DOI:
10.1039/D3TB01842C
(Perspective)
J. Mater. Chem. B, 2024,
12, 64-78
Intestinal retentive systems – recent advances and emerging approaches
Received
14th August 2023
, Accepted 15th November 2023
First published on 28th November 2023
Abstract
Intestinal retentive devices (IRDs) are devices designed to anchor within the lumen of the intestines for long-term residence in the gastrointestinal tract. IRDs can enable impactful medical device technologies including sustained oral drug delivery systems, indwelling sensors, or real-time diagnostics. The design and testing of IRDs present a myriad of challenges, including precise deployment of the device at desired intestinal locations, secure anchoring within the gastrointestinal tract to allow for natural function, and safe removal of the IRD at user-defined times. Advancing the state-of-the-art of IRD is an interdisciplinary effort that requires innovations such as new materials, novel anchoring mechanisms, and medical device design with consistent input from clinical practitioners and end-users. This perspective briefly reviews the current state-of-the-art for IRDs and charts a path forward to inform the design of future concepts. Specifically, this article will highlight materials, retention mechanisms, and test beds to measure the efficacy of IRDs and their mechanisms. Finally, potential synergies between IRD and other medical device technologies are presented to identify future opportunities.
1. Introduction
The gastrointestinal (GI) tract is an exciting testbed for resident medical devices and one that is brimming with innovative research opportunities. Home to over 400 million neurons and 100 trillion microbes, the gut is fundamental to maintaining homeostasis1,2 through host immunity, endocrine and motor functions, and nutrient absorption.3 Much like the skin, the gut is a complex organ system with significant surface area for minimally invasive biointeraction, both interventional and diagnostic, with wide-scale potential. GI pathologies severely affect the quality of life of patients and include irritable bowel syndrome (IBS), celiac disease, Crohn's disease, intestinal bleeding, ulcers, etc.4–6 Approximately 45 million people in the US are affected by irritable bowel syndrome (IBS)7 while Celiac disease8 and peptic ulcers affect 2 and 4.6 million Americans, respectively, each year.9 Intestinal retentive devices (IRDs) can leverage the accessibility of the GI tract to offer new paradigms for diagnosing and managing these debilitating diseases.
Gastro-retentive devices have been explored for a range of applications, such as biosensing, weight loss to sustained drug delivery systems for the oral delivery of medications.10–12 The oral route is the safest and most convenient route for the delivery of medications. However, patient compliance with oral medications drops significantly with the frequency of administration particularly in the case of treating chronic illnesses.13 Non-compliance causes about 100k premature deaths annually and contributes to over $100B in preventable hospital costs each year.14–20 Recent advancements in creating long-term gastroretentive medical devices include systems such as the ingestible hydrogel devices developed by Liu et al.,21 ingestible unfolding systems developed by Bellinger et al. for malaria elimination,22 and by Kirtane et al. for HIV therapy,23 and contraceptives.24 Many of these devices have focused on the delivery of small molecules in the stomach and show favorable pharmacokinetics compared to traditional oral pills but have limited utility in delivering macromolecular therapeutics such as proteins and peptides, which have poor (<1%) oral bioavailability.25 In comparison, the highly vascularized mucosa in the small intestine makes it an attractive site for drug delivery.26,27
As previously referenced, the nervous system of the gut helps coordinate peristalsis and detects and responds to nutrients, hormones, pathogens, and toxins.28,29 Therefore, there has been a growing interest in the bioelectronics community to develop non-invasive gut-interfacing electronics, which can permit the real-time monitoring of diagnostic indicators as well as the modulated release of therapeutic compounds. Research efforts in resident bioelectronics have been bolstered by the progression of miniaturized electronics,30 biocompatible power supplies,31–33 wireless power transmission, and novel biosensing pathways.34,35 Early embodiments of this technology include smart pills such as PillCam, designed to image previously inaccessible diseased tissue sites and reduce the need for more invasive endoscopic procedures.30,36–38 Additional studies have highlighted the therapeutic benefit of regulating gut microbiota39 and stimulation of nerve endings in the gut.40 Nonetheless, there are still significant unmet technical challenges to design, test, and ultimately deploy indwelling ingestible devices including continuous peristalsis,41 fluctuating fluid volume,42,43 rapid and dynamic mucus overturn,44,45 and gradual enzymatic and acidic/basic degradation of devices.22
IRD must address several key design challenges: (1) How can devices and associated retention mechanisms be stably ingested and deployed in a timely manner? (2) How can IRD reside within the GI tract while sustaining natural functions? (3) How can devices be removed from the host at the end of their functional lifecycle? This article will discuss the current state-of-the-art for IRDs, present challenges that confront the broad implementation of IRD, and will conclude with materials and device design innovations that could potentially overcome said challenges.
2. Mechanisms of retention
IRDs utilize two primary mechanisms to achieve sustained retention within the gut: chemo-adhesion across the tissue-device interface and mechano-adhesion which uses structural elements of the device that interact with the intestinal epithelium (Fig. 1).
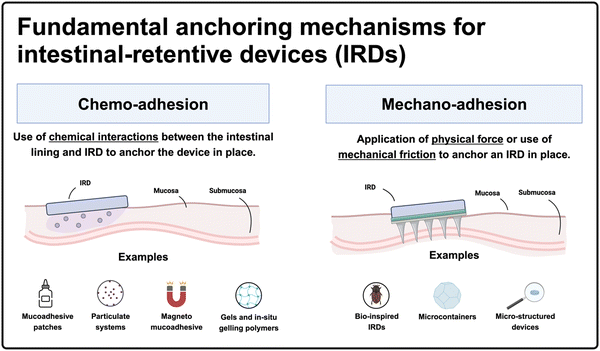 |
| Fig. 1 Summary figure. Infographic outlining the two fundamental anchoring mechanisms for IRDs as well as the seven sub-categories explored in this article (created with BioRender). | |
2.1. Chemoadhesion
The intestinal lumen is lined with a lubricious mucus layer, with thickness varying across different sections of the small intestine (based on experiments conducted with the rat intestinal tract), measuring on average 170 μm in the duodenum, 120 μm in the jejunum, and 480 μm in the ileum.46–48 Mucus is primarily composed of water (>95%) and mucins, which are high-molecular-weight glycoproteins that offer several opportunities to develop mucoadhesive systems.44,49 Various interactions, such as covalent disulfide bonds between thiomers and cysteine-rich domains of glycoproteins, electrostatic interactions between cationic chitosan and sialic acid moieties, hydrogen bonding, physical entanglements, and van der Waals forces, can generate chemo-adhesive devices.44,50 Based on the structure of chemo-adhesive devices they can be divided into four categories: (1) mucoadhesive patches, (2) particulate systems (3) magneto-mucoadhesives and (4) gels and in situ gelling polymers. In this section, we discuss each of these and provide examples.
2.1.1. Mucoadhesive patches.
Mucoadhesive patches are multi-layered devices that consist of a mucoadhesive layer which adheres to the intestinal mucosa, and a water-impermeable backing layer, which confers at least two advantages: (a) it prevents the discharge of loaded drug in the lumen thereby resulting in biased transport of the drug into the mucosa, and (b) it prevents proteolytic enzymes from reaching loaded proteins on the luminal side, thus preserving the drug's effectiveness by avoiding accelerated degradation51 (Fig. 2a). The adhesive layer often consists of polysaccharides like pectin, Carbopol, chitosan, carboxymethyl cellulose, ethyl cellulose, alginates, and gelatin since they are biocompatible, bioresorbable, and hydrogels by nature.45,52,53 Taipaleenmäki et al. have discussed advancements in polymers for intestinal adhesion at length.45 Water-impermeable backings are often composed of ethyl cellulose or cellulose acetate.53,54 For long-term IRDs, one of the most common limitations is premature release due to the constant passage of food and surface fouling caused by the biological fluids contained in the lumen.55–58 Lee et al. solved this issue by introducing structural changes to the water-impermeable cellulose acetate layer to mimic the morphology of lotus leaf, which inherently imparts superhydrophobicity to the leaf, and further chemically functionalized the layer via vapor-phase fluorination with perfluorinated silane and then lubricated with a biocompatible, medical-grade perfluorocarbon liquid (Fig. 2b). When used to back Carbopol patches, the modified cellulose acetate backings reduced device interaction with food stuffs and increased the duration of mucosal adhesion of the patches from 7 s to 10 min.54 Researchers developed tri-layered mucoadhesive patches that include a pH-sensitive layer (e.g., Eudragit polymers L or S). This barrier layer restricts premature drug release in acidic environments of the stomach and thereby allows spatially localized drug delivery to the small intestine. Quatre-layered patches usually distinguish the drug layer and mucoadhesive layer. Detailed reports on the structure of mucoadhesive patches have been reported by Kirsch et al.59 and Sanad et al.60
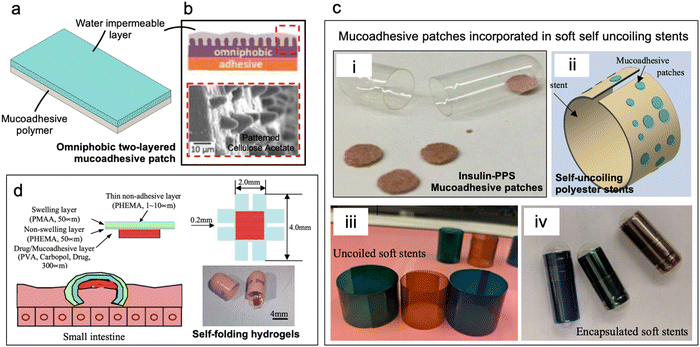 |
| Fig. 2 Mucoadhesive patches. (a) Schematic of a bilayer mucoadhesive patch with a polymeric adhesive layer and a water impermeable backing (b) omniphobic ‘Janus’ device with a lotus leaf patterned cellulose acetate water-impermeable backing reduces food and protein deposition on mucoadhesive patches. (Reproduced from ref. 54 with permission from Wiley, copyright 2016.) (c) (i) Millimeter-scale mucoadhesive patches containing drug permeation enhancer (dimethyl palmitoyl ammonio propanesulfonate) for oral delivery of insulin. (Reproduced from ref. 53 with permission from Wiley, copyright 2016.) (ii) Patches attached to soft uncoiling polyester stents ease contact of patches to intestinal lumen upon uncoiling. (Reproduced from ref. 61 with permission from ASME, copyright 2021.) (iii) Prototypes of polyester soft stents (iv) stents encased in gelatin capsules for oral delivery. (d) Dual layered self-folding hydrogels with swellable backing layer facilitate hooking onto intestinal mucosa. (Reproduced from ref. 62 with permission from Elsevier, copyright 2006.) | |
Banerjee et al. developed ingestible patches to deliver insulin through the intestinal lining for up to 24 h (Fig. 2c(i)).53 The mucoadhesive patches consisted of small adhesive pellets (diam. = 2–5 mm) comprised of Eudragit E PO, pectin, sodium carboxymethyl cellulose, and dimethyl palmitoyl ammonio propanesulfonate (PPS) to facilitate macromolecules absorption. Orally ingested patches were designed to be retained by fitting into the luminal crevices – anatomical features of the small intestine that can entrap the patches. Sarker et al. enhanced the mucoadhesive patches by incorporating them onto the outer layer of polyester-based self-uncoiling stents (Fig. 2c(i–iv)). These stents would automatically uncoil upon reaching the small intestine, allowing the patches to make prompt contact with the intestinal lumen and increase their retention time to approximately 36 h.61
Another example of intestinal mucoadhesive patches includes double-coated mucoadhesive films for enhanced delivery of recombinant Lactococcus lactis. Here a film of sodium alginate (SA) with an inner coating of Lycoat RS 720 – a hydroxypropyl pea starch – is used to enhance mucoadhesion. Maximum values of mucoadhesion were achieved by 1: (1.1) wt/wt of SA: Lycoat (∼0.098 N cm−2).63 He et al. introduced an innovative mucoadhesive patch that employs self-folding hydrogels (Fig. 2d). The patch contains a bonded bilayer with a finger-like structure and a mucoadhesive drug layer. One layer is pH-sensitive crosslinked poly(methacrylic acid) (PMAA) that swells while the other is poly(hydroxyethyl methacrylate) (PHEMA) and swells significantly less than PMAA. The self-folding device curls due to differential swelling of PMAA:PHEMA bilayers upon contact with mucus thus improving mucoadhesion and bioavailability with the PHEMA film acting as a barrier layer.62
Although mucoadhesive patches show promise for temporary adhesion to improve the oral bioavailability of drugs, they are susceptible to mucus overturn and biofouling which limits their efficacy as a drug delivery system.
2.1.2. Particulate systems.
Polymeric particulates represent a distinct class of mucoadhesives that harness materials chemistry and micron-scale dimensions to enhance adhesion. By maximizing the contact area between the mucoadhesive components of the material, these particulates facilitate their penetration into the mucus membrane and crevices of the intestine. Ponchel et al. have provided detailed insights into the mechanism of mucoadhesion of colloidal particulate systems when administered orally in the small intestine.64 To achieve this purpose, commonly used mucoadhesive polymers like chitosan and sodium alginate are employed to prepare nano/micro-particles or spheres. Ali et al. have extensively reviewed the current technology of microspheres for gastroretentive applications.65 Biodegradable ionomer polymers use electrostatic interactions to promote mucoadhesion. This mechanism however is insufficient when there are large volumes of intestinal fluid and rapid mucus turnover.64,66 Consequently, researchers are exploring thiolated modifications of particulate systems that induce covalent bond formation between the particles and the cysteine components of the mucosa.67,68
Moa et al. developed thiol-modified sodium alginate microspheres for sustained drug release in the intestine and in an in vitro test demonstrated that thiolation increased the number of retained microspheres by more than 100%.69 In a separate study, thiolated chitosan particles were investigated for their potential to enhance the absorption of Hesperidin in the intestine for obesity treatment. Although these thiolated particles achieved 6× longer retention times compared to non-thiolated particles, in vivo residence times were only 24 h as measured in mice which is comparable to the state-of-the-art.70
While particulate systems effectively enhance drug permeation through the intestinal epithelium, they tend to aggregate within the villi and are eventually expelled due to mucus turnover. Consequently, their application as intestinal retentive systems is limited.25,64
2.1.3. Magneto-mucoadhesives.
Mucoadhesive patches and particles can incorporate magnetic particles to precisely deploy devices within the small intestine and help improve their retention. Liu et al. developed an orally delivered polyvinyl alcohol hydrogel matrix doped with neodymium-iron-boron magnets which allowed for the retention of the device in the gut for up to 7 days, facilitated by the presence of an external magnet worn on the abdominal skin (Fig. 3a). Further, it enabled localized deployment of living hydrogels doped with synthetic microbes. Microbe-loaded hydrogels were engineered to enable real-time biosensing and perform diagnostic functions at specific sites within the gut.71 In another study, researchers developed a tubular mucoadhesive ring using a silicone rubber matrix doped with ferromagnetic particles (Fig. 3b). The ring features a chitosan-glycerol film to enhance mucoadhesion and other capabilities including localized cargo deployment and liquid capsule release. The chitosan-glycerol film played a vital role in inducing strong mucoadhesion between the soft robot and the mucosa, with a normal adhesion force of up to 20 mN observed for a mucus concentration of 5% and a contact time of 10 s.72 Lee et al. developed a magnetically actuated capsule with the ability to hold multiple mucoadhesive patches prepared with mussel-inspired catechol-conjugated chitosan for targeted drug delivery in cancer treatment. The capsule featured a neodymium magnet for movement within the gut using an externally applied magnetic field, along with an actuation system that allowed for the active release of patches at specific sections. The patches themselves contained magnetic microparticles, enabling hyperthermia of cancerous lesions by alternating magnetic forces and facilitating localized drug delivery. This comprehensive system not only provides precise and controlled deployment of drug-loaded patches to targeted gut lesions but also demonstrates the effectiveness and utility of magnetic systems in gastroretentive medical devices.73
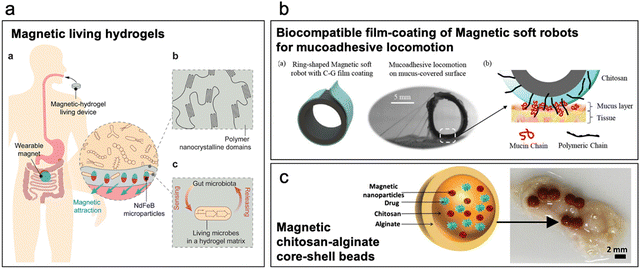 |
| Fig. 3 Magneto-mucoadhesives. (a) Magnetic living hydrogels: PVA hydrogel with NdFeB magnetic microparticles doped with living microbes for real-time biosensing. External magnet is used to retain the hydrogel in the intestine. (Reproduced from ref. 71 with permission from Wiley, copyright 2021.) (b) Tubular silicone-based soft robot is doped with ferromagnetic particles and coated with a chitosan-glycerol film to enhance mucoadhesion to the intestinal mucosa. (Reproduced from ref. 72 with permission from Wiley, copyright 2023.) (c) Magnetic chitosan-alginate core–shell microbeads developed to increase bioavailability of low permeable drugs in presence of external magnetic force. (Reproduced from ref. 74 with permission from Wiley, copyright 2014.) | |
Incorporating magnetic particles into mucoadhesive colloidal systems can significantly improve their adhesion to the intestinal lumen when subjected to an external magnetic field. For example, Teply et al. utilized negatively charged insulin-doped poly(L-lactic-co-glycolic acid) (PLGA) microparticles, which were coupled with positively charged micromagnets to form a complex. Magnetically coupled PLGA microparticles exhibited approximately seven times greater retention compared to non-coupled particles.75 In another study, Seth et al. developed a chitosan-alginate core–shell bead system with magnetic microparticles to increase the residence time and extend the timeline for drug elution thus ultimately improving the bioavailability of drugs with low mucosal permeability (Fig. 3c).74 Mucoadhesives that employ magnetic materials for retention in the GI tract permit creative avenues for materials design and device concepts. However, many of these concepts use exotic materials which complicate the pathway for regulatory approach. Furthermore, many devices and particles require external devices, complicating deployment and potentially reducing the likelihood of patient compliance, which ultimately may limit the adoption of these devices.
2.1.4. Gels and in situ gelling polymers.
Gel-based chemo adhesives are materials that encompass in situ gelling polymers or pre-gelled polymers. In situ gelling polymers react with specific components of the intestine, resulting in the formation of a gel coating on the intestinal surface.76 Li et al. developed an oral solution of in situ gelling polymer with dopamine monomers and hydrogen peroxide that polymerize upon reaching the small intestine (Fig. 4a). Catalase concentrations in the small intestine decompose hydrogen peroxide and release oxygen to accelerate polymerization of polydopamine and form a polydopamine coating on the mucosal lining.77 Polydopamine linings were evaluated for their ability to deliver digestive enzymes, nutrient blockers, and anthelmintic drugs. However, the retention of this coating was found to be limited by mucus turnover. Another example includes therapeutic luminal coatings developed by Lee et al. which were made of sucrose octasulfate aluminium complex and engineered into a coacervate formulation linked via pH-independent electrostatic interactions. The formulation exhibited hydration and dehydration properties. In the hydrated state, the solid powder transformed into a paste-like substance, allowing for effective and continuous physical coverage of the gastrointestinal mucosa.78
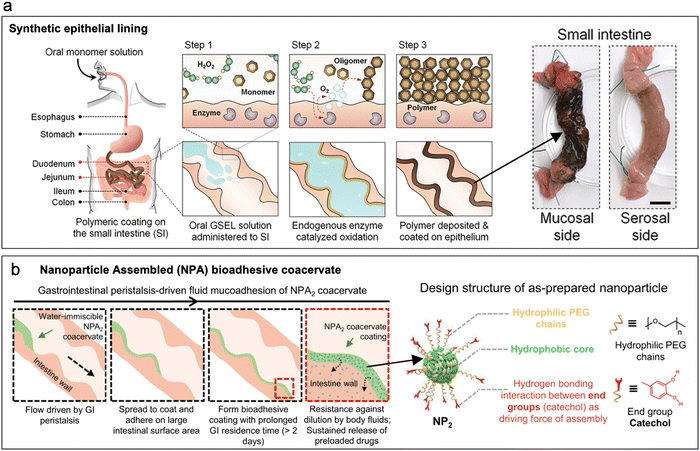 |
| Fig. 4 Gels and in situ gelling polymers. (a) Synthetic intestinal epithelial lining produced by enzyme catalyzed in situ polymerization of dopamine monomers. (Reproduced from ref. 77 with permission from AAAS, copyright 2020.) (b) Nanoparticle-assembled coacervates are an example of pre-gelled coatings that adhere to intestinal lumen via hydrogen bonding between catechol end groups and mucus. (Reproduced from ref. 79 with permission from Nature, copyright 2021.) | |
Pre-gelled polymers establish interfacial bonds with the intestine upon contact. Examples of pre-gelled intestinal coatings include complex nanoparticle coacervates developed by Zhao et al., which were assembled via hydrogen bonding between catechol end groups of the nanoparticles (Fig. 4b). The coacervate adhered to the intestinal lining via hydrogen bonding and displayed a retention time of greater than 2 days in vivo.79 Another noteworthy example is the study conducted by Lin et al., where they explored the mucoadhesive properties of nanocellulosic gels. Their findings revealed that the mucoadhesive behavior of these gels varied depending on the type of cellulose used, with different mechanisms of adhesion observed, such as changes in the zeta potential of the cellulose-mucin complex, entanglements, hydrogen bonding, and coagulation.80
Gel-based polymeric coatings offer a superior solution for forming a uniform and comprehensive coating on the intricate and convoluted lumen of the intestine.79 Unlike the mucoadhesive patches, these coatings have the potential to target a larger surface area within the intestine, enabling more effective drug delivery for conditions like inflammatory bowel syndrome.76,79 However, their retention time is limited to <48 h due to rapid mucus turnover.
2.2. Mechano-adhesion
IRDs can employ mechano-adhesion schemes such as structures that pierce, hook, or otherwise affix to the mucosal lining of the GI tract. These designs may be made of mucoadhesive materials to add a synergistic retention effect. Mechano-adhesives can be distinguished into three broad categories: (1) bio-inspired devices, (2) microcontainers, and (3) micropatterned devices. Here, we discuss these mechanisms and the current state-of-the-art of each of these schemes.
2.2.1. Bio-inspired IRDs.
Marine organisms use various mechanisms to achieve underwater adhesion in complex hydrated environments.81,82 Specifically, octopus and suckerfish have inspired adhesive structures that can be leveraged for intestinal retention.82–84 Parasitic worms have already won the battle against intestinal barriers and can remain in the intestine for several years if untreated. Inspired by their attachment mechanism, researchers have developed intestinal retentive devices. Inspired by hookworms, Xie et al. developed the tissue-attachment mechanism (TAM) which applies microneedles and a vacuum system to mimic the hooks and suction system in hookworms. These devices have shown a record in vivo retention time of six days compared to present systems.85 Inspired by the proboscis of spiny-head worms, Liu et al. 3 D printed barbed microneedles which exhibit a pull-out force of 25 mN and a low penetration force of 1.6 mN (Fig. 5a).86 Building on this mechanism, a spring-microneedle unit was fabricated for integration with pill-based resident systems. Here, the aim was to achieve the required actuation force (∼8 mN) to permit penetration of the barbed microneedles using the spring system.87 A simple yet effective solution, this mechanism was further incorporated into spiny microneedle anchoring drug deposit (SMAD) and evaluated in ex vivo test beds.88
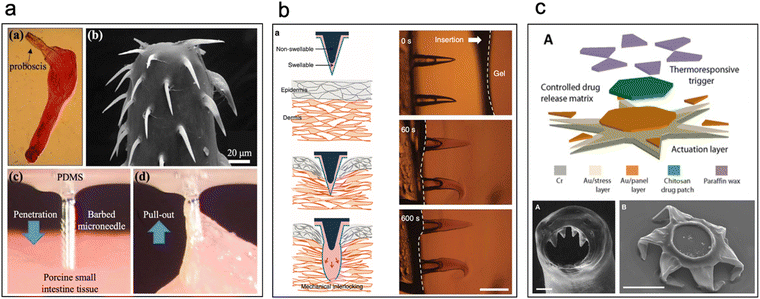 |
| Fig. 5 Bio-inspired IRDs. (a) Barbed microneedles inspired by proboscis of spiny-head worms pierce the intestinal epithelium and hook on to the intestinal mucosa. (Reproduced from ref. 86 with permission from IEEE, copyright 2020.) (b) Biphasic swellable microneedles inspired by swellable proboscis of endoparasite Pomphorhynchus laevis enable robust anchoring in the intestine. (Reproduced from ref. 89 with permission from Springer, copyright 2015.) (c) Theragrippers are micron-sized thermosensitive actuators that curl up autonomously due to the internal physiological temperature of the body which facilitates piercing of intestinal epithelium to enable retention. (Reproduced from ref. 90 with permission from Science, copyright 2020.) | |
Another device inspired by the swellable proboscis of endoparasite Pomphorhynchus laevis was developed by Yang et al. (Fig. 5b). Here, biphasic conical microneedles with a swellable tip of poly(styrene)-block-poly(acrylic acid) (PS-b-PAA) and non-swellable polystyrene core were prepared.89 Minimal insertion force was required to insert said devices into the tissue and post swelling displayed an adhesion force of 4.53 N cm−2 with intestinal tissue [this value is for flexible (PS-elastomer) based microneedles]. Yet another device inspired by a parasite – Hookworms – is Theragrippers which are micron-sized devices (250 μm when open, 150 μm when closed) which comprise thick rigid segments and residually stressed bilayer hinges, capped with a thermosensitive wax layer (Fig. 5c).90 At body temperature the microtips curl up autonomously as the wax softens and pierce the intestinal epithelium to enable retention. These devices show promise as they were retained in rat colon (administered by pneumatic microfluidic controller) and in the upper GI tract of porcine model (administered by endoscopy) for 24 h.
2.2.2. Microcontainers.
Size-dependency of sub-micron-sized polystyrene drug carriers on intestinal adhesion has been extensively studied in the treatment of IBS with size inversely related to the binding ability of the particles to mucosa.91,92 Like mucoadhesive microspheres, these devices, by virtue of their sub-micron-sized shape, can lodge into the crevices of the small intestine and further enter the mucosal layer. Previously, due to the lack of advanced fabrication techniques such as lithography, microfluidics and photopolymerization,93–95 exclusively microencapsulated containers of spherical shapes were studied. However, with the advent of new fabrication techniques, particles with shapes like plug, vase, toroidal, rods, and cones have been prepared.92,96–98
Cylindrical microcontainers with drug loading capacity developed and studied for drug delivery in the small intestine showed a promising increase of 180% oral bioavailability of model drugs like ketoprofen99 and furosemide.100 Mosgaard et al. further investigated the mucoadhesion of hollow microcontainers of cylindrical and triangular shapes, with varied aspect ratios and materials in an ex vivo intestinal perfusion model (Fig. 6a).101 High-aspect-ratio cylindrical microcontainers comprised of SU-8 adhered more tightly to the mucosa under simulated intestinal fluid flow compared to microcontainers with low aspect ratio cylinders prepared with lightweight polycaprolactone. Furthermore, microcontainers with pyramidal geometries adhered more efficiently to the mid-intestinal section because of their sharp edges which allowed easier penetration into the mucosa. Chrisfort et al. further explored the effect of cubic microcontainers and adjunct micropillars on cylindrical containers on colonic mucoadhesion and showed that cubic microcontainers are more efficient in adhering to the mucosa when compared to cylindrical and triangular shaped microcontainers (Fig. 6b). Presence of micropillars did not make a significant impact on adhesion.102 Based on this technology, radiopaque microcontainers with micropillars and arrows103 for X-ray diagnostics have been developed. To improve mucoadhesion and retention of microcontainers in the gut, 3D-printed anchor-like surface structures have been studied. The addition of anchors showed a 2× increase in mucoadhesion when compared to nonstructured controls. Branched out surface structure of anchors eased their penetration into the mucosa to interlock the microcontainers within the mucosa (Fig. 6c).104
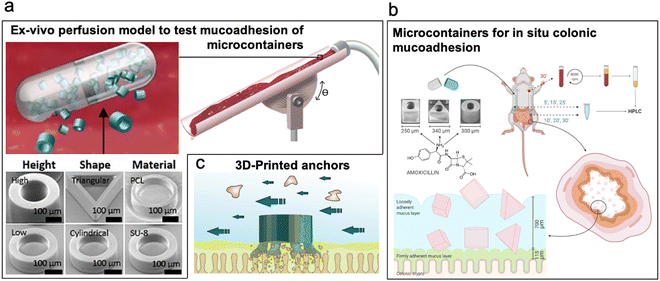 |
| Fig. 6 Microcontainers. (a) Microcontainers with varied structures such as high-aspect-ratio and low-aspect-ratio cylinders and pyramids tested for mucoadhesion via ex vivo perfusion model. (Reproduced from ref. 105 with permission from Elsevier, copyright 2019.) (b) Microcontainers (cubic, cylindrical, and pyramid) containing model drug amoxicillin tested for drug delivery in colon. (Reproduced from ref. 102 with permission from MDPI, copyright 2020.) (c) 3D-printed microcontainers with surface textured anchors for enhanced mucoadhesion. (Reproduced from ref. 104 with permission from ACS, copyright 2020.) | |
2.2.3. Micropatterned devices.
Micropatterned devices resist peristaltic shear in the gut by enhancing friction between intestinal tissue and device substrates. Rose et al.106 provide a detailed overview of prevalent and emerging microfabrication techniques for producing polymeric microstructures. The preferred method for creating micropatterned devices for intestinal retention has been replica molding of microstructures using master molds prepared via either photolithography or 3D printing.107,108
In a study by Kwon et al., micropatterned silicone-based substrates with low-aspect-ratio microposts of varying diameters and spacings were explored for their adhesion to intestinal tissue (Fig. 7a(i)).107 The highest frictional force between the substrates and the intestine occurred when the microposts had a diameter of 140 μm (aspect ratio of 0.89
:
1) and a spacing of 75% of the pillar diameter. Furthermore, the friction force was directly proportional to the applied normal force and the viscosity of the surrounding environment between the microposts and the tissue.
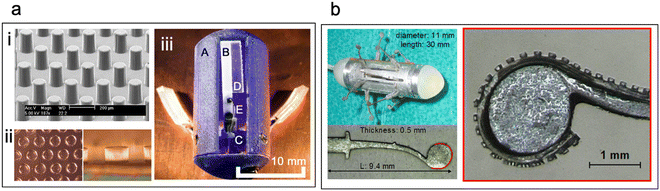 |
| Fig. 7 Micropatterned devices. (a) (i) Silicon-based micropillars for friction enhancement between intestinal mucosa and device substrates. (Reproduced from ref. 107 with permission from IOP, copyright 2006.) (ii) Soft micropillars (aspect ratio 1 : 1) used for three-legged anchoring system for capsule endoscopy (iii) three-legged anchoring system (A) capsule shell, (B) leg, (C) pulley, (D) adhesive pad, (E) cable (Reproduced from ref. 109 with permission from IEEE, copyright 2008.) (b) Nitinol legs with multiple soft-micropillars augment friction in active capsule endoscopy. (Reproduced from ref. 110 with permission from IOP, copyright 2010.) | |
Glass et al. designed a three-legged anchoring system for an endoscopic capsule to examine its retention in a simulated gut environment.109 They attached three “legs” (length = 1 cm) with microposts of an aspect ratio of 1
:
1 (diam. = 140 μm; center-to-center dist. = 175 μm) to the capsule (Fig. 7a(ii) and (iii)). An actuating system was incorporated to push the adhesive legs onto the intestinal walls and generate a high-friction interface. The researchers demonstrated that the capsule effectively resisted axial peristaltic shears when the actuating force exceeded 0.27 mN. Additionally, high-viscosity silicone oil-coated microposts increased friction by 400% compared to flat substrates. Buselli et al. developed a capsule with multiple nitinol legs inspired by insects and covered the edges with soft polymeric substrates featuring microposts of varying aspect ratios (Fig. 7b).110 Their work revealed that, aside from pillar diameter, the material of the microposts and the morphology of the intestinal tissue influenced the friction induced between the microposts and the intestine which is anatomy-dependent. Friction enhancement is valuable for slowing down the transit of endoscopic capsules, but it requires external actuators to ensure proper contact between the microposts and the tissue.
Building on this design concept, Naik et al. investigated the interlocking capabilities of micropillars with intestinal villi to withstand peristaltic shear.108 The study demonstrated that micropillar array patches with micropillar dimensions comparable to intestinal villi effectively penetrated the villi under jejunal contractile pressures and could resist peristaltic shears of up to 0.1 N cm−2. Additionally, computational models were employed to explore various factors influencing the interlocking ability of the micropillars with the villi. These factors included micropillar array pitch, the microposts' tip geometry, the arrangement of the micropillars, and the influence of the micropillars' moduli. The study further showed promising potential for villi-inspired devices to integrate mechanical interlocking and frictional enhancement simultaneously to increase retention time.
3. Current challenges and emerging technologies
3.1. Complexity of intestinal environment
The intestinal environment presents numerous challenges for the effective deployment of active devices. First, the constrictions of the intestine limit the overall device size. Moreover, the intestine is soft, mechanically compliant,111,112 and coated with the shear-thinning mucus layer that undergoes turnover at a rate comparable to the gut transit time in humans, which spans 24–48 h.25,113,114 In addition, the intestine undergoes fluctuations in fluid volumes and experiences dynamic peristaltic waves, marked by contractions and varying shear forces.41,111,115 These conditions, along with the continuous secretion and activity of digestive enzymes can challenge the mechanical integrity of the device.25 Furthermore, folds in the intestinal lumen can trap device components, leading to obstructions that require surgical removal. Lastly, there is a potential for device displacement due to the movement of the bolus within the intestine, further emphasizing the need for robust device design and secure fixation mechanisms.
Uniform testbeds must be established to evaluate the efficacy of current and emerging IRDs. A summary of retention performance, as previously tabulated by Naik et al.,108 has been extended in Table 1 to encompass additional insights derived from the mechanisms explored within this perspective. Moreover, we have explicitly indicated the model employed to evaluate retention performance. It is noteworthy that a variety of testing strategies have been applied to analyze the retention of intestinal retentive devices, emphasizing the necessity to narrow this range to establish a more standardized technique for evaluating the efficiency of IRDs. While some efforts have been made to evaluate mechano-adhesives through pull-off tests using ex vivo intestinal tissues, the testing of mucoadhesives often focuses solely on their ability to adhere to the mucosa.53 However, the effect of mucus overturn and enzymatic degradation of biodegradable mucoadhesives is often overlooked, with some tests only assessing their performance in simulated gastric fluids.77 Undoubtedly, initial testing using in vitro test beds is justified due to the resource-intensive nature and ethical considerations associated with animal subjects. Nevertheless, there is an urgent need to devise innovative testing strategies that can more accurately simulate the complex intestinal environment encountered by IRD.
Table 1 Summary of current technologies for intestinal retentive devices
Type |
Examples |
Materials/structure |
Testing strategy |
Intestinal adhesion or retention performance |
Chemoadhesives |
Mucoadhesive patches |
Janus omniphobic mucoadhesive devices54 |
Carbopol, microstructured cellulose acetate backing |
Ex vivo detachment tests and flow model using porcine intestinal mucosa |
Janus omniphobic devices improve adhesion to 10 minutes from 7 seconds vs. Carbopol adhesives |
Insulin PPS Patches53 |
Eudragit EPO, pectin, sodium carboxymethyl cellulose |
Ex vivo detachment tests with porcine intestinal mucosa |
0.98 mN mm−2 (shear adhesion) |
Self-uncoiling stents61 |
Polyester cylinders covered with mucoadhesive patches |
Benchtop experiment with simulated peristalsis using excised porcine SI and in vivo tests in porcine models |
Total retention time ∼36 hours in vivo |
Double-coated mucoadhesive film63 |
Film prepared with Sodium alginate and Lycoat RS 720 |
Mucoadhesion tensile test (animal model not specified) |
∼0.098 N cm−2 |
Self-folding device62 |
Folding bilayer: poly (methacrylic acid) poly(hydroxyethyl methacrylate) and mucoadhesive drug depot: PVA, Carbopol |
Ex vivo detachment tests with simulated fluid flow using porcine intestinal mucosa |
Avg. residence time of folded devices was estimated ∼1 hour 45 minutes |
Particulate systems |
Thiol-modified sodium alginate microspheres69 |
Thiol-modified sodium alginate microspheres |
Ex vivo adherence test using small intestine obtained from Kunming mice |
Adherence increases 100% via thiolation (absolute adhesion not tested) |
Thiolated chitosan particles70 |
Thiolated chitosan particles |
In vivo imaging in mice |
Maximum retention time 24 hours. Thiolated particles achieve 6× longer retention than non-thiolated particles |
Magneto-mucoadhesives |
Magnetic living hydrogels71 |
Polyvinyl alcohol hydrogel matrix doped with NdFeB ferromagnetic microparticles |
In vivo imaging in mice |
In vivo retention 7 days with wearable magnet intact, 6 hours without magnet |
Tubular mucoadhesive ring72 |
Magnetic silicone tube with glycerol-chitosan coating |
In vitro test using mucus plate with varying mucus concentrations |
∼45 mN at preload 20 mN, contact time 10 s, mucus concentration 5% |
Magnetically actuated capsule73 |
Neodymium magnet, mucoadhesive patches: catechol-conjugated chitosan |
Ex vivo tests performed to evaluate targeted delivery of mucoadhesive patches |
— |
Charge-coupled polymeric microparticles and micromagnets75 |
PLGA-based microparticles, Superparamagnetic iron oxide microparticles |
In vitro flow study (rate 0.8 mL min−1) and in vivo retention in mice |
Retention time with magnet ∼36 hours |
Polymeric microparticles74 |
Core–shell chitosan–alginate Fe3O4 incorporated magnetic beads |
Ex vivo tests on Wistar rats |
Tested for 2 hours |
Gels and in situ gelling polymers |
Synthetic epithelium lining77 |
Tissue surface initiated polydopamine coating |
Qualitative analysis of adhesion by scrapping lining on polycarbonate |
Retention time limited to mucus turnover |
Therapeutic luminal coating78 |
Sucrose octasulfate aluminium complex |
In vitro test using mucin-coated membranes and in vivo tests in rats |
Retention time limited to mucus turnover |
Nanoparticle-assembled bioadhesive coacervate coating79 |
Catechol functionalized end groups with polyethylene glycol hydrophilic chains |
In vivo retention tests in rats |
Work of adhesion 7.07 μJ mm−2 |
Retention time ∼2 days |
Nano cellulosic gels80 |
Various nano cellulosic gels |
Ex vivo flow-through tests with porcine intestine |
Tested for maximum 10 minutes |
|
Mechanoadhesives |
Bio-inspired IRDs |
Tissue attachment mechanism87 |
Sucker teeth of leech and tapeworms |
In vitro adhesion tests porcine intestinal tissue In vivo tests with porcine models |
Max adhesion strength 8.09 N per device |
Retention time 6 days |
Barbed microneedles – tissue anchoring microneedles86 |
Spikes of proboscis of spiny-head worm acanthocephala (later incorporated in spiny microneedle anchoring drug deposit) |
Ex vivo adhesion tests with porcine intestinal tissue |
Max pull-out force 25 mN per microneedle |
Swellable microneedles – biphasic microneedle with swellable tips89 |
Swelling of proboscis of pomphorhynchus laevis |
Ex vivo adhesion tests with porcine intestinal tissue |
Mean adhesion strength 4.53 N cm−2 |
Theragrippers90 |
Inspired by Hookworms |
In vivo adhesion tests in Wistar rat colon and in vivo tests in porcine upper GI tract |
Retains in both sites for 24 hours, removed naturally by mucosal turnover |
Microcontainers |
High and low aspect ratio cylindrical, and triangular microcontainers101 |
SU-8 and polycaprolactone-based microcontainers |
Ex vivo intestinal perfusion model using porcine small intestine |
65–81% of microcontainers located at the start of the intestine under perfusion rate of 1.55 mL min−1 |
Cubic, cylindrical, triangular, and cylindrical with microposts102 |
SU-8 based microcontainers |
Closed-loop colon perfusion study in rats |
Cubic microcontainers displayed ∼3 times greater retention |
3D printed reservoirs: microcontainers with anchoring adjuncts104 |
DLP 3D printed microcontainers (HTM 140 M V2 3D printing resin) |
Ex vivo tensile detachment tests with porcine intestinal mucosa and flow retention tests at 4.1–81.9 mL min−1 perfusion rate |
Addition of anchors showed 2× increase in mucoadhesion when compared to nonstructured controls. |
Micropatterned devices |
Micro-patterned wet adhesives (aspect ratio ∼1 : 1)107 incorporated in tri-legged capsule anchoring system109 and active capsule endoscopy |
Silicone-based 1 : 1 microstructures |
Ex vivo lap shear tests with porcine intestinal mucosa |
0.0828 N cm−2 (preload 0.131 N, height 125 μm, diameter 140 μm, edge-to-edge spacing 105 μm, viscosity 10 000 cSt) |
Villi-inspired mechanical interlockers108 |
Silicone based ∼1 : 5 microstructures |
Mechanical simulations, in vitro analyses and ex vivo tests with porcine intestinal mucosa |
Simulations predict interlocking can resist shear of 0.1 N cm−2, ex vivo tests reveal ∼1.4 mN mm−2 |
Promising inspiration can be drawn from existing models developed for the digestive tract. One such model, devised by Wang et al., features a J-shaped stomach system and a cylindrical small intestine.116 It incorporates an electromechanical driving instrument comprised of eccentric wheels, rollers, stomach and pyloric extrusion plates, motors, belts, and pulley systems. This driving device simulates natural movement and peristaltic contractions in the stomach and duodenum. Deng et al. constructed a soft tubular model equipped with artificial villi, which simulates peristalsis via a homemade cross-shaped rotating device which periodically pushes the shaft onto the tube using a stepped motor to simulate contractions.117,118 This model proves valuable for testing the residence time of fluid particles in the intestine and can be adapted for assessing the residence time of devices. Wu et al. have authored an insightful article that consolidates various developed models for digestive tracts.119 Their work serves as a comprehensive resource, providing inspiration for the construction of large-scale three-dimensional intestinal models. By leveraging the knowledge and approaches derived from these existing models, researchers can construct sophisticated 3D intestinal models that faithfully replicate the intricate dynamics of the intestine. These models facilitate more realistic testing of IRDs, taking into account crucial factors such as peristalsis, fluid volumes, luminal restrictions, and anatomical variations within the intestine.
Computational modeling of intestinal fluid flow volumes can permit the evaluation of device retention mechanisms in the gut under varying volume conditions.120,121 Additionally, finite element analysis has been effectively employed to assess the penetration capacity of mechano-adhesive gastroretentive devices, such as theragrippers90 and kirigami-inspired stents,122 designed for targeted local delivery of therapeutics. These computational techniques allow for the incorporation of diverse morphological conditions, considering factors like patients' age and the specific section within the small intestine.
Gut-on-a-chip systems are another platform that can accelerate device testing.123 These systems can simulate in vivo fluidic flow, peristalsis-like motions, host-microbe crosstalk, and multi-cell type interactions, making them highly valuable for personalized medicine applications. Noteworthy contributions to this area of research can be found in the works of Xiang et al.124 and Thomas et al.,125 who have provided detailed discussions on gut-on-a-chip in vitro models. Additionally, several in vitro models have been specifically designed to replicate the presence of mucus in the gut, offering accurate and controlled testing environments for mucoadhesive devices.126–128 By incorporating computational analysis, gut-on-a-chip systems, and specialized in vitro models, researchers can advance the field of IRDs by improving testing methodologies, optimizing device designs, and ultimately facilitating the development of more effective and patient-specific IRDs.
3.2. Path to clinical translation of intestinal retentive devices
Regulatory approval for commercial IRD products will be performed on a case-by-case basis with attention to their risk-reward profile. Prominent risks for these systems include intestinal perforation, occlusion, device migration, and fibrosis within the GI tract. Unsurprisingly, many of these complications have been documented in relation to GI stents, which clinicians use to alleviate obstructions, mitigate post-operative leaking, and facilitate gastrointestinal bypasses.129–131 So how can the potential benefit of technologies that use IRD be maximized while minimizing risk to the patient?
The risk of obstruction can be minimized by fabricating devices out of bioresorbable materials that reduce the likelihood of persistent obstruction or occlusion.132–135 As demonstrated by Bellinger et al., in cases where fully bioresorbable structures are not an option, strategic structural joints may be designed for degradation to compromise structural integrity and enable device motility.22 Special attention should be given to the anatomical limitations of the pylorus, ileocecal valve, cecum, and anus for safe passage of IRDs descending from the upper bowel.136 Compared to surgical implants, cytocompatibility of IRD materials poses less of a challenge due to the protective barrier of the intestinal lumen and toughened endothelial cells, however many of the same guidelines apply.137–139 Avoiding a microneedle penetration depth greater than 3 mm in the small intestine and greater than 1 mm in the large intestine can avoid harmful perforation of the bowel wall.140–143 These exact guidelines can vary depending on tissue condition, IRD location, and needle characteristics.144
The use of soft and compliant materials reduces the likelihood of perforation and bioresorbable elastomers such as poly(glycerol-co-sebacate) (PGS), poly(1,3-diamino-2-propanol-co-polyol sebacate) (APS), bis-urea-modified polycarbonate (PC-BU), among others can be better leveraged for IRD design.145–148 Crosslinked hydrogel systems are another favorable candidate with tunable mechanical properties, demonstrated extensively in tissue engineering and drug delivery research. Alginate, collagen, polysaccharide-based structures have been shown to survive in vivo environments for up to 7 days but still fall well short of the life spans of synthetic polymers such as polyglycolic acid (PGA), (PLGA), polylactic acid (PLA) and polycaprolactone (PCL) that have been used extensively as structural materials in other contexts.
What happens when an IRD needs to be extracted? Even fully bioresorbable structures may require early retrieval due to an adverse patient reaction or delayed complication. In many cases, manual retrieval will be performed endoscopically using biopsy forceps or specialized grippers. Devices lodged into the submucosa can be lifted from the membrane using a fluidized submucosal injection, as is used for the removal of polyps.149 In the case of microscale devices, researchers may consider implementing pre-designed points of structural failure to ensure IRD fragments are passed by the patient without irritation. Similarly, structural separation of a therapeutic payload from the anchoring body may be a way to terminate further drug delivery more easily in the case of an emergency. Small devices can leverage fluorescent or radiopaque markers to assist in device location following migration or tissue overgrowth. Mucoadhesive device designers should consider reversible chemical adhesion mechanisms that can be triggered using benign oral agents.150 In extreme cases, clinicians may resort to endoscopic laser therapy to ablate an anchored IRD out of position, trigger a thermally-reversible adhesion agent151,152 or use specialized gripping tools such as the Roth net or Dormia basket.
4. Conclusions and future outlook
IRDs hold significant promise in enabling a class of low-profile medical devices with wide-ranging applications such as long-term drug delivery platforms, continuous health monitoring, and non-invasive neuromodulation. A major challenge is designing devices that can achieve programmable non-obstructive device retention within the intestinal tract. This article presents the current state-of-the-art, opportunities, and challenges pertaining to the design of intestinal retentive medical systems.
Historically, most IRDs have been designed with drug delivery applications that allow for small-area interfaces such as particulates, patches, and capsules. As the application space of ingestible and intestinal medical devices continues to expand beyond drug delivery, there will be a need to construct strategies to design large-area interfaces as IRDs. Moving forward, there is an opportunity to leverage advances in shape memory materials,153,154 rapidly expanding stimuli-responsive materials,21,155 origami and kirigami-based expanding structures, and in situ self-assembling systems to accelerate the progress in functional and large-area IRDs. There is also an opportunity to utilize combinatorial approaches that optimize synergy between mechanical and chemical-based adhesives. While these innovations present a future-forward perspective, the fundamental challenge remains in ensuring effective and lasting adhesion within the intestinal environment.
From chemical to mechanical approaches for adhesion, it is clear that there are several distinct pathways to achieving intestinal retention. Each device application, depending on the size of the deployed structure and desired retention time, will require application-specific strategies to achieve the desired outcome. Intestinal retentive devices could leverage advances in bioelectronics, neuroengineering, soft robotics, and materials chemistry to design unique next-generation ingestible devices that can diagnose and treat a variety of diseases within the gastrointestinal tract.30,156–159
Additionally, considering patient risk-benefit and regulatory pathways during the early stages of development can accelerate the translation of intestinal retentive devices from lab to clinic. This foresight in development, combined with technological advancements, positions intestinal retentive devices as a cornerstone in the future of precision medicine and healthcare innovation.
Conflicts of interest
There are no conflicts to declare.
Acknowledgements
The authors acknowledge funding for this article from the National Institute of Biomedical Imaging, and Bioengineering (Grant no. R21EB026073).
References
- R. Sender, S. Fuchs and R. Milo, PLoS Biol., 2016, 14, e1002533 CrossRef
.
- M. Million and M. Larauche, Neurogastroenterol. Motil., 2016, 28, 1283–1289 CrossRef CAS PubMed
.
-
D. M. Denbow, in Sturkie's Avian Physiology, ed. C. G. Scanes, Academic Press, San Diego, 6th Edn, 2015, pp. 337–366 Search PubMed
.
- M. Augustyn, I. Grys and M. Kukla, Clin. Exp. Hepatol., 2019, 5, 1–10 CrossRef PubMed
.
- A. B. R. Thomson, Best Pract. Res., Clin. Gastroenterol., 2009, 23, 861–874 CrossRef PubMed
.
- J. Zhan, WJG, 2004, 10, 2585 CrossRef
.
- R. S. Choung and G. R. Locke, Clin. Gastroenterol., 2011, 40, 1–10 CrossRef PubMed
.
- C. Catassi, S. Gatti and E. Lionetti, Digital Discovery, 2015, 33, 141–146 Search PubMed
.
- J. H. Kurata and B. M. Haile, Clin. Gastroenterol., 1984, 13, 289–307 CrossRef CAS
.
- Y.-C. Chen, H.-O. Ho, T.-Y. Lee and M.-T. Sheu, Int. J. Pharm., 2013, 441, 162–169 CrossRef CAS PubMed
.
- Y. L. Kong, X. Zou, C. A. McCandler, A. R. Kirtane, S. Ning, J. Zhou, A. Abid, M. Jafari, J. Rogner, D. Minahan, J. E. Collins, S. McDonnell, C. Cleveland, T. Bensel, S. Tamang, G. Arrick, A. Gimbel, T. Hua, U. Ghosh, V. Soares, N. Wang, A. Wahane, A. Hayward, S. Zhang, B. R. Smith, R. Langer and G. Traverso, Adv. Mater. Technol., 2019, 4, 1800490 CrossRef
.
- H. Shirin, V. Richter, S. Matalon, D. Abramowich, A. Maliar, E. Shachar, S. F. Moss and E. Broide, Obes. Sci. Pract., 2019, 5, 376–382 CrossRef CAS PubMed
.
- C. I. Coleman, B. Limone, D. M. Sobieraj, S. Lee, M. S. Roberts, R. Kaur and T. Alam, JMCP, 2012, 18, 527–539 CrossRef
.
- C. M. Hughes, Drugs Aging, 2004, 21, 793–811 CrossRef
.
- F. Kleinsinger, Perm. j., 2018, 22, 18–033 Search PubMed
.
- L. Osterberg and T. Blaschke, N. Engl. J. Med., 2005, 353, 487–497 CrossRef CAS PubMed
.
- R. McCarthy, Bus Health, 1998, 16, 27–28 CAS
.
- J. S. Berg, J. Dischler, D. J. Wagner, J. J. Raia and N. Palmer-Shevlin, Ann. Pharmacother., 1993, 27, S1–S24 CAS
.
- G. Levy, M. K. Zamacona and W. J. Jusko, Clin. Pharmacol. Ther., 2000, 68, 586–591 CrossRef CAS
.
- P. J. McDonnell and M. R. Jacobs, Ann. Pharmacother., 2002, 36, 1331–1336 CrossRef
.
- X. Liu, C. Steiger, S. Lin, G. A. Parada, J. Liu, H. F. Chan, H. Yuk, N. V. Phan, J. Collins, S. Tamang, G. Traverso and X. Zhao, Nat. Commun., 2019, 10, 493 CrossRef CAS
.
- A. M. Bellinger, M. Jafari, T. M. Grant, S. Zhang, H. C. Slater, E. A. Wenger, S. Mo, Y.-A. L. Lee, H. Mazdiyasni, L. Kogan, R. Barman, C. Cleveland, L. Booth, T. Bensel, D. Minahan, H. M. Hurowitz, T. Tai, J. Daily, B. Nikolic, L. Wood, P. A. Eckhoff, R. Langer and G. Traverso, Sci. Transl. Med., 2016, 8, 365ra157 Search PubMed
.
- A. R. Kirtane, O. Abouzid, D. Minahan, T. Bensel, A. L. Hill, C. Selinger, A. Bershteyn, M. Craig, S. S. Mo, H. Mazdiyasni, C. Cleveland, J. Rogner, Y.-A. L. Lee, L. Booth, F. Javid, S. J. Wu, T. Grant, A. M. Bellinger, B. Nikolic, A. Hayward, L. Wood, P. A. Eckhoff, M. A. Nowak, R. Langer and G. Traverso, Nat. Commun., 2018, 9, 2 CrossRef
.
- A. R. Kirtane, T. Hua, A. Hayward, A. Bajpayee, A. Wahane, A. Lopes, T. Bensel, L. Ma, F. Z. Stanczyk, S. Brooks, D. Gwynne, J. Wainer, J. Collins, S. M. Tamang, R. Langer and G. Traverso, Sci. Transl. Med., 2019, 11, eaay2602 CrossRef
.
- T. D. Brown, K. A. Whitehead and S. Mitragotri, Nat. Rev. Mater., 2020, 5, 127–148 CrossRef
.
-
J. T. Collins, A. Nguyen and M. Badireddy, StatPearls, StatPearls Publishing, Treasure Island (FL), 2020 Search PubMed
.
-
P. Kahai, P. Mandiga, C. J. Wehrle and S. Lobo, StatPearls, StatPearls Publishing, Treasure Island (FL), 2022 Search PubMed
.
- J. Bernier-Latmani and T. V. Petrova, Nat. Rev. Gastroenterol. Hepatol., 2017, 14, 510–526 CrossRef CAS PubMed
.
- B. G. Nezami and S. Srinivasan, Curr. Gastroenterol. Rep., 2010, 12, 358–365 CrossRef PubMed
.
- K. Nan, V. R. Feig, B. Ying, J. G. Howarth, Z. Kang, Y. Yang and G. Traverso, Nat. Rev. Mater., 2022, 7, 908–925 CrossRef PubMed
.
- A. Khademhosseini, C. Bettinger, J. M. Karp, J. Yeh, Y. Ling, J. Borenstein, J. Fukuda and R. Langer, J. Biomater. Sci., Polym. Ed., 2006, 17, 1221–1240 CrossRef CAS PubMed
.
- N. Van Helleputte, A. J. G. Even, F. Leonardi, S. Stanzione, M. Song, C. Garripoli, W. Sijbers, Y.-H. Liu and C. Van Hoof, J. Microelectromech. Syst., 2020, 29, 645–652 CAS
.
- P.-M. Wang, G. Dubrovsky, J. C. Y. Dunn, Y.-K. Lo and W. Liu, Micromachines, 2019, 10, 525 CrossRef PubMed
.
- D. Rodrigues, A. I. Barbosa, R. Rebelo, I. K. Kwon, R. L. Reis and V. M. Correlo, Biosensors, 2020, 10, 79 CrossRef PubMed
.
- J. Li, J. Y. Liang, S. J. Laken, R. Langer and G. Traverso, Trends Chem., 2020, 2, 319–340 CrossRef CAS
.
- M. Yu, Gastroenterology Nursing, 2002, 25, 24–27 CrossRef PubMed
.
- C. Ell, S. Remke, A. May, L. Helou, R. Henrich and G. Mayer, Endoscopy, 2002, 34, 685–689 CrossRef CAS PubMed
.
- G. Ciuti, A. Menciassi and P. Dario, IEEE Rev. Biomed. Eng., 2011, 4, 59–72 Search PubMed
.
- N. C. Knox, J. D. Forbes, G. Van Domselaar and C. N. Bernstein, Curr. Treatm. Opt. Gastro., 2019, 17, 115–126 CrossRef
.
- R. H. Howland, Curr. Behav. Neurosci. Rep., 2014, 1, 64–73 CrossRef PubMed
.
-
R. Kant Avvari, in Digestive System - Recent Advances, ed. X. Qi and S. Koruth, IntechOpen, 2020 Search PubMed
.
- C. Schiller, C.-P. Frohlich, T. Giessmann, W. Siegmund, H. Monnikes, N. Hosten and W. Weitschies, Aliment. Pharmacol. Ther., 2005, 22, 971–979 CrossRef CAS PubMed
.
- M. W. Klinge, N. Sutter, E. B. Mark, A.-M. Haase, P. Borghammer, V. Schlageter, S. Lund, J. Fleischer, K. Knudsen, A. M. Drewes and K. Krogh, J. Neurogastroenterol. Motil., 2021, 27, 390–399 CrossRef PubMed
.
- G. S. Asane, S. A. Nirmal, K. B. Rasal, A. A. Naik, M. S. Mahadik and Y. M. Rao, Drug Dev. Ind. Pharm., 2008, 34, 1246–1266 CrossRef CAS
.
- E. Taipaleenmäki and B. Städler, Macromol. Biosci., 2020, 20, 1900342 CrossRef PubMed
.
- C. Atuma, V. Strugala, A. Allen and L. Holm, Am. J. Physiol.: Gastrointest. Liver Physiol., 2001, 280, G922–G929 CrossRef CAS PubMed
.
- L. M. Ensign, R. Cone and J. Hanes, Adv. Drug Delivery Rev., 2012, 64, 557–570 CrossRef CAS PubMed
.
- X. Murgia, B. Loretz, O. Hartwig, M. Hittinger and C.-M. Lehr, Adv. Drug Delivery Rev., 2018, 124, 82–97 CrossRef CAS PubMed
.
-
J. Hombach and A. Bernkop-Schnürch, in Drug Delivery, ed. M. Schäfer-Korting, Springer Berlin Heidelberg, Berlin, Heidelberg, 2010, vol. 197, pp. 251–266 Search PubMed
.
- G. P. Andrews, T. P. Laverty and D. S. Jones, Eur. J. Pharm. Biopharm., 2009, 71, 505–518 CrossRef CAS PubMed
.
- S. Eiamtrakarn, Y. Itoh, J. Kishimoto, Y. Yoshikawa, N. Shibata, M. Murakami and K. Takada, Biomaterials, 2002, 23, 145–152 CrossRef CAS PubMed
.
- T. M. Ways, W. Lau and V. Khutoryanskiy, Polymers, 2018, 10, 267 CrossRef
.
- A. Banerjee, J. Lee and S. Mitragotri, Bioeng. Transl. Med., 2016, 1, 338–346 CrossRef CAS PubMed
.
- Y.-A. L. Lee, S. Zhang, J. Lin, R. Langer and G. Traverso, Adv. Healthcare Mater., 2016, 5, 1141–1146 CrossRef CAS PubMed
.
- N. A. Peppas, J. B. Thomas and J. McGinty, J. Biomater. Sci., Polym. Ed., 2009, 20, 1–20 CrossRef CAS PubMed
.
- G. D. Bixler and B. Bhushan, Philos. Trans. R. Soc., A, 2012, 370, 2381–2417 CrossRef CAS
.
- B. Mizrahi, X. Khoo, H. H. Chiang, K. J. Sher, R. G. Feldman, J.-J. Lee, S. Irusta and D. S. Kohane, Langmuir, 2013, 29, 10087–10094 CrossRef CAS
.
- R. G. Nuzzo, Nat. Mater., 2003, 2, 207–208 CrossRef CAS PubMed
.
- K. Kirsch, U. Hanke and W. Weitschies, Eur. J. Pharm. Biopharm., 2017, 114, 135–144 CrossRef PubMed
.
- W. G. Sanad and S. N. Abd-Alhammid, Eur. J. Mol. Clin. Med., 2020, 7(2), 23–30 Search PubMed
.
-
S. Sarker, R. Jones, G. Chow and B. Terry, in 2021 Design of Medical Devices Conference, American Society of Mechanical Engineers, Minneapolis, MN, USA, 2021, p. V001T12A010 Search PubMed
.
- H. He, J. Guan and J. L. Lee, J. Controlled Release, 2006, 110, 339–346 CrossRef CAS PubMed
.
- E. W. Tan, K. Y. Tan, L. V. Phang, P. V. Kumar and L. L. A. In, PLoS One, 2019, 14, e0219912 CrossRef CAS PubMed
.
- G. Ponchel, M.-J. Montisci, A. Dembri, C. Durrer and D. Duchêne, Eur. J. Pharm. Biopharm., 1997, 44, 25–31 CrossRef CAS
.
- J. Ali, S. Baboota, R. Khar, S. Md, J. Sahni, G. Singh and A. Ahuja, Syst. Rev. Pharm., 2012, 3, 4 CrossRef
.
- D. Teutonico, S. Montanari and G. Ponchel, Int. J. Pharm., 2011, 413, 87–92 CrossRef CAS
.
- I. Bravo-Osuna, C. Vauthier, A. Farabollini, G. F. Palmieri and G. Ponchel, Biomaterials, 2007, 28, 2233–2243 CrossRef CAS PubMed
.
- V. M. Leitner, G. F. Walker and A. Bernkop-Schnürch, Eur. J. Pharm. Biopharm., 2003, 56, 207–214 CrossRef CAS PubMed
.
- X. Mao, X. Li, W. Zhang, L. Yuan, L. Deng, L. Ge, C. Mu and D. Li, ACS Appl. Bio Mater., 2019, 2, 5810–5818 CrossRef CAS PubMed
.
- T.-C. Chen, Y.-Y. Ho, R.-C. Tang, Y.-C. Ke, J.-N. Lin, I.-H. Yang and F.-H. Lin, Nutrients, 2021, 13, 4405 CrossRef CAS PubMed
.
- X. Liu, Y. Yang, M. E. Inda, S. Lin, J. Wu, Y. Kim, X. Chen, D. Ma, T. K. Lu and X. Zhao, Adv. Funct. Mater., 2021, 31, 2010918 CrossRef CAS PubMed
.
- C. Wang, A. Mzyk, R. Schirhagl, S. Misra and V. K. Venkiteswaran, Adv. Mater. Technol., 2023, 8, 2201813 CrossRef CAS
.
- J. Lee, D. Kim, S. Bang and S. Park, Adv. Intell. Syst., 2022, 4, 2100203 CrossRef
.
- A. Seth, D. Lafargue, C. Poirier, J.-M. Péan and C. Ménager, Eur. J. Pharm. Biopharm., 2014, 88, 374–381 CrossRef CAS PubMed
.
- B. A. Teply, R. Tong, S. Y. Jeong, G. Luther, I. Sherifi, C. H. Yim, A. Khademhosseini, O. C. Farokhzad, R. S. Langer and J. Cheng, Biomaterials, 2008, 29, 1216–1223 CrossRef CAS PubMed
.
- M. Tenci, S. Rossi, V. Giannino, B. Vigani, G. Sandri, M. C. Bonferoni, M. Daglia, L. M. Longo, C. Macelloni and F. Ferrari, Pharmaceutics, 2019, 11, 611 CrossRef CAS PubMed
.
- J. Li, T. Wang, A. R. Kirtane, Y. Shi, A. Jones, Z. Moussa, A. Lopes, J. Collins, S. M. Tamang, K. Hess, R. Shakur, P. Karandikar, J. S. Lee, H.-W. Huang, A. Hayward and G. Traverso, Sci. Transl. Med., 2020, 12, eabc0441 CrossRef CAS
.
- Y. Lee, T. E. Deelman, K. Chen, D. S. Y. Lin, A. Tavakkoli and J. M. Karp, Nat. Mater., 2018, 17, 834–842 CrossRef CAS PubMed
.
- P. Zhao, X. Xia, X. Xu, K. K. C. Leung, A. Rai, Y. Deng, B. Yang, H. Lai, X. Peng, P. Shi, H. Zhang, P. W. Y. Chiu and L. Bian, Nat. Commun., 2021, 12, 7162 CrossRef CAS PubMed
.
- Y.-J. Lin, J. A. Shatkin and F. Kong, Carbohydr. Polym., 2019, 210, 157–166 CrossRef CAS PubMed
.
- A. Mahdavi, L. Ferreira, C. Sundback, J. W. Nichol, E. P. Chan, D. J. D. Carter, C. J. Bettinger, S. Patanavanich, L. Chignozha, E. Ben-Joseph, A. Galakatos, H. Pryor, I. Pomerantseva, P. T. Masiakos, W. Faquin, A. Zumbuehl, S. Hong, J. Borenstein, J. Vacanti, R. Langer and J. M. Karp, Proc. Natl. Acad. Sci. U. S. A., 2008, 105, 2307–2312 CrossRef CAS PubMed
.
- S. Baik, J. Kim, H. J. Lee, T. H. Lee and C. Pang, Adv. Sci., 2018, 5, 1800100 CrossRef PubMed
.
- G. Meloni, O. Tricinci, A. Degl’Innocenti and B. Mazzolai, Sci. Rep., 2020, 10, 15480 CrossRef
.
- Y. Wang, X. Yang, Y. Chen, D. K. Wainwright, C. P. Kenaley, Z. Gong, Z. Liu, H. Liu, J. Guan, T. Wang, J. C. Weaver, R. J. Wood and L. Wen, Sci. Robot., 2017, 2, eaan8072 CrossRef
.
- W. Xie, V. Kothari and B. S. Terry, Biomed. Microdevices, 2015, 17, 68 CrossRef PubMed
.
-
S. Liu, S. Chu, G. E. Banis, L. A. Beardslee and R. Ghodssi, 2020 IEEE 33rd International Conference on Micro Electro Mechanical Systems (MEMS), IEEE, Vancouver, BC, Canada, 2020, pp. 885–888 Search PubMed
.
- S. Liu, S. Chu, L. A. Beardslee and R. Ghodssi, J. Microelectromech. Syst., 2020, 29, 706–712 CAS
.
- J. A. Levy, M. A. Straker, J. M. Stine, L. A. Beardslee, V. Borbash and R. Ghodssi, Adv. Mater. Technol., 2023, 8, 2201365 CrossRef CAS
.
- S. Y. Yang, E. D. O’Cearbhaill, G. C. Sisk, K. M. Park, W. K. Cho, M. Villiger, B. E. Bouma, B. Pomahac and J. M. Karp, Nat. Commun., 2013, 4, 1702 CrossRef PubMed
.
- A. Ghosh, L. Li, L. Xu, R. P. Dash, N. Gupta, J. Lam, Q. Jin, V. Akshintala, G. Pahapale, W. Liu, A. Sarkar, R. Rais, D. H. Gracias and F. M. Selaru, Sci. Adv., 2020, 6, eabb4133 CrossRef CAS PubMed
.
- A. Lamprecht, U. Schäfer and C. Lehr, Pharm. Res., 2001, 18, 788–793 CrossRef CAS
.
- J. A. Champion, Y. K. Katare and S. Mitragotri, J. Controlled Release, 2007, 121, 3–9 CrossRef CAS
.
- S. Xu, Z. Nie, M. Seo, P. Lewis, E. Kumacheva, H. A. Stone, P. Garstecki, D. B. Weibel, I. Gitlin and G. M. Whitesides, Angew. Chem., 2005, 117, 734–738 CrossRef
.
- D. Dendukuri, D. C. Pregibon, J. Collins, T. A. Hatton and P. S. Doyle, Nat. Mater., 2006, 5, 365–369 CrossRef CAS
.
- J. P. Rolland, B. W. Maynor, L. E. Euliss, A. E. Exner, G. M. Denison and J. M. DeSimone, J. Am. Chem. Soc., 2005, 127, 10096–10100 CrossRef CAS PubMed
.
- O. D. Velev, A. M. Lenhoff and E. W. Kaler, Science, 2000, 287, 2240–2243 CrossRef CAS PubMed
.
- P. Sozzani, S. Bracco, A. Comotti, R. Simonutti, P. Valsesia, Y. Sakamoto and O. Terasaki, Nat. Mater., 2006, 5, 545–551 CrossRef CAS PubMed
.
- J. A. Champion and S. Mitragotri, Proc. Natl. Acad. Sci. U. S. A., 2006, 103, 4930–4934 CrossRef CAS PubMed
.
- L. H. Nielsen, A. Melero, S. S. Keller, J. Jacobsen, T. Garrigues, T. Rades, A. Müllertz and A. Boisen, Int. J. Pharm., 2016, 504, 98–109 CrossRef CAS PubMed
.
- C. Mazzoni, F. Tentor, S. A. Strindberg, L. H. Nielsen, S. S. Keller, T. S. Alstrøm, C. Gundlach, A. Müllertz, P. Marizza and A. Boisen, J. Controlled Release, 2017, 268, 343–351 CrossRef CAS
.
- M. Dalskov Mosgaard, S. Strindberg, Z. Abid, R. Singh Petersen, L. Højlund Eklund Thamdrup, A. Joukainen Andersen, S. Sylvest Keller, A. Müllertz, L. Hagner Nielsen and A. Boisen, Int. J. Pharm., 2019, 570, 118658 CrossRef CAS
.
- J. F. Christfort, A. J. Guillot, A. Melero, L. H. E. Thamdrup, T. M. Garrigues, A. Boisen, K. Zór and L. H. Nielsen, Pharmaceutics, 2020, 12, 355 CrossRef CAS PubMed
.
- T. Chang, R. B. Kjeldsen, J. F. Christfort, E. M. Vila, T. S. Alstrøm, K. Zór, E. Hwu, L. H. Nielsen and A. Boisen, Adv. Healthcare Mater., 2023, 12, 2201897 CrossRef CAS PubMed
.
- L. Vaut, J. J. Juszczyk, K. Kamguyan, K. E. Jensen, G. Tosello and A. Boisen, ACS Biomater. Sci. Eng., 2020, 6, 2478–2486 CrossRef CAS
.
- M. Dalskov Mosgaard, S. Strindberg, Z. Abid, R. Singh Petersen, L. Højlund Eklund Thamdrup, A. Joukainen Andersen, S. Sylvest Keller, A. Müllertz, L. Hagner Nielsen and A. Boisen, Int. J. Pharm., 2019, 570, 118658 CrossRef CAS PubMed
.
- M. A. Rose, J. J. Bowen and S. A. Morin, Chem. Phys. Chem., 2019, 20, 909–925 CrossRef CAS PubMed
.
- J. Kwon, E. Cheung, S. Park and M. Sitti, Biomed. Mater., 2006, 1, 216–220 CrossRef CAS PubMed
.
- D. Naik, G. Balakrishnan, M. Rajagopalan, X. Huang, N. Trivedi, A. Bhat and C. J. Bettinger, Adv. Sci., 2023, 2301084 CrossRef CAS PubMed
.
- P. Glass, E. Cheung and M. Sitti, IEEE Trans. Biomed. Eng., 2008, 55, 2759–2767 Search PubMed
.
- E. Buselli, V. Pensabene, P. Castrataro, P. Valdastri, A. Menciassi and P. Dario, Meas. Sci. Technol., 2010, 21, 105802 CrossRef
.
- M. R. Carvalho, J. P. S. Ferreira, D. A. Oliveira, M. P. L. Parente and R. M. Natal Jorge, Numer. Methods Biomed. Eng., 2022, 38(5), e3588 CrossRef PubMed
.
- M. L. Grivel and Y. Ruckebusch, J. Physiol., 1972, 227, 611–625 CrossRef CAS PubMed
.
- D. Duchěne, F. Touchard and N. A. Peppas, Drug Dev. Ind. Pharm., 1988, 14, 283–318 CrossRef
.
- H. S. Ch’Ng, H. Park, P. Kelly and J. R. Robinson, J. Pharm. Sci., 1985, 74, 399–405 CrossRef
.
- B. S. Terry, A. B. Lyle, J. A. Schoen and M. E. Rentschler, J. Biomech. Eng., 2011, 133, 091010 CrossRef
.
- J. Wang, P. Wu, M. Liu, Z. Liao, Y. Wang, Z. Dong and X. D. Chen, Food Funct., 2019, 10, 2914–2925 RSC
.
- R. Deng, L. Pang, Y. Xu, L. Li, X. Wu and X. D. Chen, Int. J. Food Eng., 2014, 10, 645–655 CrossRef
.
- R. Deng, C. Selomulya, P. Wu, M. W. Woo, X. Wu and X. D. Chen, Chem. Eng. Res. Des., 2016, 112, 146–154 CrossRef CAS
.
- P. Wu and X. D. Chen, Curr. Opin. Food Sci., 2020, 35, 10–19 CrossRef
.
-
M. J. Ferrua and R. P. Singh, in New Advances in Gastrointestinal Motility Research, ed. L. K. Cheng, A. J. Pullan and G. Farrugia, Springer Netherlands, Dordrecht, 2013, vol. 10, pp. 243–266 Search PubMed
.
- N. Palmada, J. E. Cater, L. K. Cheng and V. Suresh, Fluids, 2022, 7, 40 CrossRef
.
- S. Babaee, Y. Shi, S. Abbasalizadeh, S. Tamang, K. Hess, J. E. Collins, K. Ishida, A. Lopes, M. Williams, M. Albaghdadi, A. M. Hayward and G. Traverso, Nat. Mater., 2021, 20, 1085–1092 CrossRef CAS PubMed
.
- X.-G. Li, M. Chen, S. Zhao and X. Wang, Stem Cell Rev. Rep., 2022, 18, 2137–2151 CrossRef PubMed
.
- Y. Xiang, H. Wen, Y. Yu, M. Li, X. Fu and S. Huang, J. Tissue Eng., 2020, 11, 204173142096531 CrossRef PubMed
.
- D. P. Thomas, J. Zhang, N.-T. Nguyen and H. T. Ta, Biosensors, 2023, 13, 136 CrossRef CAS PubMed
.
- V. C. Ude, D. M. Brown, V. Stone and H. J. Johnston, J. Nanobiotechnol., 2019, 17, 70 CrossRef
.
- S. Y. Lee, Y. Lee, N. Choi, H. N. Kim, B. Kim and J. H. Sung, BioChip J., 2023, 17, 230–243 CrossRef CAS
.
- J. McCright, A. Sinha and K. Maisel, Cel. Mol. Bioeng., 2022, 15, 479–491 CrossRef CAS PubMed
.
- S. Walayat, A. J. Johannes, M. Benson, E. Nelsen, A. Akhter, G. Kennedy, A. Soni, M. Reichelderfer, P. Pfau and D. Gopal, World J. Gastrointest. Endosc., 2023, 15, 309–318 CrossRef PubMed
.
-
E. A. Bonin, B. Verschoor, F. H. Silva, K. C. Vieira, S. K. Takata, E. A. Bonin, B. Verschoor, F. H. Silva, K. C. Vieira and S. K. Takata, Advanced Endoscopy, IntechOpen, 2019 Search PubMed.
- J. E. Lopera, M. A. de Gregorio, A. Laborda and R. Casta, Int. J. Gastrointest. Interv., 2016, 5, 138–148 CrossRef
.
- N. Gonzalo and C. Macaya, Vasc. Health Risk Manage., 2012, 8, 125–132 CrossRef PubMed
.
- B. D. Ulery, L. S. Nair and C. T. Laurencin, J. Polym. Sci., Part B: Polym. Phys., 2011, 49, 832–864 CrossRef CAS PubMed
.
- G. G. Stefanini, R. A. Byrne, P. W. Serruys, A. de Waha, B. Meier, S. Massberg, P. Jüni, A. Schömig, S. Windecker and A. Kastrati, Eur. Heart J., 2012, 33, 1214–1222 CrossRef CAS PubMed
.
- G. Balakrishnan, A. Bhat, D. Naik, J. S. Kim, S. Marukyan, L. Gido, M. Ritter, A. S. Khair and C. J. Bettinger, Adv. Mater., 2023, 35, 2211581 CrossRef CAS
.
- A. P. Madrona, J. A. F. Hernández, M. C. Prats, J. R. Riquelme and P. P. Paricio, Eur. J. Surg., 2000, 166, 307–309 CrossRef PubMed
.
- M. Jurak, A. E. Wiącek, A. Ładniak, K. Przykaza and K. Szafran, Adv. Colloid Interface Sci., 2021, 294, 102451 CrossRef CAS
.
-
G. Enders, Gut: The Inside Story of Our Body's Most Underrated Organ (Revised Edition), Greystone Books Ltd, 2018.
- L. W. Peterson and D. Artis, Nat. Rev. Immunol., 2014, 14, 141–153 CrossRef CAS PubMed
.
- Microneedles for drug delivery and monitoring - ScienceDirect, https://www.sciencedirect.com/science/article/abs/pii/B9780857096975500062, (accessed 15 June 2023).
- S. M. Bal, J. Caussin, S. Pavel and J. A. Bouwstra, Eur. J. Pharm. Sci., 2008, 35, 193–202 CrossRef CAS PubMed
.
- G. Cummins, Adv. Drug Delivery Rev., 2021, 177, 113931 CrossRef CAS PubMed
.
- L. T. Sørensen, U. Hemmingsen, F. Kallehave, P. Wille-Jørgensen, J. Kjærgaard, L. N. Møller and T. Jørgensen, Ann. Surg., 2005, 241, 654–658 CrossRef PubMed
.
- T. Fernandes, M. I. Oliveira, R. Castro, B. Araújo, B. Viamonte and R. Cunha, Insights Imaging, 2014, 5, 195–208 CrossRef PubMed
.
- C. J. Bettinger, Macromol. Biosci., 2011, 11, 467–482 CrossRef CAS PubMed
.
- T. R. Yeazel-Klein, A. G. Davis and M. L. Becker, Adv. Mater. Technol., 2023, 2201904 CrossRef
.
- J. Kluin, H. Talacua, A. I. P. M. Smits, M. Y. Emmert, M. C. P. Brugmans, E. S. Fioretta, P. E. Dijkman, S. H. M. Söntjens, R. Duijvelshoff, S. Dekker, M. W. J. T. Janssen-van den Broek, V. Lintas, A. Vink, S. P. Hoerstrup, H. M. Janssen, P. Y. W. Dankers, F. P. T. Baaijens and C. V. C. Bouten, Biomaterials, 2017, 125, 101–117 CrossRef CAS PubMed
.
- S. P. Nikam, Y.-H. Hsu, J. R. Marks, C. Mateas, N. C. Brigham, S. M. McDonald, D. S. Guggenheim, D. Ruppert, J. I. Everitt, H. Levinson and M. L. Becker, Biomaterials, 2023, 292, 121940 CrossRef CAS
.
- R. Castro, D. Libânio, I. Pita and M. Dinis-Ribeiro, WJG, 2019, 25, 777–788 CrossRef CAS
.
- X. Chen, H. Yuk, J. Wu, C. S. Nabzdyk and X. Zhao, Proc. Natl. Acad. Sci. U. S. A., 2020, 117, 15497–15503 CrossRef CAS
.
- S. Balamurugan, L. K. Ista, J. Yan, G. P. López, J. Fick, M. Himmelhaus and M. Grunze, J. Am. Chem. Soc., 2005, 127, 14548–14549 CrossRef CAS PubMed
.
- B. Li, J. J. Whalen, M. S. Humayun and M. E. Thompson, Adv. Funct. Mater., 2020, 30, 1907478 CrossRef CAS
.
- J. K. Park, K. Nan, H. Luan, N. Zheng, S. Zhao, H. Zhang, X. Cheng, H. Wang, K. Li, T. Xie, Y. Huang, Y. Zhang, S. Kim and J. A. Rogers, Adv. Mater., 2019, 31, 1905715 CrossRef CAS PubMed
.
- A. Melocchi, M. Uboldi, N. Inverardi, F. Briatico-Vangosa, F. Baldi, S. Pandini, G. Scalet, F. Auricchio, M. Cerea, A. Foppoli, A. Maroni, L. Zema and A. Gazzaniga, Int. J. Pharm., 2019, 571, 118700 CrossRef CAS PubMed
.
- R. Raman, T. Hua, D. Gwynne, J. Collins, S. Tamang, J. Zhou, T. Esfandiary, V. Soares, S. Pajovic, A. Hayward, R. Langer and G. Traverso, Sci. Adv., 2020, 6, eaay0065 CrossRef CAS PubMed
.
- G. Balakrishnan, J. Song, C. Mou and C. J. Bettinger, Adv. Mater., 2022, 34, 2106787 CrossRef CAS PubMed
.
-
J. Zhang, G. Balakrishnan, S. Srinidhi, A. Bhat, S. Kumar and C. Bettinger, Proceedings of the 20th ACM Conference on Embedded Networked Sensor Systems, Association for Computing Machinery, New York, NY, USA, 2023, pp. 75–90 Search PubMed
.
-
J. Hughes and D. Rus, 2020 3rd IEEE International Conference on Soft Robotics (RoboSoft), 2020, pp. 836–843 Search PubMed.
- J. A. Shulgach, D. W. Beam, A. C. Nanivadekar, D. M. Miller, S. Fulton, M. Sciullo, J. Ogren, L. Wong, B. L. McLaughlin, B. J. Yates, C. C. Horn and L. E. Fisher, Sci. Rep., 2021, 11, 12925 CrossRef CAS
.
|
This journal is © The Royal Society of Chemistry 2024 |
Click here to see how this site uses Cookies. View our privacy policy here.