No measurable “cleaning” of polychlorinated biphenyls from Rainbow Trout in a 9 week depuration study with dietary exposure to 40% polyethylene microspheres†
Received
9th April 2016
, Accepted 10th June 2016
First published on 10th June 2016
Abstract
Persistent hydrophobic chemicals sorbed to plastic can be transferred to fish and other aquatic organisms upon ingestion. However, ingestion of plastic could also lead to enhanced elimination of these chemicals if the plastic is less contaminated than the fish. Here, we attempted to measure the influence of ingestion of uncontaminated polyethylene microspheres on the depuration rates of polychlorinated biphenyls (PCBs) in an in vivo fish feeding experiment. Rainbow trout were given feed contaminated with PCBs for two consecutive days, then clean feed for three days to allow for egestion of the contaminated food. A control group of fish were then fed ordinary food pellets and a treatment group were fed pellets that additionally contained 40% by weight polyethylene microspheres. Condition factors and growth rates in both groups were similar, indicating no negative effect of the plastic microspheres on the nutritional status of the fish. Fish were sampled after zero, three, six and nine weeks, homogenized, solvent-extracted and analyzed by GC/MS. PCB concentrations declined in both groups at a rate consistent with growth dilution. There was no significant difference in the elimination rate constants between the control and treatment group, indicating that ingestion of uncontaminated plastic did not cause a measurable enhancement of depuration of PCBs by the fish in this study.
Environmental impact
Ingestion of plastic by fish and other aquatic organisms could potentially modulate their uptake and depuration of persistent hydrophobic contaminants such as polychlorinated biphenyls (PCBs). Most studies in the literature have described experiments in which plastic contaminated with chemicals is fed to relatively clean organisms, and chemicals are transferred from the plastic to the organism. Here, we fed PCB-contaminated fish a diet that included 40% by weight uncontaminated polyethylene microspheres and attempted to measure differences in depuration rates of PCBs compared to fish fed a diet with no plastic. There was no observable effect of plastic ingestion on the rate of depuration of PCBs or on the fitness of the fish.
|
Introduction
The presence of plastic in aquatic ecosystems worldwide has become a major concern1–3 since it is only slowly degradable4,5 and may be ingested by wildlife.6–10 One potential impact that has been articulated in the scientific literature and to the general public is the possibility that microplastic particles ingested by wildlife could deliver a dose of hydrophobic organic chemicals (HOCs) to organisms.11,12 This “Trojan horse”13 or “organismal-vector effect”14 describes a scenario in which microplastic sorbs HOCs15,16 from water and desorbs them in the gastrointestinal tract (GIT) of the organisms.11,12 A few experimental studies have investigated the transfer of chemicals between plastics in the GIT and organisms,12,17–19 with some focused on higher vertebrates like fish.20,21 Most studies reported to date17–21 have described experiments in which contaminated plastic was fed to relatively uncontaminated organisms, which resulted in transfer of chemicals from the plastic to the organisms. In such experiments the contaminated plastic acts as a carrier that delivers chemicals to the test organism in a manner similar to passive dosing systems used in ecotoxicology.22
In the field of human toxicology it has long been recognized that persistent hydrophobic chemicals can be eliminated through feces, and that the elimination rate depends on the characteristics of the gut contents.23 Experiments in the late 1990's demonstrated that fecal elimination of HOCs by humans was enhanced by the consumption of Olestra, a non-digestible, non-absorbable dietary fat substitute.23–26 A recent model study on the lugworm Arenicola marina27 suggested that ingestion of plastic by wildlife could attenuate the biomagnification of HOCs. Specifically, the study illustrated that ingestion of plastic could contribute either (1) to the uptake of polychlorinated biphenyls (PCBs) into the organism, or (2) to depuration of PCBs from the organism by a “cleaning” mechanism, depending on whether the fugacity of PCBs in the plastic was higher or lower than in the organism, respectively.27
Assessing the influence of ingested plastic on the body burden of chemicals in organisms in the environment has so far depended on interpretation of limited information from field studies and modeling. Using a model of piscivorous fish that assumed equilibrium partitioning, Gouin et al.28 calculated a reduction of >20% in the body burden of chemicals with a log
KOW between 6.5 and 7.5 as a consequence of including 10% microplastic in the diet.28 In a recent review, Koelmans et al.22 concluded that “microplastic ingestion is not likely to increase the exposure and thus risks of HOCs in the marine environment”, which is supported by recent observations in Northern Fulmars by Herzke et al.29
Here we report results of an in vivo study with Rainbow Trout that was designed to test the hypothesis that ingestion of microplastic can influence the rate of depuration of persistent HOCs. We measured the depuration rates of four PCB congeners by fish that had been dosed through diet and subsequently were fed with food pellets that had microplastic added at the highest feasible proportion to maximize the presumed effect. PCB elimination rates by fish fed a diet with added microplastic were compared to fish fed the same diet with no microplastic during the depuration phase of the experiment. The results are discussed with reference to the Arnot and Gobas30 fish bioaccumulation model, which is commonly used in bioaccumulation assessments of organic chemicals. Further, we investigated potential effects on the fish's fitness status.
This study addresses the call for experimental validation of the proposed “cleaning” mechanism that was made by Koelmans et al. in a recent critical review of microplastic as a vector for chemicals in the aquatic environment.22 This study was not designed to mimic natural conditions where organisms and their food are expected to be close to equilibrium with respect to the chemicals. We fed contaminated fish with clean feed to achieve a strong thermodynamic gradient favoring depuration of chemicals from the fish to the gut contents, and tested whether higher sorption capacity for chemicals in the gut provided by ingested plastic would measurably enhance depuration.
Experimental
The experiment was approved by the Swedish Animal Research Ethical Committee (Ethical permission N 119/14).
Chemicals
Acetone, toluene and n-hexane (“SupraSolv”) were from Merck, Darmstadt, Germany. Sodium chloride, phosphoric acid and sulphuric acid 98% (AnalaR NORMAPUR®) were from BDH, Pool, UK. Diethyl ether was from Poch S.A., Gliwice, Poland. Gelatin (TØrsleffs) was from Haugen-Gruppen AB, Norrköping, Sweden. Corn oil (Zeta) from Italy and soy sauce (Wanjashan) from Taiwan were purchased at a local supermarket. The water was of milli-Q grade from a milli-Q ultrapure water system, MilliQ PLUS 185 from Millipore Stockholm, Sweden. Ethanol (Spektro, 99.5%) was from Solveco, Rosersberg, Sweden. 2,2′,5-Trichlorobiphenyl (PCB 18), 2,2′,3,3′-tetrachlorobiphenyl (PCB 40), 2,2′,5,5′-tetrachlorobiphenyl (PCB 52, used as a volumetric standard), 2,2′,3,3′4,4′-hexachlorobiphenyl (PCB 128) and decachlorobiphenyl (PCB 209) were from Accustandard, Inc, New Haven, CT, USA. As surrogate standards, 13C12-2,2′,3,3′,4,4′-hexachlorobiphenyl (PCB 128) and 13C12-decachlorobiphenyl (PCB 209) from Cambridge Isotope Laboratories, Inc, Andover, MA, USA were used.
PCB-contaminated food
Regular food pellets (2 mm, EFICO Alpha from BioMar, Wernberg-Köblitz, Germany) were contaminated by adding PCB 18, PCB 40, PCB 128 and PCB 209 in toluene. The pellets were rotated overnight in a closed vial to allow the PCBs to be absorbed, then spread on a filter paper in a fume-hood and dried overnight to let the solvent evaporate.31 PCB concentrations in the contaminated food were measured in four replicate analyses to be 25.2 ± 2.5 μg gpellet−1 for PCB 18, 22.5 ± 2.1 μg gpellet−1 for PCB 40, 26.4 ± 2.2 μg gpellet−1 for PCB 128 and 9.7 ± 1.5 μg gpellet−1 for PCB 209.
Plastic-containing fish food
To prepare the plastic-containing food for the depuration experiment, commercial fish food pellets (see above) were ground in a food processor (Büchi B-400, Flawil, Switzerland). The ground pellets (100 g) were mixed with 68 g of white polyethylene microspheres (212–250 μm, density 1.3112 g mL−1, Cospheric, Santa Barbara, CA, USA). Gelatin (7 g) was soaked in 100 mL of water, slowly melted and heated to 70 °C, then added to the mixture along with soy sauce (20 mL) and corn oil (45 mL). These three ingredients acted as a binder to hold together the final pellets. The mixture of food pellets, plastic, gelatin, soy sauce and corn oil was blended in a household blender (Braun Multiquick, Neu-Isenburg, Germany). The mixture was allowed to dry for six hours, squeezed through a garlic press and left overnight in a fume hood to dry. The dried pellets were further minced by hand to obtain pellets resembling the ordinary food pellets as closely as possible. The food pellets we produced were 40% by weight plastic; attempts to incorporate a higher proportion of plastic into the food produced pellets that fell apart upon handling. Plastic-containing pellets as well as the herein processed commercial fish food pellets were extracted and analyzed using GC/MS to confirm the absence of PCB contamination.
Experimental
Experiments were conducted in three fiberglass fish tanks containing 300 L of charcoal-filtered tap water. Two tanks (treatment and control) contained eighteen rainbow trout (Oncorhynchus mykiss) each weighing between 80 and 100 g. The third tank with blank fish served as quality control for processing blank fish on each sampling day to verify the absence of cross-contaminating PCBs in the study setup and during analytical procedures. Rainbow trout were purchased from Vilstena fiskodling AB (Enköping, Sweden) at the age of 12 months and held in the laboratory's aquaria to acclimatize for 4 weeks before starting the experiment. The aquaria were circular with a central overflow drain, and were supplied with a continuous flow of charcoal-filtered, aerated tap water. An additional filter removed particles larger than 10 μm. Water was supplied to the aquaria at 42 L h−1, giving an exchange rate of 7 d−1. The water supply had a temperature of 13 °C, and the temperature in the aquaria room was maintained at 13 °C. The photoperiod was set in a cycle of 12 hours light and 12 hours dark.
Prior to the depuration experiment we conducted a preliminary feeding experiment to evaluate the performance of the plastic-containing fish food. The pellets remained intact when added to the aquaria, and the fish's ingestion of the plastic-containing pellets was not distinguishable from their ingestion of regular food pellets. No adverse effects of the plastic-containing food on the feeding activity or behavior of the fish were observed during a period of two weeks in this preliminary experiment. Fish used in the preliminary experiment were sacrificed after two weeks and dissected. Plastics were readily visible in the GIT (Fig. S1†) which gives evidence of their unhampered feeding activity. There was no evidence of accumulation of plastic or blocking of the GIT by the plastic microspheres.
Prior to feeding the fish with PCB-contaminated pellets, one fish from each tank was sacrificed to confirm the absence of PCB contamination in our setup (data not shown). Feed contaminated with PCB 18, PCB 40, PCB 128 and PCB 209 was fed as a single meal each on two consecutive days at 0.5% of bodyweight (control and treatment). Contaminated food was allowed to pass through the digestive tract for three days prior to the start of the depuration experiment to guarantee its evacuation while feeding daily at 0.5% of bodyweight with uncontaminated commercial food pellets. Previous experiments have shown that the digestive emptying rate of a single meal is less than five days in our test species.31,32 During the depuration phase, fish in the control tank were fed commercial food pellets once per day at a rate of 0.5% of their bodyweight. In the treatment tank, fish were fed pellets prepared with polyethylene microspheres at 0.5% of their body weight calculated on the basis of the plastic-free pellets to ensure that both groups received the same nutritional value. Four fish from the control and treatment tank as well as one blank fish were collected and sacrificed by severing the spine after 0, 21 and 43 days. Three individual fish were analyzed after 63 days. Their total length, total wet weight and gutted wet weight were recorded.
Analytical methods
Each entire fish (control, treatment and blank) was homogenized using a Büchi mixer B-400. On sampling days 0, 21 and 43 the four fish homogenates were pooled (within the corresponding control or treatment group) and extracted following a modified version of the Jensen et al. exhaustive solvent extraction protocol.33 Fish collected on sampling day 63 were homogenized and analyzed individually.
Briefly, to an aliquot of about 5 g of whole fish homogenate in a 90 mL centrifuge-tube (n = 3 to 4), 50 μL of a surrogate standard in n-hexane (4 ng μL−1) was added. The sample was extracted with 20 mL n-hexane/acetone (1
:
3), 20 mL hexane/diethyl ether (9
:
1) and 10 mL n-hexane respectively, using ultrasonication for 10 minutes each time. After extraction, the samples were centrifuged and the supernatant transferred to another centrifuge tube. The combined organic phases were washed with 30 mL 0.9% sodium chloride in 0.1 M phosphoric acid, and transferred to a pre-weighed beaker. The solvent was allowed to evaporate overnight after addition of 10 mL ethanol which facilitates the evaporation process. After gravimetric determination of the fat content, the residue was redissolved in 5 mL n-hexane and treated with 5 mL concentrated sulphuric acid for lipid removal. After removal of the upper organic phase the acid phase was washed again with 3 mL n-hexane. The organic phases were combined and reduced to 1 mL and analysed by GC/MS after addition of 50 μL of the volumetric standard in n-hexane (1 ng μL−1). All analytical methods were conducted with glassware blanks and a blank fish.
Gas chromatographic separation was performed on a Thermo Scientific Trace 1310 gas chromatograph using a 30 m × 0.25 mm i.d. TG-5SILMS column with a 0.25 μm film-thickness. Injections were made on a PTV-injector in the splitless mode (260 °C) with the GC oven at 60 °C. This was held for 2 min, raised to 325 °C at a rate of 30 °C min−1. Mass spectrometric determination was made using a Thermo Scientific ISQ LT single quadropole mass spectrometer in electron impact mode.
Data analysis
The total wet weight of sampled fish was natural log transformed and a linear regression model was applied to calculate growth rates. Further, we tested if the slopes differed significantly between the control and treatment group. Results were considered statistically significant at p < 0.05. To estimate the physical condition of the fish, the Fulton's condition factor, K,34 was applied. Using the recorded fish data, the condition factor is calculated as the ratio of the gutted fish weight (Wgut in gram) and its cubed length (L in cm)
.
The PCB concentrations were lipid-normalized and natural log transformed. The elimination rate constants k2 (y = k2x + b), the coefficients of determination, the 95% confidence intervals of the slopes, and the half-times t1/2
were calculated and plotted using GraphpadPrism 6.
Modeling
We evaluated our experimental results against the Arnot and Gobas first-order kinetic bioaccumulation model30 by comparing elimination kinetics measured in our in vivo study to modeled half-times for clearance of PCBs by fish in scenarios with and without microplastic in the diet. We selected log
KOW = 6 and a negligible biotransformation rate constant of 10−6 per day to represent a generic persistent PCB congener in these calculations. We further parameterized the model with conditions that are representative of our in vivo experiment; i.e. 80 g fish with 7.5% lipid content that were fed at a rate of 0.5% of bodyweight per day, and with a growth rate constant fit to the experimentally determined growth rate. Plastic was modeled as “non-lipid organic matter” (NLOM) in the diet that is not assimilated from the GIT by fish, and that contributes to the fugacity capacity of the feces. We assumed the fugacity capacity of plastic to be equal to that of octanol, which is consistent with polyethylene/water partition ratios that have been measured for PCBs.35 In contrast to previous studies that describe scenarios in which feed is replaced by microplastic in the diet,27,28 in our model scenarios and our in vivo experiment microplastic was added as an extra component to the 0.5% of bodyweight per day ingestion rate of fish feed. We compared the modeled whole body elimination rate constants for the treatment and control groups against the rate constants measured in our in vivo study.
Results
Fish health
No signs of adverse health effects were observed during the experiment. One fish died in the control group for unknown reasons. Plotting the wet weight of the fish versus time yielded a growth rate constant of 0.0062 d−1 (plastic treatment; F-value: 28.04, p < 0.05) and 0.0056 d−1 (control, p < 0.05) (see Fig. 1a) indicating that fish increased in bodyweight by over 57% in the treatment (statistically significant) and 43% in the control (not statistically significant) during the course of the experiment. The difference in the growth rate of the control and treatment groups was not statistically significant. On average, fish in the experiment were growing at a rate that corresponds to a doubling of weight in 115 days. The lipid content of the pooled fish homogenates averaged 9.1% ± 1.7% for the plastic-treated group and 8.0% ± 1.6% for the control group (see Fig. 1b). The highest and lowest lipid contents in individual fish were 13% and 7.1%, respectively. The condition factor of the plastic-fed fish and the control fish did not show any differences; averaged over time it was 1.05 ± 0.03 and 1.03 ± 0.09 for the plastic treatment and the control, respectively (see Table S1 and Fig. S2 in ESI†).
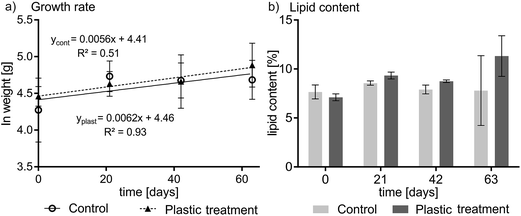 |
| Fig. 1 Growth rate (a) and lipid content (b) over the 63 day depuration experiment. (a) Fish grew by 43% and 57% in the control and treatment group, respectively. Error bars indicate the inter-individual variation in total wet weight. (b) The lipid content showed no trend, and averaged 8.0% ± 1.6% and 9.1 ± 1.7% for the control and the plastic treatment, respectively. Error bars in (b) represent the variability in repeated lipid determinations for the pooled homogenate of four fish on days 0, 21, and 42, and represent variability between three individual fish on day 63. | |
Experimental PCB concentrations
The recovery of the surrogate standards 13C PCB 128 (82% ± 7%) and 13C 209 (86% ± 5%) which were added after homogenization was high and consistent. The slopes of the kinetic plots (Fig. 2) indicate that the PCB elimination rate constants in the plastic treatment group were slightly lower than for the control group (k2 plastic = −0.0008 d−1 to −0.0081 d−1versus k2 control = −0.0076 d−1 to 0.0178 d−1) which is reflected in longer calculated elimination half-times for the treatment group (t1/2 = 86 d to 866 d) than for the control group (t1/2 = 39 d to 92 d) (see Table S2 in ESI†). There was no statistically significant difference in the depuration of PCBs between the plastic-fed fish and the control fish. This is reflected by the 95% confidence bands of the control and treatment groups which overlap for each PCB congener (see Fig. 2a–d) (for detailed PCB concentrations see Fig. S3†).
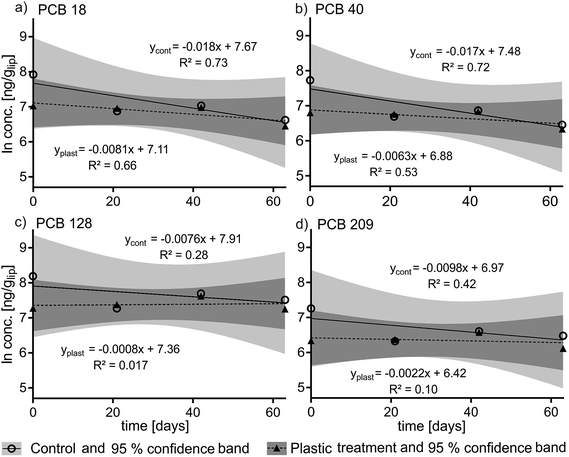 |
| Fig. 2 Kinetic plots (natural log-transformed and lipid-normalized PCB concentrations over time) of the elimination rates of PCB 18 (a), PCB 40 (b), PCB 128 (c) and PCB 209 (d) in the control (“cont”) and the plastic (“plast”) treatment over a depuration time of 63 days. | |
Modeling
In our model scenario parameterized to correspond to the experimental conditions, fish fed a diet including microplastic cleared half of their PCB body burden in 24 days, compared to t1/2 = 86 to 866 days that was observed in the in vivo experiment. The model attributes 20% of losses in the plastic treatment group to growth dilution, 72% to fecal egestion, and 8% to gill ventilation. In the model scenario, fish in the control group fed a diet without plastic cleared half of their PCB body burden in 82 days (compared to t1/2 = 39 to 92 days observed in the control group in the in vivo experiment) with 71% of losses by growth dilution, 3% by fecal egestion and 26% by gill ventilation. In the model scenario the PCB concentrations in the plastic treatment group at the end of the 63 day depuration period were a factor of 3.7 lower than in the control group.
Discussion
We did not observe a statistically significant difference in the depuration rate constants between the control and the treatment groups in our in vivo experiment. The most notable difference in PCB concentrations between the two groups is the difference in concentration between the control and treatment groups at t0 (see Fig. 2), which accounts for the higher elimination rate constants k2 calculated for the control group compared to the treatment group. The variability in contamination of fish might reflect differences in their individual fitness and thus their ability to compete for and consume the contaminated food. Controlled feeding to guarantee equal food intake, or delivering a dose of chemical by intraperitoneal injection would reduce inter-individual variability and could be considered for future studies.36
We observed an increase in fish body weight during the experiment in both the treatment and control groups (Fig. 1a), and our model scenario parameterized to represent the real experiment indicates that growth dilution should make a large contribution to the overall depuration rate in both groups.37 The average growth rate of fish in the experiment implies that PCB concentrations should fall with a half-time of 115 days in both groups due to growth dilution alone. The median values of the experimentally determined half-times for elimination of PCBs ranged from 39 to 92 days in the control group and from 86 to 866 days in the plastic treatment group for the different PCBs (Table S2†). The 95% confidence intervals of the elimination half-times for the different congeners overlap 115 days in all cases (Table S2†), however the observed depuration rate of PCBs in the control group corresponded better to the expectations based on the growth rate of the fish than the depuration rate in the treatment group. Therefore it seems more likely that the PCB concentrations at t0 for the treatment group were at the low-end of the range of variability than that the t0 concentrations in the control group were at the high-end (Fig. 2).
Depuration rate constants calculated in our modeling scenario imply that PCB concentrations in the plastic treatment group should have been a factor of 3.7 lower than in the control group at the end of the 63 day depuration period. A factor of 3.7 difference between the two groups is within the range of uncertainty of bioaccumulation studies38–40 which suggests that the difference between the two groups might exist and not have been detected in our experiment.
Another possibility is that the apparent discrepancy between the model scenario and our observations in the real experiment is due to kinetic limitations on the mobilization of PCBs from storage lipids inside the fish, or to kinetic limitations on the sorption of contaminants to the polyethylene microspheres in the GIT. The Arnot and Gobas model30 that we used includes kinetic limits on the transfer of chemicals between the GIT and the body of the fish, but assumes equilibrium partitioning within the fish and within the GIT. Physiologically based pharmacokinetic models for fish suggest that the time-scale for mobilization of hydrophobic organic chemicals similar to PCBs out of storage lipids is on the order of 5 to 10 days41,42 which is faster than the 63 day duration of our experiment, and thus does not provide support for the idea that kinetic limitations within the fish were the source of the discrepancy. On the other hand, PCBs require several weeks to reach equilibrium partitioning between polyethylene and water,35 and the gut passage time for food in rainbow trout is less than 5 days.31 Therefore it is plausible that the model assumption of equilibrium partitioning in the GIT is not appropriate, and that assumption could give rise to overestimation of the influence of microplastic ingestion on the depuration rate of PCBs by fecal elimination.
This study still leaves a number of questions unexplored, such as gut extraction efficiency and the influence of microplastic characteristics such as the polymer type, size and sorption capacity. Smaller plastic particles with larger surface area-to-volume ratios should have faster kinetics of (de)sorption.12 Additionally, large indigestible food items are known to have longer gut residence times,43 thus potentially increasing the time available for sorbing chemicals. The presence of surfactants has been shown to enhance desorption of chemicals from microplastic under simulated physiological conditions44 and may possibly counteract our investigated mechanism by the formation of micelles. These miscelles might lower the thermodynamic gradient from the GIT wall into the plastic by increasing the capacity of the gut content for the compounds.44 In contrast to model scenarios described elsewhere in the literature27,28 the polyethylene microspheres and the test organisms in our experiment were not pre-equilibrated prior to ingestion which would be an exposure scenario that is more likely to occur in nature.
This study was not undertaken with a focus on the condition of the fish, however the observed increase in fish bodyweight (Fig. 1) indicates sufficient nutrition and efficient food conversion. In the larvae of Chironomus riparius (Chironomids), exposure to nanofullerene (C60) caused shortened and damaged microvilli structure which negativeley affected food consumption and growth.45 Mussels exposed to high microplastic concentrations showed internal bruising and inflammatory responses upon ingestion.46,47 Until now, most field studies on microplastic ingestion by fish found particle sizes small enough not to cause obstructions.48–51 One experimental study reported the accumulation of 5 μm but not 20 μm sized polystyrene microspheres in fish liver52 while Batel et al. (2016) reported the absence of physical harm when zebrafish were fed 10–20 μm polystyrene particles.53 Batel et al. (2016) revealed one incidence of polystyrene microspheres migrating into intestinal epithelium cells.53 This gives rise to the question of an upper and lower size range of microplastics contributing to different effects. The current study indicates that 250 μm polyethylene microspheres present a low risk of lesions and migration in Rainbow Trout.
Our experimental temperature (13 °C) was within the optimum temperature range for growth of trout (10 to 15 °C)54,55 which can partially explain the substantial increase in body weight. Although feed was given at 0.5% of the bodyweight per day, which is at the low end of the maintenance ration,56 the trout grew, indicating sufficient food conversion efficiency. This stands in contrast with the finding that the uptake of microplastic may disturb the metabolism of lipid and energy in fish liver.52 Insightful pathological investigations were not included in this study and scope remains for such studies in the future.
The plastic content of the ingested food used in this study (i.e. 40% by weight) is the maximum we could achieve while maintaining cohesive food pellets. The experimental conditions were not intended to be representative of the real environment but to maximize fecal elimination of pollutants from the GIT, however no influence of plastic in the diet on the depuration of PCBs could be observed. Our results are consistent with the conclusion drawn by Koelmans et al. in a recent critical review22 that microplastic is not likely to be a major modulating factor on the depuration of persistent hydrophobic chemicals from fish in the real environment. Fish condition factors, lipid contents and growth rates observed in our study do not indicate a strong influence of ingested 250 μm polyethylene microspheres on the nutritional status and growth in Rainbow Trout.
Conflict of interset
The authors declare no competing financial interest.
Acknowledgements
This project was funded by the Swedish Research Council FORMAS (project number 216-2013-1010), “Ecological and ecotoxicological effects of microplastics and associated contaminants on aquatic biota”. CDR's participation was partially funded by a grant from the Baltic Ecosystem Adaptive Management (BEAM) research program. CDR and MAE contributed equally to this work, and should be recognized as co-first authors.
References
- J. G. B. Derraik, Mar. Pollut. Bull., 2002, 44, 842–852 CrossRef CAS PubMed.
- S. B. Sheavly and K. M. Register, J. Polym. Environ., 2007, 15, 301–305 CrossRef CAS.
-
GESAMP, "Sources, Fate and Effects of Microplastics in the Marine Environment: a Global Assessment” (Kershaw, P. J., ed.). IMO/FAO/UNESCO-IOC/UNIDO/WMO/IAEA/UN/UNEP/UNDP Joint Group of Experts on the Scientific Aspects of Marine Environmental Protection). Report, London, 2015, pp. 1020–4873 Search PubMed.
-
A. Andrady, in Marine Anthropogenic Litter, ed. M. Bergmann, L. Gutow and M. Klages, Springer International Publishing, Cham Heidelberg New York Dordrecht London, 2015, vol. 1, pp. 57–72 Search PubMed.
- B. Gewert, M. M. Plassmann and M. MacLeod, Environ. Sci.: Processes Impacts, 2015, 17(9), 1513–1521 CAS.
- J. Ivar do Sul and M. Costa, Environ. Pollut., 2014, 185, 352–364 CrossRef CAS PubMed.
- M. Gregory, Philos. Trans. R. Soc. London, Ser. B, 2009, 364, 2013–2025 CrossRef PubMed.
- L. A. Jantz, C. L. Morishige, G. L. Bruland and C. A. Lepczyk, Mar. Pollut. Bull., 2013, 69, 97–104 CrossRef CAS PubMed.
- S. C. Gall and R. C. Thompson, Mar. Pollut. Bull., 2015, 92, 170–179 CrossRef CAS PubMed.
- S. L. Wright, R. C. Thompson and T. S. Galloway, Environ. Pollut., 2013, 178, 483–492 CrossRef CAS PubMed.
- E. Teuten, S. Rowland, T. Galloway and R. Thompson, Environ. Sci. Technol., 2007, 41, 7759–7764 CrossRef CAS PubMed.
- E. Teuten, J. Saquing, D. Knappe, M. Barlaz, S. Jonsson, A. Bjorn, S. Rowland, R. Thompson, T. Galloway, R. Yamashita, D. Ochi, Y. Watanuki, C. Moore, P. Viet, T. Tana, M. Prudente, R. Boonyatumanond, M. Zakaria, K. Akkhavong, Y. Ogata, H. Hirai, S. Iwasa, K. Mizukawa, Y. Hagino, A. Imamura, M. Saha and H. Takada, Philos. Trans. R. Soc. London, Ser. B, 2009, 364, 2027–2045 CrossRef CAS PubMed.
- M. Cole, P. Lindeque, C. Halsband and T. Galloway, Mar. Pollut. Bull., 2011, 62, 2588–2597 CrossRef CAS PubMed.
- K. Syberg, F. R. Khan, H. Selck, A. Palmqvist, G. T. Banta, J. Daley, L. Sano and M. B. Duhaime, Environ. Toxicol. Chem., 2015, 34, 945–953 CrossRef CAS PubMed.
- H. Lee, W. J. Shim and J.-H. Kwon, Sci. Total Environ., 2014, 470–471, 1545–1552 CrossRef CAS PubMed.
- Y. Mato, T. Isobe, H. Takada, H. Kanehiro, C. Ohtake and T. Kaminuma, Environ. Sci. Technol., 2001, 35, 318–324 CrossRef CAS PubMed.
- E. M. Chua, J. Shimeta, D. Nugegoda, P. D. Morrison and B. O. Clarke, Environ. Sci. Technol., 2014, 48, 8127–8134 CrossRef CAS PubMed.
- M. A. Browne, S. J. Niven, T. S. Galloway, S. J. Rowland and R. C. Thompson, Curr. Biol., 2013, 23, 2388–2392 CrossRef CAS PubMed.
- E. Besseling, A. Wegner, E. Foekema, M. van den Heuvel-Greve and A. Koelmans, Environ. Sci. Technol., 2013, 47, 593–600 CrossRef CAS PubMed.
- C. Rochman, E. Hoh, T. Kurobe and S. Teh, Sci. Rep., 2013, 3, 3263 Search PubMed.
- C. M. Rochman, T. Kurobe, I. Flores and S. J. Teh, Sci. Total Environ., 2014, 493, 656–661 CrossRef CAS PubMed.
- A. A. Koelmans, A. Bakir, G. A. Burton and C. R. Janssen, Environ. Sci. Technol., 2016, 50, 3315–3326 CrossRef CAS PubMed.
- G. Andreas Moser and M. S. McLachlan, Chemosphere, 1999, 39, 1513–1521 CrossRef.
- S. Rohde, G. A. Moser, O. Päpke and M. S. McLachlan, Chemosphere, 1999, 38, 3397–3410 CrossRef CAS PubMed.
- R. J. Jandacek, J. E. Heubi, D. D. Buckley, J. C. Khoury, W. E. Turner, A. Sjödin, J. R. Olson, C. Shelton, K. Helms, T. D. Bailey, S. Carter, P. Tso and M. Pavuk, J. Nutr. Biochem., 2014, 25, 483–488 CrossRef CAS PubMed.
- A. Geusau, E. Tschachler, M. Meixner, S. Sandermann, O. Päpke, C. Wolf, E. Valic, G. Stingl and M. McLachlan, Lancet, 1999, 354, 1266–1267 CrossRef CAS.
- A. A. Koelmans, E. Besseling, A. Wegner and E. M. Foekema, Environ. Sci. Technol., 2013, 47, 7812–7820 CrossRef CAS PubMed.
- T. Gouin, N. Roche, R. Lohmann and G. Hodges, Environ. Sci. Technol., 2011, 45, 1466–1472 CrossRef CAS PubMed.
- D. Herzke, T. Anker-Nilssen, T. H. Nøst, A. Götsch, S. Christensen-Dalsgaard, M. Langset, K. Fangel and A. A. Koelmans, Environ. Sci. Technol., 2016, 50, 1924–1933 CrossRef CAS PubMed.
- J. A. Arnot and F. A. P. C. Gobas, Environ. Toxicol. Chem., 2004, 23 Search PubMed.
- R. Xiao, M. Adolfsson-Erici, G. Akerman, M. S. McLachlan and M. MacLeod, Environ. Toxicol. Chem., 2013, 32, 2695–2700 CrossRef CAS PubMed.
- J. W. Nichols, P. N. Fitzsimmons, F. W. Whiteman, D. W. Kuehl, B. C. Butterworth and C. T. Jenson, Drug Metab. Dispos., 2001, 29, 1013–1022 CAS.
- S. Jensen, L. Reutergårdh and B. Jansson, FAO Fish. Tech. Pap., 1983, 212, 21–33 Search PubMed.
- R. D. M. Nash, A. H. Valencia and A. J. Geffen, Fisheries, 2006, 31, 236–238 Search PubMed.
- Y. Choi, Y.-M. Cho and R. G. Luthy, Environ. Sci. Technol., 2013, 47, 6943–6950 CrossRef CAS PubMed.
- M. D. Kinkel, S. C. Eames, L. H. Philipson and V. E. Prince, J. Visualized Exp., 2010, 42, e2126 Search PubMed.
-
D. N. Brooke and M. J. Crookes, Bioaccumulation of Chemicals in Fish: Investigating the Relationship between Depuration Rate Constant and Fish Lipid Content, Environment Agency, Bristol, 2014 Search PubMed.
- H. Selck, K. Drouillard, K. Eisenreich, A. A. Koelmans, A. Palmqvist, A. Ruus, D. Salvito, I. Schultz, R. Stewart, A. Weisbrod, N. W. van den Brink and M. van den Heuvel-Greve, Integr. Environ. Assess. Manage., 2012, 8, 42–63 CrossRef CAS PubMed.
- M. Hauck, H. W. M. Hendriks, M. A. J. Huijbregts, A. M. J. Ragas, D. van de Meent and A. J. Hendriks, Environ. Toxicol. Chem., 2011, 30, 403–412 CrossRef CAS PubMed.
- A. J. Hendriks, A. van der Linde, G. Cornelissen and D. T. H. M. Sijm, Environ. Toxicol. Chem., 2001, 20, 1399–1420 CrossRef CAS PubMed.
- J. W. Nichols, J. M. McKim, M. E. Andersen, M. L. Gargas, H. J. Clewell 3rd and R. J. Erickson, Toxicol. Appl. Pharmacol., 1990, 106, 433–447 CrossRef CAS PubMed.
- R. Xiao, J. A. Arnot and M. MacLeod, Chemosphere, 2015, 138, 89–95 CrossRef CAS PubMed.
- J. dos Santos and M. Jobling, J. Fish Biol., 1991, 38, 187–197 CrossRef.
- A. Bakir, S. J. Rowland and R. C. Thompson, Environ. Pollut., 2014, 185, 16–23 CrossRef CAS PubMed.
- G. C. Waissi-Leinonen, E. J. Petersen, K. Pakarinen, J. Akkanen, M. T. Leppänen and J. V. K. Kukkonen, Environ. Toxicol. Chem., 2012, 31, 2108–2116 CrossRef CAS PubMed.
- M. A. Browne, A. Dissanayake, T. S. Galloway, D. M. Lowe and R. C. Thompson, Environ. Sci. Technol., 2008, 42, 5026–5031 CrossRef CAS PubMed.
- N. von Moos, P. Burkhardt-Holm and A. Kohler, Environ. Sci. Technol., 2012, 46, 11327–11335 CrossRef CAS PubMed.
- E. M. Foekema, C. De Gruijter, M. T. Mergia, J. A. van Franeker, A. J. Murk and A. A. Koelmans, Environ. Sci. Technol., 2013, 47, 8818–8824 CAS.
- D. Neves, P. Sobral, J. L. Ferreira and T. Pereira, Mar. Pollut. Bull., 2015, 101, 119–126 CrossRef CAS PubMed.
- C. D. Rummel, M. G. J. Löder, N. F. Fricke, T. Lang, E.-M. Griebeler, M. Janke and G. Gerdts, Mar. Pollut. Bull., 2016, 102, 134–141 CrossRef CAS PubMed.
- M. B. Phillips and T. H. Bonner, Mar. Pollut. Bull., 2015, 100, 264–269 CrossRef CAS PubMed.
- Y. Lu, Y. Zhang, Y. Deng, W. Jiang, Y. Zhao, J. Geng, L. Ding and H. Ren, Environ. Sci. Technol., 2016, 50, 4054–4060 CrossRef CAS PubMed.
- A. Batel, F. Linti, M. Scherer, L. Erdinger and T. Braunbeck, Environ. Toxicol. Chem., 2016 DOI:10.1002/etc.3361.
- J. R. Brett, J. E. Shelbourn and C. T. Shoop, J. Fish. Res. Board Can., 1969, 26, 2363–2394 CrossRef.
- E. Austreng, T. Storebakken and T. Åsgård, Aquaculture, 1987, 60, 157–160 CrossRef.
- Biomar, EFICO Alpha 714 Feeding Chart, 5/2/2015, http://www.biomar.com/Countries/common%20ceu/Datasheets/Datasheets%20Baltic/Trout%20Baltic/Trout%20German%20Forelle/DE%20EFICO%20Alpha%20717%203-6%20mm%20Trout%20%280,84%20-%201,3%29.pdf?epslanguage=de.
Footnotes |
† Electronic supplementary information (ESI) available: Additional material as cross-referenced throughout. See DOI: 10.1039/c6em00234j |
‡ Current address: Department of Bioanalytical Ecotoxicology, Helmholtz Centre for Environmental Research – UFZ, Permoserstraβe 15, DE-04318 Leipzig, Germany. |
|
This journal is © The Royal Society of Chemistry 2016 |
Click here to see how this site uses Cookies. View our privacy policy here.