrane of platelets as a coating material for synthetic nanoparticulate cores.179,210 With the plethora of functional proteins present on platelet membrane, these PNPs possess many platelet-like properties, including enhanced immunocompatibility and the ability to bind to sites of cardiovascular damage (Fig. 5). To fabricate these biomimetic nanoparticles, platelet membrane was derived from whole platelets through a repeated freeze–thaw process and then subsequently fused onto polymeric nanoparticle cores through sonication. When the resulting PNPs were examined for the presence of 15 different immunomodulatory and adhesion proteins through Western blot analysis, all were found to be present on the nanoparticles. In rats with angioplasty-induced arterial denudation, the nanoparticles preferentially bound to the damaged artery as compared to a control artery. Docetaxel-loaded PNPs were used as treatment in a rat coronary restenosis model, and the formulation was able to significantly inhibit neointima growth. Treated mice had arterial cross-sections that displayed parameters similar to those from uninjured rats, whereas all of the other control formulations had minimal effects in preventing cell proliferation.
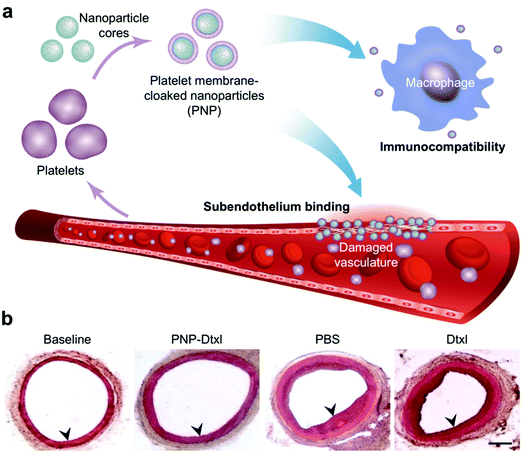 |
| Fig. 5 Biomimetic nanoparticle-based therapeutic for CVD. (a) Platelet membrane-coated nanoparticles (PNPs) take on many of the properties of whole platelets, including their immunocompatibility and ability to bind to damaged vasculature. (b) When used to treat rats in a coronary restenosis model, PNPs loaded with docetaxel (Dtxl) had a profound impact on the neointimal growth within the affected vessels. Adapted with permission.179 Copyright 2015, Springer Nature. | |
Building on the PNP concept, other researchers have used platelet membrane to coat urokinase plasminogen activator-loaded gold nanorods for pulmonary thrombus treatment,211 endothelium-protective epigenetic inhibitor-loaded dendritic nanoclusters for anti-restenosis therapy,212 and rapamycin-loaded PLGA nanoparticles for halting atherosclerotic plaque progression.213 Additionally, platelet membrane has been used in the fabrication bio-nanobubbles with a sulfur hexafluoride gaseous core for recanalization after acute ischemic stroke.194 Apart from their native platelet-derived functionality, PNPs can be further improved with other targeting ligands. In one example, prostaglandin E2-conjugated platelet membrane was used to functionalize PLGA cores loaded with stem cell secretions for targeted heart repair.214 The formulation enabled dual targeting, whereby the platelet membrane provided affinity towards sites of vasculature injury and the prostaglandin E2 enabled localization to injured cardiomyocytes. Interestingly, researchers have also leveraged the natural binding affinity of PNPs in order to eradicate blood clots while treating multiple myeloma.215 Because treatment of multiple myeloma with immunomodulatory drugs can cause thrombus complications, a PNP co-encapsulated with bortezomib, a proteasome inhibitor, and tPA was developed to eradicate tumors while simultaneously promoting clot dissolution.
4.3.4 Other cell membrane-coated nanoparticles.
Membrane from other source cells have been derived and used to coat nanoparticles for the development of CVD therapeutics. With their long circulation time and role in oxygen delivery, RBC-NPs for ischemic stroke treatment216 and for theranostic applications191 have been reported. In the former example, a dextran polymeric core was conjugated with boronic ester to create an ROS-bioresponsive particle. The nanoparticle was further functionalized with RBC membrane to prolong circulation. Finally, SHp, a stroke-homing peptide, was inserted onto the membrane surface to enable active targeting. The SHp-functionalized RBC-NP formulation was capable of targeting ischemic neuron cells in brain tissue for the delivery of a neuroprotective agent. When tested in vivo, the targeted RBC-NPs showed a marked increase in accumulation within ischemic rat brains. Using a middle cerebral artery occlusion rat model, animals treated with the nanoformulation had significantly better outcomes as indicated by a smaller infarct size and lower neurological scores. Besides RBC-NPs, leukocyte membrane-coated nanoparticles have demonstrated the ability to preferentially target inflamed endothelium,217 and treatment of mice with severe hindlimb ischemia using vascular endothelial growth factor-loaded, CXCR4-engineered stem cell membrane-coated nanoparticles has been shown to significantly improve blood reperfusion, muscle restoration, and limb salvage.218 Novel hybrid membrane-coated nanoparticles that combine the functionalities from multiple source cells into a single nanocarrier have also been applied for atherosclerosis targeting,219 and further exploration along these lines could yield platforms with interesting properties and enhanced utility for CVD applications.
5. Conclusion and outlook
As modern medicine has prolonged human life expectancy, diseases that correlate with longevity like CVD have risen to the forefront. As it currently represents the number one cause of death worldwide, significant efforts have been placed on finding ways to more effectively manage CVD in the clinic. Along these lines, nanoparticle technology, which has proven to be useful in other areas of medicine, is being actively applied towards the development of improved diagnostic and therapeutic modalities. The benefits of nanodelivery include the ability to better encapsulate functional payloads such as drugs and imaging agents, target these payloads to disease sites, and release them over time as necessary. More recently, the rational application of biomimetic principles in the design process for new nanoformulations has become increasingly commonplace. The resulting platforms have proven to be highly functional, are oftentimes easy to develop and fabricate, and have unique features that make them well-suited for CVD applications. Among these technologies, cell membrane coating has emerged an effective means of introducing biomimetic functionality onto synthetic nanoparticles. Cell membrane-coated nanoparticles can inherently participate in multivalent interactions with their surroundings, making them adept at navigating the complex biological environments that exist in vivo.
Looking forward, there are many opportunities for future development along the lines of biomimetic nanotechnology for CVD. As we better understand the various phenotypic changes associated with disease pathogenesis, it will be possible to generate nanodelivery vehicles that can target specific disease stages. Environmental sensitivity can also be engineered into these platforms such that payload release is triggered by disease-associated markers.220 In the case of cell membrane-coated nanoparticles, additional cell types that are relevant to CVD pathogenesis can be used to source the membrane coating. This can be combined with novel nanoparticulate cores in order to generate unique formulations that are custom-tailored for specific applications. Newly discovered bioactive compounds or diagnostic markers can be combined with biomimetic nanotechnology to increase their utility. For example, miRNA-based therapies have shown the potential to reverse atherosclerosis and could greatly benefit from the protection afforded by nanoencapsulation.221 With better targeting specificity, synergistic treatments that work by targeting different cell subsets can be explored. Biomimetic functionalization will also help to improve the performance of novel nanoimaging agents that are being developed by prolonging circulation or improving localization to disease sites. Regarding clinical translation, there are some significant hurdles that will need to be overcome, including achieving large-scale production under good manufacturing practice standards. Because many of these emerging technologies combine both synthetic and cell-derived components, new translational avenues will need to be established in cooperation with regulatory agencies. Overall, the future prospects for biomimetic platforms for the management of CVD are bright, and continued development along these lines will undoubtedly yield new and exciting solutions for addressing this major global health threat.
Conflicts of interest
There are no conflicts to declare.
Acknowledgements
This work is supported by the National Institutes of Health under Award Number R01CA200574.
References
- G. A. Mensah and D. W. Brown, Health Aff., 2007, 26, 38–48 CrossRef PubMed.
- R. Ross, N. Engl. J. Med., 1986, 314, 488–500 CrossRef CAS PubMed.
- D. G. Hackam and S. S. Anand, JAMA, J. Am. Med. Assoc., 2003, 290, 932–940 CrossRef.
- R. A. Vogel, Clin. Cardiol., 1997, 20, 426–432 CrossRef CAS.
- D. H. O'Leary, J. F. Polak, R. A. Kronmal, T. A. Manolio, G. L. Burke, S. K. Wolfson and C. H. S. C. R. Grp, N. Engl. J. Med., 1999, 340, 14–22 CrossRef.
- P. Amarenco, A. Cohen, C. Tzourio, B. Bertrand, M. Hommel, G. Besson, C. Chauvel, P. J. Touboul and M. G. Bousser, N. Engl. J. Med., 1994, 331, 1474–1479 CrossRef CAS PubMed.
- G. De Luca, H. Suryapranata, J. P. Ottervanger and E. M. Antman, Circulation, 2004, 109, 1223–1225 CrossRef PubMed.
- F. L. Silver, J. W. Norris, A. J. Lewis and V. C. Hachinski, Stroke, 1984, 15, 492–496 CrossRef CAS PubMed.
- C. J. Murray and A. D. Lopez, N. Engl. J. Med., 2013, 369, 448–457 CrossRef CAS PubMed.
- E. J. Benjamin, M. J. Blaha, S. E. Chiuve, M. Cushman, S. R. Das, R. Deo, S. D. de Ferranti, J. Floyd, M. Fornage, C. Gillespie, C. R. Isasi, M. C. Jimenez, L. C. Jordan, S. E. Judd, D. Lackland, J. H. Lichtman, L. Lisabeth, S. Liu, C. T. Longenecker, R. H. Mackey, K. Matsushita, D. Mozaffarian, M. E. Mussolino, K. Nasir, R. W. Neumar, L. Palaniappan, D. K. Pandey, R. R. Thiagarajan, M. J. Reeves, M. Ritchey, C. J. Rodriguez, G. A. Roth, W. D. Rosamond, C. Sasson, A. Towfighi, C. W. Tsao, M. B. Turner, S. S. Virani, J. H. Voeks, J. Z. Willey, J. T. Wilkins, J. H. Wu, H. M. Alger, S. S. Wong, P. Muntner, C. American Heart Association Statistics and S. Stroke Statistics, Circulation, 2017, 135, e146–e603 CrossRef.
- G. A. Roth, M. H. Forouzanfar, A. E. Moran, R. Barber, G. Nguyen, V. L. Feigin, M. Naghavi, G. A. Mensah and C. J. Murray, N. Engl. J. Med., 2015, 372, 1333–1341 CrossRef CAS.
- J. Stewart, G. Manmathan and P. Wilkinson, JRSM Cardiovasc. Dis., 2017, 6, 2048004016687211 Search PubMed.
- T. A. Pearson, S. N. Blair, S. R. Daniels, R. H. Eckel, J. M. Fair, S. P. Fortmann, B. A. Franklin, L. B. Goldstein, P. Greenland, S. M. Grundy, Y. Hong, N. H. Miller, R. M. Lauer, I. S. Ockene, R. L. Sacco, J. F. Sallis, Jr., S. C. Smith, Jr., N. J. Stone and K. A. Taubert, Circulation, 2002, 106, 388–391 CrossRef.
- E. Archer and S. N. Blair, Prog. Cardiovasc. Dis., 2011, 53, 387–396 CrossRef.
- J. Sanz and Z. A. Fayad, Nature, 2008, 451, 953–957 CrossRef CAS PubMed.
- D. S. Celermajer, C. K. Chow, E. Marijon, N. M. Anstey and K. S. Woo, J. Am. Coll. Cardiol., 2012, 60, 1207–1216 CrossRef.
- R. F. Gillum, Bull. W. H. O., 1988, 66, 249–258 CAS.
- R. Mastouri, S. G. Sawada and J. Mahenthiran, Expert Rev. Cardiovasc. Ther., 2010, 8, 77–91 CrossRef.
- E. Escolar, G. Weigold, A. Fuisz and N. J. Weissman, Can. Med. Assoc. J., 2006, 174, 487–495 CrossRef.
- M. Tavakol, S. Ashraf and S. J. Brener, Glob. J. Health Sci., 2012, 4, 65–93 Search PubMed.
- M. A. Crowther, Hematology Am. Soc. Hematol. Educ. Program, 2005, 436–441 CrossRef.
- J. O. Gebbers, Ger. Med. Sci., 2007, 5, Doc04 Search PubMed.
- S. S. Anand and S. Yusuf, JAMA, J. Am. Med. Assoc., 1999, 282, 2058–2067 CrossRef CAS.
- D. Ettehad, C. A. Emdin, A. Kiran, S. G. Anderson, T. Callender, J. Emberson, J. Chalmers, A. Rodgers and K. Rahimi, Lancet, 2016, 387, 957–967 CrossRef.
- S. B. King, 3rd, J. J. Marshall and P. E. Tummala, Annu. Rev. Med., 2010, 61, 199–213 CrossRef.
- A. Bivard, L. Lin and M. W. Parsonsb, J. Stroke, 2013, 15, 90–98 CrossRef.
- E. D. Bizjak and V. F. Mauro, Ann. Pharmacother., 1998, 32, 769–784 CrossRef CAS.
- A. Ciccone, L. Valvassori, M. Nichelatti, A. Sgoifo, M. Ponzio, R. Sterzi, E. Boccardi and S. E. Investigators, N. Engl. J. Med., 2013, 368, 904–913 CrossRef CAS.
- A. Z. Wang, R. Langer and O. C. Farokhzad, Annu. Rev. Med., 2012, 63, 185–198 CrossRef CAS.
- O. C. Farokhzad and R. Langer, ACS Nano, 2009, 3, 16–20 CrossRef CAS.
- J. Shi, P. W. Kantoff, R. Wooster and O. C. Farokhzad, Nat. Rev. Cancer, 2017, 17, 20–37 CrossRef CAS.
- K. Hosoyama, M. Ahumada, K. Goel, M. Ruel, E. J. Suuronen and E. I. Alarcon, Biotechnol. Adv., 2019, 37, 444–458 CrossRef CAS PubMed.
- L. Zhang, F. X. Gu, J. M. Chan, A. Z. Wang, R. S. Langer and O. C. Farokhzad, Clin. Pharmacol. Ther., 2008, 83, 761–769 CrossRef CAS PubMed.
- L. Brannon-Peppas and J. O. Blanchette, Adv. Drug Delivery Rev., 2004, 56, 1649–1659 CrossRef CAS PubMed.
- R. Singh and J. W. Lillard, Jr., Exp. Mol. Pathol., 2009, 86, 215–223 CrossRef CAS PubMed.
- J. D. Byrne, T. Betancourt and L. Brannon-Peppas, Adv. Drug Delivery Rev., 2008, 60, 1615–1626 CrossRef CAS.
- V. M. Martin Gimenez, D. E. Kassuha and W. Manucha, Ther. Adv. Cardiovasc. Dis., 2017, 11, 133–142 CrossRef CAS.
- P. Gupta, E. Garcia, A. Sarkar, S. Kapoor, K. Rafiq, H. S. Chand and R. D. Jayant, Cardiovasc. Hematol. Disord.: Drug Targets, 2019, 19, 33–44 CrossRef CAS PubMed.
- R. H. Fang, C. M. J. Hu and L. F. Zhang, Expert Opin. Biol. Ther., 2012, 12, 385–389 CrossRef CAS PubMed.
- J. W. Yoo, D. J. Irvine, D. E. Discher and S. Mitragotri, Nat. Rev. Drug Discovery, 2011, 10, 521–535 CrossRef CAS.
- A. V. Kroll, R. H. Fang and L. Zhang, Bioconjugate Chem., 2017, 28, 23–32 CrossRef CAS.
- R. Dhingra and R. S. Vasan, Trends Cardiovasc. Med., 2017, 27, 123–133 CrossRef CAS.
- R. Lopez-Mejias, F. Genre, C. Gonzalez-Juanatey and M. A. Gonzalez-Gay, Vasa, 2014, 43, 83–85 CrossRef.
- A. Qureshi, Y. Gurbuz and J. H. Niazi, Sens. Actuators, B, 2012, 171, 62–76 CrossRef.
- R. J. Martis, U. R. Acharya and H. Adeli, Comput. Biol. Med., 2014, 48, 133–149 CrossRef.
- L. C. Kovell, M. T. Ali, A. G. Hays, T. S. Metkus, J. A. Madrazo, M. C. Corretti, S. A. Mayer, T. P. Abraham, E. P. Shapiro and M. Mukherjee, Am. J. Cardiol., 2018, 122, 1443–1450 CrossRef.
- J. P. Greenwood, B. A. Herzog, J. M. Brown, C. C. Everett, J. Nixon, P. Bijsterveld, N. Maredia, M. Motwani, C. J. Dickinson, S. G. Ball and S. Plein, Ann. Intern. Med., 2016, 165, 1–9 CrossRef.
- B. Riemann, M. Schafers, M. P. Law, T. Wichter and O. Schober, Nuklearmedizin, 2003, 42, 4–9 CrossRef CAS.
- V. Gorenoi, M. P. Schonermark and A. Hagen, GMS Health Technol. Assess., 2012, 8, Doc02 Search PubMed.
- J. H. Rudd, E. A. Warburton, T. D. Fryer, H. A. Jones, J. C. Clark, N. Antoun, P. Johnstrom, A. P. Davenport, P. J. Kirkpatrick, B. N. Arch, J. D. Pickard and P. L. Weissberg, Circulation, 2002, 105, 2708–2711 CrossRef CAS.
- L. Marti-Bonmati, R. Sopena, P. Bartumeus and P. Sopena, Contrast Media Mol. Imaging, 2010, 5, 180–189 CrossRef CAS.
- M. Saeed, T. A. Van, R. Krug, S. W. Hetts and M. W. Wilson, Cardiovasc. Diagn. Ther., 2015, 5, 290–310 Search PubMed.
- R. Kalin and M. S. Stanton, Pacing Clin. Electrophysiol., 2005, 28, 326–328 CrossRef PubMed.
- J. R. Lindner, Nat. Rev. Cardiol., 2009, 6, 475–481 CrossRef CAS PubMed.
- E. A. Osborn and F. A. Jaffer, JACC Cardiovasc. Imaging, 2013, 6, 1327–1341 CrossRef PubMed.
- L. J. Lesko, Clin. Pharmacol. Ther., 2016, 100, 126–128 CrossRef CAS.
- M. R. Patel, K. W. Mahaffey, J. Garg, G. Pan, D. E. Singer, W. Hacke, G. Breithardt, J. L. Halperin, G. J. Hankey, J. P. Piccini, R. C. Becker, C. C. Nessel, J. F. Paolini, S. D. Berkowitz, K. A. Fox, R. M. Califf and R. A. Investigators, N. Engl. J. Med., 2011, 365, 883–891 CrossRef CAS.
- H. K. Nieuwenhuis, J. Albada, J. D. Banga and J. J. Sixma, Blood, 1991, 78, 2337–2343 CrossRef CAS.
- W. Mueck, A. W. Lensing, G. Agnelli, H. Decousus, P. Prandoni and F. Misselwitz, Clin. Pharmacokinet., 2011, 50, 675–686 CrossRef CAS PubMed.
- G. P. Ussia, M. Scarabelli, M. Mule, M. Barbanti, K. Sarkar, V. Cammalleri, S. Imme, P. Aruta, A. M. Pistritto, S. Gulino, W. Deste, D. Capodanno and C. Tamburino, Am. J. Cardiol., 2011, 108, 1772–1776 CrossRef CAS.
- I. M. El-Sherbiny, I. E. Elkholi and M. H. Yacoub, Glob. Cardiol. Sci. Pract., 2014, 336–349 Search PubMed.
- J. Nazari, R. Davison, K. Kaplan and D. Fintel, Med. Toxicol. Adverse Drug Exper., 1987, 2, 274–286 CAS.
- S. I. McFarlane, A. Kumar and J. R. Sowers, Am. J. Cardiol., 2003, 91, 30h–37h CrossRef CAS.
- S. E. Kjeldsen, J. Stalhammar, P. Hasvold, J. Bodegard, U. Olsson and D. Russell, J. Hum. Hypertens., 2010, 24, 263–273 CAS.
- S. E. Warren and G. S. Francis, Am. J. Med., 1978, 65, 53–62 CrossRef CAS.
- D. T. Majure, M. Hallaux, Y. Yeghiazarians and A. J. Boyle, J. Crit. Care, 2012, 27, 532.e9–532.e13 CrossRef CAS PubMed.
- T. I. Chang, G. Evans, A. K. Cheung, W. C. Cushman, M. J. Diamond, J. P. Dwyer, Y. Huan, D. Kitzman, J. B. Kostis, S. Oparil, A. Rastogi, C. L. Roumie, R. Sahay, R. S. Stafford, A. A. Taylor, J. T. Wright, Jr., G. M. Chertow and S. S. R. Group, Hypertension, 2016, 67, 550–555 CrossRef CAS PubMed.
- D. R. Abernethy and J. B. Schwartz, N. Engl. J. Med., 1999, 341, 1447–1457 CrossRef CAS.
- D. Kotecha, M. D. Flather, D. G. Altman, J. Holmes, G. Rosano, J. Wikstrand, M. Packer, A. J. S. Coats, L. Manzano, M. Bohm, D. J. van Veldhuisen, B. Andersson, H. Wedel, T. G. von Lueder, A. S. Rigby, A. Hjalmarson, J. Kjekshus, J. G. F. Cleland and G. Beta-Blockers in Heart Failure Collaborative, J. Am. Coll. Cardiol., 2017, 69, 2885–2896 CrossRef CAS.
- M. B. Leon, D. R. Rosing, R. O. Bonow and S. E. Epstein, Am. J. Cardiol., 1985, 55, 69B–80B CrossRef CAS.
- X. Y. Feng, W. M. Li and R. Y. Liu, Biomed. Res., 2017, 28, 7473–7479 CAS.
- A. Eisen, C. T. Ruff, E. Braunwald, R. A. Hamershock, B. S. Lewis, C. Hassager, T. F. Chao, J. Y. Le Heuzey, M. Mercuri, H. Rutman, E. M. Antman and R. P. Giugliano, J. Am. Heart Assoc., 2017, 6, e006035 Search PubMed.
- G. Schmitz and T. Langmann, Vasc. Pharmacol., 2006, 44, 75–89 CrossRef CAS.
- P. W. Serruys, M. J. Kutryk and A. T. Ong, N. Engl. J. Med., 2006, 354, 483–495 CrossRef CAS PubMed.
- A. Migliorini, G. Moschi, R. Vergara, G. Parodi, N. Carrabba and D. Antoniucci, Catheter. Cardiovasc. Interv., 2006, 67, 344–348 CrossRef PubMed.
- P. W. Serruys, M. C. Morice, A. P. Kappetein, A. Colombo, D. R. Holmes, M. J. Mack, E. Stahle, T. E. Feldman, M. van den Brand, E.
J. Bass, N. Van Dyck, K. Leadley, K. D. Dawkins, F. W. Mohr and S. Investigators, N. Engl. J. Med., 2009, 360, 961–972 CrossRef CAS.
- L. A. Iannone, S. M. Anderson and S. J. Phillips, Tex. Heart Inst. J., 1993, 20, 99–104 CAS.
- M. R. B. Evans, P. White, P. Cowley and D. J. Werring, Pract. Neurol., 2017, 17, 252–265 CrossRef.
- Y. Barenholz, J. Controlled Release, 2012, 160, 117–134 CrossRef CAS.
- M. R. Green, G. M. Manikhas, S. Orlov, B. Afanasyev, A. M. Makhson, P. Bhar and M. J. Hawkins, Ann. Oncol., 2006, 17, 1263–1268 CrossRef CAS.
- S. Tran, P. J. DeGiovanni, B. Piel and P. Rai, Clin. Transl. Med., 2017, 6, 44 CrossRef.
- J. A. Barreto, W. O'Malley, M. Kubeil, B. Graham, H. Stephan and L. Spiccia, Adv. Mater., 2011, 23, H18–H40 CrossRef CAS.
- J. Xie, S. Lee and X. Chen, Adv. Drug Delivery Rev., 2010, 62, 1064–1079 CrossRef CAS.
- T. L. Doane and C. Burda, Chem. Soc. Rev., 2012, 41, 2885–2911 RSC.
- H. Maeda, J. Wu, T. Sawa, Y. Matsumura and K. Hori, J. Controlled Release, 2000, 65, 271–284 CrossRef CAS.
- R. Mout, D. F. Moyano, S. Rana and V. M. Rotello, Chem. Soc. Rev., 2012, 41, 2539–2544 RSC.
- A. Kumari, R. Singla, A. Guliani and S. K. Yadav, EXCLI J., 2014, 13, 265–286 Search PubMed.
- R. H. Fang and L. F. Zhang, Sci. Signaling, 2014, 7, pe13 CrossRef.
- B. R. Smith and S. S. Gambhir, Chem. Rev., 2017, 117, 901–986 CrossRef CAS.
- J. Zhuang, R. H. Fang and L. Zhang, Small Methods, 2017, 1, 1700147 CrossRef.
- M. Longmire, P. L. Choyke and H. Kobayashi, Nanomedicine, 2008, 3, 703–717 CrossRef CAS.
- J. S. Suk, Q. G. Xu, N. Kim, J. Hanes and L. M. Ensign, Adv. Drug Delivery Rev., 2016, 99, 28–51 CrossRef CAS.
- J. V. Jokerst, T. Lobovkina, R. N. Zare and S. S. Gambhir, Nanomedicine, 2011, 6, 715–728 CrossRef CAS.
- L. Zhang, Z. Cao, Y. Li, J. R. Ella-Menye, T. Bai and S. Jiang, ACS Nano, 2012, 6, 6681–6686 CrossRef CAS.
- R. A. Sperling and W. J. Parak, Philos. Trans. R. Soc., A, 2010, 368, 1333–1383 CrossRef CAS.
- D. D. Von Hoff, M. M. Mita, R. K. Ramanathan, G. J. Weiss, A. C. Mita, P. M. LoRusso, H. A. Burris, 3rd, L. L. Hart, S. C. Low, D. M. Parsons, S. E. Zale, J. M. Summa, H. Youssoufian and J. C. Sachdev, Clin. Cancer Res., 2016, 22, 3157–3163 CrossRef CAS.
- S. Sharma, P. G. Jackson and J. Makan, J. Clin. Pathol., 2004, 57, 1025–1026 CrossRef CAS.
- M. Abdorahim, M. Rabiee, S. N. Alhosseini, M. Tahriri, S. Yazdanpanah, S. H. Alavi and L. Tayebi, TrAC, Trends Anal. Chem., 2016, 82, 337–347 CrossRef CAS.
- M. Diaz-Gonzalez, X. Munoz-Berbel, C. Jimenez-Jorquera, A. Baldi and C. Fernandez-Sanchez, Electroanalysis, 2014, 26, 1154–1170 CrossRef CAS.
- Q. Xu and J. J. Davis, Electroanalysis, 2014, 26, 1249–1258 CrossRef CAS.
- D. Brondani, J. V. Piovesan, E. Westphal, H. Gallardo, R. A. Fireman Dutra, A. Spinelli and I. C. Vieira, Analyst, 2014, 139, 5200–5208 RSC.
- B. Wang, R. Jing, H. L. Qi, Q. Gao and C. X. Zhang, J. Electroanal. Chem., 2016, 781, 212–217 CrossRef CAS.
- D. W. Kimmel, G. LeBlanc, M. E. Meschievitz and D. E. Cliffel, Anal. Chem., 2012, 84, 685–707 CrossRef CAS.
- F. T. C. Moreira, R. A. F. Dutra, J. P. C. Noronha, A. L. Cunha and M. G. F. Sales, Biosens. Bioelectron., 2011, 28, 243–250 CrossRef CAS.
- S. R. Mikkelsen and G. A. Rechnitz, Anal. Chem., 1989, 61, 1737–1742 CrossRef CAS.
- I. Lee, X. Luo, J. Huang, X. T. Cui and M. Yun, Biosensors, 2012, 2, 205–220 CrossRef CAS.
- P. Singh, S. Pandit, V. Mokkapati, A. Garg, V. Ravikumar and I. Mijakovic, Int. J. Mol. Sci., 2018, 19, 1979 CrossRef.
- J. E. Lemaster and J. V. Jokerst, Wiley Interdiscip. Rev.: Nanomed. Nanobiotechnol., 2017, 9, e1404 CrossRef.
- L. Rouleau, R. Berti, V. W. Ng, C. Matteau-Pelletier, T. Lam, P. Saboural, A. K. Kakkar, F. Lesage, E. Rheaume and J. C. Tardif, Contrast Media Mol. Imaging, 2013, 8, 27–39 CrossRef CAS.
- B. Wang, J. L. Su, J. Amirian, S. H. Litovsky, R. Smalling and S. Emelianov, Opt. Express, 2010, 18, 4889–4897 CrossRef CAS.
- M. Adhi and J. S. Duker, Curr. Opin. Ophthalmol., 2013, 24, 213–221 CrossRef.
- J. Hu, F. Rivero, R. A. Torres, H. Loro Ramirez, E. M. Rodriguez, F. Alfonso, J. Garcia Sole and D. Jaque, J. Biophotonics, 2017, 10, 674–682 CrossRef CAS.
- J. Hu, F. Sanz-Rodriguez, F. Rivero, E. M. Rodriguez, R. A. Torres, D. H. Ortgies, J. G. Sole, F. Alfonso and D. Jaque, Nano Res., 2018, 11, 676–685 CrossRef CAS.
- D. T. Ginat and R. Gupta, Annu. Rev. Biomed. Eng., 2014, 16, 431–453 CrossRef CAS.
- L. E. Cole, R. D. Ross, J. M. R. Tilley, T. Vargo-Gogola and R. K. Roeder, Nanomedicine, 2015, 10, 321–341 CrossRef CAS PubMed.
- P. Chhour, P. C. Naha, S. M. O'Neill, H. I. Litt, M. P. Reilly, V. A. Ferrari and D. P. Cormode, Biomaterials, 2016, 87, 93–103 CrossRef CAS.
- M. Salerno and C. M. Kramer, Expert Opin. Med. Diagn., 2009, 3, 673–687 CrossRef.
- V. M. Runge, B. R. Carollo, C. R. Wolf, K. L. Nelson and D. Y. Gelblum, Radiographics, 1989, 9, 929–958 CrossRef CAS.
- A. L. Doiron, K. Chu, A. Ali and L. Brannon-Peppas, Proc. Natl. Acad. Sci. U. S. A., 2008, 105, 17232–17237 CrossRef CAS.
- A. B. Sigalov, Contrast Media Mol. Imaging, 2014, 9, 372–382 CrossRef CAS.
- Z. Shen, A. Wu and X. Chen, Mol. Pharmaceutics, 2017, 14, 1352–1364 CrossRef CAS.
- F. F. Li, D. B. Zhi, Y. F. Luo, J. Q. Zhang, X. Nan, Y. J. Zhang, W. Zhou, B. S. Qiu, L. P. Wen and G. L. Liang, Nanoscale, 2016, 8, 12826–12833 RSC.
- Y. B. Wang, J. W. Chen, B. Yang, H. Y. Qiao, L. Gao, T. Su, S. Ma, X. T. Zhang, X. J. Li, G. Liu, J. B. Cao, X. Y. Chen, Y. D. Chen and F. Cao, Theranostics, 2016, 6, 272–286 CrossRef CAS.
- C. Tarin, M. Carril, J. L. Martin-Ventura, I. Markuerkiaga, D. Padro, P. Llamas-Granda, J. A. Moreno, I. Garcia, N. Genicio, S. Plaza-Garcia, L. M. Blanco-Colio, S. Penades and J. Egido, Sci. Rep., 2015, 5, 17135 CrossRef CAS.
- D. F. Cheng, X. Li, C. F. Zhang, H. Tan, C. Wang, L. F. Pang and H. C. Shi, ACS Appl. Mater. Interfaces, 2015, 7, 2847–2855 CrossRef CAS.
- B. S. Harrison and A. Atala, Biomaterials, 2007, 28, 344–353 CrossRef CAS.
- R. H. Baughman, A. A. Zakhidov and W. A. de Heer, Science, 2002, 297, 787–792 CrossRef CAS.
- L. G. Delogu, G. Vidili, E. Venturelli, C. Menard-Moyon, M. A. Zoroddu, G. Pilo, P. Nicolussi, C. Ligios, D. Bedognetti, F. Sgarrella, R. Manetti and A. Bianco, Proc. Natl. Acad. Sci. U. S. A., 2012, 109, 16612–16617 CrossRef CAS PubMed.
- E. J. Keliher, Y. X. Ye, G. R. Wojtkiewicz, A. D. Aguirre, B. Tricot, M. L. Senders, H. Groenen, F. Fay, C. Perez-Medina, C. Calcagno, G. Carlucci, T. Reiner, Y. Sun, G. Courties, Y. Iwamoto, H. Y. Kim, C. Wang, J. W. Chen, F. K. Swirski, H. Y. Wey, J. Hooker, Z. A. Fayad, W. J. Mulder, R. Weissleder and M. Nahrendorf, Nat. Commun., 2017, 8, 14064 CrossRef CAS PubMed.
- G. Fredman, N. Kamaly, S. Spolitu, J. Milton, D. Ghorpade, R. Chiasson, G. Kuriakose, M. Perretti, O. Farokzhad and I. Tabas, Sci. Transl. Med., 2015, 7, 275ra20 CrossRef CAS PubMed.
- N. Kamaly, G. Fredman, J. J. Fojas, M. Subramanian, W. I. Choi, K. Zepeda, C. Vilos, M. Yu, S. Gadde, J. Wu, J. Milton, R. Carvalho Leitao, L. Rosa Fernandes, M. Hasan, H. Gao, V. Nguyen, J. Harris, I. Tabas and O. C. Farokhzad, ACS Nano, 2016, 10, 5280–5292 CrossRef CAS PubMed.
- N. V. Goncharov, P. V. Avdonin, A. D. Nadeev, I. L. Zharkikh and R. O. Jenkins, Curr. Pharm. Des., 2015, 21, 1134–1146 CrossRef CAS PubMed.
- Y. Wang, L. Li, W. Zhao, Y. Dou, H. An, H. Tao, X. Xu, Y. Jia, S. Lu, J. Zhang and H. Hu, ACS Nano, 2018, 12, 8943–8960 CrossRef CAS PubMed.
- P. M. Ridker and N. R. Cook, Lancet, 2013, 382, 1762–1765 CrossRef.
- S. Katsuki, T. Matoba, S. Nakashiro, K. Sato, J. Koga, K. Nakano, Y. Nakano, S. Egusa, K. Sunagawa and K. Egashira, Circulation, 2014, 129, 896–906 CrossRef CAS.
- R. Duivenvoorden, J. Tang, D. P. Cormode, A. J. Mieszawska, D. Izquierdo-Garcia, C. Ozcan, M. J. Otten, N. Zaidi, M. E. Lobatto, S. M. van Rijs, B. Priem, E. L. Kuan, C. Martel, B. Hewing, H. Sager, M. Nahrendorf, G. J. Randolph, E. S. Stroes, V. Fuster, E. A. Fisher, Z. A. Fayad and W. J. Mulder, Nat. Commun., 2014, 5, 3065 CrossRef.
- J. Tang, M. E. Lobatto, L. Hassing, S. van der Staay, S. M. van Rijs, C. Calcagno, M. S. Braza, S. Baxter, F. Fay, B. L. Sanchez-Gaytan, R. Duivenvoorden, H. Sager, Y. M. Astudillo, W. Leong, S. Ramachandran, G. Storm, C. Perez-Medina, T. Reiner, D. P. Cormode, G. J. Strijkers, E. S. Stroes, F. K. Swirski, M. Nahrendorf, E. A. Fisher, Z. A. Fayad and W. J. Mulder, Sci. Adv., 2015, 1, e1400223 CrossRef.
- K. J. Moore, F. J. Sheedy and E. A. Fisher, Nat. Rev. Immunol., 2013, 13, 709–721 CrossRef CAS.
- M. Yu, J. Amengual, A. Menon, N. Kamaly, F. Zhou, X. D. Xu, P. E. Saw, S. J. Lee, K. Si, C. A. Ortega, W. I. Choi, I. H. Lee, Y. Bdour, J. J. Shi, M. Mahmoudi, S. Jon, E. A. Fisher and O. C. Farokhzad, Adv. Healthcare Mater., 2017, 6, 1700313 CrossRef.
- D. R. Lewis, L. K. Petersen, A. W. York, K. R. Zablocki, L. B. Joseph, V. Kholodovych, R. K. Prud'homme, K. E. Uhrich and P. V. Moghe, Proc. Natl. Acad. Sci. U. S. A., 2015, 112, 2693–2698 CrossRef CAS.
- R. Kapadia, J. H. Yi and R. Vemuganti, Front. Biosci., 2008, 13, 1813–1826 CrossRef CAS.
- S. Nakashiro, T. Matoba, R. Umezu, J. Koga, M. Tokutome, S. Katsuki, K. Nakano, K. Sunagawa and K. Egashira, Arterioscler., Thromb., Vasc. Biol., 2016, 36, 491–500 CrossRef CAS.
- N. A. Michel, A. Zirlik and D. Wolf, Front. Cardiovasc. Med., 2017, 4, 40 CrossRef.
- M. Lameijer, T. Binderup, M. M. T. van Leent, M. L. Senders, F. Fay, J. Malkus, B. L. Sanchez-Gaytan, A. J. P. Teunissen, N. Karakatsanis, P. Robson, X. X. Zhou, Y. X. Ye, G. Wojtkiewicz, J. Tang, T. T. P. Seijkens, J. Kroon, E. S. G. Stroes, A. Kjaer, J. Ochando, T. Reiner, C. Perez-Medina, C. Calcagno, E. A. Fischer, B. Zhang, R. E. Temel, F. K. Swirski, M. Nahrendorf, Z. A. Fayad, E. Lutgens, W. J. M. Mulder and R. Duivenvoorden, Nat. Biomed. Eng., 2018, 2, 279–292 CrossRef CAS PubMed.
- A. Bonaventura, F. Montecucco and F. Dallegri, Expert Opin. Biol. Ther., 2016, 16, 1323–1340 CrossRef CAS.
- M. Juenet, R. Aid-Launais, B. Li, A. Berger, J. Aerts, V. Ollivier, A. Nicoletti, D. Letourneur and C. Chauvierre, Biomaterials, 2018, 156, 204–216 CrossRef CAS PubMed.
- C. L. Pawlowski, W. Li, M. Sun, K. Ravichandran, D. Hickman, C. Kos, G. Kaur and A. Sen Gupta, Biomaterials, 2017, 128, 94–108 CrossRef CAS PubMed.
- Y. Zhong, Y. Zhang, J. Xu, J. Zhou, J. Liu, M. Ye, L. Zhang, B. Qiao, Z.-G. Wang, H.-T. Ran and D. Guo, ACS Nano, 2019, 13, 3387–3403 CrossRef CAS.
- R. X. Yin, D. Z. Yang and J. Z. Wu, Theranostics, 2014, 4, 175–200 CrossRef CAS PubMed.
- J. Zhao, Z. Mo, F. Guo, D. Shi, Q. Q. Han and Q. Liu, J. Biomed. Mater. Res., Part B, 2018, 106, 88–95 CrossRef CAS.
- D. Son, J. Lee, D. J. Lee, R. Ghaffari, S. Yun, S. J. Kim, J. E. Lee, H. R. Cho, S. Yoon, S. Yang, S. Lee, S. Qiao, D. Ling, S. Shin, J. K. Song, J. Kim, T. Kim, H. Lee, J. Kim, M. Soh, N. Lee, C. S. Hwang, S. Nam, N. Lu, T. Hyeon, S. H. Choi and D. H. Kim, ACS Nano, 2015, 9, 5937–5946 CrossRef CAS.
- T. Ishida, M. Harada, X. Y. Wang, M. Ichihara, K. Irimura and H. Kiwada, J. Controlled Release, 2005, 105, 305–317 CrossRef CAS.
- T. Ishida and H. Kiwada, Int. J. Pharm., 2008, 354, 56–62 CrossRef CAS.
- X. Q. Zhang, X. Xu, N. Bertrand, E. Pridgen, A. Swami and O. C. Farokhzad, Adv. Drug Delivery Rev., 2012, 64, 1363–1384 CrossRef CAS.
- A. Z. Wang, F. Gu, L. Zhang, J. M. Chan, A. Radovic-Moreno, M. R. Shaikh and O. C. Farokhzad, Expert Opin. Biol. Ther., 2008, 8, 1063–1070 CrossRef CAS PubMed.
- D. Dehaini, R. H. Fang and L. Zhang, Bioeng. Transl. Med., 2016, 1, 30–46 CrossRef.
- R. H. Fang, Y. Jiang, J. C. Fang and L. Zhang, Biomaterials, 2017, 128, 69–83 CrossRef CAS.
- C. M. Hu, R. H. Fang and L. Zhang, Adv. Healthcare Mater., 2012, 1, 537–547 CrossRef CAS.
- C. L. Modery-Pawlowski, L. L. Tian, V. Pan and A. Sen Gupta, Biomacromolecules, 2013, 14, 939–948 CrossRef CAS.
- T. G. Kapp, F. Rechenmacher, S. Neubauer, O. V. Maltsev, E. A. Cavalcanti-Adam, R. Zarka, U. Reuning, J. Notni, H. J. Wester, C. Mas-Moruno, J. Spatz, B. Geiger and H. Kessler, Sci. Rep., 2017, 7, 39805 CrossRef CAS.
- T. Zhang, Z. She, Z. Huang, J. Li, X. Luo and Y. Deng, Asian J. Pharm. Sci., 2014, 9, 75–81 CrossRef.
- R. H. Fang, A. V. Kroll, W. Gao and L. Zhang, Adv. Mater., 2018, 30, 1706759 CrossRef.
- C. M. Hu, L. Zhang, S. Aryal, C. Cheung, R. H. Fang and L. Zhang, Proc. Natl. Acad. Sci. U. S. A., 2011, 108, 10980–10985 CrossRef CAS.
- C. M. Hu, R. H. Fang, B. T. Luk, K. N. Chen, C. Carpenter, W. Gao, K. Zhang and L. Zhang, Nanoscale, 2013, 5, 2664–2668 RSC.
- B. T. Luk, R. H. Fang, C. M. Hu, J. A. Copp, S. Thamphiwatana, D. Dehaini, W. Gao, K. Zhang, S. Li and L. Zhang, Theranostics, 2016, 6, 1004–1011 CrossRef CAS.
- J. G. Piao, L. M. Wang, F. Gao, Y. Z. You, Y. J. Xiong and L. H. Yang, ACS Nano, 2014, 8, 10414–10425 CrossRef CAS.
- Z. Chai, X. Hu, X. Wei, C. Zhan, L. Lu, K. Jiang, B. Su, H. Ruan, D. Ran, R. H. Fang, L. Zhang and W. Lu, J. Controlled Release, 2017, 264, 102–111 CrossRef CAS.
- R. H. Fang, C. M. Hu, K. N. Chen, B. T. Luk, C. W. Carpenter, W. Gao, S. Li, D. E. Zhang, W. Lu and L. Zhang, Nanoscale, 2013, 5, 8884–8888 RSC.
- R. H. Fang, B. T. Luk, C. M. Hu and L. Zhang, Adv. Drug Delivery Rev., 2015, 90, 69–80 CrossRef CAS.
- C. M. Hu, R. H. Fang, J. Copp, B. T. Luk and L. Zhang, Nat. Nanotechnol., 2013, 8, 336–340 CrossRef CAS PubMed.
- J. A. Copp, R. H. Fang, B. T. Luk, C. M. J. Hu, W. W. Gao, K. Zhang and L. F. Zhang, Proc. Natl. Acad. Sci. U. S. A., 2014, 111, 13481–13486 CrossRef CAS.
- Z. Q. Pang, C. M. J. Hu, R. H. Fang, B. T. Luk, W. W. Gao, F. Wang, E. Chuluun, P. Angsantikul, S. Thamphiwatana, W. Y. Lu, X. G. Jiang and L. F. Zhang, ACS Nano, 2015, 9, 6450–6458 CrossRef CAS.
- X. L. Wei, G. Zhang, D. N. Ran, N. Krishnan, R. H. Fang, W. W. Gao, S. A. Spector and L. F. Zhang, Adv. Mater., 2018, 30, 1802233 CrossRef.
- S. Thamphiwatana, P. Angsantikul, T. Escajadillo, Q. Zhang, J. Olson, B. T. Luk, S. Zhang, R. H. Fang, W. Gao, V. Nizet and L. Zhang, Proc. Natl. Acad. Sci. U. S. A., 2017, 114, 11488–11493 CrossRef CAS.
- Q. Zhang, D. Dehaini, Y. Zhang, J. Zhou, X. Chen, L. Zhang, R. H. Fang, W. Gao and L. Zhang, Nat. Nanotechnol., 2018, 13, 1182–1190 CrossRef CAS.
- X. L. Wei, J. Gao, R. H. Fang, B. T. Luk, A. V. Kroll, D. Dehaini, J. R. Zhou, H. W. Kim, W. W. Gao, W. Y. Lu and L. F. Zhang, Biomaterials, 2016, 111, 116–123 CrossRef CAS.
- X. Wei, J. Gao, F. Wang, M. Ying, P. Angsantikul, A. V. Kroll, J. Zhou, W. Gao, W. Lu, R. H. Fang and L. Zhang, Adv. Mater., 2017, 29, 1701644 CrossRef.
- P. Angsantikul, R. H. Fang and L. F. Zhang, Bioconjugate Chem., 2018, 29, 604–612 CrossRef CAS.
- C. M. Hu, R. H. Fang, K. C. Wang, B. T. Luk, S. Thamphiwatana, D. Dehaini, P. Nguyen, P. Angsantikul, C. H. Wen, A. V. Kroll, C. Carpenter, M. Ramesh, V. Qu, S. H. Patel, J. Zhu, W. Shi, F. M. Hofman, T. C. Chen, W. Gao, K. Zhang, S. Chien and L. Zhang, Nature, 2015, 526, 118–121 CrossRef CAS.
- R. H. Fang, C. M. Hu, B. T. Luk, W. Gao, J. A. Copp, Y. Tai, D. E. O'Connor and L. Zhang, Nano Lett., 2014, 14, 2181–2188 CrossRef CAS PubMed.
- L. Rao, L. L. Bu, B. Cai, J. H. Xu, A. Li, W. F. Zhang, Z. J. Sun, S. S. Guo, W. Liu, T. H. Wang and X. Z. Zhao, Adv. Mater., 2016, 28, 3460–3466 CrossRef CAS.
- J. Y. Zhu, D. W. Zheng, M. K. Zhang, W. Y. Yu, W. X. Qiu, J. J. Hu, J. Feng and X. Z. Zhang, Nano Lett., 2016, 16, 5895–5901 CrossRef CAS PubMed.
- Q. Hu, W. Sun, C. Qian, C. Wang, H. N. Bomba and Z. Gu, Adv. Mater., 2015, 27, 7043–7050 CrossRef CAS.
- M. Xuan, J. Shao, L. Dai, Q. He and J. Li, Adv. Healthcare Mater., 2015, 4, 1645–1652 CrossRef CAS.
- C. Gao, Z. Lin, B. Jurado-Sanchez, X. Lin, Z. Wu and Q. He, Small, 2016, 12, 4056–4062 CrossRef CAS.
- S. Willoughby, A. Holmes and J. Loscalzo, Eur. J. Cardiovasc. Nurs., 2002, 1, 273–288 CrossRef.
- H. M. Nording, P. Seizer and H. F. Langer, Front. Immunol., 2015, 6, 98 Search PubMed.
- B. L. Sanchez-Gaytan, F. Fay, M. E. Lobatto, J. Tang, M. Ouimet, Y. Kim, S. E. van der Staay, S. M. van Rijs, B. Priem, L. Zhang, E. A. Fisher, K. J. Moore, R. Langer, Z. A. Fayad and W. J. Mulder, Bioconjugate Chem., 2015, 26, 443–451 CrossRef CAS PubMed.
- G. P. Robbins, R. L. Saunders, J. B. Haun, J. Rawson, M. J. Therien and D. A. Hammer, Langmuir, 2010, 26, 14089–14096 CrossRef CAS PubMed.
- J. O. Martinez, R. Molinaro, K. A. Hartman, C. Boada, R. Sukhovershin, E. De Rosa, D. Kirui, S. R. Zhang, M. Evangelopoulos, A. M. Carter, J. A. Bibb, J. P. Cooke and E. Tasciotti, Theranostics, 2018, 8, 1131–1145 CrossRef CAS.
- R. Vankayala, S. R. Corber, J. T. Mac, M. P. Rao, M. Shafie and B. Anvari, Macromol. Biosci., 2018, 18, 1700379 CrossRef.
- L. Rao, L. L. Bu, Q. F. Meng, B. Cai, W. W. Deng, A. Li, K. Y. Li, S. S. Guo, W. F. Zhang, W. Liu, Z. J. Sun and X. Z. Zhao, Adv. Funct. Mater., 2017, 27, 1604774 CrossRef.
- J. Jin, T. Liu, M. Li, C. Yuan, Y. Liu, J. Tang, Z. Feng, Y. Zhou, F. Yang and N. Gu, Colloids Surf., B, 2018, 163, 385–393 CrossRef CAS.
- M. Li, Y. Liu, J. Chen, T. Liu, Z. Gu, J. Zhang, X. Gu, G. Teng, F. Yang and N. Gu, Theranostics, 2018, 8, 4870–4883 CrossRef CAS.
- X. Wei, M. Ying, D. Dehaini, Y. Su, A. V. Kroll, J. Zhou, W. Gao, R. H. Fang, S. Chien and L. Zhang, ACS Nano, 2018, 12, 109–116 CrossRef CAS.
- Y. Ikeda, M. Handa, K. Kawano, T. Kamata, M. Murata, Y. Araki, H. Anbo, Y. Kawai, K. Watanabe and I. Itagaki,
et al.
, J. Clin. Invest., 1991, 87, 1234–1240 CrossRef CAS.
- W. S. Nesbitt, E. Westein, F. J. Tovar-Lopez, E. Tolouei, A. Mitchell, J. Fu, J. Carberry, A. Fouras and S. P. Jackson, Nat. Med., 2009, 15, 665–673 CrossRef CAS.
- Z. M. Ruggeri, J. N. Orje, R. Habermann, A. B. Federici and A. J. Reininger, Blood, 2006, 108, 1903–1910 CrossRef CAS.
- N. Korin, M. Kanapathipillai, B. D. Matthews, M. Crescente, A. Brill, T. Mammoto, K. Ghosh, S. Jurek, S. A. Bencherif, D. Bhatta, A. U. Coskun, C. L. Feldman, D. D. Wagner and D. E. Ingber, Science, 2012, 337, 738–742 CrossRef CAS.
- N. Korin, M. Kanapathipillai and D. E. Ingber, Isr. J. Chem., 2013, 53, 610–615 CrossRef CAS.
- C. Chen, S. Li, K. Liu, G. Ma and X. Yan, Small, 2016, 12, 4719–4725 CrossRef CAS.
- A. M. Wen, Y. Wang, K. Jiang, G. C. Hsu, H. Gao, K. L. Lee, A. C. Yang, X. Yu, D. I. Simon and N. F. Steinmetz, J. Mater. Chem. B, 2015, 3, 6037–6045 RSC.
- S. Y. Lee, M. Ferrari and P. Decuzzi, Nanotechnology, 2009, 20, 495101 CrossRef.
- A. S. Pitek, Y. Wang, S. Gulati, H. Gao, P. L. Stewart, D. I. Simon and N. F. Steinmetz, Mol. Pharmaceutics, 2017, 14, 3815–3823 CrossRef CAS.
- A. S. Pitek, J. Park, Y. Wang, H. Gao, H. Hu, D. I. Simon and N. F. Steinmetz, Nanoscale, 2018, 10, 16547–16555 RSC.
- S. Kona, J. F. Dong, Y. Liu, J. Tan and K. T. Nguyen, Int. J. Pharm., 2012, 423, 516–524 CrossRef CAS PubMed.
- A. C. Anselmo, C. L. Modery-Pawlowski, S. Menegatti, S. Kumar, D. R. Vogus, L. L. Tian, M. Chen, T. M. Squires, A. Sen Gupta and S. Mitragotri, ACS Nano, 2014, 8, 11243–11253 CrossRef CAS.
- R. Molinaro, C. Corbo, J. O. Martinez, F. Taraballi, M. Evangelopoulos, S. Minardi, I. K. Yazdi, P. Zhao, E. De Rosa, M. B. Sherman, A. De Vita, N. E. Toledano Furman, X. Wang, A. Parodi and E. Tasciotti, Nat. Mater., 2016, 15, 1037–1046 CrossRef CAS PubMed.
- J. Tang, T. Su, K. Huang, P. U. Dinh, Z. Wang, A. Vandergriff, M. T. Hensley, J. Cores, T. Allen, T. Li, E. Sproul, E. Mihalko, L. J. Lobo, L. Ruterbories, A. Lynch, A. Brown, T. G. Caranasos, D. Shen, G. A. Stouffer, Z. Gu, J. Zhang and K. Cheng, Nat. Biomed. Eng., 2018, 2, 17–26 CrossRef CAS PubMed.
- O. C. Farokhzad, Nature, 2015, 526, 47–48 CrossRef CAS.
- T. Yang, X. Ding, L. Dong, C. Hong, J. Ye, Y. Xiao, X. Wang and H. Xin, ACS Biomater. Sci. Eng., 2018, 4, 4219–4224 CrossRef CAS.
- B. Wang, G. Chen, G. Urabe, R. Xie, Y. Wang, X. Shi, L. W. Guo, S. Gong and K. C. Kent, Biomaterials, 2018, 178, 293–301 CrossRef CAS.
- Y. Song, Z. Huang, X. Liu, Z. Pang, J. Chen, H. Yang, N. Zhang, Z. Cao, M. Liu, J. Cao, C. Li, X. Yang, H. Gong, J. Qian and J. Ge, Nanomedicine, 2019, 15, 13–24 CrossRef CAS.
- T. Su, K. Huang, H. Ma, H. Liang, P.-U. Dinh, J. Chen, D. Shen, T. A. Allen, L. Qiao, Z. Li, S. Hu, J. Cores, B. N. Frame, A. T. Young, Q. Yin, J. Liu, L. Qian, T. G. Caranasos, Y. Brudno, F. S. Ligler and K. Cheng, Adv. Funct. Mater., 2019, 29, 1803567 CrossRef.
- Q. Hu, C. Qian, W. Sun, J. Wang, Z. Chen, H. N. Bomba, H. Xin, Q. Shen and Z. Gu, Adv. Mater., 2016, 28, 9573–9580 CrossRef CAS.
- W. Lv, J. Xu, X. Wang, X. Li, Q. Xu and H. Xin, ACS Nano, 2018, 12, 5417–5426 CrossRef CAS.
- A. Parodi, N. Quattrocchi, A. L. van de Ven, C. Chiappini, M. Evangelopoulos, J. O. Martinez, B. S. Brown, S. Z. Khaled, I. K. Yazdi, M. V. Enzo, L. Isenhart, M. Ferrari and E. Tasciotti, Nat. Nanotechnol., 2013, 8, 61–68 CrossRef CAS.
- R. J. Bose, B. J. Kim, Y. Arai, I. B. Han, J. J. Moon, R. Paulmurugan, H. Park and S. H. Lee, Biomaterials, 2018, 185, 360–370 CrossRef CAS PubMed.
- D. Dehaini, X. Wei, R. H. Fang, S. Masson, P. Angsantikul, B. T. Luk, Y. Zhang, M. Ying, Y. Jiang, A. V. Kroll, W. Gao and L. Zhang, Adv. Mater., 2017, 29, 1606209 CrossRef PubMed.
- C. S. Kim, B. Duncan, B. Creran and V. M. Rotello, Nano Today, 2013, 8, 439–447 CrossRef CAS.
- N. Nouraee and S. J. Mowla, Front. Genet., 2015, 6, 232 Search PubMed.
Footnote |
† These authors contributed equally to this work. |
|
This journal is © The Royal Society of Chemistry 2020 |
Click here to see how this site uses Cookies. View our privacy policy here.