DOI:
10.1039/C8EN01167B
(Tutorial Review)
Environ. Sci.: Nano, 2019,
6, 1006-1025
Metal–organic framework-based nanomaterials for adsorption and photocatalytic degradation of gaseous pollutants: recent progress and challenges
Received
20th October 2018
, Accepted 23rd November 2018
First published on 18th February 2019
Abstract
The development of porous nanomaterials with high efficiency for environmental remediation has been attracting significant attention and becoming an important topic recently. Metal–organic frameworks (MOF) are hybrid inorganic–organic porous materials containing a metal–oxygen cluster and organic molecules, which are becoming an alternative to traditional inorganic porous materials, such as zeolite and silica, for environmental remediation owing to their fascinating characteristics. Recent studies have demonstrated that MOF are one of the most efficient adsorbents or catalysts in gas separation, solar energy conversion and photocatalytic applications. This review mainly summarizes the recent progress on the adsorptive and degradation treatment of various gaseous pollutants by MOF materials. In this review, the physical and chemical adsorption of gaseous pollutants in air by MOFs will be discussed, and strategies for maximizing the adsorption capacity by tuning the physical and chemical properties of MOFs at the atomic level are systematically summarized. In particular, a promising strategy based on the synergistic effect of adsorption-concentrated photocatalytic oxidation of gaseous pollutants with this newly emerging MOF is also introduced, as it holds great potential in the treatment of gaseous pollutants in consideration of its high efficiency, low cost and being free from secondary pollution. In the end, the challenges faced, the prospects, and our personal perspective on future research directions are also estimated and elucidated.
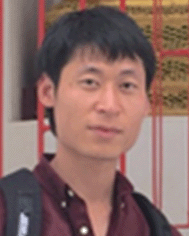 Meicheng Wen | Dr. Meicheng Wen received his Ph.D. degree in engineering from Osaka University under the supervision of Prof. Hiromi Yamashita in 2016. He was a specially appointed assistant professor in the Division of Materials and Manufacturing Science at Osaka University in 2016–2017. He is now an associate professor at Guangdong University of Technology, China. His research mainly focuses on preparation of nanomaterials, and their applications in energy conversion and environmental remediation. |
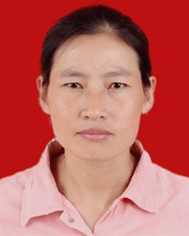 Guiying Li | Prof. Guiying Li received her bachelor's and master's degrees from Nanjing Agricultural University and Northwest Normal University, China, respectively, and her PhD degree in environmental science from Griffith University, Australia. She is a full professor of the Institute of Environmental Health and Pollution Control as well as the School of Environmental Science and Engineering, Guangdong University of Technology. Her academic background covers both microbiology and environmental science. Her research interest mainly focuses on the development of photocatalysis- and photoelectrocatalysis-based bactericidal methods, and the application mechanism of photocatalytic bactericidal decomposition; and the biodegradation of organic pollutants in liquid and gaseous phases using isolated bacterial strains. She has published more than 170 SCI papers in reputable journals. She is the holder of 30 issued patents both from China and the USA, and the second award winner of the Natural Science Prize from the Ministry of Education (MOE), China. Now, she also serves on the editorial board of the Research Journal of Biotechnology. |
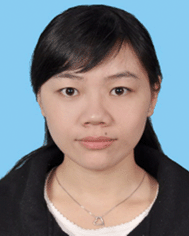 Hongli Liu | Dr. Hongli Liu received her PhD degree from the school of chemistry and chemical engineering of South China University of Technology in 2013, and she then conducted postdoctoral work at the same university from 2013 to 2015. She is currently an associate professor of the Institute of Environmental Health and Pollution Control as well as the School of Environmental Science and Engineering, Guangdong University of Technology. Her research focuses on the design and preparation of MOF-based composite materials for heterogeneous catalysis as well as gas adsorption and separation. |
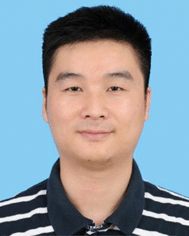 Jiangyao Chen | Dr. Jiangyao Chen received his PhD degree in Environmental Science from Guangzhou Institute of Geochemistry, CAS under the supervision of Prof. Taicheng An. He is now an associate professor at Guangdong University of Technology, China with research interest in environmental function photocatalyst design, VOC photochemical transformation mechanisms and polluted air photocatalytic purification. |
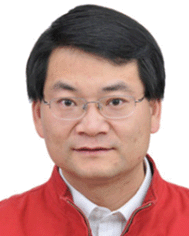 Taicheng An | Prof. Taicheng An received his PhD in environmental science from Sun Yat-Sen University, China in 2002. He is currently a full professor at the School of Environmental Sciences and Engineering, and the director of the Institute of Environmental Health and Pollution Control, Guangdong University of Technology. His research interests mainly focus on: 1. the transportation mechanisms, fate prediction and health effects of emerging organic contaminants; 2. design and synthesis of nanostructured catalysts and natural mineral materials in environmental remediation; 3. applications of advanced oxidation processes, especially heterogeneous photocatalysis in organic degradation and biohazard inactivation in water and air. He has over 70 patents (40 issued) and has published over 300 refereed research papers (including 260 SCI papers) in reputable journals. He is the winner of the National Natural Science Funds for Distinguished Young Scholars, the second award winner of the Natural Science Prize from the Ministry of Education (MOE, China), a Distinguished Professor of Chang Jiang Scholars of MOE as well as the Pearl River Scholars Program, and the Young Scientist Winner of the Scientific Committee on Problems of the Environment (SCOPE) in 2011. |
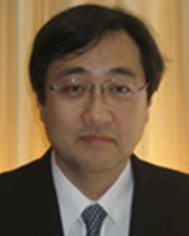 Hiromi Yamashita | Prof. Hiromi Yamashita has been a professor of Osaka University since 2004. He received his PhD degree from Kyoto University in 1987. He was an assistant professor of Tohoku University, an associate professor of Osaka Prefecture University, and an invited professor of University Pierre and Marie Curie (UPMC). He was also visiting research fellow at Pennsylvania State University, the University of Texas at Austin, and California Institute of Technology. His research interests include the design of single-site photocatalysts and application of nanostructured catalysts. |
Environmental significance
Metal–organic frameworks (MOFs) are hybrid organic–inorganic porous crystalline nanomaterials that have aroused great attention in environmental remediation due to their crystalized nano- (<2 nm) or meso- (2–50 nm) porous structure, extremely high surface area, and significant chemical diversity. Considering the rapid development of MOFs in the application of gaseous pollutant abatement, this review article mainly summarizes the recent progress and challenges with the design and engineering of MOFs for gaseous pollutant remediation, including inorganic toxic gases, organic vapors as well as particulate matter. The effects of cavity and pore size, functional groups, hydrophobic/hydrophilic properties, and surface unsaturated metal ions of various nanostructured MOFs on the adsorption/photocatalytic degradation of various gaseous pollutants are reviewed in detail. This review paper may serve as a valuable platform that offers guidelines on the design of MOFs for selective adsorption/degradation of targeted gaseous pollutants in future applications.
|
1. Introduction
The release of toxic pollutants into environmental matrixes is a worldwide risk of growing concern.1 A large number of different types of hazardous materials are emitted into water and air every year, including NOx, SOx, CO, nitrogen-containing organic compounds (e.g. hydrogen cyanide), sulfur-containing compounds (e.g. organothiols) as well as volatile organic compounds.2–4 These toxic pollutants are mainly derived from anthropogenic activities,5–7 such as burning of fossil fuel, the leakage of harmful industrial gases and vapors, and chemical warfare agents, as well as industrial sewage discharge.8–10 The discharge of harmful gases/vapors into air can cause significant harm to the eco-environment and human health.11–14 Owing to the considerable increase of harmful industrial gases/vapors emissions, a stringent regulation has been proposed whereby the maximum VOC emission level by 2020 should be reduced by half in comparison with that emitted in 2000 in EU member countries.8 Therefore, efficient removal of toxic pollutants from the environment has attracted a great deal of attention and is becoming a very hot topic.
The effective removal of harmful gases/vapors is of significant importance for the protection of the environment and for the people who are at danger of being exposed to such hazardous compounds.15 Currently, there are many technologies for environmental purification, including membrane separation, incineration and oxidation.16–18 However, most treatment methods suffer from high cost, low efficiency and/or the generation of secondary pollutants. In this regard, adsorption and photocatalysis are emerging as cost-effective advanced technologies for environmental purification in the view of their low cost, low levels of secondary products, easy separation and environmentally benign nature.19
The adsorption capture of harmful gas/vapor is based on the adsorption capacity and selectivity of a porous adsorbent, of which the specific toxic materials can access the pores of a solid adsorbent and be trapped via van der Waals force (physical adsorption) or chemical bond formed between the adsorbent and adsorbate (chemical adsorption).20–22 The physical adsorption capacity of a solid adsorbent is significantly affected by its pore and cavity size as well as surface area, where the chemical adsorption capacity of a solid adsorbent is highly dependent on its surface functionality (acid or basic), surface atomic coordination, and electron density (electron deficient or electron rich). There are many porous materials with large surface area and rigid structure that have been explored as adsorbents for physical adsorption of toxic compounds. These materials include activated carbon, porous silica, aluminosilicate zeolites, carbon nanotubes, resins, molecular sieves and pillared clays.18,23–27 Considerable achievements have been obtained by using these inorganic porous materials as adsorbents for capturing toxic compounds, some of which are now being used in industry in practical applications.28 However, owing to their inner surface properties, the adsorption capacity of these inorganic porous materials is mainly based on physical adsorption, whilst chemical adsorption contributes a little. To increase the chemical adsorption capacity, complicated chemical modification processes are usually required to functionalize these adsorbents to create specific adsorption sites.29,30 On the basis of the above discussion, a solid material, which is able to offer sufficient design opportunities, is highly desirable for efficiently removing harmful gas/vapor from the environment. Metal–organic frameworks (MOF) are an attractive class of organic–inorganic porous materials, constructed from inorganic secondary building units (metal oxide clusters or metal ions) coordinating to organic moieties.31–33 Such coordination polymers offer significant chemical and structural diversity, and have outperformed the traditional porous materials in adsorption of harmful gas/vapor owing to the ability to elaborately tailor the pore size and chemical property of MOFs at the molecular level.34,35 According to the size and chemical features of the adsorbate, MOF with well-defined adsorption sites anchored on ligands as well as certain pore sizes can be designed and fabricated by altering the identity of either the connecting center or organic linker, holding great potential in gas/vapor adsorption applications. Normally, the quantum sized nodes of MOFs composed of a transitional metal ion linked with the oxygen of an organic linker offer a large number of electron-deficient open metal sites, which benefit the chemical adsorption of gas/vapor via adsorbate–surface interactions. In addition, MOFs are a class of highly crystallized solid materials having semiconducting property, affording remarkable photocatalytic activity to the degradation of organic pollutants with considerable thermal stability. Therefore, these unique characteristics endow MOFs with a “self-cleaning” ability, in which the adsorbed gas/vapor is in situ degraded upon light irradiation. Thus, the purification of gaseous pollutants from the environment by MOFs will likely play a vital role in the near future.
In this review, the physical and chemical adsorption of hazardous pollutants existing in the atmospheric environment by MOFs is mainly summarized, and this review is conceptually divided into four major categories: (1) toxic inorganic gases, volatile organic compounds (VOCs) and particulate matter adsorption based on size confinement and adsorbate–surface interactions are respectively reviewed and summarized. (2) Strategies for maximizing the adsorption capacity, as the adsorption uptake is significantly affected by many factors, including functionality, humidity, coordinatively unsaturated metal cations, acidity, basicity and defects in the MOF. In this section, the successful strategies for tuning the surface properties to enhance the adsorption capacity are systematically summarized. (3) MOF-based photocatalysts for the degradation of gaseous pollutants as well as adsorbent regeneration. (4) Finally, the challenges faced and our personal perspective on future research directions are also discussed and pointed out. We do believe that MOFs have a bright future in environmental purification, not only for physical and chemical adsorption removal, but also for photocatalytic degradation of hazardous atmospheric pollutants.
2. Adsorption of gaseous pollutants with MOF-based nanomaterials
2.1 Toxic inorganic gas adsorption with MOF-based nanomaterials
Usually, named toxic and hazardous gases, including H2S, CO, NOx, NH3, halogens and SOx, mainly released by burning of fossil fuels, are of major concern for environmental air pollution. These toxic gases are a major threat to the environment and human health, leading to photochemical smog, acid rain, and greenhouse effect, as well as various respiratory diseases from their chronic exposure even at very low concentrations. Therefore, the removal of these harmful gases from the environment is an important aspect for pollution control and the protection of human health. Considering the physicochemical characteristics of these toxic gases, most of them are redox active, σ-donor or π-acceptor substances, so their physical adsorption by using an adsorbent with adequate pore size and cavity is not enough, since the weak force of physical attraction between the adsorbate and adsorbent leads to a low adsorption capacity and secondary pollution. Therefore, chemical adsorption by forming specific interactions between the adsorbent and the adsorbate is highly desirable. In this section, the chemical adsorption of toxic gases by MOFs will be discussed.
Adsorption of H2S.
H2S is a flammable, poisonous and corrosive gas emitted from municipal sewage, animal farms, polluted sewers and ports, and may travel along the ground as it is heavier than air. This substance causes inflammation of the eyes, skin burns, and respiratory diseases, and significantly affects human health. The toxicity level of 100 ppm is reported to be immediately dangerous to life or health.20 Therefore, MOF with high affinity, capacity and selectivity for H2S are vital to remove trace H2S. Up to now, several MOFs, such as HKUST-21, MIL-47(V), and MIL-53(Cr, Al), have been applied to the physical adsorption of H2S, while MIL-101(Cr), MIL-53(Fe), and UiO-67 have been employed for adsorption of H2S.36,37 Those MOFs displayed excellent adsorption capacity and affinity towards H2S. The newly formed coordination bond between the open metal site and the functionality of MOFs and the redox active H2S is considered as the main reason for the extremely high adsorption capacity of MOFs.
To systematically understand the adsorption mechanism, Zou and co-workers employed 11 MOFs with various open metal sites, organic linkers, surface areas and porous structures for the experimental and theoretical study of H2S capturing.38 Different adsorption mechanisms were found for the 11 different MOFs: reversible physical adsorption occurred on Mg-MOF-74, UiO-66, ZIF-8, MIL-101(Cr), and Ce-BTC, while irreversible chemical adsorption happened on the other Cu- and Zn-based MOFs owing to the formation of metal sulfide. Among them, Mg-MOF-74 is the most stable adsorbent for H2S capture with uptake of 0.24 mmol g−1, as shown in Fig. 1. The high stability of Mg-MOF-74 for H2S capture can be attributed to the strong O–Mg–O bond in Mg-MOF-74, which prevents the formation of S–Mg bonds, leading to the physical adsorption of H2S. In contrast, MIL-101(Cr) showed enhanced adsorption capacity towards H2S and remarkable stability as compared with Mg-MOF-74 because the p orbital electrons of sulfur approach the open metal site of MIL-101(Cr) as well as the strong Cr–O bond. It should be noted that Cu-BDC, Zn-MOF-74, MOF-5, HKUST-1, UiO-66-NH2 and Fe-BTC(gel) all exhibited excellent adsorption capacity for H2S capture because of the strong chemical interactions between S atoms and open metal sites as well as the functional groups of MOFs. It is well known that by transferring the p electron of sulfur to the d orbital of a transition metal, p–d bonds can be formed. Therefore, Cu, Zn and Fe cations could easily coordinate with H2S to form metal sulfide, resulting in the enhancement of the adsorption capacity towards H2S. Such strong interaction of MOF with H2S can be observed by means of X-ray diffraction and X-ray pair distribution function analysis as the crystal structure of MOFs and the electron density of the open metal site change slightly after H2S adsorption. The strong binding force between H2S and the open metal sites of the MOF can efficiently prevent the secondary pollution problem of H2S. However, in the case of MOF regeneration, irreversible adsorption capacity is observed, which can be ascribed to the strong sulfur binding by covalent bond. It has been reported that the adsorbed H2S can be released by treating these materials in a humid atmosphere where H2O molecules replace the H2S at the metal site, resulting in the partial recovery of the MOF. However, there are few studies reported on the regeneration of MOFs in the case of H2S adsorption. Reactive adsorption of H2S by MOF is currently believed to be an effective method. The results from the above showed that MOFs displayed many advantages in the capture of H2S. It can be summarized from the above studies that H2S, as an acidic redox active substance, can be captured via the strong chemical interactions between H2S atoms and open metal sites as well as the functionalities of MOFs.
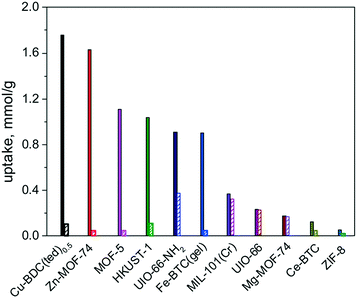 |
| Fig. 1 H2S uptake on different MOFs at 298 K under 1 atm (H2S, 1 kPa; filled column: fresh MOF adsorbents; striped column: refreshed adsorbents). Reproduced with permission from ref. 38. | |
Adsorption of SO2.
It is well known that sulfur is naturally contained in fossil fuels, in both oils and natural gas. The combustion of fossil fuels and oils produces a large amount of SO2, which can immediately affect human health after breathing it in. The toxicity level that is immediately dangerous to life or health is reported to be 100 ppm.20 Therefore, the efficient capturing and removal of SO2 is of significant importance to human life owing to the adverse health effects of SO2. Given that SO2 is a σ-donor/π-acceptor substance and the open metal site of MOF is electron deficient, a strong interaction between SO2 and the open metal site is expected. This hypothesis is confirmed by Schmidt and co-workers' recent DFT calculation study, which showed that SO2 can interact with the open metal site of Mg-MOF-74 with very high binding strength up to 72.8 kJ mol−1.39 Experimental and theoretical calculation study on the interaction of SO2 with M-MOF-74 (M = Mg, Zn) was also investigated by Chabal and co-workers, who demonstrated that SO2 is strongly adsorbed onto MOF via one oxygen of SO2 interacting with the open metal site as the primary interaction, as well as the interaction between sulfur and the oxygen of the linker. The binding energy between SO2 and MOFs was calculated to be >90 kJ mol−1 for Mg-MOF-74 and >70 kJ mol−1 for Zn-MOF-74.40 However, up to now, only a few works have reported the adsorption of SO2 by using MOFs as adsorbents, mainly owing to the poor coordination force between the metal cluster and the organic linker of MOF and highly reactive SO2. On the other hand, after adsorption of SO2 by MOFs, H2SO3 and H2SO4 may form and bind extremely strongly with the metal sites of MOFs, resulting in irreversible blocking pf the adsorption capacity of MOFs and collapse of the structure of MOFs.39 In this regard, a highly robust MOF MFM-300(In) constructed by using biphenyl-3,3′,5,5′-tetracarboxylic acid as an organic linker and In3+ as a metal centre was reported.41 The multi coordination between clusters and organic linkers of MFM-300(In) led to a highly porous and robust material with one-dimensional pore channels. MFM-300(In) has high affinity for SO2, and the capacity of MFM-300(In) for SO2 adsorption was measured to be 8.28 mmol g−1 at 298 K and 1 bar. It was suggested that the interaction of SO2 with the hydroxyl group of the MOFs and the interaction between two SO2 molecules accounted for the high adsorption capacity of MFM-300(In) and, more importantly, the framework structure of MFM-300(In) is well preserved after contacting with SO2, H2SO3 and H2SO4, displaying extremely high stability and applicability for SO2 capture. On the basis of the above study, optimizing the coordination bond between the metal cluster and the organic linker to form robust MOFs and rational design of the pore and cavity structure to confine a certain amount of adsorbate within the MOFs by forming an interaction between the adsorbate and the adsorbent, as well as the interaction between adsorbates, are promising strategies to enhance the adsorption capacity and stability of MOFs for toxic gas adsorption.
Adsorption of NH3, CO, and NOx.
Besides H2S and SO2, NH3, CO, and NOx can also be efficiently captured by MOFs. MOFs with open metal sites have been shown to behave as efficient adsorbents for toxic gas adsorption, which is highly beneficial for the capture of redox active toxic gases. Therefore, synthesis of MOFs with large numbers of open metal sites is highly desirable to bind toxic gases. Subsequently, a series of biodegradable iron-based MOFs, including MIL-88A (Fe3O(CH3OH)3(fumarate)3), MIL-88B (Fe3OX(1,4-benzenedicarboxylate)3 (X = F, Cl, OH)), MIL-88B-NO2 (Fe3OX(2-nirtoterephthalate)3), MIL-88B-2OH (Fe3OX(2,5-dihydroxyterephthalate)3) were synthesized at large scale and investigated for the adsorption of toxic gases.42 The results showed that significant amounts of NO can be captured by these highly flexible iron-based MOFs, and the adsorption capacities of these MOFs are obtained in the range of 1–2.5 mmol g−1, which is lower than the theoretical values (2.5–3.6 mmol g−1) owing to the low accessibility of the pores, preventing the diffusion and migration of NO to bind with the open metal site of iron of the MOF. Similar to the adsorption of NO, MIL-88(B) and amino-functionalized MIL-88(B) have also been applied in the adsorption of CO through the binding of CO with the open metal sites of the iron cations of the MOF.43 CO adsorption performance was also tested with Cu-BTC (BTC: 1,3,5-benzenetricarboxylate), where a remarkable amount of CO was adsorbed onto the open metal site of the MOFs through the electrostatic interaction between electron-deficient copper cations and the CO dipole.44 MOFs with open metal sites have been shown to behave as efficient adsorbents in toxic gas adsorption.
Adsorption of halogens.
Halogens are reactive chemicals and are widely used in many aspects of human life, including disinfection of drinking water, swimming pools, drug discovery, and the chemical industry. The high toxicity, corrosiveness and volatility of light halogens has seriously affected human health. MOFs have also displayed excellent adsorption capacity towards halogens, because both the open metal site and the linker of MOF are capable of interacting with halogens. The earliest study of Cl2 gas adsorption onto MOFs was reported by Britt and co-workers, who employed four isoreticular MOFs for Cl2 adsorption.45 Among those MOFs, amino-functionalized IRMOF-3 (constructed by using 2-aminoterephthalate as the organic linker and Cu cations as the metal centres) displayed the highest adsorption capacity owing to the formation of an ammonium chloride adduct between IRMOF-3 and chloride.45 Subsequently, several MOFs, such as ZIF-8, UiO-66-NH2, and MIL-53-NH2(Al), have also been tested for the adsorption of Cl2.46,47 However, exposing MOFs to high concentrations of light halogens will cause the collapse of the framework of the MOFs owing to the generation of corrosive acid attacking the nodes of the MOFs.47 In this case, the development of redox-active MOFs for the adsorption of halogens via the oxidation reaction between halogens and metal sites of MOFs is a promising strategy for preventing the generation of corrosive acid, resulting in the reversible adsorption of halogens. Tulchinsky et al. developed a redox-active MOFs constructed by using Co(II) ions as the metal centres and BTDD (bis(1H-1,2,3-triazolo[4,5-b],[4,5-i])dibenzo[1,4]dioxin) as the organic linkers for reversible capture and release of Cl2 and Br2.48 It was suggested that halogen was captured by the redox reaction between Cl2/Br2 and Co(II) to form Co(III)–Cl/Br bonds, as illustrated in Fig. 2a. The possible halogen desorption mechanism pathway is also displayed in Fig. 2b. That is, treating the oxidized MOFs at considerable high temperature can cause the reduction of Co(III) to Co(II) along with the release of halogen. Importantly, the reversible adsorption–desorption via the chemical reaction occurs with no significant loss of adsorption capacity or structural integrity. These works highlighted the advantages of MOFs for removing halogen gas.
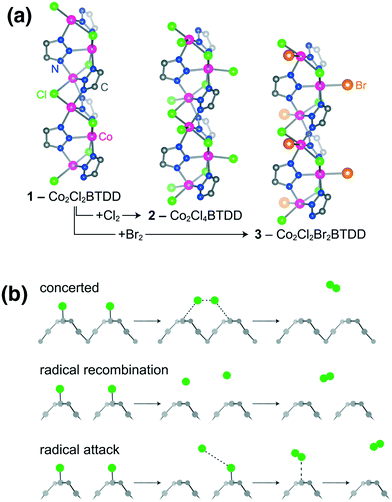 |
| Fig. 2 The possible adsorption mechanism of halogens on MOFs (a) and the possible desorption pathways (b). Reproduced with permission from ref. 48. | |
However, the main drawback to the application of MOFs with open metal sites in gas adsorption is that water in the air may significantly hamper the adsorption capacity of MOFs as a result of the irreversible coordination bonds between molecular water and the open metal sites of MOF. It is important to fully understand how water affects the adsorption performance of MOFs. In this regard, Mavrandonakis and co-workers carried out a theoretical study of the interaction of various gases (CO, NO, OCS, SO2, NO, NO2, N2O, NH3, PH3) with Cu3(BTC)2,49 and the calculated adsorption energies of the toxic gases onto the open Cu(II) metal site of Cu3(BTC)2 follow this order: NH3 > H2O > PH3 > H2S > SO2 > CO ∼ OCS ∼ CO2 ∼ NyOx > N2 > O2. It can be concluded that only NH3 is adsorbed more strongly than water by Cu3(BTC)2, which means that water has the advantage of preferentially adsorbing onto open metal sites in Cu3(BTC)2 as compared to CO, NO, OCS, SO2, NO, NO2, N2O, PH3. These results are well consistent with the experimental observations reported by Peterson et al.50 Overall, these works present useful information for exploring MOF-based materials for toxic gas removal. However, their stability is still very low, since the strong interaction between these toxic gases and the open metal sites of MOFs cause the decomposition of the framework with a great loss of porosity. Therefore, further study for improving the stability of these adsorbents should be carried out as stability towards water is a major requirement for practical application.51
Many strategies, such as tuning the hydrophobicity/hydrophilicity properties or steric factors, have been developed for improving the stability of MOFs used under high humidity conditions. The hydrophobic MOFs can prevent the H2O approaching the cluster or MOFs, hindering the hydrolysis reaction. Similarly, steric factors can also reduce the hydrolysis reaction kinetics by increasing the activation energy barriers. Increasing the coordination number between metal cations and organic linkers can significantly enhance the stability of MOFs in the presence of water by creating a crowding effect around the metal nodes. Subsequently, a series of zirconium-based MOFs were prepared via a solvothermal method, and the Zr atoms in the nodes of the MOFs exhibit a seven-coordination mode with the oxygen of the organic linkers. The as-obtained MOFs are highly stable when they are exposed to water and have a remarkably high thermal stability up to 500 °C.52
Except for optimizing the framework stability and tuning the hydrophobicity/hydrophilicity properties of MOFs, creating new pore and binding sites by coupling MOFs with other functional materials is also suggested to improve the physical and chemical adsorption capacity of MOFs.53–55 Two porous MOFs with open metal sites, Co-MOF(Co2(C8H2O6)(H2O)2)8H2O and Ni-MOF (Ni2(C8H2O6)(H2O)2)8H2O, were synthesized by Morris and co-workers and employed for the adsorption and delivery of NO.56 It was found that molecular NO can strongly bind with the open metal sites within the wall of the MOFs. Importantly, all of the captured NO is completely released when the absorbent is exposed to water moisture. Bandosz and Petit developed several MOF–graphite oxide (GO) composites for NH3, H2S and NO2 adsorption under ambient conditions.55 After combing the MOFs with GO, a new pore space in the interface between the MOF and the GO is created owing to the strong interaction between the metallic centres of the MOF and GO oxygen groups. Such a new structural property greatly enhances the physical adsorption capacity of MOF–GO composites towards toxic gases in comparison with parent MOFs. Besides the physical adsorption, chemical adsorption is also observed on these composites via acid–base and hydrogen bonding interactions. It is well known that open metal sites in MOFs are electron-deficient centres, therefore, not only can electron-rich toxic gases contact with the open metal sites of MOFs, but the sites can also be utilized for grafting functionality for chemical adsorption of toxic gases. For example, a post-synthetic method was developed by Rosseinsky and co-workers to anchor 4-(methylamino)-pyridine onto open metal sites of MOFs to create secondary amine functionalities, which are capable of binding NO to produce N-diazeniumdiolates.57 The adsorption capacity towards NO is drastically increased in comparison with the parent MOF.
However, the limited number of open metal sites of MOFs and the repulsion effect between adsorbed molecules led to the low adsorption capacity, which hinders MOFs in flue gas purification applications. Beside open metal sites, considerable effort has also been contributed to anchoring new binding sites within the walls of MOFs. MOFs bearing functionality are also widely used for improving the adsorption capacity for toxic gases. Amino, one of the most popular functionalities, has been widely used as a powerful functionality to tune the adsorption capacity of MOFs by forming H-bonds between amino groups and the adsorbate. Recently, Janiak and co-workers also introduced a urea R–NH–CO–NH–R functional group within a zinc-based MOF for the adsorption of SO2 and NH3.58 It was found that the urea-functionalized MOF exhibits an uptake of 10.9 mmol g−1 toward SO2 by H-bonding interaction. To conclude, this section briefly summarizes the recent studies on the chemical adsorption of various toxic gases through chemical bonding by MOFs. MOFs display high reactive adsorption capacity towards toxic gases owing to their high surface area, abundant functionalities and rich open metal sites. Several types of chemical bonds can be formed between toxic gases and MOFs. The interaction forces can be divided into the following four aspects according to the reported works: (1) adsorbate coordinates with open metal sites (coordinatively unsaturated metal cations); (2) adsorbate adsorbed via hydrogen bonding; (3) adsorbate adsorbed via acid–base interaction; (4) the adsorbates in the framework of MOF.
2.2 Adsorption of volatile organic compounds with MOF-based nanomaterials
VOCs are a major group of organic air pollutants with high vapor pressures, released from burning of fuel, or emitted from various industries, gas fields and diesel exhausts. The major classes of VOCs are oxygenated volatile organic compounds (OVOCs), aromatic compounds, alkanes/alkenes, and N- or S-containing organic compounds. Most VOCs can react with nitrogen oxides to form ground-level ozone, aerosol, smog and particulate matter owing to their high reactivity.59 Development of porous materials for efficient capturing and removal of VOCs is of significant importance and a very hot topic in both environmental sciences and human life.60–62 Up to now, activated carbon, activated carbon fiber and zeolites have been widely employed for the adsorption of VOCs. Recently, MOF-based nanomaterials have been proved as important alternatives to those traditional porous materials owing to their tunable pore size and structure, flexible synthetic methods and various functionalities of MOFs to tune the adsorption ability. In recent years, the vast majority of works have been contributed to the adsorption of VOCs onto MOFs. For example, Zn4O(L)3 (L: 4,4′-((4-(tert-butyl)-1,2-phenylene)-bis(oxy))dibenzoate), MIL-53(Al), MIL-47(V), and MIL-53(Fe), ZIF-8(Zn) and UiO-66(Zr) have been successfully fabricated for the adsorption of various VOCs.63–68
Adsorption of OVOCs.
Atmospheric OVOCs, such as aldehydes, ketones, alcohols, and ethers, originate from the incomplete combustion of fuels, evaporation of oxygenated solvents or fuels, emissions from natural activities, and the atmospheric oxidation of hydrocarbons. The oxidation of hydrocarbons existing in the atmosphere is the most important source of OVOCs. Take the oxidation of CH4 as an example; the chemical reaction between CH4 and hydroxyl radicals mainly formed by the photolysis of ozone gives rise to methanol, formic acid, or formaldehyde as products. The oxidation of larger hydrocarbons may result in the formation of a variety of OVOCs. It is well known that OVOCs are generally more reactive than the other types of VOCs owing to their high polarity, making a significant contribution to ozone and aerosol formation and posing a serious health risk. It should be pointed out that O3 as a major pollutant in the atmosphere has very high oxidising potential; it is mainly generated by atmospheric photochemical reactions. However, owing to the limited study in this area, the adsorption of O3 with MOFs will not be discussed in this review. In contrast, the adsorption of OVOC pollutants has been significantly studied. Considering that most VOCs are heteroatom-containing molecules, van der Waals interactions, cation–π bonding interaction between open metal sites of MOFs and functional groups of OVOCs, and polar–polar interaction are expected to enhance the adsorption capacity of MOFs. Aldehydes, particularly formaldehyde, are common toxic vapours that need to be controlled at ppb level. Recently, the development of polar MOFs for formaldehyde detection has been attracting considerable attention because the polar formaldehyde prefers to be adsorbed onto polar MOFs rather than nonpolar adsorbents. Jiang et al. have reported a novel Cu-MOF synthesized based on Cu(II) coordinated with 5-aminoisophthalic acid; such Cu-MOFs displayed excellent adsorption performance towards formaldehyde owing to the large number of open metal sites and amine groups anchored within Cu-MOFs.69
It should be pointed out that formaldehyde is extremely toxic to humans; the maximum acceptable formaldehyde concentration is 0.1 ppb in 30 min.70 Currently available porous materials usually display very low sensitivity for formaldehyde adsorption. Therefore, it is very important but challenging to develop a sensitive adsorbent towards formaldehyde at the ppb level. MOF featuring both open metal sites and uncoordinated amino groups may offer available adsorption sites for formaldehyde at ppb level. In this case, MOF-5 was employed as an adsorbent for detecting formaldehyde at very low ppb levels (0.012 to 0.048 ppb), and the performance was much better than that of graphitized carbon black and TenaxTA,71 showing the great potential for practical applications.
As mentioned above, most MOFs display poor stability towards water. Therefore, Zheng and co-workers developed a diamine-appended MOF for improving formaldehyde adsorption in highly humid conditions. The adsorption capacity of diamine-appended MOFs increased with the increase of amino groups to a certain concentration. The maximum adsorption capacity reached 5.49 mmol g−1. Importantly, the diamine-appended MOFs also greatly improved the recyclability and water resistibility owing to the reversible interaction between formaldehyde and amino groups. The lone pair of the amine group interacts with the carbonyl carbon of formaldehyde, followed by the proton exchange process and dehydration to form imine. This reaction process is reversible, leading to recoverable MOFs. In a reaction analogous to formaldehyde adsorption onto diamine-appended MOFs, the produced imine can also be detected in the adsorption of acetaldehyde with amino groups of NH2-MIL-101(Al) and NH2-MIL-53(Al).72 Meanwhile, Cu-based MOF-199 and Zr-based MOFs were also exploited for the detection of aldehydes ranging from C1 to C5 carbonyl compounds.73 These studies suggest that MOFs are a very sensitive adsorbate for detecting aldehydes at low concentration, showing great potential in the design of in-door aldehyde adsorbents. In addition to aldehydes, the adsorption of alcohols, ketones and ethers has also been studied extensively. Trens et al. experimentally and computationally studied the adsorption behaviours of acetone vapor on Cu-BTC. Two main adsorption interactions, van der Waals interaction between cavities of MOFs and acetone as well as the interaction between the open metal site (Cu(II)) of Cu-BTC and the carbonyl functional groups of acetone, were found in the adsorption of acetone.74 Lin et al. reported the adsorption of polar acetone with MIL-101(Cr) and MOF-177, and the adsorption capacities of MOF-177 (∼589 mg g−1) and MIL-101(Cr) (∼1291 mg g−1) are comparable to or much higher than those of commercially available adsorbents such as Y-zeolite, ACFC (activated carbon fibre cloth) and PCH (porous clay heterostructures).75,76 Considerable adsorption performance was also observed for methanol, ethyl acetate and butanone onto MIL-101(Cr).77,78 The theoretical and experimental results suggested that MOFs have higher affinity for polar OVOCs owing to the interaction between the OVOCs and the MOFs.
Adsorption of N- or S-containing organic compounds.
N- or S-containing organic compounds are specially discussed in this review because they have long been associated with nuisance odors. These odorous vapors are emitted from both fossil fuels and human activity. The discharge of N- or S-containing organic compounds causes various environmental hazards and health effects. Thus, the efficient control and treatment of N- or S-containing organic compounds is very important. Recent studies have shown that selective capturing of these odorants with MOFs is very attractive and effective approach. According to Pearson's HSAB theory, MOFs with hard Lewis acid sites or soft Lewis acid sites have a preference to interact with N- or S-containing organic compounds through acid–base interaction. It should be noted that the soft bases, i.e., S-containing organic compounds, preferentially interact with soft Lewis acid sites (Cu2+, Zn2+, Co2+ and Ni2+), while hard bases, i.e. N-containing organic compounds, prefer to interact with hard Lewis acid sites (Cr3+, Al3+, Fe3+). Therefore, the type of open metal sites is an important point for selective adsorption of N- or S-containing organic compounds. For example, Cu-BTC and MOF-199 displayed much higher capacities for the adsorption of tetrahydrothiophene than those of commercial activated carbons.45,79 Navarro et al. also reported the adsorption of thiophene onto Zn- and Ni-based MOFs with very high capacities. The open metal sites of the MOFs were demonstrated to be the most important binding sites for tetrahydrothiophene and thiophene adsorption.80 In contrast, Yan et al. reported MIL-101(Cr) for the effective adsorption of six VOCs with different functional groups and polarities.78 The authors found that MIL-101(Cr) displayed the strongest affinity to n-butylamine. Similar to the adsorption of toxic gases with high polarity, n-butylamine can serve as an electron donor, and the open metal sites of MIL-101(Cr) can be considered as electron acceptors during the adsorption process, resulting in the strong electron donor–acceptor interactions. The adsorption of N- or S-containing organic compounds has been mainly explained with acid–base interaction owing to the electron rich N or S atom of the adsorbate and the electron-deficient metal sites of the adsorbent. In addition to the acid–base interaction, the specific interactions such as H-bonding and π-complexation as well as synthesis of hydrophobic MOFs were also observed to enhance the adsorption capacity of pyridine and benzothiophene.81,82 Recently, Zhong and co-workers demonstrated that the adsorption capacity of UiO-66 towards pyridine increased with increasing amino groups in UiO-66 owing to the increased H-bonding between the amino groups of UiO-66 and the N atom of pryidine.82 Navarro and co-workers synthesized a highly hydrophobic MOF for the capture of harmful VOCs by using a pyrazolate-based organic linker and nickel cation as a connecting centre.83 MOFs with trifluoroalkyl incorporated exhibit a significant increase of adsorption capacity towards diethylsulfide owing to the super hydrophobic property of MOFs. However, most of the reported studies have been focused on the adsorption of inorganic pollutants, such as NO2, NO, NH3, H2S, and SO2. Only a few studies have been conducted on the adsorption of gaseous N- or S-containing organic compounds. Hopefully, attention will be continuously paid to this research area.
Adsorption of halogenated organic compounds.
In addition to N- or S-containing organic compounds, halogenated organic compounds, as a large class of heteroatom-containing organic compounds containing halogens such as fluorine, chlorine, or iodine, have also received significant attention. This class of compounds is extremely important in the production of insecticides, herbicides, and fungicides as the starting materials or additives.84 The inefficient disposal of these halogenated organic compounds causes harmful effects to our ecosystem and health.85 Therefore, the adsorption and enrichment of halogenated organic compounds is highly important and demanded. It is reported that MIL-101 with a large number of open metal sites display very high affinity for dichloromethane as a result of a strong interaction between the unsaturated metal sites of MIL-101 as an electron acceptor and the dichloromethane as an electron donor.78 Li et al. also studied the adsorption of 1,2-dichloroethane by MIL-101, and the adsorption capacity was measured to be 19 mmol g−1, much higher than those of zeolite, activated carbon and carbon nanotubes.86 It was found that the interaction between the open metal site of MIL-101 and 1,2-dichloroethane is much stronger than the interaction formed between the space of the framework and 1,2-dichloroethane. Bullot and co-workers also the reported the adsorption of 1,2-dichlorobenzene and 1,2,4-trichlorobenzene by using MIL-101 as the adsorbent.87 It can be imagined that a strong π–π interaction between the MOFs and the adsorbate may play a vital role in the adsorption of halogenated aromatic compounds. In this regard, MOFs appear as very promising adsorbents for halogenated aromatic compounds. However, the presence of water vapor significantly reduces the adsorption capacity of MOFs towards halogenated organic compounds since water is considered as a hard base, which strongly interacts with the open metal sites of MOFs through acid–base interaction.88 Therefore, it is important to reduce the hardness of metal sites or change the hydrophobicity of MOFs to make water-resistant MOFs. On the other hand, halogenated aromatic compounds are highly toxic. It is widely accepted that halogens are electron-withdrawing groups in halogenated aromatic compounds. Therefore, the selective adsorption of these highly toxic halogenated aromatic compounds is very important. In this regard, Zhao et al. reported a highly hydrophobic MOF (N-coordinated UiO-66(Zr) for the adsorption of chlorobenzene over water. It was found that the hardness of Zr in the MOF is decreased by the electron donation of N to Zr, along with the enhanced hydrophobicity of the MOF, resulting in the dramatic reduction of the water adsorption capacity. Undoubtedly, the adsorption of chlorobenzene is significantly enhanced by N-coordinated UiO-66(Zr) as compared to unmodified MOFs.89 This study may also shed light on the selective adsorption of halogenated aromatic compounds over parent aromatic compounds, in which MOFs with soft Lewis acid sites may display preferable adsorption of halogenated aromatic compounds over aromatic compounds owing to the reduced electron density of the benzene rings.
Adsorption of aromatics.
Aromatic pollutants including benzene, benzene homologues and other polycyclic aromatic hydrocarbons with ring structure, widely found in effluents and the atmosphere, have been reported to have numerous potential negative health effects. Aromatic pollutants, as ubiquitous pollutants, are very stable and need a long time to be degraded. The adsorption technology with porous materials has attracted significant attention owing to the high control efficiency and green process for removing aromatic pollutants. As is well known, a large number of MOFs have a phenyl ring in the wall of their structure and open metal sites in the node, which benefits VOCs adsorption through π–π interaction and cation–π interaction between aromatic pollutants and MOFs. Furthermore, the ultrahigh surface area as well as the unique pore structure also play positive role in the pore/size selective adsorption of aromatic pollutants. Taking MIL-101(Cr) as an example, constructed from Cr cations as connecting centers and 1,4-dicarboxybenzene as the organic linker, it has a large surface area and numerous mesoporous cages connected with microporous windows,90 holding great potential for aromatic pollutant adsorption. Indeed, MIL-101 has been proven to be effective in the adsorption of a wide range of VOCs with different functionalities and polarities, such as benzene, ethylbenzene, and xylenes owing to the π–π interaction and cation–π interaction as well as the adequate pore/cavities and ultrahigh surface area.75,88,91 Moreover, the size and shape of VOCs also affect their adsorption on MIL-101(Cr). In addition, MIL-101(Cr) outperforms other commercially available inorganic oxide adsorbents in the adsorption of benzene, ethylbenzene and xylenes. Taking benzene adsorption as an example, the adsorption capacity of MIL-101 (∼13 mmol g−1) is much higher than that of activated carbon (8.0 mmol g−1), NDA-201 resin (5.2 mmol g−1), HZSM-5 zeolite (1.9 mmol g−1) and SBA-15 (3.0 mmol g−1), making MIL-101(Cr) an ideal adsorbent for practical application in VOC adsorption.75,92,93 In addition to MIL-101(Cr), HKUST-1, MOF-177, MIL-47, Al-MIL-53, Zn(bpb) (1,4-(4-bispyrazolyl)benzene) and Ni(bpb) have also been studied for the adsorption of benzene and benzene homologues, and these MOFs with a phenyl ring all displayed considerable removal efficiencies.76,80,94–96 Undoubtedly, the reactive adsorption by forming specific interactions between the adsorbent and the VOCs is vital for selective adsorption of VOCs. Differing from the selective adsorption of toxic gases, the size and shape of VOCs greatly affect their adsorption on MIL-101(Cr) and has been significantly studied.
Moreover, owing to the size/shape exclusion, some adsorbates with large size and unique shape are prevented from diffusing into the pores of an adsorbent while adsorbates with specific size or shape are allowed to enter the pores. MIL-101(Cr) has two types of cages (29 and 34 Å in diameter) with two different windows (12 and 16 Å); Yang and co-workers applied MIL-101(Cr) to the selective adsorption of ethylbenzene and p-xylene from o-xylene and m-xylene.75 In their study, ethylbenzene and p-xylene were adsorbed within the pores of MIL-101(Cr) while o-xylene and m-xylene are prevented from diffusing into the pores of MIL-101(Cr) owing to the steric effect. In addition, a monoclinic MOF (Zn3(bdc)3(H2O)3(DMF)4) was also applied in the adsorption of xylene and displayed high selectivity towards p-xylene over other xylene isomers owing to the fast diffusion and migration rate into the pores of the MOFs. However, another zinc-based MOF (MOF-5) showed preferable adsorption of ethylbenzene over xylene isomers.97 Titanium-based MOFs, Ti8O8(OH)4(NH2-BDC)6 and Ti8O8(OH)4(BDC)6, and an aluminum-based MOF, (Al8(OH)4–(OCH3)8(NH2-BDC)6), with two unique types of cage are all able to separate p-xylene from a mixture of xylene isomers.98 A zinc-based MOF (Zn(ip)(bpa)) (ip: isophthalate; bpa: 1,4-bis(4-pyridyl)acetylene) with a one dimension pore channel was synthesized for selective adsorption of benzene at room temperature.99 In the case of polycyclic aromatic pollutant separation, the size/shape exclusion of MOFs may play a vital role. Currently, promising results for polycyclic aromatic pollutant adsorption have been obtained in the liquid phase,100,101 while the separation of polycyclic aromatic hydrocarbons in the gas phase using MOFs has not been widely reported, which provides a strong motivation for studying this topic. These studies suggest that the pore-filling mechanism by adjusting the nature and size of pores in the framework as well as the π–π interaction and cation–π interaction mechanisms show great potential for selective adsorption of aromatic pollutants with certain size and shape.
Adsorption of aliphatic hydrocarbons.
Aliphatic hydrocarbons composed of carbon and hydrogen are mainly emitted from petroleum or natural sources. The oxidation of aliphatic hydrocarbons with hydroxyl radicals in the atmosphere is the most important source of OVOCs. Aliphatic hydrocarbons can also be directly involved in the formation of aerosols. More importantly, most aliphatic hydrocarbons are flammable. Therefore, the adsorption and separation of aliphatic hydrocarbons is significantly important from environmental and economic perspectives. Aliphatic hydrocarbons can be saturated or unsaturated, straight chain, branched chain or cyclic, and compositions among aliphatic hydrocarbons vary widely. The development of MOFs that could selectively adsorb a certain aliphatic hydrocarbon is attracting great attention. Indeed, a great number of MOFs have been applied for the adsorption and separation of aliphatic hydrocarbons.102,103 In general, MOFs with high surface area preferentially adsorb the short chain hydrocarbons owing to van der Waals interactions.104–106 Increasing the chain length of pollutants may cause a decrease of the uptake capacity in most cases.103,107 The pore shape as well as the open metal sites of MOFs provide a novel platform for separating alkenes from alkanes and branched alkanes from linear alkanes.108–110 For example, Chen et al. used Zn-BTM (Zn ions reacted with bis(5-methyl-1H-1,2,4-triazol-3-yl)methane) for 1,3-butadiene separation.111 Zn-BTM has a quasi-discrete pore structure, in which the isolated cavity is used for controlling C4H6 conformation and the continuous channels are applied for diffusion of other C4 hydrocarbons. Thus, Zn-BTM eluted 1,3-butadiene first, then butane, butene, and isobutene, finally realizing the C4H6 purification. In addition, the efficient adsorption of C4–C6 alkane isomers by IRMOF-1 and IRMOF-6 was investigated by Liu and co-workers. They found that the alkane isomers are firstly adsorbed onto Zn4O, followed by filling the pore of the MOFs with linear alkanes, while it is difficult for branched alkanes to enter to the pores of the MOFs.108 Considerable work has also been conducted on methane/acetylene separation by utilizing MOFs with open metal sites. For example, a novel MOF, Cu2(EBTC) (EBTC = 1,1′-ethynebenzene-3,3′,5,5′-tetracarboxylate), displays an acetylene uptake capacity of 7.14 mmol g−1,112 which is significantly higher than that of Cu-BTC. The enhanced adsorption capacity of Cu2(EBTC) can be attributed to the interaction between the open metal sites of Cu2+ in Cu2(EBTC) and acetylene, a weak interaction between the C–C triple bond and the organic linker, as well as a pore filling effect. It seems that the open metal sites of MOFs hold great potential for the adsorption and separation of alkene from alkanes. The double alkene bond preferentially interacts with the electron-deficient open metal sites of the MOF through the cation–π interaction, leading to the selective adsorption of alkenes from alkanes.113 It should be noted that aliphatic hydrocarbons composed of hydrogen and carbon display relatively low reactive as compared with other VOCs with heteroatoms in general, but vary in shape and size. Therefore, the adsorption and separation of aliphatic hydrocarbons is mainly based on the molecular sieving effect of MOFs and partially based on special interactions between MOFs and the double/triple C–C bonds of aliphatic hydrocarbons. The current achievements shed light on the development of new MOFs with unique structures or chemical properties for selective adsorption of aliphatic hydrocarbons.
Adsorption of warfare agents.
Warfare agents, as a special family of VOCs, are extremely toxic. Therefore, the development of efficient adsorbents for capturing and degradation of those harmful molecules is of significant importance. In this context, a few efforts have been dedicated to this research area.114–116 Roy et al. reported the efficient adsorption and degradation of sulfur mustard, 2-chloroethyl ethyl sulfide (CEES) and bis(2-cholorethyl) sulfide with Cu-BTC.117 However, the processes of removing warfare agent with MOF is different from the adsorption of aromatic pollutants. In the case of warfare adsorption, the removal of warfare agents is usually carried out under high humidity and chemical reactions occurs in most cases. Therefore, the development of highly hydrophobic MOFs is a promising strategy. In this context, zinc-based MOFs (constructed by using zinc ions as the connecting centers and 3,5-dimethyl-4-carboxypyrazolato as the organic centers), with a similar structure to MOF-5, was proven to be effective for the removal of sarin nerve gas (diisopropyl fluorophosphate) and vesicant mustard simulant (diethyl sulfide).116 The as-obtained MOFs displayed high VOC-H2O partition coefficients owing to their high hydrophobicity, and would provide high adsorption efficiency for sarin under humid conditions. Up to now, limited work has been conducted on chemical warfare agent adsorption and degradation, probably owing to the low stability of MOFs under humid conditions. However, MOFs offer incredible opportunities for fine tuning their chemical and physical properties. The specific functional groups and pore structure can be tailored. There is still much room for improvement of the application potential in warfare agent removal.
This section comprehensively reviews the recent progress on VOC adsorption, and a vast number of MOFs have been designed and employed to efficiently remove VOCs from air, since MOFs surpass other porous sorbents in terms of adsorption capacity and selectivity because of their high surface area, various pore structures and high tunability. Several interactions have been observed for the enhanced adsorption performance, such as cation–π interaction, π–π interaction/stacking, hydrophobic interactions, H-bonding, acid–base interaction, and pore/size-selective adsorption. Understanding those adsorption mechanisms may shed light on establishing optimal design strategies that could be applied for the removal of a broad range of VOCs under harsh conditions. The versatility in the characteristics of MOFs allows the easy modification of functional groups and tuning of hydrophobic/hydrophilic properties as well as pore structure, holding great potential for their application in real life.
2.3 Particulate matter (PM) adsorption with MOFs
PMs containing inorganic substances and organic compounds, as a major air pollution source, have emerged as one of the most serious environmental issues, causing significant harm to human health. PM is highly polar owing to the existence of metal cations, water and organic compounds.118 There are many functional groups on the surface of PM, such as C–N, –NO3, –SO3H, and C–O, resulting in the formation of charged PM.119 Therefore, the development of air-purification materials with electric charges is beneficial for PM capture. Similar to PM, the composition of MOFs involves an inorganic oxide cluster and an organic linker; the coordinated unsaturated metal cations and defects make MOFs charged, and this structure has the advantage of capturing the polarized PMs via dipole–dipole or electrostatic interactions between MOFs and PM. The first report related to MOFs applied in PM purification was contributed by Wang's group.120 By using a versatile templated freeze-drying protocol method, ZIF-8@SA (SA represents sodium alginate) hollow tubes with different ZIF-8 loading amounts were fabricated.120 The as-prepared cellular ZIF-8@SA was employed as a PM filter for PM capture (Fig. 3a). It displayed superior efficiency for PM removal owing to the hollow structure giving abundant interaction pathways, with the high positive charge of ZIF-8 and the high polarity of the PM affording 92 ± 2.2% PM2.5 removal efficiency and 95 ± 2.6% for PM10, as displayed in Fig. 3b. The removal efficiency of ZIF-8@SA is much higher than that of SA-based hollow tubes (PM2.5 = 31 ± 1.2%; PM10 = 33 ± 1.4%). To further increase the removal efficiency, Kim and co-workers developed hierarchical MOFs by assembling two-dimensional Zn-based zeolite imidazole frameworks, and comparable removal efficiency was observed for PM2.5 (atmospheric particulate matter with a diameter of 2.5 micrometers or less) and slightly enhanced removal efficiency was obtained for PM1.0.121 Undoubtedly, those works open a new way for the application of MOFs in PM filtration.
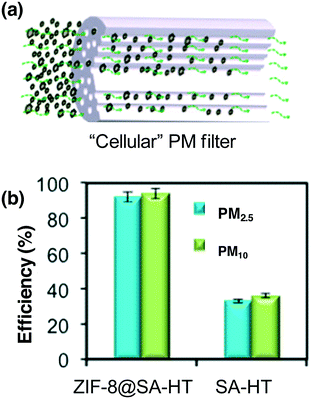 |
| Fig. 3 (a) Schematic illustration of a “cellular” PM filter. (b) The removal efficiency of the “cellular” PM filter as well as a reference sample. Reproduced with permission from ref. 120. | |
For practical application, it is important to develop a novel and cost-effective method to scale up MOF-based air-purification materials in mass production as MOFs show great potential in PM filtration. Recently, Wang and co-workers developed a roll-to-roll fabrication method for mass production of MOF-based PM filters by using commercially available substrates (plastic mesh, metal mesh, nonwoven fabric and glass cloth) as hard templates.122 After immersing the substrate into the MOF precursor containing polyethylene glycol as the initiator, the treated substrate is rolled at 80 °C at a speed of 15 rpm several times, then ZIF-8 is coated with the substrates after the rolling back-and-forth, as evidenced by SEM and PXRD characterization. Importantly, such novel fabrication method can be easily scaled up, and the loading number of the MOFs can be controlled by varying the rolling cycles. This fabrication method can not only be applied in synthesizing ZIF-8-based filters but can also be used for synthesis of Co-ZIF-67- and Ni-ZIF-67-based filters. In addition, the porosity and surface area were tested by N2 sorption. The surface area of ZIF-8@plastic mesh with seven rolling cycles was measured to be 234 m2 g−1, and all fabricated filters showed high removal efficiency towards PM pollution over a wide range of temperatures from 80 to 300 °C. For practical application, an experiment using as-obtained ZIF-8@plastic mesh filters is carried out in simulated living environment conditions; the filters exhibited excellent removal efficiency for PM2.5 (56.3 ± 1.6%) and PM10 (atmospheric particulate matter with a diameter of 10 micrometers or less) (58.4 ± 2.1%) in comparison with bare plastic mesh (14.2 ± 0.4% for PM2.5 and 17.4 ± 0.8% for PM10) at room temperature. The obtained ZIF-8@plastic mesh filters also display high durability; after cleaning by brush and water and ethanol washing, the removal efficiency of the recycled filter was almost unchanged after three cycles. The easy preparation method for mass production combined with the high efficiency and stability of those filters for PM removal are very promising for real application in PM pollution control. More recently, Yang and co-workers reported a hot-pressing method to coat an imidazole-based Zn(II) MOF onto various substrates, including carbon fiber, cloth, glass cloth, plastic mesh and melamine foam, which displayed high removal efficiency for real polluted air purification.123
Nowadays, the development of advanced porous materials for PM purification has become a hot topic, especially in developing countries as PM is a major air pollution source. Recent studies are mainly focused on PM10 and PM2.5 captured by using carbon- or polymer-based purification materials. However, little attention has been paid to the capture of PM1.0 (atmospheric particulate matter with a diameter of 1 micrometer or less). Owing to the high toxicity of PM1.0 and small particle size, it may enter into human organs, particularly in the case of lung. The capture of PM1.0 should be the primary target in the near future. In this case, the development of MOF-based air purification materials to efficiently capture PMs with a large range of sizes is important. The strategy idea is to synthesize MOFs with a hierarchical pore structure, where the mesopore and micropore can be utilized to capture PM1.0. On the other hand, owing to the high polarity of PM, as discussed above, MOFs with a large number of functional groups and open metal sites offer great opportunities to capture PM with a broad size distribution via dipole–dipole interaction or electrostatic interactions.
3. Modification of MOF-based nanomaterials for enhanced selective VOC adsorption capacity
Gaseous pollutants cause health problems that vary significantly from high toxic pollutants to those with unknown health effects. Some of them may cause immediate symptoms after the exposure to a certain level of concentration.60 Therefore, the selective adsorption of highly toxic gaseous pollutants is an ongoing challenge. The open metal sites, a large number of functionalities and the tailorable framework of MOFs offer great opportunities for selective adsorption of toxic gaseous pollutants and VOCs. It is clear that targeting a particular functional group on MOFs is critically important for selective adsorption instead of optimizing the surface area alone. Recently, considerable efforts have been dedicated to the modification of MOFs through node engineering and organic linker design strategies.31,32,124,125 For example, according to the size and composition of VOCs, several modification strategies, such as introducing new functionality to the linker and open metal sites or within the cavity of MOFs, and tuning the electron density of nodes at the atomic level to enhance the adsorption capacity of MOFs towards targeted gaseous pollutants, are reviewed and summarized in detail in the following.
3.1 Post-modification via grafting functional groups on open metal sites of MOFs
The coordinatively unsaturated metal nodes within MOFs provide great opportunities for grafting functional groups. Taking MIL-101(Cr) as an example, ethylene diamine was grafted on the coordinatively unsaturated chromium clusters after removing bound solvent molecules under vacuum conditions. The free amino group was successfully introduced within the pores of the MOFs, leading to binding the gaseous pollutant.126 Post-modification via grafting functional groups on open metal sites of MOFs has been demonstrated as a promising strategy for tuning the hydrophilic/hydrophobic properties of MOFs. It is widely accepted that the hydrophilic/hydrophobic properties and the thermal stability of an adsorbent significantly affect the adsorption performance towards specific adsorbates. Generally, VOCs are hydrophobic molecules, but most MOFs with high thermal stability are hydrophilic, hindering the efficient adsorption of hydrophobic VOCs onto MOFs.127–129 In this case, a novel mechanochemical strategy is proposed to fabricate a dopamine-modified UiO-66(Zr) (noted as M-UiO-66(Zr-N3.0)) with the aim of enhancing chlorobenzene adsorption.89 The authors claimed that the modification of MOFs with dopamine significantly weakened the interaction between the electrophilic metal sites of the MOFs and nucleophilic H2O, leading to the generation of hydrophobic MOFs as evidenced by the results of the H2O adsorption test showing that the H2O adsorption uptake of M-UiO-66(Zr-N3.0) decreased as compared with their parent MOFs. This hydrophobic MOFs display a significant enhanced adsorption capacity for acetaldehyde (9.42 mmol g−1) and chlorobenzene (4.94 mmol g−1) as compared with the unmodified MOFs. The stability of M-UiO-66(Zr-N3.0) was also investigated by assessing the recycling performance over a wide range of temperature and pressure, and M-UiO-66(Zr-N3.0) can be repeatedly used for four cycles with a slightly decrease in its adsorption capacity, indicating the outstanding reversibility of VOC adsorption. Such dopamine-modified MOFs exhibiting remarkable competitive adsorption towards VOCs against H2O emerge as a promising adsorbent for VOCs under highly humid conditions, as H2O easily decreased the adsorption capacity of MOFs towards VOCs by occupying the reactive sites and collapsing the structure of the MOFs.
There are many other advantages for the modification of coordinatively unsaturated metal nodes of MOFs with functional groups, such as changing the interactions with gaseous pollutants, increasing the binding sites, tuning the hydrophilic/hydrophobic properties of MOFs, preventing the adsorption of H2O on coordinatively unsaturated metal nodes, and enhancing the stability of MOFs. Therefore, this strategy is specially recommended for adsorption of toxic gases under humid conditions.
3.2 Post-modification via grafting functional groups on organic linkers of MOFs
As elucidated above, MOFs are highly porous materials based on organic molecules as linkers, possessing high surface area and adjustable skeletons. These advantages provide unprecedented possibilities for post-synthetically grafting functional groups on organic linkers to introduce new functionalities for chemical adsorption of VOCs.
Gao and co-workers reported a post-modification method for the synthesis of MOFs with a free binding site by using a multi-dentated molecule (2,2′-bipyridyl-5,5′-dicarboxylate (bpydc)) as an organic linker to bridge the Zr6O4(OH)4 cluster (UiO-67-bpy). Then an N-methylated MOF (UiO-67-dmbpy) was prepared by stirring UiO-67-bpy and methyl trifluoromethanesulfonate (TfOMe) under mild and neat conditions, as shown in Fig. 4.130 The surface area of N-methylated MOFs decreased significantly after N-methylation because the trifluoromethanesulfonate anions and methyl groups occupied the pores of the MOFs, confirming the successful N-methylation of UiO-67-bpy. The adsorption properties of UiO-67-dmbpy were tested with a wide range of VOCs. N-Methylated MOFs displayed a significantly enhanced adsorption capacity for butylamine as compared with unmodified MOFs, suggesting that N-methylated MOFs show a higher affinity than that of the parent MOFs towards alkylamine adsorption. The authors claimed that the enhanced affinity is essentially caused by the interactions between the electron-deficient bipyridinium moiety and the electron-rich adsorbate. Moreover, the adsorbed adsorbate can be removed by exposing the adsorbent to air or by heating or washing with common solvents, and the performance for capturing alkylamines was also same as the first cycle, suggesting the good recyclability of N-methylated MOFs. In addition, the introduction of an electron-donating group, such as –OH, –NH2, –COOH, –NO2, or SO3H, within MOFs is also reported to enhance the adsorption performance of MOFs for gaseous pollutants via H-bonding and acid–base interaction. Moreover, using strong-field ligands such as an azolate-terminated organic ligand to build MOFs, and pre- or post-synthetic incorporation of alkane or fluoroalkane in the organic linker is also reported for tuning the hydrophilic/hydrophobic properties of MOFs against water vapor and introducing new binding sites for gaseous pollutant adsorption, leading to the improved adsorption performance of modified MOFs.83,131–133
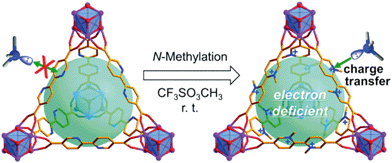 |
| Fig. 4 Post-modification by N-methylation for optimizing the adsorption capacity towards alkylamines. Reproduced with permission from ref. 130. | |
3.3 Post-modification via incorporating ionic elements, polyoxometalates (POMs) and other functional materials within MOFs
POMs are nanosized metal-oxide macroanions with 3-dimensional frameworks that consist of a few transition metal oxyanions bridged by oxygen atoms. POMs can interact with appropriate macrocations to form ionic crystals. Therefore, it is easy to imagine that incorporation of POMs within the cavity of MOFs can greatly enhance the adsorption capacity towards polar organic compounds, holding great potential in VOC removal. Furthermore, the corporation of metal cations within MOFs may also offer binding sites for organic vapors owing to the formation of cation–π interactions, a noncovalent molecular interaction formed by donation of some electrons from π-orbitals of organic vapors (especially for aromatic compounds or olefins) to the vacant s-orbitals of the metal cations, and along with electrons of d-orbitals of metal cations donated to the empty of anti-bonding π-orbitals of organic vapors. For example, Su and co-workers reported the incorporation of POMs within porous MOFs (denoted as POMs/MOFs) for VOC adsorption.134 After incorporation of POMs within MOFs, the thermal stability of such hybrid composites increased from 240 °C to 300 °C, but the surface area of the POMs/MOFs decreased from 1507 m2 g−1 to 405 m2 g−1 because the cavities of the MOFs are occupied by POMs, as illustrated in Fig. 5. The MOFs functionalized by POMs showed considerable enhancement in adsorption performance towards short-chain alcohols (C < 4) and various aromatic compounds. Further enhancement of the adsorption capacity towards organic compounds can be achieved by immersing POMs/MOFs in KCl solution. The enhanced performance is attributed to the increased number of adsorption sites afforded by POMs and K+ cations. The extensive H-bonding interactions between the adsorbate and the O atom of POMs as well as the ion–dipole electrostatic interactions between the adsorbate and alkali metal cations may result in the increase of the adsorption capacity of MOF with POMs and K+ cations incorporated towards VOCs. The high adsorption capacity of K+ cation-modified POMs/MOFs towards 1-propanol and 2-propanol confirms the impact of POMs and alkali metal cations on VOC adsorption. However, the adsorption capacity becomes smaller for cyclohexane, benzene and toluene as compared to 1-propanol and 2-propanol at room temperature owing to the decreased polarity, indicating the importance of incorporating alkali metal cations and POMs in MOFs. Incorporation of metal cations within MOFs offering open coordination sites and open shell electronic structures is beneficial for enhancing the adsorption interaction between MOFs and targeted adsorbates.135 In addition to POMs and alkali metal cations, the incorporation of Cu+ and Ag+ cations within porous materials is also widely used for enhancing the adsorption of hazardous pollutants owing to their large charge density.136 This strategy can also be applied for MOFs to enhance the adsorption performance towards hazardous pollutants. For example, Jhung et al. loaded CuCl2 on MIL-47(V) for the adsorption of benzothiophene, and such Cu2+ loaded MOFs displayed an enhanced adsorption capacity for benzothiophene owing to the π-complexation as well as the porosity/acidity of the MOFs.81 Ma and co-workers incorporated Ag+ within a porous aromatic framework for selective adsorption of ethylene over ethane. The Ag+-functionalized porous materials displayed significantly higher affinity towards ethylene than ethane owing to the formation of π-complexation.137
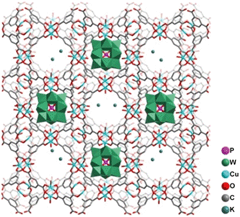 |
| Fig. 5 Structural illustration of K+-exchanged POMs/MOFs. Reproduced with permission from ref. 134. | |
In addition to incorporating metal cations within the cavity of MOFs, a facile strategy that has been employed for introducing an accessible metal ion with high affinity to certain toxic gas/vapors is post-synthetic metalation. This method can be applied to incorporate various metal ions into the pores of MOFs with the aim of enhancing the adsorption capacity of MOFs. There are a large number of multidentate organic molecules that can be used for the construction of MOFs, such as TCPP (4,4′4′′,4′′′-(porphyrin-5,10,15,20-tetrayl)tetrabenzoate) and 2,2′-bipyridine-5,5′-dicarboxylic acid.138 The primary functional group, –COOH, is used for the construction of the framework, while the secondary functional group within the framework is applied for chelating metal ions. For example, Kaskel et al. synthesized UiO-67(bipy) (constructed by using 2,2′-bipyridine-5,5′-dicarboxylic acid as the organic linker and Zr ion as the connecting center). In this case, the primary functional group of the organic ligand, –COOH, is used for linking the Zr ion to form the framework of the MOFs, where the secondary functional group, unoccupied N, is applied for chelating other metal ions (such as Ni2+, Co2+, Co2+) and those metal-chelated MOFs showed enhanced adsorption capacities for H2S.37
Graphite and GO are layered materials that possess many functional groups, also showing high capacity for organic and inorganic toxic gas/vapor adsorption.18 Therefore, the integration of various MOFs with graphite and GO for toxic gas adsorption is also promising for gaseous pollutant adsorption. Indeed, the adsorption performance for gaseous pollutants, including NH3, NO2, H2S, acetone and n-hexane, is enhanced after the integration of graphite or graphite oxide.139–142 The interaction between MOFs and oxygen on graphite and graphite oxide leads to the formation of new pore space, which can enhance the adsorption of toxic gases.54
4. MOF-based photocatalysts for gaseous pollutant degradation
MOF-based nanomaterials display enormous potential for the adsorption of gaseous pollutants owing to their high porosity and chemical diversity. However, the problems with the regeneration of MOF adsorbents and secondary pollution need to be solved. Recently, photocatalysis with a semiconductor has been proven to be efficient in the degradation of a wide range of organic pollutants as well as detoxification of hazardous compounds in practical applications.16,60–62,143 Recently, MOFs have also been demonstrated as semiconductors and are capable of generating reactive charges and transferring the photo-generated charges to reactive sites for in situ degrading organic compounds into CO2 and water upon light irradiation, resulting in the regeneration of adsorbent MOFs and avoiding secondary pollution. The unique chemical and physical properties of MOFs hold great potential for photocatalytic degradation of VOCs. MOFs are composed of transition metal ions as connecting centers and organic molecules as linkers. It is easy to understand that MOFs are typically characterized by the lowest unoccupied molecular orbital (LUMO) and the highest occupied molecular orbital (HOMO). Similar to semiconductors, photo-generated electrons migrate from the HOMO state to the LUMO state after light adsorption by the organic linker and quantum sized cluster. The photogenerated charges subsequently transfer to the surface of the metal oxide cluster to take part in photocatalytic oxidation of VOCs, and the charge transfer pathways are various based on the composition of the MOFs.144–146 Most of the charge-transfer pathways are based on ligand-to-metal charge transfer (LMCT); in addition to the LMCT mechanism, ligand-to-ligand transfer, metal-to-ligand charge transfer, and metal-to-metal-to-ligand charge transfer mechanisms have also been proposed in various photocatalytic reactions.144
As discussed above, the open metal sites of MOFs play an important role in the adsorption of toxic gases via various interactions between the open metal sites of the MOF and toxic gas. However, the strong binding force significantly hampered the regeneration of adsorbent MOFs. Photocatalytic oxidation/degradation of toxic gases over adsorbent MOFs is a promising way to the regeneration of adsorbent MOFs. It is well known that the open metal site is transitional metal, which can not only serve as a binding site for toxic gas adsorption but also as an active site for the generation of active radicals, which is highly active for toxic gas oxidation/decomposition. Recently, an Ag-doped MOF-like organotitanium polymer was synthesized for photocatalytic NO oxidation. It was found that the photo-generated active charges, including super oxide anions, hydroxyl radicals, and holes, are the reason for the high photocatalytic activity towards NO oxidation.147 Further study also focused on the integration of GO with titanium-based MOFs (NH2-MIL-125(Ti)) to improve photocatalytic NO oxidation, and high photocatalytic activity and stability of this composite was observed. In addition, doping MOFs with semiconductors/POMs should also be regarded as the ideal case for toxic gas oxidation, where MOFs have a positive effect on the adsorption and activation of toxic gas. Dai and co-workers doped ZIF-8 onto Au/TiO2 for CO oxidation. It was demonstrated that the adsorption and activation of CO and O2 by Au/TiO2 were promoted after ZIF-8 doping.148 In addition, POMs encapsulated within copper-based MOFs displayed enhanced adsorption capacity towards NO as well as photocatalytic activity for NO decomposition.149 Therefore, coupling semiconductors/electronic materials with MOFs is feasible to increase the photocatalytic performance for toxic gas decomposition/oxidation and will be a hot topic for air purification in future study.148
Chen and co-workers reported iron-based MOFs with hexagonal microspindle structure (NH2-MIL-101(Fe)) for visible-light driven photocatalytic degradation of toluene.151 The photocatalytic activity of NH2-MIL-101(Fe) is superior to that of P25 under visible light irradiation owing to the high efficiency in visible-light adsorption and photogenerated charge separation. This work highlights the great potential of MOFs for photocatalytic degradation of VOCs. However, the poor stability and limited active sites of MOFs in terms of photocatalytic oxidation of VOCs make this research area very challenging. Generally, attempts to improve the photocatalytic performance are primarily focused on two directions: loading semiconductors onto MOFs to in situ degrade the adsorbed VOCs and combining with electronic materials to promote the photo-generated electron and hole separation. Recently, Ye and co-workers developed a novel hybrid photocatalyst HKUST-1@TiO2 with core–shell structure by coating TiO2 onto the surface of KHUST-1 (KHUST-1 is a typical MOF built from Cu cation as a connecting center and benzene-1,3,5-tricarboxylate as a linker).152 The as-obtain composites are applied in photocatalytic degradation of isopropanol to CO2 and H2O. The evolution rate of acetone and CO2 onto HKUST-1@TiO2 is much higher than that of TiO2 nanocrystals. Particularly, CO2 generated from isopropanol decomposition is dramatically enhanced with HKUST-1@TiO2 as compared with the bare TiO2, clearly showing the niche of HKUST-1@TiO2 hybrid composites in the photocatalytic decomposition of isopropanol. More recently, our group developed a hard–soft acid–base (HSAB) synthetic strategy for the fabrication of TiO2@NH2-UiO-66 composites with controllable TiO2 content and size.150 The as-obtain composite was applied in the adsorption-concentrated photocatalytic degradation of styrene. Fig. 6a displays the adsorption performance of different adsorbents. It is clearly observed that TiO2 displayed poor adsorption performance towards styrene. In contrast, when NH2-UiO-66 was coated onto the surface of TiO2, the adsorption performance dramatically increased and the adsorption capacity could be varied by changing the ratio between NH2-UiO-66 and TiO2. Photocatalytic degradation of styrene is carried out in a continuous flow mode. As can be observed in Fig. 6b, higher CO2 amounts were generated onto TiO2@NH2-UiO-66 composites than that of individual TiO2 and NH2-UiO-66, suggesting high efficiency of TiO2@NH2-UiO-66 composites in photocatalytic degradation of styrene. Importantly, TiO2@NH2-UiO-66 displayed considerable stability as the catalytic activity of TiO2@NH2-UiO-66 is retained even after 10 h continuous reaction. The enhanced catalytic activity of TiO2@NH2-UiO-66 can be explained by the synergistic effect between MOFs and TiO2 in concentrating styrene around TiO2 for in situ degradation, facilitating the photogenerated electron transfer, and preventing TiO2 nanoparticle aggregation. Those works have opened a new avenue for the development of efficient porous materials for removing harmful gases/vapors through the synergistic effects of adsorption concentration and in situ photocatalytic degradation.
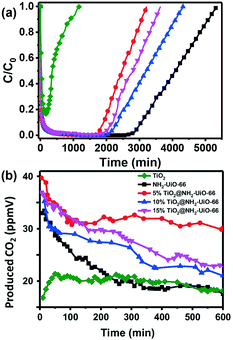 |
| Fig. 6 Adsorption kinetic curves of styrene (a) and evolution of CO2 from photocatalytic degradation of styrene after adsorption equilibrium onto adsorbent (b) by TiO2, NH2-UiO-66 and TiO2@NH2-UiO-66 composites. Reproduced with permission from ref. 150. | |
On the other hand, the integration of a conductive material with a semiconductor has been demonstrated as an effective way of inhibiting photogenerated electron–hole recombination. Among various conductive materials, GO has been widely used for effectively improving the semiconductor photocatalytic performance owing to its large surface area and abundant functional groups in accelerating photogenerated electron–hole separation and allowing MOFs to disperse well onto the surface of GO. Recently, titanium-based MOFs (NH2-MIL-125(Ti)) have been decorated on the surface of GO for photocatalytic oxidation of acetaldehyde under visible light irradiation. The as-obtained composites displayed higher photocatalytic activity than that of pure titanium-based MOFs. In the case of NH2-MIL-125(Ti) for photocatalytic reaction, the electron was excited from the linker of the MOFs upon light irradiation, then the excited electrons migrate to the cluster of MOFs, and the electrons are further injected into the GO to form superoxide anions for acetaldehyde oxidation. Besides loading semiconductors/POMs onto MOFs, embedding metal nanoparticles within MOFs that can thermally decompose the VOCs at a certain reaction temperature is a proposed research direction for the regeneration of adsorbent MOFs.8,153
With the aim of thoroughly solving pollution problems caused by gaseous pollutants, particular attention should be paid to the research opportunity regarding the development of advanced MOFs photocatalysts for photocatalytic degradation of gaseous pollutants into non-toxic substances under mild reaction conditions, which will be regarded as a green and sustainable technology in the near future since it overcomes the problems of adsorbent regeneration and secondary pollution, which often occur in adsorption technology. Although MOF-based photocatalysts for the degradation of gaseous pollutants are still in their infancy, the significant chemical diversity and high tunability of MOFs offer incredible opportunities for the rational design of MOF-based photocatalysts for enhancing photocatalytic performance, and we believe that the research on this topic will attract great attention in the future.
5. Conclusion and outlooks
This review comprehensively summarizes the advantages and high versatility of MOFs in gaseous pollutant purification by means of adsorption capturing or photocatalytic oxidation. MOFs have emerged as an alternative to traditional porous materials owing to their fascinating structure and unique property. The improved treatment efficiency for gaseous pollutants was due to i) ultrahigh surface area and adequate pore size/shape, significant structural diversity of MOFs for adsorptive capture gaseous pollutants, and ii) abundant open metal sites as well as certain functionalities within MOFs for chemical adsorption of gaseous pollutants via specific interactions (including acid–base interaction, electrostatic interaction, π-complex formation, π–π interaction, H-bonding and coordination bonds). Understanding the adsorption mechanisms summarized in this review is highly desirable for properly designing MOFs for capturing targeted organic and inorganic gaseous pollutants, because the modular nature of MOF synthesis allows us to rationally design and finely tune their physical and chemical properties at the molecular level, making the pores, cavities, electronic structures, surface functional groups and hydrophobic/hydrophilic properties of MOFs. However, despite such significant efforts, there still have many scientific issues and challenges in the purification of gaseous pollutants.
First, the most reported MOFs are still suffering from low stability and poor recyclability. The coordination bonds between single metal ions or polynuclear metal clusters and multi-dentate organic molecules are mild. The entire framework of MOFs is supported by weak coordination bonds. On the other hand, the nodes of MOFs are mainly composed of a transition metal and oxygen, which have very high affinity towards water, and hydrolysis reactions occur after water adsorption, which cause a significant decrease of the binding sites and collapse of the MOF framework. Therefore, the stability of MOFs is the most serious problem hindering their practical application. The key strategy is to controllably synthesize MOFs with a robust framework and high resistance towards water vapor. The following aspects are suggested for enhancing the stability of MOFs: 1) enhancing the coordination force and optimizing the coordination number between the inorganic and organic parts; 2) tuning the hydrophobic/hydrophilic properties of MOFs to endow MOFs with water-resisting property; 3) incorporation of rigid pillars into MOFs, to enhance the adsorption capacity as well as the stability of MOFs; and 4) grafting functional groups on the open metal sites of MOFs to prevent the adsorption of H2O and hinder the hydrolysis of metal clusters.
Second, the photocatalytic efficiency of MOFs for removing gaseous pollutants is still very low and the photocatalytic reaction mechanisms have not been carefully studied. The following issues still need further elaboration with the aim of completely converting VOCs to non-toxic substances: 1) further in-depth studies on the photogenerated electron transfer pathways are urgently needed; 2) the positive and negative effects of photogenerated active species on the stability of MOF should be carefully studied; and 3) much attention still needs to be paid to elucidation of the pollutant degradation pathways. The understanding of these issues can help in the rational design of MOF-based photocatalysts with high removal efficiency.
Third, the selective adsorption of highly toxic gaseous pollutants is still an ongoing challenge. The reasons that gaseous pollutants cause health problems vary significantly from highly toxic pollutants to those with unknown health effects. Based on the polarity, size/shape, and functionality of the adsorbate, a proper modeling and simulation method is highly desirable, not only for simulating the interactions between MOFs and gaseous pollutants, but also for calculating the adsorption capacity, enthalpy and diffusion constant. This is essentially important for the rational design of MOFs for selective adsorption of a targeted gaseous pollutant.
Finally, the cost for fabrication of MOFs is still very high. As potential adsorbents for industrial application, MOFs have attracted a great deal of interest. However, the starting materials are very expensive, and the fabrication of MOFs is usually carried out in organic solvents at high reaction temperatures, and the yield is still very low. These shortcomings hamper the industrial applications of MOFs. Therefore, looking for cheap starting materials and efficient synthetic fabrication methods for the preparation of MOFs with a large production scale is highly desirable.
The future development of MOF-based adsorbents for removing gaseous pollutants from the environment still needs deeper understanding and many challenges remain in this emerging field. We believe that in the near future these new porous materials with unique properties can serve as alternatives to the traditional inorganic adsorbents that are available on the market.
Conflicts of interest
There are no conflicts to declare.
Acknowledgements
This work was supported by the National Natural Science Foundation of China (41425015, 21707020, 41731279, 21406075, 500170054 and U1401245), the Local Innovative and Research Teams Project of Guangdong Pearl River Talents Program (2017BT01Z032), the innovation team project of Guangdong Provincial Department of Education (2017KCXTD012) and the Leading Scientific, Technical and Innovation Talents of Guangdong special support program (2016TX03Z094).
Notes and references
- U. Poeschl and M. Shiraiwa, Chem. Rev., 2015, 115, 4440–4475 CrossRef CAS PubMed.
- M. Kampa and E. Castanas, Environ. Pollut., 2008, 151, 362–367 CrossRef CAS PubMed.
- Z. Zhang, J. Chen, Y. Gao, Z. Ao, G. Li, T. An, Y. Hu and Y. Li, J. Cleaner Prod., 2018, 185, 266–274 CrossRef CAS.
- J. Tang, T. An, J. Xiong and G. Li, Environ. Geochem. Health, 2017, 39, 1487–1499 CrossRef CAS PubMed.
- J. L. Adgate, B. D. Goldstein and L. M. McKenzie, Environ. Sci. Technol., 2014, 48, 8307–8320 CrossRef CAS PubMed.
- D. Granieri, F. Vita and S. Inguaggiato, Environ. Pollut., 2017, 231, 219–228 CrossRef CAS PubMed.
- E. Webb, J. Hays, L. Dyrszka, B. Rodriguez, C. Cox, K. Huffling and S. Bushkin-Bedient, Rev. Environ. Health, 2016, 31, 225–243 CAS.
- L. F. Liotta, Appl. Catal., B, 2010, 100, 403–412 CrossRef CAS.
- G. L. Wei, X. L. Liang, D. Q. Li, M. N. Zhuo, S. Y. Zhang, Q. X. Huang, Y. S. Liao, Z. Y. Xie, T. L. Guo and Z. J. Yuan, Environ. Int., 2016, 92–93, 373–387 CrossRef CAS PubMed.
- R. Liu, J. Chen, G. Li, X. Wang and T. An, J. Environ. Manage., 2018 DOI:10.1016/j.jenvman.2018.07.034 , in press.
- M. A. Bari and W. B. Kindzierski, Environ. Pollut., 2018, 235, 602–614 CrossRef CAS PubMed.
- S. Mentese and D. Tasdibi, Global NEST J., 2017, 19, 726–732 Search PubMed.
- T. An, Y. Huang, G. Li, Z. He, J. Chen and C. Zhang, Environ. Int., 2014, 73, 186–194 CrossRef CAS PubMed.
- J. Chen, R. Liu, Y. Gao, G. Li and T. An, J. Cleaner Prod., 2017, 148, 268–275 CrossRef CAS.
- M. Hakim, Y. Y. Broza, O. Barash, N. Peled, M. Phillips, A. Amann and H. Haick, Chem. Rev., 2012, 112, 5949–5966 CrossRef CAS PubMed.
- J. Chen, Z. He, G. Li, T. An, H. Shi and Y. Li, Appl. Catal., B, 2017, 209, 146–154 CrossRef CAS.
- M. Yao, Y. Ji, H. Wang, Z. Ao, G. Li and T. An, J. Phys. Chem. C, 2017, 121, 13717–13722 CrossRef CAS.
- X. Zhang, B. Gao, A. E. Creamer, C. Cao and Y. Li, J. Hazard. Mater., 2017, 338, 102–123 CrossRef CAS PubMed.
- H. Chen, C. E. Nanayakkara and V. H. Grassian, Chem. Rev., 2012, 112, 5919–5948 CrossRef CAS PubMed.
- E. Barea, C. Montoro and J. A. R. Navarro, Chem. Soc. Rev., 2014, 43, 5419–5430 RSC.
- N. A. Khan, Z. Hasan and S. H. Jhung, J. Hazard. Mater., 2013, 244, 444–456 CrossRef PubMed.
- C. O. Ania and T. J. Bandosz, Langmuir, 2005, 21, 7752–7759 CrossRef CAS PubMed.
- W. Huang, Y. Zhang and D. Li, J. Environ. Manage., 2017, 193, 470–482 CrossRef CAS PubMed.
- X. D. Zhang, Y. Wang, Y. Q. Yang and D. Chen, Acta Phys.-Chim. Sin., 2015, 31, 1633–1646 CAS.
- Z. Guo, J. Huang, Z. Xue and X. Wang, Chem. Eng. J., 2016, 306, 99–106 CrossRef CAS.
- N. Brodu, H. Zaitan, M.-H. Manero and J.-S. Pic, Water Sci. Technol., 2012, 66, 2020–2026 CrossRef CAS PubMed.
- S. Zuo, F. Liu, R. Zhou and C. Qi, Catal. Commun., 2012, 22, 1–5 CrossRef CAS.
- S. Kamravaei, P. Shariaty, M. J. Lashaki, J. D. Atkinson, Z. Hashisho, J. H. Phillips, J. E. Anderson and M. Nichols, Ind. Eng. Chem. Res., 2017, 56, 1297–1305 CrossRef CAS.
- P. Kumar, K. H. Kim, E. E. Kwon and J. E. Szulejko, J. Mater. Chem. A, 2016, 4, 345–361 RSC.
- B. Dou, Q. Hu, J. Li, S. Qiao and Z. Hao, J. Hazard. Mater., 2011, 186, 1615–1624 CrossRef CAS PubMed.
- M. Wen, Y. Kuwahara, K. Mori, D. Zhang, H. Li and H. Yamashita, J. Mater. Chem. A, 2015, 3, 14134–14141 RSC.
- M. Wen, Y. Cui, Y. Kuwahara, K. Mori and H. Yamashita, ACS Appl. Mater. Interfaces, 2016, 8, 21278–21284 CrossRef CAS PubMed.
- J. L. Wang, C. Wang and W. B. Lin, ACS Catal., 2012, 2, 2630–2640 CrossRef CAS.
- K. K. Tanabe and S. M. Cohen, Chem. Soc. Rev., 2011, 40, 498–519 RSC.
- M. Wen, K. Mori, Y. Kuwahara and H. Yamashita, ACS Energy Lett., 2017, 2, 1–7 CrossRef CAS.
- L. Hamon, C. Serre, T. Devic, T. Loiseau, F. Millange, G. Ferey and G. De Weireld, J. Am. Chem. Soc., 2009, 131, 8775–8777 CrossRef CAS PubMed.
- G. Nickerl, M. Leistner, S. Helten, V. Bon, I. Senkovska and S. Kaskel, Inorg. Chem. Front., 2014, 1, 325–330 RSC.
- J. Liu, Y. Wei, P. Li, Y. Zhao and R. Zou, J. Phys. Chem. C, 2017, 121, 13249–13255 CrossRef CAS.
- K. Yu, K. Kiesling and J. R. Schmidt, J. Phys. Chem. C, 2012, 116, 20480–20488 CrossRef CAS.
- K. Tan, S. Zuluaga, Q. Gong, Y. Gao, N. Nijem, J. Li, T. Thonhauser and Y. J. Chabal, Chem. Mater., 2015, 27, 2203–2217 CrossRef CAS.
- M. Savage, Y. Cheng, T. L. Easun, J. E. Eyley, S. P. Argent, M. R. Warren, W. Lewis, C. Murray, C. C. Tang, M. D. Frogley, G. Cinque, J. Sun, S. Rudic, R. T. Murder, M. J. Benham, A. N. Fitch, A. J. Blake, A. J. Ramirez-Cuesta, S. Yang and M. Schroder, Adv. Mater., 2016, 28, 8705–8711 CrossRef CAS PubMed.
- A. C. McKinlay, J. F. Eubank, S. Wuttke, B. Xiao, P. S. Wheadey, P. Bazin, J. C. Lavalley, M. Daturi, A. Vimont, G. De Weireld, P. Horcajada, C. Serre and R. E. Morris, Chem. Mater., 2013, 25, 1592–1599 CrossRef CAS.
- M. Ma, H. Noei, B. Mienert, J. Niesel, E. Bill, M. Muhler, R. A. Fischer, Y. Wang, U. Schatzschneider and N. Metzler-Nolte, Chem. – Eur. J., 2013, 19, 6785–6790 CrossRef CAS PubMed.
- J. R. Karra and K. S. Walton, Langmuir, 2008, 24, 8620–8626 CrossRef CAS PubMed.
- D. Britt, D. Tranchemontagne and O. M. Yaghi, Proc. Natl. Acad. Sci. U. S. A., 2008, 105, 11623–11627 CrossRef CAS PubMed.
- M. A. Browe, A. Napolitano, J. B. DeCoste and G. W. Peterson, J. Hazard. Mater., 2017, 332, 162–167 CrossRef CAS PubMed.
- J. B. DeCoste, M. A. Browe, G. W. Wagner, J. A. Rossin and G. W. Peterson, Chem. Commun., 2015, 51, 12474–12477 RSC.
- Y. Tulchinsky, C. H. Hendon, K. A. Lomachenko, E. Borfecchia, B. C. Melot, M. R. Hudson, J. D. Tarver, M. D. Korzynski, A. W. Stubbs, J. J. Kagan, C. Lamberti, C. M. Brown and M. Dinca, J. Am. Chem. Soc., 2017, 139, 5992–5997 CrossRef CAS PubMed.
- B. Supronowicz, A. Mavrandonakis and T. Heine, J. Phys. Chem. C, 2013, 117, 14570–14578 CrossRef CAS.
- G. W. Peterson, G. W. Wagner, A. Balboa, J. Mahle, T. Sewell and C. J. Karwacki, J. Phys. Chem. C, 2009, 113, 13906–13917 CrossRef CAS PubMed.
- X. Li, H. Liu, X. Jia, G. Li, T. An and Y. Gao, Sci. Total Environ., 2018, 621, 1533–1541 CrossRef CAS PubMed.
- V. Guillerm, F. Ragon, M. Dan-Hardi, T. Devic, M. Vishnuvarthan, B. Campo, A. Vimont, G. Clet, Q. Yang, G. Maurin, G. Ferey, A. Vittadini, S. Gross and C. Serre, Angew. Chem., Int. Ed., 2012, 51, 9267–9271 CrossRef CAS PubMed.
- C. Petit and T. J. Bandosz, Adv. Funct. Mater., 2010, 20, 111–118 CrossRef CAS.
- C. Petit, B. Mendoza and T. J. Bandosz, Langmuir, 2010, 26, 15302–15309 CrossRef CAS PubMed.
- C. Petit and T. J. Bandosz, Dalton Trans., 2012, 41, 4027–4035 RSC.
- A. C. McKinlay, B. Xiao, D. S. Wragg, P. S. Wheatley, I. L. Megson and R. E. Morris, J. Am. Chem. Soc., 2008, 130, 10440–10444 CrossRef CAS PubMed.
- M. J. Ingleson, R. Heck, J. A. Gould and M. J. Rosseinsky, Inorg. Chem., 2009, 48, 9986–9988 CrossRef CAS PubMed.
- S. Glomb, D. Woschko, G. Makhloufi and C. Janiak, ACS Appl. Mater. Interfaces, 2017, 9, 37419–37434 CrossRef CAS PubMed.
- Y. Ji, J. Zhao, H. Terazono, K. Misawa, N. P. Levitt, Y. Li, Y. Lin, J. Peng, Y. Wang, L. Duan, B. Pan, F. Zhang, X. Feng, T. An, W. Marrero-Ortiz, J. Secrest, A. L. Zhang, K. Shibuya, M. J. Molina and R. Zhang, Proc. Natl. Acad. Sci. U. S. A., 2017, 114, 8169–8174 CrossRef CAS PubMed.
- J. Chen, D. Zhang, G. Li, T. An and J. Fu, Chem. Eng. J., 2016, 301, 299–305 CrossRef CAS.
- R. Liu, J. Chen, G. Li and T. An, Chem. Eng. J., 2017, 318, 57–63 CrossRef CAS.
- G. Li, Z. Zhang, H. Sun, J. Chen, T. An and B. Li, J. Hazard. Mater., 2013, 250, 147–154 CrossRef PubMed.
- S. Mukherjee, B. Joarder, B. Manna, A. V. Desai, A. K. Chaudhari and S. K. Ghosh, Sci. Rep., 2014, 4, 5761 CrossRef CAS PubMed.
- V. Finsy, C. E. A. Kirschhock, G. Vedts, M. Maes, L. Alaerts, D. E. De Vos, G. V. Baron and J. F. M. Denayer, Chem. – Eur. J., 2009, 15, 7724–7731 CrossRef CAS PubMed.
- M. Maes, F. Vermoortele, M. Boulhout, T. Boudewijns, C. Kirschhock, R. Ameloot, I. Beurroies, R. Denoyel and D. E. De Vos, Microporous Mesoporous Mater., 2012, 157, 82–88 CrossRef CAS.
- S. Abe and T. Ueno, RSC Adv., 2015, 5, 21366–21375 RSC.
- K. Zhang, R. P. Lively, C. Zhang, R. R. Chance, W. J. Koros, D. S. Sholl and S. Nair, J. Phys. Chem. Lett., 2013, 4, 3618–3622 CrossRef CAS.
- M. T. Gokmen, J. Brassinne, R. A. Prasath and F. E. Du Prez, Chem. Commun., 2011, 47, 4652–4654 RSC.
- W. Q. Xu, S. He, C. C. Lin, Y. X. Qiu, X. J. Liu, T. Jiang, W. T. Liu, X. L. Zhang and J. J. Jiang, Inorg. Chem. Commun., 2018, 92, 1–4 CrossRef CAS.
- M. Woellner, S. Hausdorf, N. Klein, P. Mueller, M. W. Smith and S. Kaskel, Adv. Mater., 2018, 30, 1704679 CrossRef PubMed.
- Z. Y. Gu, G. Wang and X. P. Yan, Anal. Chem., 2010, 82, 1365–1370 CrossRef CAS PubMed.
- Z. Wang, W. Wang, D. Jiang, L. Zhang and Y. Zheng, Dalton Trans., 2016, 45, 11306–11311 RSC.
- T. Dutta, K. H. Kim, R. J. C. Brown, Y. H. Kim and D. Boukhvalov, Sci. Rep., 2018, 8, 5033 CrossRef PubMed.
- T. Terencio, F. Di Renzo, D. Berthomieu and P. Trens, J. Phys. Chem. C, 2013, 117, 26156–26165 CrossRef CAS.
- K. Yang, Q. Sun, F. Xue and D. Lin, J. Hazard. Mater., 2011, 195, 124–131 CrossRef CAS PubMed.
- K. Yang, F. Xue, Q. Sun, R. Yue and D. Li, J. Environ. Chem. Eng., 2013, 1, 713–718 CrossRef CAS.
- J. Shi, Z. Zhao, Q. Xia, Y. Li and Z. Li, J. Chem. Eng. Data, 2011, 56, 3419–3425 CrossRef CAS.
- C. Y. Huang, M. Song, Z. Y. Gu, H. F. Wang and X. P. Yan, Environ. Sci. Technol., 2011, 45, 4490–4496 CrossRef CAS PubMed.
- U. Mueller, M. Schubert, F. Teich, H. Puetter, K. Schierle-Arndt and J. Pastre, J. Mater. Chem., 2006, 16, 626–636 RSC.
- S. Galli, N. Masciocchi, V. Colombo, A. Maspero, G. Palmisano, F. J. Lopez-Garzon, M. Domingo-Garcia, I. Fernandez-Morales, E. Barea and J. A. R. Navarro, Chem. Mater., 2010, 22, 1664–1672 CrossRef CAS.
- N. A. Khan and S. H. Jhung, Angew. Chem., Int. Ed., 2012, 51, 1198–1201 CrossRef CAS PubMed.
- Z. Hasan, M. Tong, B. K. Jung, I. Ahmed, C. Zhong and S. H. Jhung, J. Phys. Chem. C, 2014, 118, 21049–21056 CrossRef CAS.
- N. M. Padial, E. Quartapelle Procopio, C. Montoro, E. Lopez, J. Enrique Oltra, V. Colombo, A. Maspero, N. Masciocchi, S. Galli, I. Senkovska, S. Kaskel, E. Barea and J. A. R. Navarro, Angew. Chem., Int. Ed., 2013, 52, 8290–8294 CrossRef CAS PubMed.
- L. Wang, Y. A. Li, F. Yang, Q. K. Liu, J. P. Ma and Y. B. Dong, Inorg. Chem., 2014, 53, 9087–9094 CrossRef CAS PubMed.
- W. D. Reid, G. Krishna, R. Gillette and B. B. Brodie, Pharmacology, 1973, 10, 193–214 CrossRef CAS PubMed.
- S. Xian, X. Li, F. Xu, Q. Xia and Z. Li, Sep. Sci. Technol., 2013, 48, 1479–1489 CrossRef CAS.
- L. Bullot, L. Vieira-Sellai, G. Chaplais, A. Simon-Masseron, T. J. Daou, J. Patarin and E. Fiani, Environ. Sci. Pollut. Res., 2017, 24, 26562–26573 CrossRef CAS PubMed.
- S. Xian, Y. Yu, J. Xiao, Z. Zhang, Q. Xia, H. Wang and Z. Li, RSC Adv., 2015, 5, 1827–1834 RSC.
- P. Hu, X. Liang, M. Yaseen, X. Sun, Z. Tong, Z. Zhao and Z. Zhao, Chem. Eng. J., 2018, 332, 608–618 CrossRef CAS.
- G. Ferey, C. Mellot-Draznieks, C. Serre, F. Millange, J. Dutour, S. Surble and I. Margiolaki, Science, 2005, 309, 2040–2042 CrossRef CAS PubMed.
- P. Trens, H. Belarbi, C. Shepherd, P. Gonzalez, N. A. Ramsahye, U. H. Lee, Y. K. Seo and J. S. Chang, J. Phys. Chem. C, 2012, 116, 25824–25831 CrossRef CAS.
- P. Liu, C. Long, Q. Li, H. Qian, A. Li and Q. Zhang, J. Hazard. Mater., 2009, 166, 46–51 CrossRef CAS PubMed.
- S. H. Jhung, J. H. Lee, J. W. Yoon, C. Serre, G. Ferey and J. S. Chang, Adv. Mater., 2007, 19, 121–124 CrossRef CAS.
- Z. Zhao, S. Wang, Y. Yang, X. Li, J. Li and Z. Li, Chem. Eng. J., 2015, 259, 79–89 CrossRef CAS.
- Y. Wu, H. Chen, D. Liu, J. Xiao, Y. Qian and H. Xi, ACS Appl. Mater. Interfaces, 2015, 7, 5775–5787 CrossRef CAS PubMed.
- L. Duan, X. Dong, Y. Wu, H. Li, L. Wang and L. Song, J. Porous Mater., 2013, 20, 431–440 CrossRef CAS.
- Z. Y. Gu, D. Q. Jiang, H. F. Wang, X. Y. Cui and X. P. Yan, J. Phys. Chem. C, 2010, 114, 311–316 CrossRef CAS.
- F. Vermoortele, M. Maes, P. Z. Moghadam, M. J. Lennox, F. Ragon, M. Boulhout, S. Biswas, K. G. M. Laurier, I. Beurroies, R. Denoyel, M. Roeffaers, N. Stock, T. Dueren, C. Serre and D. E. De Vos, J. Am. Chem. Soc., 2011, 133, 18526–18529 CrossRef CAS PubMed.
- N. Chang, Z. Y. Gu, H. F. Wang and X. P. Yan, Anal. Chem., 2011, 83, 7094–7101 CrossRef CAS PubMed.
- X. F. Chen, H. Zang, X. Wang, J. G. Cheng, R. S. Zhao, C. G. Cheng and X. Q. Lu, Analyst, 2012, 137, 5411–5419 RSC.
- S. H. Huo and X. P. Yan, Analyst, 2012, 137, 3445–3451 RSC.
- Z. R. Herm, E. D. Bloch and J. R. Long, Chem. Mater., 2014, 26, 323–338 CrossRef CAS.
- N. A. Ramsahye, T. Thuy Khuong, L. Scott, F. Nouar, T. Devic, P. Horcajada, E. Magnier, O. David, C. Serre and P. Trens, Chem. Mater., 2013, 25, 479–488 CrossRef CAS.
- D. Fairen-Jimenez, R. Galvelis, A. Torrisi, A. D. Gellan, M. T. Wharmby, P. A. Wright, C. Mellot-Draznieks and T. Dueren, Dalton Trans., 2012, 41, 10752–10762 RSC.
- R. Krishna and J. M. van Baten, Langmuir, 2010, 26, 8450–8463 CrossRef CAS PubMed.
- M. T. Luebbers, T. Wu, L. Shen and R. I. Masel, Langmuir, 2010, 26, 11319–11329 CrossRef CAS PubMed.
- V. Finsy, S. Calero, E. Garcia-Perez, P. J. Merkling, G. Vedts, D. E. De Vos, G. V. Baron and J. F. M. Denayer, Phys. Chem. Chem. Phys., 2009, 11, 3515–3521 RSC.
- L. Zhang, Q. Wang, T. Wu and Y. C. Liu, Chem. – Eur. J., 2007, 13, 6387–6396 CrossRef CAS PubMed.
- J. Jiang and S. I. Sandler, Langmuir, 2006, 22, 5702–5707 CrossRef CAS PubMed.
- D. Farrusseng, C. Daniel, C. Gaudillere, U. Ravon, Y. Schuurman, C. Mirodatos, D. Dubbeldam, H. Frost and R. Q. Snurr, Langmuir, 2009, 25, 7383–7388 CrossRef CAS PubMed.
- P. Q. Liao, N. Y. Huang, W. X. Zhang, J. P. Zhang and X. M. Chen, Science, 2017, 356, 1193–1196 CrossRef CAS PubMed.
- Y. Hu, S. Xiang, W. Zhang, Z. Zhang, L. Wang, J. Bai and B. Chen, Chem. Commun., 2009, 7551–7553 RSC.
- U. Boehme, B. Barth, C. Paula, A. Kuhnt, W. Schwieger, A. Mundstock, J. Caro and M. Hartmann, Langmuir, 2013, 29, 8592–8600 CrossRef CAS PubMed.
- Y. Li, Q. Gao, Y. Zhou, L. Zhang, Y. Zhong, Y. Ying, M. Zhang, Y. Liu and Y. a. Wang, J. Hazard. Mater., 2018, 358, 113–121 CrossRef CAS PubMed.
- A. Roy, A. K. Srivastava, B. Singh, D. Shah, T. H. Mahato and A. Srivastava, Dalton Trans., 2012, 41, 12346–12348 RSC.
- C. Montoro, F. Linares, E. Quartapelle Procopio, I. Senkovska, S. Kaskel, S. Galli, N. Masciocchi, E. Barea and J. A. R. Navarro, J. Am. Chem. Soc., 2011, 133, 11888–11891 CrossRef CAS PubMed.
- A. Roy, A. K. Srivastava, B. Singh, T. H. Mahato, D. Shah and A. K. Halve, Microporous Mesoporous Mater., 2012, 162, 207–212 CrossRef CAS.
- R. D. Gawhane, P. S. P. Rao, K. B. Budhavant, V. Waghmare, D. C. Meshram and P. D. Safai, Environ. Sci. Pollut. Res., 2017, 24, 21065–21072 CrossRef CAS PubMed.
- C. Liu, P. C. Hsu, H. W. Lee, M. Ye, G. Zheng, N. Liu, W. Li and Y. Cui, Nat. Commun., 2015, 6, 6205 CrossRef CAS PubMed.
- Y. Chen, F. Chen, S. Zhang, Y. Cai, S. Cao, S. Li, W. Zhao, S. Yuan, X. Feng, A. Cao, X. Ma and B. Wang, J. Am. Chem. Soc., 2017, 139, 16482–16485 CrossRef CAS PubMed.
- W. T. Koo, J. S. Jang, S. Qao, W. Hwang, G. Jha, R. M. Penner and I. D. Kim, ACS Appl. Mater. Interfaces, 2018, 10, 19957–19963 CrossRef CAS PubMed.
- Y. Chen, S. Zhang, S. Cao, S. Li, F. Chen, S. Yuan, C. Xu, J. Zhou, X. Feng, X. Ma and B. Wang, Adv. Mater., 2017, 29, 1606221 CrossRef PubMed.
- A. Wang, R. Fan, X. Zhou, S. Hao, X. Zheng and Y. Yang, ACS Appl. Mater. Interfaces, 2018, 10, 9744–9755 CrossRef CAS PubMed.
- M. Wen, K. Mori, Y. Kuwahara, T. An and H. Yamashita, Appl. Catal., B, 2017, 218, 555–569 CrossRef CAS.
- L. Chen, R. Lupue and Y. Li, Chem. Soc. Rev., 2017, 46, 4614–4630 RSC.
- Y. K. Hwang, D. Y. Hong, J.-S. Chang, S. H. Jhung, Y. K. Seo, J. Kim, A. Vimont, M. Daturi, C. Serre and G. Ferey, Angew. Chem., Int. Ed., 2008, 47, 4144–4148 CrossRef CAS PubMed.
- P. Siman, C. A. Trickett, H. Furukawa and O. M. Yaghi, Chem. Commun., 2015, 51, 17463–17466 RSC.
- Y. K. Seo, J. W. Yoon, J. S. Lee, Y. K. Hwang, C. H. Jun, J. S. Chang, S. Wuttke, P. Bazin, A. Vimont, M. Daturi, S. Bourrelly, P. L. Llewellyn, P. Horcajada, C. Serre and G. Ferey, Adv. Mater., 2012, 24, 806–810 CrossRef CAS PubMed.
- M. Zhu, P. Hu, Z. Tong, Z. Zhao and Z. Zhao, Chem. Eng. J., 2017, 313, 1122–1131 CrossRef CAS.
- N. N. Yang, W. Sun, F. G. Xi, Q. Sui, L. J. Chen and E. Q. Gao, Chem. Commun., 2017, 53, 1747–1750 RSC.
- A. J. Rieth, Y. Tulchinsky and M. Dinca, J. Am. Chem. Soc., 2016, 138, 9401–9404 CrossRef CAS PubMed.
- C. Yang, U. Kaipa, Q. Z. Mather, X. Wang, V. Nesterov, A. F. Venero and M. A. Omary, J. Am. Chem. Soc., 2011, 133, 18094–18097 CrossRef CAS PubMed.
- S. I. Noro and T. Nakamura, NPG Asia Mater., 2017, 9, e433 CrossRef.
- F. J. Ma, S. X. Liu, D. D. Liang, G. J. Ren, F. Wei, Y. G. Chen and Z. M. Su, J. Solid State Chem., 2011, 184, 3034–3039 CrossRef CAS.
- A. R. Kim, T. U. Yoon, E. J. Kim, J. W. Yoon, S. Y. Kim, J. W. Yoon, Y. K. Hwang, J. S. Chan and Y. S. Bae, Chem. Eng. J., 2018, 331, 777–784 CrossRef CAS.
- R. T. Yang, A. J. Hernandez-Maldonado and F. H. Yang, Science, 2003, 301, 79–81 CrossRef CAS PubMed.
- B. Li, Y. Zhang, R. Krishna, K. Yao, Y. Han, Z. Wu, D. Ma, Z. Shi, T. Pham, B. Space, J. Liu, P. K. Thallapally, J. Liu, M. Chrzanowski and S. Ma, J. Am. Chem. Soc., 2014, 136, 8654–8660 CrossRef CAS PubMed.
- J. D. Evans, C. J. Sumby and C. J. Doonan, Chem. Soc. Rev., 2014, 43, 5933–5951 RSC.
- P. Samaddar, Y.-S. Son, D. C. W. Tsang, K.-H. Kim and S. Kumar, Coord. Chem. Rev., 2018, 368, 93–114 CrossRef CAS.
- X. Zhou, W. Huang, J. Shi, Z. Zhao, Q. Xia, Y. Li, H. Wang and Z. Li, J. Mater. Chem. A, 2014, 2, 4722–4730 RSC.
- C. Petit, L. Huang, J. Jagiello, J. Kenvin, K. E. Gubbins and T. J. Bandosz, Langmuir, 2011, 27, 13043–13051 CrossRef CAS PubMed.
- X. Sun, Q. Xia, Z. Zhao, Y. Li and Z. Li, Chem. Eng. J., 2014, 239, 226–232 CrossRef CAS.
- W. Wang, G. Li, D. Xia, T. An, H. Zhao and P. K. Wong, Environ. Sci.: Nano, 2017, 4, 782–799 RSC.
- M. Wen, K. Mori, Y. Kuwahara, T. An and H. Yamashita, Chem. – Asian J., 2018, 13, 1767–1779 CrossRef CAS PubMed.
- T. Zhang and W. Lin, Metal-organic frameworks for artificial photosynthesis and photocatalysis, Chem. Soc. Rev., 2014, 43, 5982–5993 RSC.
- H. Yamashita, K. Mori, Y. Kuwahara, T. Kamegawa, M. Wen, P. Verma and M. Che, Chem. Soc. Rev., 2018, 47, 8072–8096 RSC.
- W. Zhu, P. Liu, S. Xiao, W. Wang, D. Zhang and H. Li, Appl. Catal., B, 2015, 172, 46–51 CrossRef.
- Y. Zhang, Q. Li, C. Liu, X. Shan, X. Chen, W. Dai and X. Fu, Appl. Catal., B, 2018, 224, 283–294 CrossRef CAS.
- F. J. Ma, S. X. Liu, G. J. Ren, D. D. Liang and S. Sha, Inorg. Chem. Commun., 2012, 22, 174–177 CrossRef CAS.
- P. Yao, H. Liu, D. Wang, J. Chen, G. Li and T. An, J. Colloid Interface Sci., 2018, 522, 174–182 CrossRef CAS PubMed.
- Z. Zhang, X. Li, B. Liu, Q. Zhao and G. Chen, RSC Adv., 2016, 6, 4289–4295 RSC.
- H. Wang, T. Yu, X. Tan, H. Zhang, P. Li, H. Liu, L. Shi, X. Li and J. Ye, Ind. Eng. Chem. Res., 2016, 55, 8096–8103 CrossRef CAS.
- H. Huang, Y. Xu, Q. Feng and D. Y. C. Leung, Catal. Sci. Technol., 2015, 5, 2649–2669 RSC.
|
This journal is © The Royal Society of Chemistry 2019 |
Click here to see how this site uses Cookies. View our privacy policy here.