A review of modified and hybrid anaerobic baffled reactors for municipal wastewater treatment with a focus on emerging contaminants
Received
8th November 2023
, Accepted 19th March 2024
First published on 5th April 2024
Abstract
This review discusses municipal wastewater treatment using anaerobic baffled reactors (ABRs) and modified ABRs. Conventional ABRs can convert organic carbon to renewable energy in the form of biogas. ABRs can achieve more than 90% COD removal at HRT as low as 8 hours at mesophilic temperatures, while COD removal in the range of 70–90% is typical at uncontrolled temperatures. However, effluents from ABRs do not meet discharge criteria and must be polished. Several techniques have been applied to improve the effluent quality including: pre-screening of raw wastewater using a mesh or sedimentation tank, inoculation with acclimatized sludge, effluent recirculation, electrocoagulation, microbial electrodes for improved VFA degradation, COD degradation and methane production, packing materials, carriers or meshes in individual compartments, polymeric membranes in the final compartment or external to the ABR, constructed wetlands and aerobic bioreactors. Recently, much research has focused on concurrent carbon and nitrogen removal in modified ABRs using novel strategies including microaeration, membrane aerated biofilms, an ABR followed by an aerobic membrane bioreactor with sludge recycling, anammox bacteria and nitrite/nitrate-dependent anaerobic methane oxidation bacteria. For P removal, promising chemical techniques include electrocoagulation and biological P removal includes denitrifying phosphate accumulating microorganisms. Some of these techniques applied in independent studies resulted in effluents containing <20 mg L−1 BOD, <1 mg L−1 TN and 0.2 mg L−1 TP, indicating the feasibility of mainstream anaerobic treatment of municipal wastewater, but pilot scale studies on biogas production and C, N and P removal are still lacking. Furthermore, ABRs have also been found to degrade concurrently emerging contaminants in municipal wastewater such as perchlorate, nitrophenols, and antibiotics with no effect on COD removal at typical concentrations found in municipal wastewater, but for some complex organics, an aerobic step is required for the complete oxidation.
Water impact
In this review paper, we focus on the most recent advances in municipal wastewater treatment using anaerobic baffled reactors. We reveal the most advanced modifications made to ABRs to achieve high organic removal while producing energy in the form of biogas. We also explain how nitrogen and phosphorus can also be removed in this type of reactor as well as emerging contaminants.
|
1 Introduction
Municipal wastewater is the general term associated with wastewater released from urban areas and includes not only human waste but also stormwater and industrial wastewater. It generally contains low organic strength, high particulate organic matter1 and a high percentage of suspended solids (SS).2,3 Due to the drastic urbanisation, large amounts of municipal wastewater are produced, which is harmful to human health and the environment if left untreated.4 Although it is a very diluted stream, it has a potential energy content of up to 7.6 kJ L−1 if converted to biogas using anaerobic digestion.5 The conventional activated sludge (AS) process for municipal wastewater treatment is controversial because some consider it as not sustainable as it consumes between 2.5 kJ g−1 COD and 7.2 kJ g−1 COD (chemical oxygen demand).6,7 It has many drawbacks such as high energy consumption, high sludge production, and large amounts of greenhouse gas production.8 Approximately 1 to 3% of the electricity is spent on wastewater treatment in European countries and more than 4% of the energy is used in drinking water production, delivery and wastewater treatment combined in the US.9 Due to the increase in electricity prices, it becomes urgent to rethink the way we treat municipal wastewater, reduce energy consumption and greenhouse gas emissions and create renewable energy simultaneously. Anaerobic reactors are generally applied in remediating high-strength wastewater or municipal sludge10 but it can also be used to treat low strength municipal wastewater (COD ranging from 400–700 mg L−1).11 Compared with the aerobic process, the anaerobic process is more economic in terms of construction and operation, aeration costs, and sludge production and methane gas is produced as an alternative energy resource.12 However, the low growth rate of anaerobic bacteria in anaerobic treatment plants requires long hydraulic retention times (HRTs) and large volumes.12 The problem of long HRT is solved when the separation of HRT and solids retention time (SRT) is developed.13 The anaerobic baffled reactor (ABR) is a multi-stage bioreactor founded by McCarty and co-workers and has a high biomass retention time, and the separation of hydrolytic and organic acid-producing bacteria with methanogens is achieved with the compartments and a series of vertical baffles.14 ABRs have a great ability to remove organic pollutants and produce less sludge because each chamber's up-flow region can retain the biomass.15 Furthermore, these systems are resistant to high pH levels or saturated toxic chemicals such as phenols, ammonia, and dyes.16 However, ABRs have drawbacks such as difficulties in regulating and distributing the water flow across the entire reactor volume and the occurrence of dead zones. They are cost friendly, sustainable and appropriate for wastewater remediation and applied in developing countries such as India, Columbia, Brazil, etc.,17 but despite their implementation for high-strength and industrial wastewater, there is still a lack of information about their application for low-strength and municipal wastewater. Recent research has also shown that nitrogen and P removal is possible in ABRs using different strategies. For these reasons, this review will focus on the application of ABRs and modified ABRs for municipal wastewater treatment including carbon, nitrogen and phosphorus removal. In addition, the ABR performance when treating emerging pollutants in municipal wastewater is also reviewed.
2 Basic biology in ABRs
Aerobic and anaerobic treatments are two common biological treatment methods, but anaerobic treatment surpasses aerobic treatment in various aspects, including lower capital and operational costs, reduced energy consumption, straightforward design and operation, and efficient conversion of organic matter into biogas.18,19 Anaerobic digestion involves four major processes: hydrolysis, acidogenesis, acetogenesis, and methanogenesis.20 In the hydrolysis stage, complex organic feedstock is broken down into simpler components like glucose and amino acids. Acidogenesis follows, where these components are converted into volatile fatty acids (VFAs) and alcohols. The subsequent acetogenesis stage transforms propionic and butyric acids into acetic acid and CO2/H2. Finally, methanogenesis results in the conversion of these products into CH4 (methane) and CO2 by methanogens.21,22
3 Discharge limits for municipal wastewater
The purpose of discharge limit values (DLVs) is to set limits to the nutrient loads into the water body in different countries. DLVs are normally evaluated from the point sources where the effluents are discharged from wastewater treatment plants (WWTPs). Table 1 shows the standard parameters for wastewater discharge in various regions around the world and parameters vary widely depending on the receiving water, flowrate, type of receiving water (estuaries, rivers, lake…) and local legislation. Generally, COD discharge levels vary between 45 and 125 mg L−1, BOD between 15 and 45 mg L−1, total suspended solids (TSS) between 35 and 60 mg L−1, TN between 2 and 15 mg L−1 and TP between 0.5 and 2 mg L−1. There is no uniformity in terms of parameters as some countries prefer to use COD instead of BOD, and other countries use NH4-N instead of total nitrogen (TN), but there are limits imposed on N and/or P to prevent eutrophication and algal blooms in receiving waters. Effluent standards do not reflect the actual response of the water bodies and often are not justified enough from an ecological point of view.23 For comparison's sake, the last row of Table 1 refers to some of the recommended quality parameters for water reused in irrigation according to the Food and Agricultural Organisation (FAO). The guidelines are general in nature and should not be associated with any specific type of irrigation method, crop or soil.
Table 1 Treated wastewater quality parameters in various geographic regions (PE = population equivalent)
WWTP category |
COD, mg l−1 |
BOD5, mg l−1 |
TSS, mg L−1 |
TN, mg l−1 |
TP, mg l−1 |
Region |
Ref. |
<2000 PE |
125 |
25 |
— |
— |
— |
EU |
24
|
2000–10 000 PE |
125 |
25 |
60 |
— |
— |
EU |
10 000–100 000 PE |
125 |
25 |
35 |
15 (areas sensitive to eutrophication) |
2 (areas sensitive to eutrophication) |
EU |
<100 000 PE |
125 |
25 |
35 |
10 (areas sensitive to eutrophication) |
1 (areas sensitive to eutrophication) |
EU |
200–10 000 PE |
60 |
20 |
— |
2 (sum of NH3-N and NH4-N) |
0.8 PO43−-P |
Switzerland |
25
|
>10 000 PE |
45 |
15 |
— |
2 (sum of NH3-N and NH4-N) |
0.8 PO43−-P |
Effluent class A |
50 |
— |
— |
15 |
0.5 |
China |
26
|
— |
— |
30 |
— |
3–5 in areas sensitive to eutrophication |
0.1–1 in areas sensitive to eutrophication |
USA |
25
|
Maximum daily flow >50 m3 d−1 |
— |
45 |
— |
— |
1.0 |
Canada |
25
|
Effluent to be released into industrial fishing areas |
— |
3.0 (BOD20) |
— |
0.39 NH4+-N, mg l−1, 0.02 (NO2−-N) 9.1 (NO3−-N) |
2.0 PO43−-P, mg l−1 (0.2 in eutrophic waters, 0.15 in mesotrophic waters, 0.05 in oligotrophic waters) |
Russia |
25
|
Irrigation water |
90 |
30 |
45 |
15 (NO3−-N) and 5 (NH4+-N) |
— |
— |
27
|
4 Application of ABRs for municipal wastewater
4.1 Laboratory scale studies
Application of ABRs for low-strength municipal wastewater is heavily constrained by temperature and is usually implemented in tropical regions as the climate is suitable for microbial communities to thrive under anaerobic conditions.28,29 The average removal efficiencies performed by ABRs reported in the literature are 84 ± 10%, 84 ± 12%, 80 ± 12%, 37 ± 26%, and 33 ± 4%, for BOD, COD, TSS, TN, and P, respectively.30 Latin America is leading the way in treating full-scale dilute municipal wastewater and has proven their feasibility.28 However, application of anaerobic treatment in temperate areas has long been regarded as unfeasible29,31–33 because the low temperatures increase the difficulty in treating dilute wastewater,28,31,33,34 which means that traditional discharge limits cannot be met with conventional ABRs without temperature control. Low temperatures in ABRs also increase the solubility of methane in the effluent: 40 mg L−1 at 0 °C but only ∼20 mg L−1 at 25 °C.35
Table 2 shows recent studies related to synthetic, raw or screened municipal wastewater treatment using ABRs and modified ABRs with a focus on COD removal. In Renuka et al.'s study (2016),36 the ABR was combined with an anaerobic filter to form a 115 L panelled anaerobic baffle-cum-filter reactor (PABFR) to treat municipal wastewater at 25–35 °C. The COD, BOD and TSS removal was 90, 91 and 94%, respectively, at 8 hours HRT which resulted in a methane percentage in the range of 68 to 74% and a methane yield of 0.23 m3 kg−1 COD removed which is lower than the theoretical value of 0.35 m3 kg−1 COD37,38 because some of the methane gas was dissolved in the treated effluent. Faecal coliform removal decreased from 99.7% at 40 h HRT to 77.2% at 2 h HRT, and faecal coliform in the colloidal form (0.05 to 0.45 mm) was discharged with the final effluent.39 Post treatment was therefore required to meet the discharge guideline (<1000 faecal coliform colonies/100 mL) set by the FAO for irrigation purposes.40 In comparison with a conventional ABR, this system demonstrated a recovery performance that is twice as effective due to the presence of a filter, particularly following short-term transient hydraulic shocks, and its compartmentalized structure enhanced its recovery compared to other high-rate reactors after the long-term step hydraulic shock which normally happens in small-scale treatment facilities. A three-month starvation experiment was carried out and it was found that the ABR could treat wastewater from seasonally operated facilities in decentralized areas facing seasonal pollution overloads, particularly during the tourist season. In another paper, screened raw municipal wastewater was treated in a 37 L ABR modified with an electrocoagulation system (EABR).41 Electrocoagulation was reported to enhance the removal of contaminants.42 The removal of TSS, sCOD and tCOD was 93, 81 and 88%, respectively. However, further decreasing the HRT to 24 h affected the total COD removal efficiency from approximately 90 to 83%. Effluent recirculation did not improve the performance of the ABR. Using steel or aluminium electrodes at a current density of 0.1 mA cm−2, the EABR could decrease the concentrations of TSS, tCOD, BOD, sulfate and phosphate below the standard limits for discharge into local surface water bodies.41 In a 32 L modified ABR with 9 compartments treating municipal wastewater at 35 °C, about 2 to 3 months was required to acclimatize the sludge.37 At 6 hour HRT, the COD, BOD and TSS removal was reported to be 84%, 87% and 86%, respectively. It was found that hydrolysis, acidogenesis and acetogenesis occurred in the first 3 compartments while methanogenesis occurred in the other 6 compartments demonstrating the efficient spatial separation of anaerobic trophic groups. Biomass washout did not take place because of the baffles, the adequate number of the compartments and the development of granular sludge. The specific biogas yield and methane content were found to be 0.34 m3 CH4 kgCODr−1 and 67%, respectively. It was recommended that it can be used as an onsite compact wastewater treatment plant for individual houses or small communities in India.37
Table 2 Raw municipal wastewater treatment by ABRs and modified ABRs
Contaminant |
Raw WW composition |
ABR characteristics |
Process performance |
Ref. |
COD in mg L−1 |
(OLR in kg m−3 d−1) |
BOD (mg L−1) |
TSS (mg L−1) |
NH4+ (mg L−1) |
PO43− (mg L−1) |
Modification (number of compartments) |
Temperature (°C) |
Volume (L), HRT |
COD effluent (mg L−1) (removal%) |
BOD (mg L−1) |
TSS effluent (mg L−1) (% removal) |
Methane yield (m3 kg−1 COD destroyed) |
Methane % in the biogas |
Raw municipal wastewater |
760 |
1.52 |
320 |
510 |
— |
— |
ABR (4) |
12 to 23 |
1000, 12 days |
440 (42%) |
169 |
83 |
— |
20 |
43
|
Municipal wastewater |
650 |
1.95 |
275 |
23 |
— |
— |
ABR (5) + AF |
25–35 |
115, 8 hours |
68 (90%) |
24 (91%) |
94 (94%) |
0.22 |
68–74 |
36
|
Screened raw municipal wastewater |
564 |
0.282 |
352 |
267 ± 14 |
2.4 ± 0.3 |
23 ± 2 |
ABR (6) + EABR |
— |
37, 2 days |
70 (88%) |
31 |
93 |
— |
— |
41
|
Pre-settled municipal wastewater |
386 |
0.92 |
— |
23 |
22 |
— |
UASB + AF + UASB–AF (3) |
18–28 |
3.6, 10 h |
64 (83.4%) |
— |
22 |
0.09 m3 m−3 d−1 |
72.1 |
44
|
Municipal wastewater |
400 |
1.6 |
200–300 |
300–450 |
— |
5–6 |
ABR (9) |
35 |
32, 6 h |
44 (84%) |
30 |
40 |
0.5 m3 kg−1 COD |
— |
37
|
Municipal wastewater |
305 |
0.15 |
— |
80 |
38 |
7.25 |
CABR (6) |
28 |
17, 2 days |
17 (79%) |
— |
12 |
0.15 |
— |
16
|
Pilot scale municipal wastewater |
716 |
0.78 |
— |
480 |
24.9 |
4.9 |
ABR (8) |
20–25 |
3000, 22 h |
192 (73%) |
— |
225 |
— |
— |
15
|
Pilot scale municipal wastewater |
300,391 (winter) |
0.48, 0.63 |
162–193 |
130–180 |
38.4–43.9 |
— |
ABFR (4) + aerobic post-treatment |
4.5–23 °C |
750, 15 h (anaerobic), 4 h (aerobic) |
(81.5–83%) (winter) |
12–13.1 (winter) |
11–12.4 (winter) |
— |
— |
45
|
Septic tank wastewater |
774 |
0.19 |
— |
— |
53 |
— |
PABR |
16–32 |
240, 96 h |
86 |
— |
— |
— |
— |
46
|
University dormitory wastewater |
467 |
196.7 g COD m−2 d−1 |
311 |
466 |
20.1 |
13.4 |
ABR + VFCW + HFCW |
5–37.5 |
103 m3, 5.4 days (ABR), 2.5 days (VFCW) 4 days (HFCW) |
65.5 (89%) |
34.5 |
36.3 |
— |
— |
47
|
Decentralized wastewater |
187–380 |
0.76 |
|
|
30–60 |
3–7 |
MPABR (4) |
35 |
90, 12 h |
76.70% |
|
|
|
|
48
|
Synthetic wastewater |
1500–7500 |
2.77 g sCOD m2 d−1 |
— |
0 |
25–125 (TN) |
5–25 (TP) |
ABR (2) + aerobic baffled reactor (4) + carriers |
25 |
12 h (anaerobic), 18 h (aerobic) total: 30 h |
69 mg L−1 45% (anaerobic) 92% (aerobic) total: 95.4% |
|
1680 mg per day |
0.3 |
|
49
|
Raw wastewater from WWTP |
682 ± 191 |
— |
— |
57.2 ± 6.5 |
31.6 ± 3.5 |
12.3 ± 3.3 |
ABR (4) + MBR |
— |
105 (ABR) + 58 L (MBR) |
93.3% |
— |
17 mg L−1 (>99%) |
— |
— |
50
|
Carriers and packing materials made of plastic or organic materials can assist to provide ABRs with additional retention mechanisms for biomass which can increase the SRT and shorten acclimatization and start-up times. For instance, in a 17 L carrier anaerobic baffled reactor (CABR) treating municipal wastewater at 28 °C,16 the carrier was made up of bamboo that was able to retain the biomass and entrap SS from wastewater. Biofilm growth can provide great tolerance of microbial mass to the organic loading and hydraulic retention time changes. It required nearly 3 weeks for the start-up and the TCOD removal decreased from 79 to 69% when the HRT was reduced from 2 days to 0.75 days due to washed-out biomass, but the SCOD remained constant. The effluent SS concentration increased from 11.8 mg L−1 (HRT 2 days) to 14 mg L−1 (0.75 days) due to sludge washout at higher upflow velocities. 65% of the substrate was consumed for cell synthesis during the start-up phase while only 20% was taken during the steady state. Although it could remove pathogenic species, suitable post disinfection was still required to guarantee the effluent safety. The CABR was deemed suitable to treat domestic sewage in rural areas in China.16 In a 90 L modified packed anaerobic baffled reactor (MPABR) treating decentralized wastewater at 35 °C,48 polyvinylidene fluoride (PVDF) in the downstream part of the compartments improved biomass retention and extended the SRT. During the first 25 days (start-up phase), the COD removal was lower than 70%. Then, the COD removal was 70.7% and 76.6% when the HRT was 24 h and 12 h, respectively, and the COD removal decreased afterward when the HRT was 8 h (74.8%) and 5 h (68.3%). It was reported that most COD removal happened in the first 2 compartments with the sum of COD removal equal to 59.4%. VFAs indicated the extent of acidogenesis and methanogenesis during the anaerobic process. At 12 h HRT, the VFA concentration in the 4 compartments was 1.75, 1.12, 0.85 and 0.69 mmol L−1, respectively, indicating that most of the organic pollutants were degraded to VFAs by hydrolysis and acidogenesis.48 An integrated 2-phase fixed film baffled reactor was set up to treat synthetic wastewater at 25 °C. The anaerobic phase comprised 2 baffled compartments (2 L), followed by 4 aerobic baffled compartments (4 L). The anaerobic and aerobic compartments were filled with high-density polyethylene carriers with filling ratios of 90 and 75%, respectively. The carriers acted as a fixed-bed for the attached growth of microbial biomass. The first compartment of the aerobic system was not filled with carriers in order to remove the possible generated gases (e.g. H2S) by the anaerobic bacteria with pre-aeration to avoid the possible inhibition of aerobic bacteria, but this may have resulted in a loss of methane gas. The anaerobic part of the system achieved 45% COD removal at 12 h HRT and 4.6 days SRT, while the aerobic part achieved 92% COD removal at 18 h HRT and 19 days SRT. In total, 95.4% COD removal was achieved at 30 h HRT and 13.4 days SRT while producing 0.3 L CH4 per g sCODremoved. The short SRT in the ABR resulted in low COD removal and this required further sludge processing in anaerobic reactors. The study demonstrated the requirement to include aerobic post-treatment of the ABR effluent. The anaerobic part could operate at 4 h HRT but achieved only 33% COD removal. Since the excess sludge was regularly removed from both parts of the ABR to achieve the desired SRT, biochemical methane potential tests were carried out to determine the additional methane that could be produced from the sludge: 0.25 L CH4 gTS−1 and 0.19 L CH4 gTS−1 from the anaerobic and aerobic sludge, respectively. The SRT is one of the important factors which substantially affect the efficacy of organic matter oxidation and can be used as a good index to evaluate the condition and performance of the bioreactor, yet most studies on ABRs do not mention the SRT (Table 2). In this study, the values of SRT varied from 19.25 to 5.19 days by decreasing HRT and it was observed that removal efficiencies of the aerobic unit were reduced as SRTs were reduced. These SRTs may have been sufficient for synthetic wastewater made of glucose, but higher SRTs (min. 30–50 days) are recommended for real wastewater. Although some of the runs met the local discharge criteria, the authors recommended further polishing steps to accommodate for transient organic and hydraulic loads.51 The energy production was in the range of 2.8–12 kW h m−3, whereas the overall energy consumption of wastewater treatment was in the range of 0.32–0.79 kW h kg−1 sCODremoved, while the total energy production was 3.7–5.1 kW h kg−1 sCODremoved. Therefore, the designed bioreactor was energy positive with a net energy production of 3.39–4.5 kW h kg−1 sCODremoved. The total energy requirement for both wastewater treatment and bio-wasted sludge digestion was 7–15.5% of the total energy production. The contribution of anaerobic wastewater treatment and anaerobic digestion of bio-wasted sludge of the aerobic and anaerobic units for energy recovery as bio-methane was 53, 26, and 21%, respectively. An ABR coupled with an anaerobic filter (AF) was investigated by Khan et al. (2023)52 to study four different filtration media for domestic wastewater treatment: PVC pipe (25 mm), PVC pipe (20 mm), PVC pipe (15 mm), and Kaldnes K3. The existence of packing media allows the growth of attached biomass, but the principal role of the media is to retain suspended growth. Among all the filtration media tested, the highest performance efficiency of the system was found with the PVC pipe (20 mm), which showed COD, TP, and TKN removal of 79–81%, 32 (9 mg L−1 in effluent), and 63% (10–12 mg L−1 in effluent), respectively, at 12 h HRT.52 Although not achieving complete nutrient removal, the study showed the importance of packing materials to enhance the ABR performance. The coupled growth processes (suspended and attached) showed more nitrogen removal compared to suspended growth alone as the treatment performance was linked to the larger surface area in the attached growth process due to more bio-accumulation of nitrogen in attached biomass.53 The integrated system (ABR–AF) with a total HRT of 24 h produced water that met the local discharge standards with an effluent COD of 113 mg L−1. When a membrane filtration (MF) step was added, the combined system (ABR–AF–MF) achieved COD removal of 94.6% with an effluent COD concentration of approximately 28.1 mg L−1 at an HRT of 24 h with a membrane flux of 6 LMH.52
Aerobic reactors are commonly used to treat ABR effluents. A study was conducted on a modified 105 L ABR coupled with a 58 L aerobic membrane bioreactor (MBR) featuring submerged hollow fibers for the treatment of actual municipal wastewater containing 682 ± 191 mg L−1 COD. The investigation focused on evaluating the system's performance in terms of removing organic matter, suspended solids, turbidity, and nitrogen. The integrated modified ABR–MBR process resulted in the complete removal of total suspended solids (TSS). Furthermore, the recycling of mixed liquor from the MBR to the modified ABR resulted in some denitrification occurring in the first compartment of the ABR, resulting in 53 ± 6% removal of nitrogen by the integrated process.50 The ABR effluent contained 241.7 ± 71.2 mg COD L−1 and further removal was achieved by the subsequent MBR which resulted in an overall COD removal of 93.3 ± 3.8% (less than 40 mg L−1 in the permeate). The membrane flux was stable and above 20 L m−2 h−1.50 In a similar study, the average permeate flux of the membrane was kept at 30 L m−2 h−1.54 The ABR–MBR system could remove more than 90% COD, total nitrogen, and total phosphorus from building wastewater at a total operating HRT of only 3 h.54 The research suggests that the ABR–MBR system can be a promising system for water reuse and reclamation for high-rise buildings.
Despite successful studies at low HRT (3–8 h) and high COD, BOD and SS removal (∼90%), laboratory studies require between 3 weeks and 3 months of acclimatization and usually fail to meet discharge limits. On average, the BOD, COD and SS in the effluent of an ABR was 60 mg L−1, 200 mg L−1 and 90 mg L−1, respectively, which is too high to directly discharge it into the environment according to Table 1. However, this is usually sufficient to meet the criteria for irrigation water. Results also indicate that coupling the ABR with a membrane process would be a suitable option should more stringent standards be required. An aerobic MBR can also be used to treat the ABR effluent and operate at low mixed liquor total solids of 2–3 g L−1 to minimize membrane fouling. Air sparging can be used for nitrification and membrane scouring while achieving a membrane flux of 20–30 LMH.50 Even though the ABR (min. HRT 6–8 h) can compete with the activated sludge process (6–10 h) in terms of HRT, the ABR would need to be operated at a minimum HRT between 10 and 48 hours to provide a sufficient buffer in the case of toxic, organic and hydraulic shocks. The ABR also requires longer SRT (min. 30 days) due to the slow growth of methanogens. It is also worth noting that the waste activated sludge process also requires an anaerobic digester operating at 30 days HRT for excess sludge digestion and that would not be required for the mainstream anaerobic treatment of municipal wastewater. Additional work should therefore focus on coupling the ABR with additional techniques such as electrocoagulation, electrochemical oxidation, coarse filtration, plastic or natural carriers, polymeric microfiltration membranes and an aerobic polishing step to investigate performance, stability and effluent concentrations during transient hydraulic and organic loadings.30
4.2 Pilot-scale studies
Several pilot scale studies of ABRs were carried out to investigate the effect of temperature, HRT, OLR and post-treatment. A 1000 L unmodified 4-chambered ABR was operated at a pilot scale for 2 years to treat raw municipal wastewater at ambient water and air temperatures of 12–23 °C and −10 to 35 °C, respectively, and at an average OLR of 1.3 kg COD m−3 d−1.43 The COD removal was in the range of 41–64% at temperatures below 20 °C. This is consistent with Álvarez et al. and León et al. who stated that anaerobic treatment in laboratory-scale and pilot-scale experiments can be done at temperatures around 15 to 20 °C on dilute wastewater with the COD and BOD removal around 50 to 75%. BOD5 tests were carried out on ABR influent and effluent in compartment 4. The average influent BOD5 was 320 ± 80 mg L−1 and the removal was 47 ± 15%.33,55 Although BOD removal was low at low temperature, the removal was higher than the average 25–35% removal for conventional primary clarification56 showing that the ABR can act as an efficient clarification unit while producing energy. The average estimated daily BOD removal was 388 g BOD d−1 over the two years of study. No settled sludge was cleared from the ABR during the two years of operation, yet it achieved 83% TSS removal at 12 h HRT demonstrating that excess sludge withdrawal hardly occurred in the ABR treating municipal wastewater as the compartments retained the solids and sludge production by anaerobic microorganisms was low. This study demonstrated feasible treatment at low HRT, but failed to achieve high COD removal due to low temperature, which highlights the importance of temperature control in the ABR for municipal wastewater treatment. Better TSS removal could also be achieved using a sedimentation tank prior to the ABR, more compartments (8 instead of 4) and a sedimentation tank after the ABR. Another issue of conventional ABRs is that total nitrogen and total phosphorus removal is usually limited to ∼37 and ∼33%, respectively, due to bacterial uptake for cell synthesis.30 In a 3000 L ABR, domestic wastewater was treated at ambient temperature as on-site primary sanitation for low-income communities. It was reported that at 22 h HRT, COD removal of 58 to 72% was achieved and the removal of TSS was 53%. The effluent fulfilled the COD guidelines for irrigation, but it didn't meet the guidelines for discharge to surface water. The ABR was found to be hydraulically overloaded at 22 h HRT and corresponding flow rates. It was recommended that further investigation on different flow rates and suitable post-treatments should be carried out to eliminate pathogens.16
Some pilot scale ABRs investigated concurrent C and N removal. For instance, a 240 L packed anaerobic baffled reactor (PABR) was used to treat wastewater from a septic tank.46 At 32 °C and 96 h HRT, the highest COD, NH4+-N and TN removal was 86, 17 and 5.6%, respectively. The COD removal was only 49% at 36 h HRT, indicating that prolonged HRT was advantageous in COD removal. The HRT did not affect significantly the TN and NH4+-N removal. In winter (16 °C), the highest COD removal was 52% at 96 h HRT. 72 h was chosen as the optimum HRT in this study. Due to the packing material, the PABR was found to be efficient in removing suspended solids and colloidal COD, leaving only dissolved COD in the effluent. It was also discovered that the first and second compartments contained mainly acid-resistant hydrogen trophic methanogens, while more acetic acid trophic methanogens were detected in the following compartments.46 Volatile fatty acids (VFAs) are the major electron donor for methanogenesis to produce methane gas,57 so monitoring of VFAs in the ABR is important. Short chain fatty acid-oxidation syntrophs (SFAS) could enhance the degradation of propionic and butyric acids to acetic acid and exoelectrogens can further utilise acetic acid.58 The growth of SFAS and exoelectrogens was promoted using electrodes in a 360 L pilot-scale ABR with 5 compartments at 35 °C to treat the sludge filtrate for methane production (Wang et al., 2020).59 The electrodes were placed in the upstream region of the middle of second, third and fourth compartments to investigate acid production and COD removal. The total COD removal efficiency was 74.1% without current and 86.6% with current flowing to the electrodes, showing that the improvement in COD removal was attributed to the presence of electrodes. This study at a pilot scale demonstrated that VFA degradation was promoted by SFAS and exoelectrogens at the anode because more electrons were produced for hydrogenotrophic methanogens at the cathode. Without the electrodes, the total methane yield was 241 mL g−1 COD, but with the electrodes, it increased to 282 mL g−1 COD, which proved that symbionts of SFAS and exoelectrogens performed better than in the conventional ABR. Through microbial electrolysis, the energy recovered from methane was 2.944 kW h m−3 while the microbial electrodes consumed 0.6 kW h m−3, so the net energy generated was 2.344 kW h m−3.59
Plastic carriers were also investigated as a growth support mechanism in pilot scale ABR studies. A 750 L pilot-scale ABR was used to treat real municipal wastewater in Bratislava, Slovakia at temperatures between 4.5 and 23 °C.45 The ABR was designed with a primary settling tank connected to 4 compartments that were filled with plastic tubes with 2–4 cm diameter and 3–5 cm length. Bigger tubes were used in the first two compartments while smaller tubes were used in the last two compartments. An aerobic polishing step was included in the process train. The HRT in anaerobic and aerobic reactors was around 15 h and 4 h, respectively. All monitored parameters were removed with relatively high efficiencies (COD = 78.6–83%, BOD5 = 92.5–94.0% and SS = 80.9–92.7%).45 The presence of the primary sedimentation tank and the good filter characteristics of the anaerobic baffled filter reactor prevented the washout of the seed sludge. The average removal of NH4-N varied during the year from 46.4% to 87.3%, while the denitrification process achieved 75–80%, demonstrating the benefits of even a small aerobic post-treatment of ABR effluents. In the summer, the COD slightly decreased but the BOD removal was slightly higher than during winter. Interestingly, the nitrification process was successful for a whole year even at low temperatures.45
Another polishing strategy of ABR effluents at a large scale involves constructed wetlands. A 103 m3 ABR (5.4 days HRT) was connected to a vertical flow constructed wetland (VFCW – 2.5 days HRT) and lastly connected to a horizontal subsurface flow constructed wetland (HFCW – 4 days HRT) to treat university dormitory wastewater at air temperatures ranging from 5 to 37.5 °C.47 The ABR was divided into 3 compartments and the first one was used as a sedimentation tank to pre-treat the water before transferring the water to the VFCW system that contained three layers of gravel acting as an intermittently loaded system to provide an anaerobic environment at the bottom of the VFCW before the effluent flowed by gravity to the HFCW. The ABR was able to remove most of the suspended solids and organic matter (48% BOD, 46% COD and 25% NH4-N removal) before reaching the VFCW. The facility proved to be effective and removed 88.9%, 86%, 92.2%, 63.5%, 66.5%, and 65.7% of BOD5, COD, TSS, PO4-P, NH4-N, and NO3-N, respectively, while the effluent met the reuse standard. The VFCW was planted with giant reed (Arundo donax) that removed 53.5% of BOD5, 42.2% of COD and 40% of NH4-N. The aerobic conditions at the top of the bed in the VFCW enhanced nitrification and oxidation of organic matter. In the last stage, the HFCW planted with common reed (Phragmites australis) removed 67.8% of TSS which was less than the national effluent reuse limit while the TDS value slightly increased which may have been caused by plant growth and increased evapotranspiration and salts released during wastewater–substrate interactions. Further removal of BOD5 (54.2%) and COD (54.7%) took place in the HFCW. Nitrate in the effluent was 5.2 mg L−1 which was below the local discharge standard (11.3 mg L−1), showing that some denitrification was achieved. Higher temperature enhanced the performance of the constructed wetlands compared to the ABR in removing BOD5 and COD by 12–15%.47
4.3 Dissolved methane in ABR effluent
In Hahn and Figueroa's pilot scale study, the dissolved methane concentration in the effluent was found to be 21 ± 5 mg L−1, which represented 39 ± 10% of the total production. It is still a challenge to recover the dissolved methane to maximise the energy value from the anaerobic process.43 Degassing membranes have been investigated by Bandara et al. and at 35 °C and 10 h HRT, the average dissolved CH4 concentration could be reduced from 63 mg COD L−1 to 15 mg COD L−1 which in turn, resulted in an increase in total methane (CH4) recovery efficiency from 89% to 97%. The dissolved CH4 concentration could be reduced down to 12 ± 1 mg COD L−1 by degasification at an HRT of 6.7 h, so the CH4 recovery rate was 1.5 times higher under degasification than under normal operation.60 Using a poly-di-methyl-siloxane (PDMS) membrane contactor, 72% of dissolved methane was recovered.61 In 2023, a laboratory granule-based sequencing batch reactor (GSBR) coupling anammox and nitrite/nitrate-dependent anaerobic methane oxidation (n-DAMO) microorganisms was developed to treat wastewater mimicking effluent from mainstream anaerobic treatment. The GSBR achieved high-level nitrogen and dissolved methane removal rates (>250 mg N L−1 d−1 and >65 mg CH4 L−1 d−1) and efficiencies (>99% total nitrogen removal and >90% total methane removal) during the long-term demonstration. This process is therefore an interesting approach to achieve concurrent nitrogen and dissolved methane removal from anaerobic effluents.62
It appears from Table 2 that municipal wastewater containing typically between 300 and 2914 mg L−1 COD could be treated successfully in ABRs and modified ABRs at HRT ranging from 6 h to 96 hours at a temperature of at least 25 °C. At organic loading rates in the range of 0.15 to 2.43 kg COD m−3 d−1, the COD removal is generally higher than 80%. It is clear that better temperature control strategies should be implemented in ABRs at a large scale, including the use of a greenhouse to house ABR, an in-ground ABR, heat exchange by recirculating warm water on the roofs heated by solar radiation, heat exchange between ABR effluent and influent or effluent recycling as well as the use of biogas for producing heat and maintaining a more constant and optimum temperature throughout the year. There is also a lack of studies focusing on the optimum temperature in ABRs and how to maintain it at a large scale. COD removal higher than 95% has been shown to be possible even at 20 °C if the SRT is high (∼100–200 days) and this is possible when a membrane is integrated to the anaerobic process, while operation at 10 °C led to COD removal lower than 50%.63 Renuka et al. achieved 90% COD removal at temperatures between 25 and 35 °C, indicating that maintaining at least 25 °C is highly recommended. Applying 35 °C may only increase the performance by a few percent and may result in a smaller volume, so there is a trade-off to find between optimum temperature and ABR performance.36 Currently, the optimum temperature in an ABR treating real municipal wastewater in terms of performance, size and economics has not been documented. Nevertheless, an aerobic post-treatment will always be required. However, it was shown that the use of sedimentation tanks before and after the ABR could lead to 80–85% COD and TSS removal at temperatures as low as 6 °C.45 The packing material in an ABR was also very efficient in removing suspended and colloidal COD, maintaining high biomass concentration, and increasing SRT, leading to better stability. A membrane system (submerged or external) is also useful to polish the effluent COD from the ABR, but there is a lack of studies looking at the inclusion of a membrane in an ABR setting as well the combination of a packing material and a membrane in an ABR.46
5 Nitrogen removal in ABRs treating municipal wastewater
The removal of nitrogenous pollutants from bioreactors is challenging.64 Organic carbon and dissolved oxygen are used to remove nitrogen conventionally by nitrification and denitrification in aerobic and anoxic tanks, respectively. However, in an ABR 60–80% of organic carbon is converted to methane before it is used for nitrogen removal.65 As a result, only recalcitrant or slowly biodegradable COD may remain after the ABR and this carbon source may not be suitable for conventional denitrification. The following sections review the latest development related to N removal in ABRs using aeration, membrane aerated biofilms, anammox and nitrite/nitrate dependent anaerobic methane oxidation bacteria. Relevant and recent studies on N removal in ABR have been compiled in Table 3.
Table 3 N removal in ABRs treating municipal wastewater
Contaminant |
Type of reactor |
HRT (h) |
TN in influent (mg L−1) |
TN removal (%) |
Ref. |
Lab-scale synthetic wastewater |
GSBR |
6 |
60 |
99% |
62
|
Synthetic wastewater |
ABR + MBR |
7.5 |
44 ± 16 |
76% |
66
|
Pilot-scale municipal wastewater |
ABR + MBR |
7.5 h |
44 ± 16 |
70% |
67
|
Domestic and nitrate wastewater |
ABR–CSTR |
5.56 |
NH4+-N 50–60 |
97.57% |
26
|
Municipal wastewater |
ABR–MBR |
— |
40.3 ± 3.6 |
53% |
50
|
Tempeh wastewater |
ABR–RBC |
34 |
300 |
90.9% |
68
|
Domestic wastewater treatment |
ABR–AF–MF |
12 |
33.48 ± 2.0 |
69% |
52
|
Municipal wastewater |
ABR–CSTR |
5.5 |
NH4+-N: 35 mg L−1, NO2−-N: 35 mg L−1, NO3−-N: 35 mg L−1 |
97.75% |
69
|
5.1 ABRs with nitrification using aeration
To achieve nitrification, aeration is required and this usually takes place in a separate reactor and by splitting the ABR into anaerobic followed by aerobic compartments. For instance, 106 L 4-compartment baffled anaerobic–aerobic reactors (AOBRs) with modified basalt fiber (MBF) carriers and felt were used to treat domestic wastewater (DWW) at 25 °C.70 A J-shaped aerator and a felt filter were operated aerobically in the first compartment whereas MBF carriers were installed in the next 3 anaerobic compartments to hold more biomass. The optimal combination was an HRT of 15 h, a recycling ratio of 300% and a COD/TN ratio = 17.1. In the second stage, the DO was fixed at 1.0–2.0 mg L−1 in the first compartment as determined by optimization experiments. Interestingly, the AOBR removed 90.9 ± 0.9% of COD, and the removal of NH4+-N was 91.4 ± 0.9%, which outperformed a similar ABR71 which removed only 14.2% of NH4+-N from black water at an HRT of 48 h at 36 °C. Nitrification in the aerobic compartment promoted the TN removal where the TN removal in the AOBR (80.7%) outperformed the study done by Feng et al. (2008),16 where only 19.2% of TN was removed in domestic wastewater using bamboo media in ABRs. The presence of MBF carriers enhanced the removal of COD at an organic loading rate of 0.59 ± 0.01 kg COD m−3 d−1.70 In an ABR–aerobic membrane bioreactor (MBR) combined process, the NOx−-N formed in the MBR can be recycled into the ABR and converted into nitrogen gas resulting in an overall nitrogen removal.66 It was found that 3 mg L−1 dissolved oxygen (DO), an HRT of 7.5 h and a recycling ratio of 200% were suitable to reduce 44 ± 16 mg L−1 TN to 10.5 mg L−1. The appropriate concentration of DO enhanced the nitrification process in the aerobic zone to remove more nitrates but excessive DO limited the activity of the denitrification process in the ABR.66 TN removal increased with the increase of the recycling ratio because more nitrates moved to the denitrification zone and were denitrified by denitrifying bacteria.72 Thus, it is important to maintain the right balance of denitrification and nitrification processes to achieve high TN removal efficiency.66
In 2015, a pilot-scale ABR–MBR was used to study the removal of nutrients (N and P) in municipal wastewater via partial nitrification and denitrifying phosphorus accumulating microorganisms (DPAOs).67 It consisted of three parts: an ABR of 60 L, an aerobic tank of 20 L, and an MBR tank of 40 L. To promote nitrification and denitrification under 20–28 °C, 7.5 h HRT and 1 mg L−1 DO conditions, the MBR mixed liquor was recycled to the first compartment of the ABR with a recycling ratio of 200%, while the aerobic tank mixed liquor was recycled to the third compartment of the ABR with a recycling ratio of 100%. The combined process achieved good effluent quality with chemical oxygen demand (COD) of 25 mg L−1, NH4+-N of 4 mg L−1, total nitrogen (TN) of 11 mg L−1, and total phosphorus (TP) of 0.7 mg L−1. The MBR achieved partial nitrification as evidenced by the presence of NO2−-N at 4 mg L−1. The final effluent TN was 11 mg L−1 (76% TN removal) due to the short-cut denitrification responsible for 51% of the TN removed, denitrifying phosphate accumulating microorganisms (14% of TN removed), and simultaneous nitrification and denitrification (11% of TN removed). In addition, phosphorus accumulating organisms achieved 84% TP removal in the MBR. Moreover, a very low sludge production (0.15–0.34 kg MLSS kg−1 COD) was achieved.67 Denitrifying bacteria and nitrate recycled back from the MBR back into the first compartment of the ABR. The first compartment contained organic carbon from raw sewage which acted as an electron donor. The removal of nitrogen was low during the first two weeks (17–22%), but its removal increased to 53 ± 6% afterwards. Recycling of the mixed liquor also reduced the ammonium concentration in the ABR effluent and the nitrate concentration in the MBR effluent.50
5.2 Membrane aerated biofilm (MAB)
In a MAB system, oxygen or air is supplied to a biofilm through a membrane so that molecular oxygen is provided directly to microbes without the need for mechanical aeration. The microbial stratification in the MAB consists of an aerobic layer near the membrane for nitrification, followed by anoxic and anaerobic layers where nitrate is removed via the denitrification process.73 This strategy was applied in a 9.5 L hybrid membrane aerated biofilm (MAB)–ABR (HMABR) which was made up of an aerating membrane module installed into the second compartment of a 3-comparment ABR to improve the removal rate of carbonaceous and nitrogenous pollutants.73 Initially, the COD of the synthetic wastewater was 1600 mg L−1 with 80 mg L−1 ammonium-nitrogen and then the COD was adjusted to 2400 mg L−1 to investigate an organic shock. Before the installation of the MABR into the second compartment of the ABR, the effluent COD concentration from the second compartment of the ABR was 373 mg L−1 and that from the third compartment was 126 mg L−1, and after installation of the MABR and initiation of membrane aeration, the effluent COD concentration decreased to 127 mg L−1 and 51 mg L−1, respectively, in compartments 2 and 3. Interestingly, the COD in the effluent from the third compartment was still below 60 mg L−1 although the influent concentration was 2400 mg L−1, proving the efficacy of the HMABR. During the experiment, the average values of ammonium, nitrite and nitrate concentrations in the second compartment effluent were 13.2, 1.6 and 12.5 mg N L−1, respectively. After installation of the membrane in the initial phase, nitrate in the third compartment decreased to below detection. However, ammonium in the third compartment effluent was higher than the second one possibly due to ammonium production from nitrate or cell lysis linked to the low substrate level. Increased influent COD adversely affected the nitrogen removal and the ammonium effluent increased to 36 mg N L−1. This is because when COD increased, more oxygen was needed for the activity of the aerobic heterotrophic bacteria and nitrifying bacteria. The overgrowth of aerobic heterotrophic bacteria thickened the biofilm and restricted the flow of ammonium from liquid to the biofilm. The average VFA concentration was 148 mg L−1 and it decreased to 39 mg L−1 as VFAs were degraded by aerobic heterotrophic bacteria. In the third compartment, methane gas was produced by acetoclastic methanogenesis by consuming 64% of acetic acid. The total VFAs and COD in the HMABR effluent were reduced by 68.1 and 59.5%, respectively, and 83.5% of nitrogenous pollutants were removed by ammonia-oxidizing bacteria, nitrite-oxidizing bacteria, aerobic heterotrophic bacteria, and denitrifying bacteria.73
5.3 Anammox process in ABRs
Anaerobic ammonium oxidation (anammox) is an autotrophic process that converts ammonium and nitrite to dinitrogen gas and nitrate (NO3−),74,75 is recognised as an effective way to remove nitrogen and has been used to treat side-stream wastewater at a field scale76,77 to reduce N and carbon compounds.78 However, anammox is still facing some challenges such as the growth rate of biomass and partial nitritation (Wang et al., 2020).77 Long start-up time, keeping up the anaerobic environment in the reactors, temperature, pH, and the presence of sulphide, nitrite, ammonia, phosphate and other compounds in various wastewater streams adversely affect the performance of growing anammox bacteria for large-scale wastewater treatment.79,80 Anammox bacteria (AnAOB) remove nitrogen without using carbon and oxygen. Hence, in theory they are applicable to treat wastewater with a low C/N ratio from an ABR.76,81 The AnAOB are sensitive to temperature and the optimum temperature for AnAOB is approximately 37 °C.82 The anammox process in treating real WWTPs is inhibited when the temperature is lower than 20 °C.83 Nitrite for anammox is supplied via nitritation (NH4+-N → NO2−-N). However, the excessive growth of nitrite-oxidizing bacteria (NOB) regularly occurs in the current mainstream nitritation/anammox process which leads to fluctuation in the nitrite to ammonium ratios and reduced nitrogen removal efficiencies. Recently, a 32 L ABR was used to treat domestic wastewater supplemented with trace nutrients to study the growth and activity of anammox bacteria under controlled DO conditions and at temperatures between 19 and 22 °C.78 Initially, the nutrient-rich feed and trace element solution were fed to the ABR for 14 days and anammox biomass was added to compartments 2 to 4. A semi-batch feeding mode with continuous pumping for 18 h followed by 6 h of static conditions mimicked a decentralised wastewater treatment system. The removal of NH4-N and NO2-N was greater than 90%. The removal of NH4-N was effective in compartment 1 (58%), and an additional 31% removal occurred in compartment 2. The removal reached 90% in compartments 3 and 4. For NO2-N, the removal was the highest in the first two compartments, but the removal process still occurred in compartments 3 and 4. Anaerobic batch tests containing biomass, ammonia and nitrite showed a specific anammox activity (SAA) ranging from 0.28–0.48 g N g−1 VSS d−1. The highest removal of NH4-N (56%) and NO2-N (23%) occurred at 0.48 g N g−1 VSS d−1. The low maintenance, intermittently-flowing and ambient temperature ABR was found to be effective in removing NH4-N and NO2-N. The authors recommended a 3 compartment ABR with biomass carrier media in one or more chambers for full-scale treatment.78 This study shows that anammox treatment could take place following COD removal and biogas production from an ABR treating raw municipal wastewater. However, the application of simultaneous partial nitrification, anammox, and denitrification (SNAD) faces two major problems including low ammonium concentration (<100 mg L−1) and a high ratio of chemical oxygen demand (COD) to nitrogen (C/N ≥2) in domestic sewage, which are likely to bring nitrate accumulation and lead to the deterioration of effluent quality. Previous studies (Wang et al., 2018)84 indicated that when treating simulated urban domestic sewage (NH4+-N = 50 mg L−1) with the SNAD process, the highest total nitrogen (TN) removal efficiency was still below 80%, once the C/N ratio was over 2.0. Assuming that the influent TN, COD, and TP were 50, 150, and 8–15 mg L−1, respectively, the first ABR in Fig. 1 would achieve 90–95% COD removal efficiency with simultaneous denitrifying phosphorus removal, reducing the effluent C/N to 0.8–1.0, satisfying the operating conditions of the following SNAD process in the second reactor.
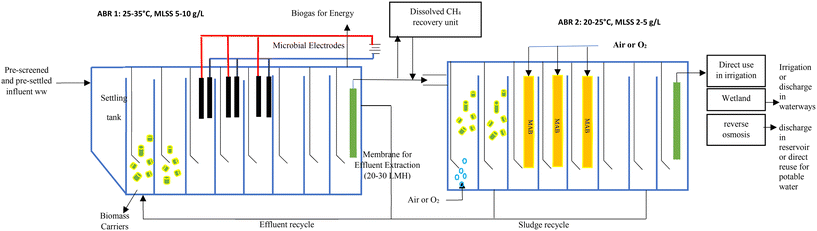 |
| Fig. 1 Proposed ABR process for concurrent COD and nitrogen removal and energy production. LMH = L m−2 h−1. MAB = membrane aerated biofilm. | |
Recent reports also showed that endogenous partial-denitrification (EPD) driven by glycogen accumulating organisms (GAOs) could provide steady NO2−-N for anammox with a high nitrate-to-nitrite transformation ratio of 87%.85 There is therefore potential for achieving nitrogen and phosphorus removal by combining anammox with EPD and denitrifying dephosphatation in two-stage reactors.86 Wu et al. studied the feasibility of NH4+-N, NO3−-N and PO43−-P removal via denitrifying phosphorus removal (DPR) + partial denitrification anammox (PDA) without using aeration for 220 days as nitrite/nitrate is used instead of dissolved oxygen. The DPR + PDA process was operated at 33 °C and 5.56 h HRT in an ABR followed by a CSTR for simultaneously treating synthetic domestic and nitrate wastewater in continuous mode. The process required inoculation with anammox bacteria and an external source of nitrate to control C/NO3−-N accurately. In this study, 58.65% of TN was mainly due to the dilution effect via three compartments, the presence of nitrogen affiliated bacteria and DPR bacteria that removed the nitrogen while PDA strengthened the NH4+-N removal and contributed 37.07% in removing TN. Overall, 97.57% N and 95.66% P removal was obtained under external C/NO3−-N of 0.7, with the effluent total inorganic nitrogen and PO43−-P concentrations of 3.51 mg L−1 and 0.28 mg L−1, respectively. The high-efficiency partial denitrification and anammox (PDA) coupling process has been well developed for simultaneously treating nitrate and municipal wastewater, with reduction in 50% aeration energy, 80% organic demand, 80% CO2 contribution and 64.8% less biomass production. Yuguang et al. (2022)69 used the DPR and PDA processes in an ABR (3 compartments, 5.4 L) and CSTR (2.7 L) integrated process to remove nitrogen and phosphorus. The final effluent PO34−-P and TN concentrations were as low as 0.22 mg L−1 and 3.30 mg L−1, respectively. The removal of PO43−-P and TN by DPR accounted for 99.07% and 60.23% of the total respectively, while the proportion of TN removal by PDA increased gradually from 4.53% to 37.52%. The highly-efficient partial denitrification process (92.25%) supplied a stable electron acceptor (NO2−-N) for anammox, and the residual NH+4-N of the DPR system was mainly eliminated by NO2−-N oxidation. Therefore, the DPR + PDA system achieved high-efficiency and synchronous nitrogen and phosphorus removal. High throughput sequencing showed that Thauera (7.24%) and Candidatus Brocadia (3.12%) were the key nitrogen removal bacteria in the PDA system.26
5.4 Nitrite/nitrate-dependent anaerobic methane oxidation (n-DAMO)
Dissolved methane in wastewater can be used as an external carbon source to perform denitrification.87–89 Methane-supported nitrate removal (reaction 1) and methane-supported nitrite removal (reaction 2) could be conducted by an archaeal (Haroon et al., 2013)88 and a bacterial group (Ettwig et al., 2010),87 respectively. These two microbial groups are named after their functions as n-DAMO archaea and n-DAMO bacteria (nitrite/nitrate-dependent anaerobic methane oxidation), respectively. | CH4 + 4NO3− → CO2 + 4NO2− + 2H2O | (1) |
| 3CH4 + 8NO2− + 8H+ → 3CO2 + 4N2 + 10H2O | (2) |
Liu et al. used a 2 L laboratory granule-based sequencing batch reactor (GSBR) to study the effectiveness of n-DAMO and anammox at 22 °C using synthetic wastewater. With different influent nitrite to ammonium molar ratios (2
:
1–5
:
1), the system was robust in terms of a stable TN removal efficiency of >99% and a TN removal rate of >115 mg N L−1 d−1 at a fixed HRT of 12 h. The GSBR achieved high-level nitrogen and dissolved methane removal rates at 6 h HRT (>250 mg N L−1 d−1 and >65 mg CH4 L−1 d−1) and efficiencies (>99% total nitrogen removal and >90% total methane removal) during the long-term demonstration. Effluent quality <1 mg N L−1 was achieved when nitrite was used as an electron acceptor. This study showed that N removal is feasible in mainstream wastewater treatment, but more work should be done in continuous reactors treating real wastewater. Given the fact that real wastewater composition varies from time to time, the robustness of the GSBR coupling anammox bacteria and n-DAMO microorganisms against dynamic feedings is a valuable feature in practical applications. To scale up, a 3 step process including the upfront mainstream bioenergy recovery via anaerobic wastewater treatment followed by an anoxic/oxic process was suggested. Granules coupling anammox and n-DAMO microorganisms are implemented in the anoxic tank to remove ammonium and dissolved methane in anaerobic effluent, using electron acceptors recirculated from the oxic tank performing nitrification or nitritation.62
In conclusion, previous studies show that ammonia and nitrogen removal is possible in a modified ABR. An ABR followed by aerobic and anoxic tanks or an MBR with internal recycling can also achieve simultaneous N and P removal of 76% and 84%, respectively.67 80% TN removal was achieved in an ABR with aerobic conditions in the first compartment, while 83.5% TN removal was reported in a membrane aerated biofilm reactor.73 Anammox studies using partial nitritation (NH4+ → NO2−) have demonstrated up to 90% NH4+-N and NO2− removal, but the process was relatively unstable.78 Instead of partial nitritation, partial denitrification (NO3− → NO2−) can provide a more stable stream of nitrite for anammox and coupling denitrifying phosphorus removal could lead to N and P effluent values well below the discharge standards. Up to 99% TN removal and <1 mg N L−1 in the effluent were obtained with synthetic wastewater in a sequencing batch reactor using n-DAMO bacteria and archaea. However, these studies were carried out under well controlled laboratory conditions (temperature, DO, C/N ratios) using synthetic wastewater and inoculating the reactors with anammox and denitrification bacteria. These studies have not looked at concurrent production of methane gas from raw organics in municipal wastewater and demonstration at a pilot scale remains to be seen. Based on the above studies, the process depicted in Fig. 1 is proposed to remove COD, N and P while producing biogas from screened municipal wastewater. It can be seen that COD and N removal would take place in 2 separate ABRs. In ABR1 (max. 7–8 compartments with temperature control), up to 90–95% COD removal will take place at 48 hours HRT along with maximum 20% N removal due to cell synthesis. A sedimentation tank would be required prior to the ABR to capture TSS from raw municipal wastewater. The ABR would contain some packing material to retain slow-growing methanogens and could include microbial electrodes for efficient VFA consumption, an enhanced methane yield and COD polishing (Wang et al., 2020).59 A dissolved methane recovery unit should be included to minimize methane losses. In addition, a submerged membrane could be included in the last compartment as high COD could inhibit the anammox process in ABR2 (Fig. 1). Ammonia and TN removal would take place in ABR2 using a combination of the anammox process and MAB and by providing 1–2 mg L−1 DO in the first compartment. Recycling to the first compartment of ABR1 (anoxic) will provide denitrification. A MAB system can be incorporated within ABR2 as shown by Hu et al. who achieved simultaneous carbon (60% COD removal) and nitrogen (83.5%) removal within the same ABR, but the process was unstable at higher loading rates. It is therefore suggested to include several MAB membranes within ABR2 with regular maintenance of biofilm thickness. A NO2/NO3 liquid and sludge recycling from ABR2 to ABR1 would promote denitrification, anammox, n-DAMO and denitrifying phosphate accumulating microorganisms in ABR1 achieving simultaneous N and P removal, but the exact location of the recycling lines and recycling ratios would need to be optimized. Finally, residual COD can be removed with either an electrochemical oxidation system using electrodes or with a final microfiltration membrane for effluent extraction. Final polishing could be achieved using a constructed wetland if space is available or reverse osmosis if the water is to be reused for drinking water production. Appropriate temperature control would be required for both ABRs if stringent limits were required, but temperature control would not be necessary if the effluent was to be used for irrigation.90
6 Phosphorus removal in ABRs treating municipal wastewater
Conventional enhanced biological phosphorus removal in wastewater treatment plants requires an anaerobic tank for phosphate accumulating microorganisms (PAOs) to grow using acetate and release phosphate during feasting. In the subsequent anoxic and oxic tanks, PAOs absorb excessive phosphate, thereby removing phosphate from wastewater.91 This requires both internal and sludge recycling streams. The anaerobic environment in a single ABR is generally not suitable for the traditional phosphorus removal process and since up to 33% TP can be removed due to bacterial uptake in the ABR alone, it will generally not be sufficient to meet strict discharge criteria.30,92 This is consistent with Khan et al. who investigated an ABR–anaerobic filter and anaerobic membrane filter process. Because of anaerobic conditions, the TP removal was 33 ± 1.98% at an HRT of 12 h and it increased to 35 ± 1.34% of TP with Kaldnes media in the filter. A combination of suspended growth and attached growth in the ABR and anaerobic filter therefore enhanced the TP removal efficiency.52 Recent studies have focused on novel and advanced strategies to improve phosphorus and phosphate removal in modified ABRs (Table 4).
Table 4 P removal in ABRs treating municipal wastewater
Wastewater |
Type of reactor |
HRT (h) |
TP in influent (mg L−1) |
TP removal (%) |
PO43−-P in influent (mg L−1) |
PO43−-P in effluent (mg L−1) |
Ref. |
Raw municipal wastewater |
ABR + EABR |
24 |
23 ± 2 |
>98 |
23 ± 1 |
0.4 |
41
|
Tempeh wastewater |
ABR–RBC |
34 |
90 |
88.8 |
— |
— |
68
|
Synthetic wastewater |
ABR + MBR |
7.5 |
4 ± 3 |
0.4 mg L−1 |
— |
— |
66
|
>90% |
Pilot-scale municipal wastewater |
ABR + MBR |
7.5 |
4 ± 3 |
84 |
— |
— |
67
|
Domestic and nitrate synthetic wastewater |
ABR–CSTR |
5.56 |
4–8 mg L−1 |
95.66 |
4–8 |
0.28 |
26
|
Municipal wastewater |
ABR–MBR |
— |
— |
— |
12.3 ± 3.3 |
— |
50
|
Municipal wastewater |
ABR–CSTR |
5.5 |
— |
96.91% |
6–8 |
0.18 |
69
|
Domestic wastewater treatment |
ABR–AF–MF |
12 |
14.48 ± 0.60 |
35% |
— |
9.43 ± 1.7 (TP) |
52
|
Aqaneghad and Moussavi investigated phosphate removal in a 37 L ABR modified with an electrocoagulation system (EABR) fed with screened raw municipal wastewater containing 23 ± 2 mg L−1 phosphate. Using aluminium electrodes at a current density of 0.1 mA cm−2, the EABR could decrease the phosphate concentration below the standard limits for discharge into local surface water bodies (0.4 mg L−1). Aluminium electrodes were found to be more efficient than steel ones and the process could further lower the BOD from 47 to 19 mg L−1. Conventional coagulation using aluminium or iron dosing could also benefit the ABR in terms of phosphorus removal, but this has not yet been documented. The exact location of iron dosing (pre-ABR or post-ABR) would also need to be investigated, but the EABR is a promising and simple-to-operate process for efficient treatment of municipal wastewater to meet regulatory levels without the need for chemical dosing.41
Enhanced phosphorus removal using biological methods has also been investigated in ABRs. Peng and Ge investigated the effect of DO on P release and uptake. The optimum DO of 3 mg L−1 was suitable for aerobic phosphorus uptake and didn't affect the anaerobic phosphorus release at 7.5 h HRT with a recycling ratio of 200% which recycled an appropriate amount of nitrate to the anaerobic zone to be taken by phosphorus accumulating organisms (PAOs).91 When the microorganism population and nitrate concentration in the anaerobic zone are maintained, around 90% of TP can be achieved by PAOs.66 Denitrifying phosphorus removal (DPR) is considered the most promising EBPR process using NO3−-N/NO2−-N instead of oxygen.93,94 In this process, the functional microorganisms denitrifying phosphorus accumulating organisms (DPAOs) using one same exogenous carbon source are the key factor in achieving simultaneous N and P removal. In a pilot scale ABR–aerobic tank–MBR (60–20–40 L) operating at 7.5 h HRT, the MBR mixed liquor was recycled to the first compartment of the ABR with a recycling ratio of 200%, while the aerobic tank mixed liquor was recycled to the third compartment of the ABR with a recycling ratio of 100%. The combined process achieved good effluent quality with chemical oxygen demand (COD) of 25 mg L−1, NH4+-N of 4 mg L−1, total nitrogen (TN) of 11 mg L−1, and total phosphorus (TP) of 0.7 mg L−1 (Wu et al., 2015).67 A low DO in the aerobic MBR can inhibit the activity of PAOs, but they were able to adapt to the low DO conditions and enhanced the phosphorus removal. The presence of phosphorus in the second compartment of the ABR, P uptake by denitrifying phosphorus accumulating organisms (DNPAOs) in the third compartment of the ABR and sufficient aerobic phosphorus uptake by PAOs in the aerobic MBR all together contributed to the removal of 84% of the influent TP and the final effluent TP was around 0.7 mg L−1.67 These studies demonstrate promising outcomes for mainstream anaerobic treatment of municipal wastewater with N and P removal. Denitrifying phosphorus removal (DPR) also played a major role in PO43−-P removal in an ABR–CSTR process using NO3−-N as an electron acceptor.26 The phosphorus release and uptake rates were 7.73 and 22.17 mg P g−1 VSS h−1, respectively. Accumulibacter (6.24%) dominated high phosphorus removal performance. A decline in P removal can be related to high organic matter in the influent, which can cause excessive residual COD into the subsequent anoxic zone, and as a result, the P uptake anoxically might be inhibited by the overgrowth of denitrifiers and GAOs. Favourable conditions for DPAOs against glycogen accumulating organisms (GAOs) included 200 mg L−1 COD, 90 mg L−1 NO3−-N and a suitable influent NO3−-N/NH4+-N ratio (1.0–1.3) which led to less than 0.5 mg L−1 P in the effluent.26 The optimum COD/NO3−-N was 0.7 together with the nitrogen loading rate (NLR), organic loading rate (OLR) and phosphorus loading rate (PLR) maintained at 0.64 kg m−3 d−1, 0.90 kg m−3 d−1 and 0.03 kg m−3 d−1, respectively, leading to consistently low concentrations averaging 1.09 mg N L−1 and 0.34 mg P L−1 in the effluent. However, to achieve this, the process required strict control of influent organic matter and NO3−-N concentrations at 90 mg L−1 which is unlikely in municipal sewage, as well as controlling the external COD/NO3−-N ratio using synthetic solutions. It also required a long time to achieve stable operation. More than 90% P removal was also obtained in an ABR–MBR system operating at 3 h HRT with a specific phosphorus uptake rate of 4.82 mg P g−1 MLSS h−1, suggesting that PAO biomass can grow well in the ABR–MBR system.54
In the denitrifying phosphorus removal (DPR) and partial denitrification anammox (PDA) processes established in an ABR–CSTR integrated process,69 the initial PO43−-P in the influent was 6–8 mg L−1. During the DRP startup, the removal of PO43− was only 34.2% which was caused by the slow activity and high concentration of COD entering the anoxic zone and affecting the absorption of P. Batch experiments showed that a high concentration of COD (300 mg L−1) significantly inhibited the activity of DPR bacteria. PO43−-P was mainly removed by DPR with NO3−-N as an electron acceptor and organic matter as an electron donor under anoxic conditions. After reducing the concentration of COD and NO3−-N, the removal of PO43− was 96.91% showing that DPAOs have the ability to absorb excess phosphorus under anoxic conditions. High throughput sequencing showed that Accumulibacter (7.41%) was the functional phosphorus removal bacteria in the DPR system. However, studies on simultaneous N and P removal using DPR and PDA processes used synthetic wastewater in a laboratory with a very short anaerobic stage. These studies did not consider carbon conversion to biogas and dissolved methane in effluent. Application of DPR and PDA during the mainstream treatment of municipal wastewater using real wastewater remains therefore to be seen.
7 Application of ABRs for emerging or persistent pollutant removal in municipal wastewater
7.1 Introduction
Large amounts of domestic wastewater are being generated due to rapid urbanization threatening human health and the environment.4 Different chemical substances such as perchlorate, nitrobenzene and nitrophenols, 2-chlorophenol and antibiotics such as kemicetine and azo dye are commonly detected in municipal wastewater. Perchlorate and nitrophenols are listed by the United States Environmental Protection Agency (USEPA) as drinking water contaminants95 and appear in the Priority Pollutants List96 because perchlorate can interfere with our thyroid system84 while nitrophenols are carcinogenic and mutagenic97 and it was reported that nitrophenols and other nitrogenous compounds are biorecalcitrant and difficult to degrade in conventional wastewater treatment plants.98 Antibiotics derived from pharmaceutical wastewater are commonly detected in surface water and groundwater99 due to the difficulty of treating it in conventional treatment systems such as the aerobic activated sludge system and anaerobic process.100 This is a problem that needs to be addressed as it causes an increase in antibiotic-resistant bacteria.101,102 ABRs have been used successfully to treat municipal wastewater at a laboratory scale but their implementation at a large scale requires to look carefully at their sensitivity towards emerging contaminants. For this reason, the application of ABRs for the treatment of specific pollutants in municipal wastewater is reviewed in the following sections. Table 5 shows the application of ABRs in treating raw municipal wastewater with a specific focus on emerging contaminants, pesticides, antibiotics and other pharmaceutical and personal care products and their impact on the treatment of municipal wastewater.
Table 5 Pollutant removal by ABRs treating municipal wastewater
Raw WW composition |
ABR characteristics |
ABR performance |
Contaminant (concentration in mg L−1) |
COD (g L−1) |
SS (g L−1) |
Temperature, (°C) |
Design modification |
Volume (L), HRT (d) |
Contaminant removal (%) |
COD removal (%) |
Methane yield (L per day or L g−1 COD removed or L g−1 added |
Methane % in the biogas |
Ref. |
Perchlorate (step feeding) (SW, 800) |
0.398 |
NR |
28 ± 2 |
45° baffle |
46, 2 d |
97.56 |
90.7–91.7 |
NR |
NR |
103
|
Perchlorate (normal-feeding) (SW, 800) |
0.398 |
NR |
28 ± 2 |
45° baffle |
46, 2 d |
86.27 |
90.7–92.2 |
NR |
NR |
p-Nitrophenol(700) (80) |
3 |
40 |
37 ± 1 |
45° baffle |
38.4 L |
99 |
90 |
1.5 L per day |
60 |
104
|
3 |
NR |
37 ± 1 |
45° baffle + aerobic CSTR |
38.4 L, 10.38 days |
93–99 |
80–99 |
Methane yield 4.32 L per day |
34–39 |
105
|
Kemicetine antibiotic (SW, 49) |
2.8–3.3 |
3.4–4.1 |
37 ± 1 |
45° baffle + aerobic CSTR |
38.4 L, 12.8 days |
96 |
83 |
0.025 m3 per day |
67 |
100
|
2-Chlorophenol (SW, 200) |
<0.56 |
12 |
24 ± 3 |
45° baffle. Anoxic baffled reactor using nitrate |
13.7 L, 1 day |
>99 |
>99 |
NR |
NR |
106
|
Nitrobenzene (RW, 80) |
0.72 |
|
30 ± 1 |
ABR with wetland as post-treatment |
12.8 L (ABR) |
97.02 (ABR) |
73.53 (ABR) |
NR |
NR |
107
|
6000 L (wetland), 2 days |
73.93 (wetland) |
83.22 (wetland) |
Nitrobenzene (SW, 100) |
3.08 |
40 (ABR), 2.9 (CSTR) |
37 ± 1 |
ABR + CSTR |
38.4 L, 10.38 days |
95 |
98 |
1.5 L per day |
48–50 |
108
|
Catechol solution (RW, 150) |
3 |
29 |
Room temperature |
Coupling reactor ABR-BEF |
3.15 L, 1 day |
97.8 |
95.44 |
NR |
NR |
109
|
7.2 Impact of perchlorate on municipal wastewater treatment in ABRs
Perchlorate (ClO4−) is a strong oxidant that is chemically stable and highly soluble in water and has low adsorption affinity that can remain in water and sediments for a long time.110,111 It is generated during the manufacturing of rocket propellants, fireworks and explosives.112 An experiment was conducted by Li et al. (2019)103 to compare the performance of a step-feeding ABR (SF-ABR) and a normal-feeding ABR (NF-ABR) in treating synthetic municipal wastewater contaminated with perchlorate at 24 h HRT. At influent COD concentrations in the range of 160–545 mg L−1, the COD removal increased over time from 80% to 92 indicating that perchlorate did have a negative impact in the range of 300–1000 mg L−1, but the authors noted an improvement over time due to acclimatization and the stepwise feeding strategy. The ABR, with its separated reaction units connected in a series, has superiority over other anaerobic reactors in segregating functional bacteria. Perchlorate removal was achieved not only through heterotrophic perchlorate reduction in the first 5 compartments filled with activated sludge, but also via sulfur-based autotrophic perchlorate reduction in the last 3 compartments filled with sulfur particles acting as an electron donor. The removal of 800 mg L−1 perchlorate was 98%. The COD concentration increased due to perchlorate, but then decreased to 30–50 mg L−1 after sludge acclimated. This shows that perchlorate is not likely to affect municipal wastewater treatment in an ABR as long as the concentration remains below 500 mg L−1. A positive relationship between tryptophan-like materials in the ABR and the perchlorate removal rate suggested that the tryptophan-like materials can promote heterotrophic perchlorate sludge activity. In addition, Methyloversatilis, Sulfurisoma, Sulfuritalea and Ignavibacterium were associated with sulfur-autotrophic perchlorate reduction, while Acinetobacter, Pseudomonas and Longilinea were responsible for heterotrophic perchlorate reduction in the ABR. The stepwise feeding strategy, feed splitting between compartments 1 and 3 to avoid toxic shock, careful monitoring of influent wastewater and inoculation of these specific strains will assist in maintaining COD removal higher than 90%.103
7.3 Impact of nitrophenols on municipal wastewater treatment in ABRs
Nitrophenols and nitroaromatic compounds are commonly used in manufacturing explosives, pharmaceuticals, leather and rubber chemicals.96,97,113,114 2-Nitrophenol (2-NP), 4-nitrophenol (4-NP), and 2,4-dinitrophenol (2,4-NP) are listed by the US Environmental Protection Agency (USEPA) as “Priority Pollutants”.105 Nitrophenols and nitroaromatic compounds are difficult to degrade by conventional wastewater treatment methods.98 Anaerobic biological remediation might be able to remove nitrophenol pollutants as anaerobic microorganisms can degrade these compounds.115 Kuşçu and Sponza (2005)104 used a 38.4 L 4 compartment ABR to study the removal of p-NP from synthetic municipal wastewater. Each compartment was further divided into two by slanted edge (45 °C) baffles to encourage mixing within each compartment, and within each compartment, down-comer and up-comer regions were created. The HRT was 10.38 days and the SRT ranged from 400–600 days. After an initial phase (60 days) with no p-NP, the concentration of p-NP was adjusted from 10 to 700 mg L−1 at a loading rate of 0.96 and 67.9 g m−3 per day while the concentration of COD was kept at 3000 mg L−1. The steady state was achieved when the COD removal was greater than 75% and the methane percentage was 45%. After 45 days, it was found that the COD removal was constant at 94% and the methane gas yield increased from 24 (10% CH4 in the biogas) to 1120 mL per day (55% CH4 in the biogas) on day 18 and remained stable at 1500 mL per day (60% CH4 in the biogas) indicating that the performance of the ABR was stable. At an initial p-NP loading rate of 0.96 mg L−1 per day, 92% of COD was removed and the removal remained above 90% until 33.9 mg L−1 per day. At the loading rate of 67.9 mg L−1 per day, the COD dropped to 79%. The p-NP removal efficiency was the lowest (82%) when the loading rate was 0.97 mg L−1 per day and the highest (99%) at 8.32 mg L−1 per day and remained at 99% until 67.9 mg L−1 per day.104 The methane percentage in the biogas rose from 44% to 53% when the p-NP concentrations ranged from 15 to 85 mg L−1 indicating no inhibition of methanogens at these concentrations through acclimatization of anaerobic granules. p-Aminophenol was found to be the main intermediate through anaerobic degradation of p-NP which later was broken down to phenol and ammonia via deamination under methanogenic conditions. Through the anaerobic toxicity assay, the p-NP phenol that resulted in a 50% decrease in methanogenic activity was found to be 26.5 mg L−1. In 2006, Kuşçu and Sponza carried out another experiment to study the removal of 80 mg L−1p-NP in a 4-compartment ABR followed by an aerobic completely stirred tank reactor (aerobic CSTR). The optimum OLRs for maximum methane gas production ranged between 0.3 and 2.1 kg COD m−3 per day.105 An aerobic step was required to degrade nitrophenol to form carbon dioxide and water which would otherwise not be possible with the ABR alone. The overall COD removal in the ABR + CSTR was 94% until an OLR of 2.1 kg COD m−3 while the p-NP removal ranged from 93 to 99%. 28% to 38% of the phenol in the effluent of the anaerobic reactor was removed in the aerobic CSTR while 42% to 97% of the ammonia generated in the anaerobic reactor was converted to nitrite and nitrate in the aerobic reactor.105 This demonstrates that an aerobic step is required to achieve removal of certain emerging contaminants from municipal wastewater.
7.4 Impact of chlorophenols on municipal wastewater treatment in ABRs
Chlorophenols (CPs) are highly soluble and persistent compounds generated from petrochemicals, oil refining, tannery and paper mills or generated from commercial and domestic products such as pesticides, biocides, drugs, dyes, and home-care products.116,117 CPs and nitrate acted as an external electron donor and an external electron acceptor that can enhance the biodegradation and mineralisation of chlorophenols. Moussavi et al. (2014) investigated the effectiveness of an anoxic baffled reactor (AnBR) in treating chlorophenols using nitrate as an electron receptor.106 The batch mode operation was switched to a continuous mode where the CP concentration (50–700 mg L−1), HRT (2–24 h), salinity (0.5–40 g L−1 NaCl), and anaerobic conditions were studied for 167 days after completing the start-up. 97.6% COD was removed at 24 hours HRT when 200 mg L−1 CP was fed after the start-up operation. COD removal was 92.6% and 90.7%, respectively, at CP concentrations of 350 mg L−1 and 500 mg L−1, while the CP removal did not change significantly (from 99.9% and 99.3%). However, the COD removal decreased below 5% when the concentration of CP increased from 500 to 700 mg L−1. CP could not be degraded completely as the sole substrate during the reaction. With 200 mg L−1 CP, the COD removal was over 99% when the HRT was reduced from 24 h to 18 h and then 12 h. The AnBR could remove over 94% of both COD and CP at 8 hours HRT, but further reduction of HRT to 2 h led to 5% and 0, respectively. Because of the anoxic conditions, no methane gas was produced in the process. The granules in the AnBR were found to have a size ranging from 0.6 to 1 cm after 60 days of operation. The effect of salinity on the degradation of CP was also investigated. With 10 mg L−1 NaCl, 99.9% of 200 mg L−1 CP was removed, but the removal decreased to 75.3% when the NaCl concentration increased to 20 mg L−1. With 40 mg L−1 NaCl, severe inhibition was observed, but the AnBR recovered within 2 days to reach 99% removal again.106
7.5 Impact of kemicetine on municipal wastewater treatment in ABRs
Kemicetine is an antibiotic commonly used for bacterial infections. Aerobic activated and sewage treatment systems were not able to remove antibiotics so they have been detected in the effluent from wastewater treatment plants and water bodies.118,119 In a study conducted by Sponza and Demirden (2010),100 an acclimatization period of 30 days was applied before spiking the wastewater with kemicetine at concentrations ranging from 32 to 125 mg L−1. The HRT was kept constant at 1.2 days and the SRT was 45 days in the ABR which was followed by an aerobic CSTR with a sludge retention time of 20 days. The influent COD concentration and COD organic loading rate were 3000 mg L−1 and 0.23 g L−1 per day, respectively. 83% of COD and 96% of kemicetine could be removed in the process. The methane percentage in the ABR decreased from 70% to 67%, when the concentration of kemicetine was 49 mg L−1. When the concentration of kemicetine increased to 125 mg L−1, the COD removal decreased to 78%, 94% of kemicetine was removed and the methane percentage dropped to 62%. The high kemicetine removal in the ABR was due to the conversion of kemicetine to the corresponding metabolites. However, residual COD in the effluent was not due to undegraded kemicetine, but due to the presence of inerts and slowly degradable organics in pharmaceutical wastewater.120,121 The BOD5/COD ratios in the effluent rose from 0.15 to 0.37 in the ABR and from 0.19 to 0.49 in the aerobic CSTR indicating that kemicetine was converted to more biodegradable by-products. The aerobic CSTR was beneficial for removing COD and residual kemicetine from the ABR.100
7.6 Impact of nitrobenzene on municipal wastewater treatment in ABRs
Nitrobenzene (NB) is an intermediate to synthesize aniline122 and its derivatives are widely used to manufacture pesticides, explosives, plastics and pharmaceuticals.107 Improper handling of nitrobenzene wastewater can pollute surface waters and groundwater. The nitro groups of NB make it biorecalcitrant and difficult to remove by biological treatment and conventional chemical oxidation.123 A 12.8 L ABR maintained at 30 °C followed by a 6 m3 constructed wetland was used to study the removal of 80 mg L−1 NB.107 The function of the wetland was to filter and adsorb suspended solids. The ABR alone was able to remove 8.26–79.73 mg L−1 NB with a removal percentage of 98.9% (NB organic load 0.34 mg NB L−1 h−1) and 93.9% (3.32 mg NB L−1 h−1) respectively. The activity of microorganisms was slowed down by high nitrobenzene concentration. When the HRT of the ABR was 24 h and the hydraulic load for the constructed wetland was 5.4 cm d−1, 97.02% of 79.73 mg L−1 NB and 73.94% of aniline in the ABR–wetland were removed. NB was nearly converted to aniline by anaerobic reactions but it was difficult to decompose in the ABR. In the wetland, the removal efficiency was 97.29% and the aniline effluent concentration of 0.49 mg L−1 was safe to discharge into the water body. The COD in the effluent was less than 58 mg L−1 (>92% removal) indicating that the ABR–wetland performed better than the ABR (73.53%) and wetland (83.22%) alone.107 In another paper, an ABR followed by an aerobic CSTR was constructed to study how the HRT and NB concentrations affected the performance of the ABR–CSTR.108 The HRT was initially fixed at 14 days in the ABR/CSTR and the NB concentrations ranged from 30 to 700 mg L−1. When the NB concentration was 30 mg L−1, the COD removal was 88% and the removal increased to 92% (210 mg L−1) and decreased to 85 (550 mg L−1) and 79% (700 mg L−1). Nearly 100% of all NB concentrations were removed in the ABR and most of the NB was degraded to aniline. Aniline was completely removed in the aerobic reactor until an influent NB concentration of 210 mg L−1 and decreased to 89% (ref. 108) when the concentration of NB increased due to high toxicity towards aerobic microorganisms.124 The overall NB and COD removal in the ABR–CSTR was 95% and 98%, respectively. The highest methane percentage was 48–50% when the NB concentration was 210 mg L−1. The optimum HRT for maximum aniline removal varied between 5.19 and 10.38 days in the anaerobic reactor and 3.5 to 6.9 days in the aerobic reactor. An HRT of 3.5 days and high NB (700 mg L−1) did not significantly affect the efficiency of the ABR/CSTR sequential system. However, the HRT significantly affected the production of methane gas. The ABR/CSTR can be used to treat toxic compounds such as NB which was converted to its intermediate, aniline, in the ABR and continuously converted to catechol in the CSTR. From Table 5, it can be seen that the removal of specific compounds was successful up to a certain threshold that must be determined on a case-by-case basis. For instance, p-nitrophenol, kemicetine and 2-chlorophenol were found to be detrimental to COD removal when present above a certain concentration. It was also observed that nitrogen and ammonia–nitrogen were not investigated in these studies, so there is a lack of information available to ascertain whether xenobiotics affect nitrogen or phosphorus removal. Typical ABRs treating wastewater containing xenobiotics can achieve more than 90% COD removal at xenobiotic loading rates (XLRs) ranging from 0.004 to 0.5 kg COD m−3 d−1 and at HRTs ranging from 1 to 12 days depending on the temperature (see Table 5). It is worth noting that COD removal higher than 95% is also possible at HRTs of 24–48 h in the presence of emerging contaminants while energy is produced in the form of biogas and no sludge withdrawal takes place (apart from sampling). However, most studies were carried out with synthetic wastewater, but a few research studies with real wastewater demonstrated that the performance is also feasible with real wastewater. With typical xenobiotic concentrations expected in municipal wastewater (ng to μg L−1), the HRT in an ABR would be between 6 hours and 12 days at OLRs ranging from 0.3 to 1.3 kg COD m−3 d−1. This must be compared with the conventional waste activated sludge process that can achieve 99% BOD removal at OLRs of 0.3 to 1.6 kg BOD m−3 per day, at HRTs ranging from 3–6 hours and SRTs ranging from 3 to 15 days according to Metcalf and Eddy.125 The SRT in ABR studies is seldom reported but would be at least 10 times longer. When xenobiotic compounds are treated in the waste activated sludge process, the recommended minimum SRT is 5–50 days depending on the temperature, specific bacteria and compounds,125 and the compounds may not be fully degraded and a multiple barrier approach including reverse osmosis would be required if the water was to be recycled. Table 6 shows the typical OLR, HRT, SRT and xenobiotic loading rate (XLR) for various ABR studies treating municipal wastewater containing xenobiotic compounds. Based on this review, it can be concluded that the most successful process would include a sequence of units including an ABR followed by an aerobic tank (CSTR or aerobic baffled reactor), followed by an electrochemical oxidation unit, membrane unit, a wetland or a combination of these processes to polish the final effluent depending on the reuse and discharge options.
Table 6 Typical design parameters for the removal of xenobiotic compounds in ABRs treating municipal WW. NR = not reported, XLR = xenobiotic loading rate
Contaminant |
HRT |
SRT |
OLR (kg COD m−3 d−1) |
XLR (kg m−3 d−1) |
Ref. |
Perchlorate |
24 h |
NR |
0.398 |
0.5 kg m−3 d−1 |
103
|
p-Nitrophenol |
1–10.38 days |
400–600 days |
0.3 to 3.16 |
0.0077 kg m−3 d−1 |
105
|
Chlorophenols |
2–24 h |
— |
0.56 |
=0.2 kg m−3 d−1 |
106
|
Kemicetine |
12.8 days |
45 days |
=0.25 |
0.0038 kg m−3 d−1 |
100
|
Nitrobenzene/aniline |
24 h |
|
0.51 (to the ABR) |
0.08 kg m−3 d−1 (both ABR and wetland) |
107
|
0.72 (to the wetland) |
5.19 and 10.38 days (anaerobic) |
109–371 d (ABR) |
0.3 |
NB COD 0.1 kg m−3 d−1 (both anaerobic and aerobic) |
108
|
3.5 to 6.9 days (aerobic) |
129–391 (CSTR) |
|
Aniline 0.3 kg COD m−3 d−1 (ABR) |
8 Conclusions
This review summarizes the performance of ABRs and modified ABRs in treating low strength municipal wastewater and emerging or recalcitrant pollutants. In most of the ABR and modified ABR studies, high removal (>90%) of COD and emerging/recalcitrant pollutants was achieved. Various strategies were proposed to achieve concurrent carbon and nitrogen removal in ABRs based on recent insightful research. Accurate temperature control, biomass carriers, effluent recycling, filters, sponges, and submerged membranes are all relevant strategies to improve the performance of ABRs for energy production. Novel technologies such as membrane electrodes and in situ electrochemical oxidation have the potential to enhance further the biochemical processes in the ABR either by improving VFA degradation or by targeting recalcitrant organics. Strategies such as microaeration, membrane aerated biofilms and annamox have all shown great potential to achieve nitrogen removal in ABRs. The presence of an additional unit could enhance the performance of the ABR in treating various municipal wastewater. For instance, an aerobic CSTR could remove inhibitory/toxic compounds that cannot be degraded anaerobically, or by combining heterotrophic and autotrophic processes in a step-feeding ABR. The combination of the ABR with electrochemical oxidation provides new opportunities to remove pollutants and generate electricity at the same time. More efforts should be done to enhance the performance of ABRs using other combinations of chemical and electrochemical oxidation to enhance the production of methane gas while meeting effluent discharge criteria.
Author contributions
All authors contributed to the study conception and design. Material preparation and data collection were performed by Poh Lin Lau. The first draft of the manuscript was written by Poh Lin Lau and Antoine P. Trzcinski improved the first draft and commented on previous versions of the manuscript. All authors read and approved the final manuscript.
Conflicts of interest
The authors have no relevant financial or non-financial interests to disclose.
Acknowledgements
The authors declare that no funds, grants, or other support were received during the preparation of this manuscript.
References
- M. L. Sikosana, K. Sikhwivhilu, R. Moutloali and D. M. Madyira, Procedia Manuf., 2019, 35, 1018–1024 CrossRef.
- L. Seghezzo, G. Zeeman, J. B. van Lier, H−1. V. M. Hamelers and G. Lettinga, Bioresour. Technol., 1998, 65, 175–190 CrossRef CAS.
- J. B. Van Lier, A. Tilche, B. K. Ahring, H. Macarie, R. Moletta, M. Dohanyos, L. W. Hulshoff Pol, P. Lens and W. Verstraete, Water Sci. Technol., 2001, 43, 1–18 CrossRef CAS PubMed.
- S. Lyu, W. Chen, W. Zhang, Y. Fan and W. Jiao, J. Environ. Sci., 2016, 39, 86–96 CrossRef PubMed.
- S. A. Hameed, R. Riffat, B. Li, I. Naz, M. Badshah, S. Ahmed and N. Ali, J. Chem. Technol. Biotechnol., 2019, 94, 1816–1831 CrossRef CAS.
- D. Leicester, J. Amezaga and E. Heidrich, Renewable Sustainable Energy Rev., 2020, 133, 110279 CrossRef CAS.
- D. Pant, A. Singh, G. Van Bogaert, Y. A. Gallego, L. Diels and K. Vanbroekhoven, Renewable Sustainable Energy Rev., 2011, 15, 1305–1313 CrossRef CAS.
- D. Mamais, A. Noutsopoulos, C. Dimopoulou, A. Stasinakis and T. Lekkas, Water Sci. Technol., 2015, 71, 303–308 CrossRef CAS PubMed.
- S. Longo, B. M. d'Antoni, M. Bongards, A. Chaparro, A. Cronrath, F. Fatone, J. M. Lema, A. Mauricio-Iglesias, M. Soares and A. Hospido, Appl. Energy, 2016, 179, 1251–1268 CrossRef.
- H. Lin, W. Peng, M. Zhang, J. Chen, H. Hong and Y. Zhang, Desalination, 2013, 314, 169–188 CrossRef CAS.
- Y. An, Z. Wang, Z. Wu, D. Yang and Q. Zhou, Chem. Eng. J., 2009, 155, 709–715 CrossRef CAS.
- A. Plevri, D. Mamais and C. Noutsopoulos, Chemosphere, 2021, 275, 129961 CrossRef CAS PubMed.
- S. Nachaiyasit and D. C. Stuckey, J. Chem. Technol. Biotechnol., 1997, 69, 276–284 CrossRef CAS.
- P. L. McCarty and D. P. Smith, Environ. Sci. Technol., 1986, 20(12), 1200–1206 CrossRef CAS.
- K. M. Foxon, S. Pillay, T. Lalbahadur, N. Rodda, F. Holder and C. A. Buckley, Water SA, 2004, 30, 592–598 CAS.
- H. Feng, L. Hu, Q. Mahmood, C. Qiu, C. Fang and D. Shen, Int. Biodeterior. Biodegrad., 2008, 62, 232–238 CrossRef CAS.
- G. V. T. Gopala Krishna, P. Kumar and P. Kumar, Bioresour. Technol., 2008, 99, 8193–8200 CrossRef CAS PubMed.
- M. Farhadian, D. Duchez, C. Vachelard and C. Larroche, Water Res., 2008, 42, 325–1341 CrossRef PubMed.
- A. Elreedy, A. Tawfik, A. Enitan, S. Kumari and F. Bux, Energy Convers. Manage., 2016, 122, 119–130 CrossRef CAS.
- L. Ju, E. Augustine, J. Born and J. B. Holm-nielsen, Int. J. Hydrogen Energy, 2015, 40, 12154–12161 CrossRef.
-
M. T. Madigan, J. M. Martinko and J. Parker, Brock biology of microorganisms, Prentice Hall, Upper Saddle River, NJ, 1997, vol. 11 Search PubMed.
-
J. Malina, F. Joseph and F. G. Pohland, Design of anaerobic processes for the treatment of industrial and municipal wastes, Routledge, 2017 Search PubMed.
-
V. J. Inglezakis, S. G. Poulopoulos, E. Arkhangelsky, A. A. Zorpas and A. N. Menegaki, Chapter 3 - Aquatic Environment, in Environment and Development Basic Principles, Human Activities, and Environmental Implications, ed. S. G. Poulopoulos and V. J. Inglezakis, Elsevier, Amsterdam, 2016, pp. 137–212 Search PubMed.
- In Council Directive 91/271/EEC of 21 May 1991 concerning urban waste-water treatment, EC, Brussels, Belgium, 1991 Search PubMed.
- M. Preisner, E. Neverova-Dziopak and Z. Kowalewski, Environ. Manage., 2020, 66, 694–708 CrossRef PubMed.
- P. Wu, X. Zhang, Y. Wang, C. Wang, L. Ma, F. Wani Victor Jenario, W. Liu and L. Xu, Bioresour. Technol., 2021, 327, 124795 CrossRef CAS PubMed.
- Guidelines for irrigation water quality (G.N. No. 617 of 1999), Mauritius Government Gazette, 1999, FAOLEX No: LEX-FAOC053079, https://www.fao.org/faolex/results/details/en/c/LEX-FAOC053079/ Search PubMed.
- C. A. L. Chernicharo, J. B. Van Lier, A. Noyola and T. Bressani Ribeiro, Rev. Environ. Sci. Bio/Technol., 2015, 14, 649–679 CrossRef CAS.
- E. J. Bowen, J. Dolfing, R. J. Davenport, F. L. Read and T. P. Curtis, Water Sci. Technol., 2014, 69, 1004–1013 CrossRef CAS PubMed.
- A. Fernández del Castillo, M. V. Garibay, C. Senés-Guerrero, D. A. Orozco-Nunnelly, J. de Anda and M. S. Gradilla-Hernández, J. Cleaner Prod., 2022, 371, 133428 CrossRef.
- C. Keating, D. Hughes, T. Mahony, D. Cysneiros, U. Z. Ijaz, C. J. Smith and V. O'Flaherty, FEMS Microbiol. Ecol., 2018, 94, fiy095 CrossRef CAS PubMed.
- E. Petropoulos, J. Dolfing, R. J. Davenport, E. J. Bowen and T. P. Curtis, Water Res., 2017, 112, 100–109 CrossRef CAS PubMed.
- E. S. León, E. L. Vargas-Machuca, J. A. P. Corona, Z. Arbib, F. Rogalla and M. F. Boizán, J. Cleaner Prod., 2018, 198, 931–939 CrossRef.
- R. M. McKeown, D. Hughes, G. Collins, T. Mahony and V. O'Flaherty, Curr. Opin. Biotechnol., 2012, 23, 444–451 CrossRef CAS PubMed.
- Metals and Alloys - Densities, https://www.engineeringtoolbox.com/metal-alloys-densities-d_50.html.
- R. Renuka, S. M. Mohan, B. Sowmiya and S. A. Raj, Ecol. Eng., 2016, 97, 1–12 CrossRef.
- S. Y. Bodkhe, J. Environ. Manage., 2009, 90, 2488–2493 CrossRef CAS PubMed.
-
L. Metcalf, H. P. Eddy and G. Tchobanoglous, Wastewater engineering: treatment, disposal, and reuse, McGraw-Hill, 4th edn, 1991 Search PubMed.
- A. Tawfik, F. El-Gohary, A. Ohashi and H. Harada, Water Sci. Technol., 2008, 58, 185–194 CrossRef CAS PubMed.
-
D. W. Westcot, Quality control of wastewater for irrigated crop production (Water reports - 10), Food and Agriculture Organization of the United Nations, Rome, 1997, ISSN 1020-1203, accessible from https://www.fao.org/3/w5367e/w5367e06.htm Search PubMed.
- M. Aqaneghad and G. Moussavi, Sustainable Environ. Res., 2016, 26, 203–208 CrossRef CAS.
- G. Moussavi, R. Khosravi and M. Farzadkia, Desalination, 2011, 278, 288–294 CrossRef CAS.
- M. J. Hahn and L. A. Figueroa, Water Res., 2015, 87, 494–502 CrossRef CAS PubMed.
- H. Yu and G. K. Anderson, Resour., Conserv. Recycl., 1996, 17, 259–271 CrossRef.
- I. Bodík, K. Kratochvíl, E. Gašpariková and M. Hutan, Bioresour. Technol., 2003, 86, 79–84 CrossRef PubMed.
- H. Zheng, H. X. Chai, L. Zhao, Y. Liao, X. Cao, L. Feng and F. Ji, Environ. Res., 2023, 223, 115475 CrossRef CAS PubMed.
- A. Gholipour and A. I. Stefanakis, Ecol. Eng., 2021, 170, 106360 CrossRef.
- J. Liu, N. Zang, L. Gao, X. Liu, H. Tian, P. Yue and T. Li, Biochem. Eng. J., 2022, 183, 108455 CrossRef CAS.
- E. Ahmadi, S. Yousefzadeh, A. Mokammel, M. Miri, M. Ansari, H. Arfaeinia, M. Y. Badi, H. R. Ghaffari, S. Rezaei and A. H. Mahvi, Renewable Sustainable Energy Rev., 2020, 121, 109674 CrossRef CAS.
- H. Sung, E. Katsou, E. Statiris and L. Anguilano, Environ. Technol., 2018, 1–6 Search PubMed.
- E. Ahmadi, S. Yousefzadeh, A. Mokammel, M. Miri, M. Ansari, H. Arfaeinia, M. Y. Badi, H. R. Ghaffari, S. Rezaei and A. H. Mahvi, Renewable Sustainable Energy Rev., 2020, 121, 109674 CrossRef CAS.
- A. Khan, S. J. Khan, W. Miran, W. Q. Zaman, A. Aslam and H. M. A. Shahzad, Membranes, 2023, 13(1), 79 CrossRef CAS PubMed.
- H. D. Ryu, D. Kim, K. Y. Kim and S. I. Lee, Environ. Eng. Sci., 2008, 25, 601–614 CrossRef CAS.
- C. Ratanatamskul, C. Charoenphol and K. Yamamoto, Desalin. Water Treat., 2015, 54, 908–915 CrossRef CAS.
- J. A. Álvarez, E. Armstrong, M. Gómez and M. Soto, Bioresour. Technol., 2008, 99, 7051–7062 CrossRef PubMed.
-
Wef, Design of municipal wastewater treatment plants, McGraw-Hill, 2009 Search PubMed.
- Z. Zhang, J. Li, X. Hao, Z. Gu and S. Xia, Environ. Technol., 2019, 40, 2337–2344 CrossRef CAS PubMed.
- A. J. Stams, D. Z. Sousa, R. Kleerebezem and C. M. Plugge, Water Sci. Technol., 2012, 66, 352–362 CrossRef CAS PubMed.
- T. Wang, G. Zhu, C. Li, M. Zhou, R. Wang and J. Li, Water Res., 2020, 170, 115329 CrossRef CAS PubMed.
- W. M. K. R. T. W. Bandara, H. Satoh, M. Sasakawa, Y. Nakahara, M. Takahashi and S. Okabe, Water Res., 2011, 45, 3533–3540 CrossRef CAS PubMed.
- J. Cookney, E. Cartmell, B. Jefferson and E. J. McAdam, Water Sci. Technol., 2012, 65(4), 604–610 CrossRef CAS PubMed.
- T. Liu, S. Hu, Z. Yuan and J. Guo, Water Res., 2023, 242, 120194 CrossRef CAS PubMed.
- A. P. Trzcinski and D. C. Stuckey, Water Res., 2010, 44, 671–680 CrossRef CAS PubMed.
- A. Grobicki and D. C. Stuckey, Water Res., 1992, 26, 371–378 CrossRef CAS.
- B. C. Crone, J. L. Garland, G. A. Sorial and L. M. Vane, Water Res., 2016, 104, 520–531 CrossRef CAS PubMed.
- P. Wu, X. Ji, X. Song and Y. Shen, Chem. Eng. J., 2013, 232, 273–279 CrossRef CAS.
- P. Wu, L. Xu, J. Wang, Z. Huang, J. Zhang and Y. Shen, Appl. Biochem. Biotechnol., 2015, 177, 1003–1012 CrossRef CAS PubMed.
- N. Hendrasarie and M. N. Trilta, IOP Conf. Ser. Earth Environ. Sci., 2019, 245, 012017 CrossRef.
- W. Yuguang, Z. Xingxing, W. Chaochao, X. Yunkang, W. Yao, Z. Cheng, W. Yiling, W. Peng and X. Lezhong, Huagong Jinzhan, 2022, 41, 2191–2201 Search PubMed.
- X. Zhou, M. Arslan, Z. Liu, D. Li, H. Xi, Y. Feng, S. Li, J. Wei, X. Rong, Z. Liang, X. Wang, Z. Wu and M. Gamal El-Din, Bioresour. Technol., 2022, 359, 127346 CrossRef CAS PubMed.
- X. Zha, J. Ma, P. Tsapekos and X. Lu, J. Environ. Manage., 2019, 251, 109599 CrossRef CAS PubMed.
- J. A. Baeza, D. Gabriel and J. Lafuente, Process Biochem., 2004, 39, 1615–1624 CrossRef CAS.
- S. Hu, F. Yang, S. Liu and L. Yu, Water Res., 2009, 43, 381–388 CrossRef CAS PubMed.
- M. S. Strous, M. Kuenen and J. G. Jetten, Appl. Environ. Microbiol., 1999, 65, 3248–3250 CrossRef CAS PubMed.
- A. A. Van de Graaf, P. de Bruijn, L. A. Robertson, M. S. Jetten and J. G. Kuenen, Appl. Environ. Microbiol., 1995, 61(4), 1246–1251 CrossRef CAS PubMed.
- S. Lackner, E. M. Gilbert, S. E. Vlaeminck, A. Joss, H. Horn and M. C. M. van Loosdrecht, Water Res., 2014, 55, 292–303 CrossRef CAS PubMed.
- W. Wang, H. Xie, H. Wang, H. Xue, J. Wang, M. Zhou, X. Dai and Y. Wang, Water Res., 2020, 184, 116197 CrossRef CAS PubMed.
- E. Rivera, N. Mladenov, L. Astete Vasquez, G. McKenzie and V. Gonzalez, Bioresour. Technol., 2022, 364, 128047 CrossRef CAS PubMed.
- A. Talan, R. D. Tyagi and P. Drogui, Environ. Technol. Innovation, 2021, 23, 101553 CrossRef CAS.
- R.-C. Jin, G.-F. Yang, J.-J. Yu and P. Zheng, Chem. Eng. J., 2012, 197, 67–79 CrossRef CAS.
- M. Strous, J. J. Heijnen, J. G. Kuenen and M. S. M. Jetten, Appl. Microbiol. Biotechnol., 1998, 50, 589–596 CrossRef CAS.
- K. Isaka, Y. Date, Y. Kimura, T. Sumino and S. Tsuneda, FEMS Microbiol. Lett., 2008, 282, 32–38 CrossRef CAS PubMed.
- P. Wu, Y. Chen, X. Ji, W. Liu, G. Lv, Y. Shen and Q. Zhou, Bioresour. Technol., 2018, 267, 696–703 CrossRef CAS PubMed.
- C. Wang, S. Liu, X. Xu, C. Zhang, D. Wang and F. Yang, Chemosphere, 2018, 203, 457–466 CrossRef CAS PubMed.
- J. Ji, Y. Peng, B. Wang and S. Wang, Bioresour. Technol., 2017, 224, 140–146 CrossRef CAS PubMed.
- X. Wang, J. Zhao, D. Yu, G. Chen, S. Du, J. Zhen and M. Yuan, Chem. Eng. J., 2019, 355, 560–571 CrossRef CAS.
- K. F. Ettwig, M. K. Butler, D. Le Paslier, E. Pelletier, S. Mangenot, M. M. M. Kuypers, F. Schreiber, B. E. Dutilh, J. Zedelius, D. de Beer, J. Gloerich, H. J. C. T. Wessels, T. van Alen, F. Luesken, M. L. Wu, K. T. van de Pas-Schoonen, H. J. M. Op den Camp, E. M. Janssen-Megens, K.-J. Francoijs, H. Stunnenberg, J. Weissenbach, M. S. M. Jetten and M. Strous, Nature, 2010, 464, 543–548 CrossRef CAS PubMed.
- M. F. Haroon, S. Hu, Y. Shi, M. Imelfort, J. Keller, P. Hugenholtz, Z. Yuan and G. W. Tyson, Nature, 2013, 500, 567–570 CrossRef CAS PubMed.
- A. A. Raghoebarsing, A. Pol, K. T. van de Pas-Schoonen, A. J. P. Smolders, K. F. Ettwig, W. I. C. Rijpstra, S. Schouten, J. S. S. Damsté, H. J. M. Op den Camp, M. S. M. Jetten and M. Strous, Nature, 2006, 440, 918–921 CrossRef CAS PubMed.
- S. Hu, F. Yang, S. Liu and L. Yu, Water Res., 2009, 43, 381–388 CrossRef CAS PubMed.
- Y. Peng and S. Ge, Bioresour. Technol., 2011, 102, 6405–6413 CrossRef CAS PubMed.
- C. Zhang, G. Zhang, F. Wu and T. Zhou, Sustainability, 2020, 12(6), 2325 CrossRef CAS.
- X. Xu, L. Qiu, C. Wang and F. Yang, Bioresour. Technol., 2019, 284, 80–89 CrossRef CAS PubMed.
- W. Zeng, X. Bai, Y. Guo, N. Li and Y. Peng, Enzyme Microb. Technol., 2017, 105, 1–8 CrossRef CAS PubMed.
- Y. Zhu, M. Wu, N. Gao, W. Chu and S. Wang, Chemosphere, 2016, 165, 134–143 CrossRef CAS PubMed.
- V. Uberoi and S. K. Bhattacharya, Water Environ. Res., 1997, 69, 146–156 CrossRef CAS.
- R. M. Melgoza and G. Buitrón, Water Sci. Technol., 2001, 44, 151–157 CrossRef CAS PubMed.
- A. Di Paola, V. Augugliaro, L. Palmisano, G. Pantaleo and E. Savinov, J. Photochem. Photobiol., A, 2003, 155, 207–214 CrossRef CAS.
- X. Pan, N. Lv, C. Li, J. Ning, T. Wang, R. Wang, M. Zhou and G. Zhu, Chem. Eng. J., 2019, 359, 662–671 CrossRef CAS.
- D. T. Sponza and P. Demirden, J. Hazard. Mater., 2010, 176, 64–75 CrossRef CAS PubMed.
- A. Lateef, World J. Microbiol. Biotechnol., 2004, 20, 167–171 CrossRef CAS.
- S. Gartiser, E. Urich, R. Alexy and K. Kümmerer, Chemosphere, 2007, 67, 604–613 CrossRef CAS PubMed.
- K. Li, J. Guo, H. Li, Y. Han, Z. Chen, Y. Song, Y. Xing and C. Zhang, Bioresour. Technol., 2019, 279, 297–306 CrossRef CAS PubMed.
- Ö. S. Kuşçu and D. T. Sponza, Enzyme Microb. Technol., 2005, 36, 888–895 CrossRef.
- Ö. S. Kuşçu and D. T. Sponza, Process Biochem., 2006, 41, 1484–1492 CrossRef.
- G. Moussavi, S. Ghodrati and A. Mohseni-Bandpei, J. Biotechnol., 2014, 184, 111–117 CrossRef CAS PubMed.
- Y. Lin, J. Yin, J. Wang and W. Tian, Bioresour. Technol., 2012, 118, 128–135 CrossRef CAS PubMed.
- Ö. S. Kuscu and D. T. Sponza, Bioresour. Technol., 2009, 100, 2162–2170 CrossRef PubMed.
- C. Su, Y. Lu, Q. Deng, S. Chen, G. Pang, W. Chen, M. Chen and Z. Huang, Ecotoxicol. Environ. Saf., 2019, 177, 39–46 CrossRef CAS PubMed.
- M. Vega, R. Nerenberg and I. T. Vargas, Environ. Res., 2018, 164, 316–326 CrossRef CAS PubMed.
- F. Cao, J. Jaunat, N. Sturchio, B. Cancès, X. Morvan, A. Devos, V. Barbin and P. Ollivier, Sci. Total Environ., 2019, 661, 737–749 CrossRef CAS PubMed.
- J. K. Choe, M. H. Mehnert, J. S. Guest, T. J. Strathmann and C. J. Werth, Environ. Sci. Technol., 2013, 47, 4644–4652 CrossRef CAS PubMed.
- Z. I. Bhatti, H. Toda and K. Furukawa, Water Res., 2002, 36, 1135–1142 CrossRef CAS PubMed.
- K. Karim and S. K. Gupta, Water Res., 2003, 37, 2953–2959 CrossRef CAS PubMed.
- B. A. Donlon, E. Razo-Flores, G. Lettinga and J. A. Field, Biotechnol. Bioeng., 1996, 51, 439–449 CrossRef CAS PubMed.
- S. K. Basu, J. A. Oleszkiewicz and R. Sparling, Water Res., 1996, 30, 315–322 CrossRef CAS.
- B. Erol Nalbur and U. Alkan, Int. Biodeterior. Biodegrad., 2007, 60, 178–188 CrossRef.
- B. N. N. S. Halling-Sørensen, S. N. Nielsen, P. F. Lanzky, F. Ingerslev, S. E. Lützhøft and H. H. Jørgensen, Chemosphere, 1998, 36, 357–393 CrossRef PubMed.
- X. S. Miao, F. Bishay, M. Chen and C. D. Metcalfe, Environ. Sci. Technol., 2004, 38, 3533–3541 CrossRef CAS PubMed.
- E. U. Cokgor, I. A. Alaton, O. Karahan, S. Dogruel and D. Orhon, J. Hazard. Mater., 2004, 116, 159–166 CrossRef CAS PubMed.
- E. U. Cokgor, O. Karahan and D. Orhon, J. Hazard. Mater., 2008, 156, 292–299 CrossRef CAS PubMed.
- W.-S. Chen and Y.-C. Liu, Heliyon, 2021, 7, e06984 CrossRef CAS PubMed.
- Y. Mu, H.-Q. Yu, J.-C. Zheng, S.-J. Zhang and G.-P. Sheng, Chemosphere, 2004, 54, 789–794 CrossRef CAS PubMed.
- M. A. Aziz, W. J. Ng and X. J. Zhou, Bioresour. Technol., 1994, 48, 37–42 CrossRef CAS.
-
Metcalf & Eddy, Wastewater Engineering: Treatment and Resource Recovery, McGraw-Hill, New York, 5th edn, 2014 Search PubMed.
|
This journal is © The Royal Society of Chemistry 2024 |