DOI:
10.1039/D2TA09715J
(Review Article)
J. Mater. Chem. A, 2023,
11, 6646-6658
Impacts of host–guest assembly on the photophysical and photocatalytic properties of heterogenized molecular photosensitizer and catalysts†
Received
13th December 2022
, Accepted 24th February 2023
First published on 28th February 2023
Abstract
This review focuses on taking porous matrixes as ‘molecular containers’ to tune and regulate the photophysical and photocatalytic properties of molecular photosensitizers and catalysts for application in solar energy conversion. The host–guest assemblies feature the physical separation of catalytic sites as well as a precise preorganization of photosensitizer and catalytic species in close spatial proximity. The host–guest effects on the photophysical processes, such as non-radiative or radiative energy dissipation and photoinduced energy transfer or electron transfer, and on the photocatalytic processes, such as regulating mass transfer, diverting the reaction pathway with preferable intermediate species, and modulating reaction dynamics, are demonstrated. The geometrical confinement of molecular catalysts and photosensitizers to construct the enzyme-like microenvironments provides a promising avenue for mimicking sophisticated natural photosynthesis.
1. Introduction
The high-efficiency utilization and transformation of renewable energy are currently attracting unprecedented attention given the need for sustainable development for the future of mankind. Natural photosynthesis is a prototype for transforming renewable solar energy into chemical energy based on PSI and PSII with fundamental reaction cascades that are carried out by precisely organized molecular components in a smart protein matrix. Taking nature as an example, artificial photosynthesis manipulates light-harvesting antennae and redox-active centers to fulfill the conversion of small molecules of H2O, CO2, and NH3etc. into solar fuels. The atom-efficient molecular complex catalysts, paired with molecular chromophores, are promising candidates in artificial photosynthesis with the advantages of outstanding intrinsic activities, definite active sites, as well as fundamental insights into the catalytic mechanism.1 However, the vulnerability of homogeneous molecular systems under reaction conditions has to be circumvented for practical application in solar fuel production.
An emerging strategy of host–guest assembly, the heterogenization of molecular systems into porous architectures as sterically isolated sites, could maintain the intrinsic activities of molecular systems while promoting their sustainability and recyclability. Significantly, the integration of molecular guests with porous hosts could engender a microenvironment substantially different from that corresponding to the homogeneous bulk solution, such as hydrophobicity/hydrophilicity, electrostatics, numerous apertures and constrained void spaces. The host–guest assembly provides an unexpected opportunity to modulate the photophysical properties of bound-guest species, dictate the diffusion of the substrate and ions, and stabilize higher-energy transition states or radical intermediates,2 reminiscent of the procedure for natural enzymes.3–11 The numerous porous architectures could serve as host containers to accommodate molecular guests of photosensitizers and catalysts, such as periodic mesoporous silica (PMS),12 metal–organic frameworks (MOFs), and covalent organic frameworks (COFs) etc. The modular nature of MOFs, constructed by organic-ligand backbone linking inorganic-node building blocks, affords a versatile platform to hierarchically organize light-harvesting antennae and catalytic centers with controllable positions, mutual distances, and relative orientations,3,4,13 which could optimize the energy migration and electron transfer required for efficient artificial photosynthesis.14–17
The host–guest assemblies of molecular photosensitizers and catalysts with a variety of MOF hosts have been well reviewed for photocatalysis.3,10,11,13–15,18–20 However, the host–guest effects in these heterogenous host–guest assemblies have plenty of scope for fundamental research, particularly in comparison with the relatively mature field of homogeneous supramolecular coordination self-assembly.21,22 In this contribution, we will specifically focus on the host–guest effects on photophysical and photocatalytic processes during artificial photosynthesis for solar fuel production. The design and classification of host–guest assemblies are first described in terms of the spatial population of guest species within MOF hosts, together with a brief depiction of the assembling strategy. Next, the host–guest effects on photophysical processes are discussed separately based on photo-active MOFs and photo-inert MOFs. Then, the host–guest effects on photocatalytic reactions are depicted from two aspects of spatial confinement and the resultant mass transfer. Finally, efforts to understand the intertwined host–guest effects in the future are suggested.
2. The design, classification and synthesis of the heterogenous host–guest assembly
Benefiting from the development of inorganic chemistry and synthetic chemistry, various molecular complexes have sprung up for artificial photoconversion, such as a simple molecule with a single light-harvesting/redox-active function or double functions, and a photochemical molecular device (PMD) with the covalent linkage of the photosensitizing center and catalytic unit as a single molecular entity.23,24 These guest molecules can be incorporated into the MOF host as inorganic-node building blocks, organic-linker backbones, porous inclusion or scaffold defects via crystal engineering.25–28 Therefore, the versatility of the host–guest assembly can be designed with the types and positions of guest molecules.
Given the initial light harvesting by molecular photosensitizers to drive a desired excited state redox reaction, the photo-response of the functional-MOF host was determined to classify the host–guest assembly into photo-active hosts and photo-inert hosts, as shown in Fig. 1. As the photo-active host (top panel), the MOF is constructed by photo-functional organic linkers, where engineered molecular photosensitizers are installed at organic linkers via modifying, grafting or exchanging,23 or light-harvesting functions are carried by organic linkers intrinsically, such as porphyrin-derived ligands and 2-aminoterephthalic acid ligands.29,30 As for the photo-inert host (bottom panel), the MOF is an “innocent” container, where molecular photosensitizers are positioned in the MOF cavity or stuck in MOF scaffolds as structural imperfections.
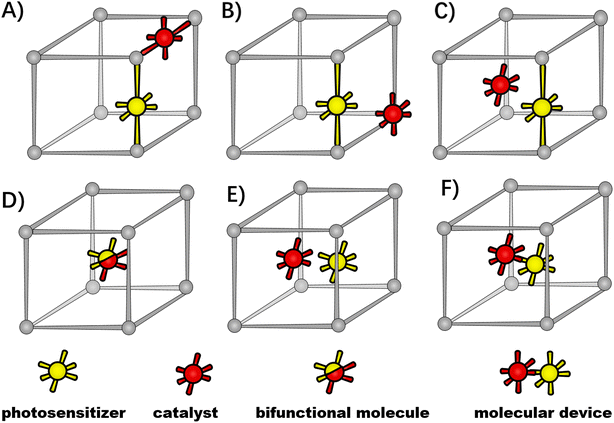 |
| Fig. 1 The design and classification of host–guest assemblies discussed in this review. (A–C) Photo-active MOFs with chromophores anchored on organic linkers and catalytic sites, respectively, populating the organic linker, vertices/nodes and porous cavity. (D–F) Photo-inert MOFs encapsulated with a single molecule bearing photo-responsive and redox-active dual roles, double molecules acting as a chromophore and a catalyst separately, and a photochemical molecular device with the covalent linkage of the photosensitizing center and catalytic unit. The chromophore and catalyst are highlighted in yellow and red, respectively. | |
For the photo-active hosts, molecular redox-active catalysts could be incorporated into the MOFs' functional-organic linkers, metal vertices or porous cavities to form host–guest assemblies.26–28,30,31 So far, various molecular photosensitizers and catalysts have been integrated into MOFs as functional-organic linkers through the routine synthetic strategies of the mixed-ligand multivariate approach, post-synthetic exchange and sequent post-synthetic metalation (Fig. 2A),5,29,31–34 such as Ru and Ir-based molecular photosensitizers,33,35–38 Ru-based water oxidation catalysts,39–41 Pt-based H2 evolution catalysts,33,42 and Mn, Re and Ir-based CO2 reductive catalysts,36,43etc. In these cases, molecular catalysts are installed into functionalized linkers, where the structural tailoring of guest molecules is necessary to fit the inherent organic linker length. As a complement, a molecular catalyst could be built around the MOF node itself. A rare example is Lin's work of directly incorporating the redox-active Ru2 centers as metal vertices of photoactive porphyrin MOFs.30 Alternatively, molecular catalysts could be accommodated in the MOFs' cavity with a “ship-in-a-bottle” strategy,44 where the molecular dimensions are matched with the inner spaces of MOFs' cavities. For example, Co-dioxime-diimine was trapped in the cavities of a photo-active NH2-MIL-125(Ti) for light-driven H2 production,29 and a Ru-based catalyst was covalently incorporated into the cages of MIL-101(Cr) through the “amide bond” as bridges.45 An active catalyst of ruthenium complex with a dimension larger than the aperture size of a MOF host was encapsulated in the pores of zirconium-based UiO-66 for the hydrogenation of CO2, taking advantage of aperture-opening events resulting from dissociative linker exchange (Fig. 2B).46
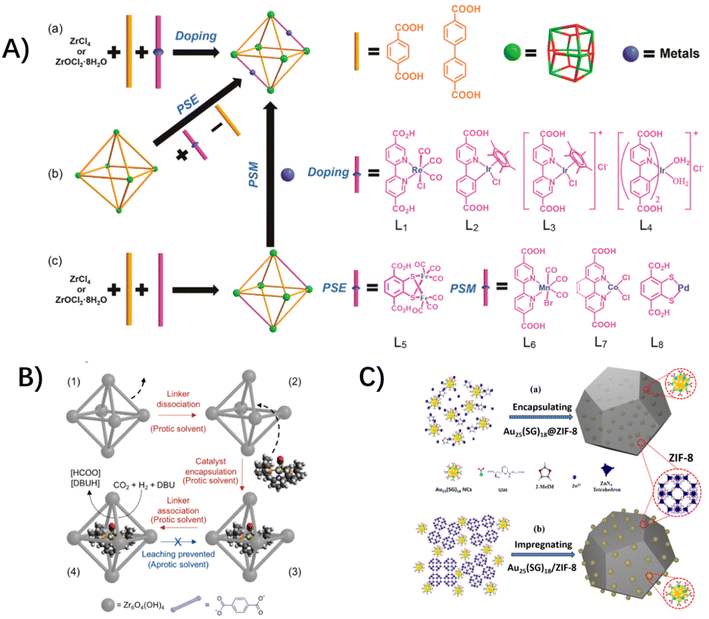 |
| Fig. 2 (A) The synthetic strategies for the (a) mixed-ligand multivariate approach (doping), (b) post-synthetic exchange (PSE) and (c) post-synthetic metalation (PSM). Reprinted with permission from ref. 34. Copyright 2017 American Chemical Society. (B) A “ship-in-a-bottle” strategy of aperture-opening encapsulation. Reprinted with permission from ref. 46. Copyright 2018 American Chemical Society. (C) A “bottle-around-a-ship” strategy of coordination assisted self-assembly. Reprinted from ref. 47 with permission from Wiley-VCH. | |
The preceding host–guest assemblies rely upon the photo-active hosts with functionalized organic linkers for molecular catalyst installation. Another class of photo-inert hosts serve as the physical scaffolds to isolate the light-harvesting and redox-active molecules with a confined environment around bound guest species. Under these conditions, we developed a universal “bottle-around-a-ship” coordination-assisted self-assembly to integrate molecular guests into MOFs (Fig. 2C),47–49 where the incarcerated molecular complexes coordinate with MOF nodes or organic linkers improperly before the building of the MOFs, concomitantly generating the structural imperfections and defects around these mismatch-sites. The unique superiority of this strategy is to break through the limitations of the structure and dimensions of molecular guests. In our previous works, a Ru(bda)L2-based water oxidative catalyst, an atomically precise Au25(SG)18 nanocluster, and a PMD of an octahedral metal–organic cage (MOC) of [Pd6(RuL3)8]28+ (MOC-16) comprising multiple photosensitive ruthenium-units and catalytic palladium-units,50,51 were successfully encapsulated in the MOF scaffolds of ZIF-8 and/or UiO-66 due to their high tolerances to structural defects.48,49,51 In our ensuing unpublished works, a Re-based molecular catalyst and a couple of Re and Ru molecular complexes have been incorporated into MOF hosts, and the relevant work is underway. The extending of guest molecular types provides an unprecedented opportunity to mimic natural photosynthesis.
3. The photophysical properties of the host–guest assembly
Artificial photoconversion begins with the light-harvesting of chromophores to achieve a high-energy excitation state, followed by a series of excited state dynamic processes in an ultrafast timescale, concomitantly involving radiative and non-radiative relaxation electron and energy transfer. In these steps, the electron and energy transfer steps, either with or without the participation of electron donors or acceptors, induce the redox activity of catalytic centers to trigger the consequent catalytic conversion along with the breaking and making of chemical bonds, obeying the macro-kinetic law. In a homogeneous molecular system, the photophysical processes of metal–organic complexes, such as polypyridyl Ru(II) and Ir(II) chromophores, etc., could be adjusted easily based on the universal regulations of coordination engineering and synthesis chemistry. In a counterpart of host–guest assembly, the photophysical properties of heterogenized chromophores within MOFs are inevitably influenced by the host–guest effects, which could be further reflected in the subsequent catalytic performance. Understanding the mechanistic aspects of light-initiated dynamics between bound chromophores and the surrounding array of the host, catalytic sites or electrons and holes, is pivotal for artificial photosynthesis.
In this section, the host–guest effects are discussed separately based on photo-active MOFs and photo-inert MOFs. For the photo-active MOFs with inherent or acquired photo-active ligands, the chromophores are covalently assembled into MOF hosts adopting precise spatial organization as part of MOF crystals, thereby, the photoinduced electrons and electron transfer involve the quasi-intramolecular host–guest transitions, such as ligands to ligands, ligands to nodes, or ligands to inclusive guests. In contrast, the photo-inert MOFs serve as molecular containers to provide a microenvironment different from the homogeneous bulk solution, therefore, the photophysics of guest chromophores is more sensitive to the polarity and the steric, geometric and torsional constraints, which are induced by the non-covalent interactions of hydrogen bonding, π–π stacking, electrostatic interactions, van der Waals forces, etc.
3.1 The photo-induced energy transfer or electron transfer with photo-active MOFs
3.1.1 Photo-induced energy transfer (EnT).
The photo-induced energy transfer (EnT) within organic solids typically takes place via the movement of localized excitation or excitons, i.e., tightly bound electron–hole pairs,52 which can be described using Förster and Dexter models as long- and short-range processes, respectively. Organizing chromophores into the extended architectures with different MOF functionalities could manipulate EnT pathways, such as ligand-to-ligand, metal-to-metal, metal-to-ligand (or ligand-to-metal), and guest-to-MOF,13 in terms of the spatial separation and relative orientation of chromophores.
For photo-active MOFs with light-harvesting organic linkers, the directional and efficient long-range energy transport has been studied in two model systems incorporating ruthenium-based and porphyrin-based units.19,53,54 The rapid intra-MOF energy migration over long distances dominated the photophysical process of the Ru(II) (2,2′-bipyridine)3-derived Zr-MOF of UiO-67, and the facile intracrystal site-to-site energy migration appeared in the isomorphous UiO-67 with mixed Ru(II)/Os(II) (2,2′-bipyridine)3-derived building blocks.54–56 In photo-active MOFs composed of Zn(II) porphyrin struts, the photogenerated exciton migrated directionally over a distance of up to ∼45 porphyrin struts within its lifetime.57
The intended destination of exciton migration is productive dissociation into electrons and holes in a specific site to take part in solar fuel production, although it has yet to be experimentally explored. In photo-active MOFs composed of the 1,1,2,2-tetrakis(4-(pyridin-4-yl)phenyl)-ethene (tppe) ligand (Fig. 3A), the efficient host-to-guest energy transfer from tppe ligands to inclusion guests of Rho6G, ended up as the mobile exciton on a dye molecule within its emission lifetime.58 In two MOFs constructed from truxene-derived ligands and zinc nodes, following the exciton migration via ligand-to-ligand energy exchange, a coumarin guest within the MOF cavity acted as an energy trap to quench the excitation via host-to-guest energy transfer.59 In this example, exciton migration “through-space” jumping over a longer distance, rather than routine step-by-step random hopping, was proposed to estimate the EnT efficiency (Fig. 3B).59
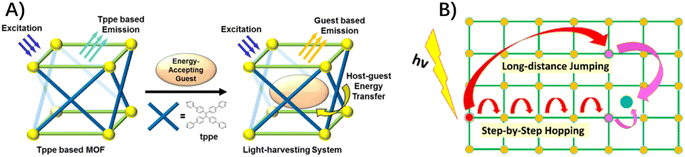 |
| Fig. 3 (A) Energy transformation from a tppe-based MOF to a spatially confined energy-accepting guest. Reprinted with permission from ref. 58. Copyright 2018 American Chemical Society. (B) Two distinct pathways in exciton migration on a network of chromophores with step-by-step nearest neighbour hopping and long-distance jumping. Reprinted from ref. 59 with permission from American Chemical Society. | |
3.1.2 Photo-induced electron transfer (ET).
The photo-induced electron transfer (ET) between chromophores and catalytic centers could be utilized for the generation of reactive species in photo-active MOFs. The electron transport in frameworks could be electron hopping or band transport, related to the position of photo-active chromophores and redox-active sites. Both types of transport can either occur through space (such as through π–π interactions) or through bonds (by pairing electron donors and acceptors).11 So far, a wide range of host–guest assemblies have been explored to utilize the photo-induced electron transfer between host and guest molecules for H2 evolution and CO2 reduction.29,33,35 Herein, some typical examples are given, classified by ET pathways of ligand-to-ligand, ligand-to-node, and ligand-to-inclusion.
3.1.2.1 Ligand to ligand.
In a photo-functional UiO-67 with [Ru(dcbpy)(bpy)2]2+ (bpy: bipyridine, dcbpy: dicarboxylate bipyridine) chromophores as light-absorbing linkers, the catalytic moieties of Pt(dcbpy)Cl2 and Co(dcbpy)Cl2 were respectively incorporated as a second type of functionalized linker.60,61 The possibility that charge transfer from the photoexcited Ru moiety to the catalytic Pt and Co moiety occurred, which accounted for the photocatalytic proton reduction. In another case, a light-harvesting moiety of [Ru(bpy)2(dcbpy)]2+ and a catalytic complex of [Re(CO)3(dcbpy)Cl]+ were co-installed into MOF-253, constructed from Al–O clusters and dicarboxylate bipyridine.62 The facilitated photocatalytic CO2 reduction in Ru–MOF-253–Re, relative to MOF-253–Re, supported the presence of a charge transfer between the light-harvesting moiety and the catalytic moiety (Fig. 4A). This efficient charge transfer was also highlighted when grafting a Ru(bpy)3-based photosensitizer and a Re(CO)3Cl-based catalyst into MOF-808.63 Notably, a highly efficient self-healing system was developed in a bipyridine-embedded UiO-type MOF with the arrangement of diimine sites closely and densely surrounding the H2-evolving catalyst of PtII(L)Cl2 and the photosensitizer of IrIII(ppy)2(L) (L: 2,2′-bipyridine-5,5′-dicarboxylate, ppy: phenylpyridine) (Fig. 4B),33 which presented persistent H2 evolution for 6.5 days at least, in contrast to the homogeneous counterpart for 7.5 h.
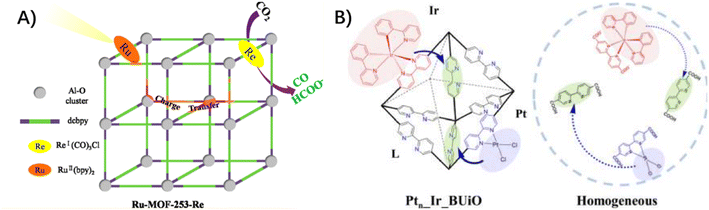 |
| Fig. 4 (A) The proposed ligand-to-ligand electron transfer from a light-harvesting moiety of [Ru(bpy)2(dcbpy)]2+ to a catalytic complex of [Re(CO)3(dcbpy)Cl]+. Reprinted with permission from ref. 62. Copyright 2018 American Chemical Society. (B) Schematic operation principle of a self-healing MOF (Ptn_Ir_BUiO) and the corresponding homogeneous system. Reprinted with permission from ref. 33. Copyright 2016 American Chemical Society. | |
3.1.2.2 Ligand to node.
In photo-active MOFs composed of porphyrin-derived tetracarboxylate ligands and catalytic centers of Ru2 secondary building units (SBUs), the proximity of Ru2 SBUs to porphyrin ligands facilitated multielectron transfer from excited porphyrins to Ru2 SBUs, which enabled an efficient visible-light-driven hydrogen evolution reaction (Fig. 5A).30 Similarly, with visible-light-excitation of the Eu–Ru(phen)3–MOF, the electron injection from Ru(phen)3-derived ligands into the nodes of dinuclear Eu(III)2 clusters was confirmed by fluorescence quenching to generate the dinuclear Eu(II)2 active sites, which drove the selective reduction of CO2 to formate in a two-electron process (Fig. 5B).64
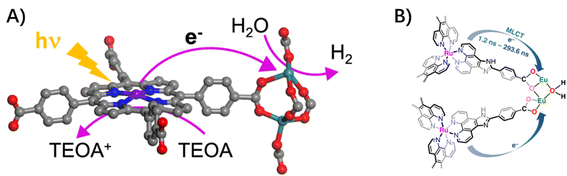 |
| Fig. 5 (A) The ligand-to-node multielectron transfer from excited porphyrin-linkers to Ru2 SBUs. Reprinted with permission from ref. 30. Copyright 2018 American Chemical Society. (B) Schematic light-induced electron transfer from Ru-metalloligand photocenter to catalytic Eu2 oxo-cluster center in Eu–Ru(phen)3–MOF. Reprinted from ref. 64. | |
3.1.2.3 Ligand to inclusion.
The proton reductive center of [Fe2S2] was incorporated into a Zn(II) porphyrin-derived zirconium-MOF through post-binding to the porphyrin zinc center. The electron transfer between the photo-active porphyrin linkers and the reactive [Fe2S2] center was facilitated due to their close vicinity and chemical bonding nature.31 An example related to multi-electron transfer involves the negatively charged polyoxometalate (POMs) being accommodated in the cavity of cationic MOFs yielding a di-positively charged [Ru(bpy)3]2+-derived linker through electrostatic interaction. The multi-electron injection from the excited Ru photosensitizer to the encapsulated POMs enabled efficient visible-light-driven hydrogen production (Fig. 6A).65 In addition, the bidirectional electron transfer from [Ru(bpy)3]2+-based ligands to the encapsulated copper(II) clusters within cavities was proposed in the Cu–Ru–MOF hybrid catalyst for catalytic CO2 selective hydrogenation to ethanol (Fig. 6B).66 In this case, the CuI species could be generated via single-electron transfer from photoexcited [Ru(bpy)3]2+-based ligands to initial CuII centers, meanwhile, CuI species could be photo-oxidatively regenerated from Cu0via photoexcited Ru2+.
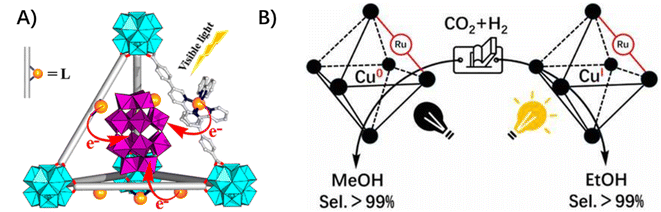 |
| Fig. 6 (A) The ligand-to-inclusion multi-electron injection from the excited Ru photosensitizer to the encapsulated POMs. Reprinted with permission from ref. 65. Copyright 2015 American Chemical Society. (B) The bidirectional electron transfer from [Ru(bpy)3]2+-based ligands to the encapsulated copper(II) clusters within cavities in the Cu–Ru–MOF hybrid catalyst. Reprinted with permission from ref. 66. Copyright 2020 American Chemical Society. | |
In summary, the proximity of chromophores to catalysts oriented within the host–guest assembly facilitates the intra-assembly electron transfer via a pseudo-intramolecular pathway. As a result, the host–guest assembly gives better photocatalytic performances as compared with their homogeneous counterparts. As for the presence of external species to take part in electron transfer, the oxidative and reductive quenching could take place at the assembly–solution interface or the assembly cavity where the mass transfer has to be considered (vide supra).
3.2 The photophysical process of host–guest assembly with photo-inert MOFs
For photo-inert MOFs, the hosts are photophysically unremarkable and serve as containers for guest molecules based on their porous structures and accommodation ability. In these conditions, the complete isolation and restriction of guest molecules within rigid MOF skeletons will inevitably modulate the photophysical properties of incarcerated guests without altering their chemical structures. The detailed energy dissipation following the photoexcitation of chromophores includes the non-radiative channels of vibration and rotation relaxation and intersystem crossing (ISC) from 1MLCT to 3MLCT, the ensuing radiative and nonradiative deactivation of the 3MLCT excited state, and the desired electron transfer from the excited state of the photocenter to the catalytic center, etc. In this section, the photophysical process of host–guest assembly is depicted in light of the type of incarcerated guests, a single molecule, double molecules and a PMD molecule.
3.2.1 Single molecule assembly.
The photophysical properties of three RuL3@InBTB MOFs were studied using the photoluminescence (PL) technique (Fig. 7),67 where three cationic [Ru(bpy)3]2+ (bpy: 2,2′-bipyridine), [Ru(phen)3]2+ (phen: 1,10-phenanthroline), and [Ru(bpz)3]2+ (bpz: 2,2′-bipyrazine) were encapsulated in the mesopores of a three-dimensional InBTB MOF (H3BTB = 1,3,5-benzenetribenzoic acid) through cation-exchange. In comparison with the control bulk sample, the host–guest encapsulation induced a blue-shifted PL emission band of [Ru(bpy)3]2+ in Ru(bpy)3@InBTB, in addition to a prolonged average lifetime of the triplet excited state (3MLCT) through retarding nonradiative decay. In contrast, the PL emission band position remained unaltered for Ru(phen)3@InBTB, while it shifted to the long-wavelength region for Ru(bpz)3@InBTB. These dramatic disparities could be related to the spatial orientations of three RuL32+ ions and distinct nanoscale environments in InBTB channels.
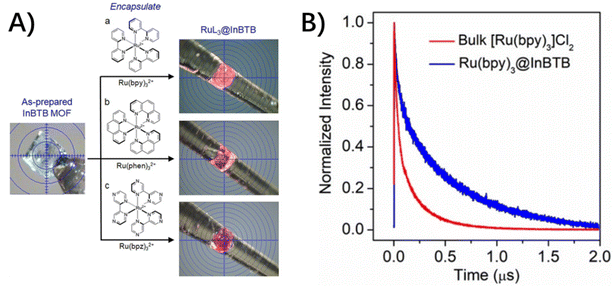 |
| Fig. 7 (A) Photo images of the colorless as-prepared InBTB and the color change in RuL3@InBTB MOFs. (B) Normalized time-resolved photoluminescence decay curves obtained for bulk [Ru(bpy)3]Cl2 and Ru(bpy)3@InBTB upon excitation at 470 nm. Adapted from ref. 67 with permission from Wiley-VCH. | |
3.2.2 Double molecule assembly.
When simultaneously encapsulating a photosensitizer and a catalyst in the photo-inert MOF framework, the separation of the photosensitizer and catalyst in space and the energy migration or electron hopping through space is challenging, especially on involving the third component of sacrificial reagents. The imperfect or mismatched scaffolds of MOF hosts can divert energy and charges away from catalysts. In this type of functional host–guest architecture, the tunability of the pair-wise encapsulation, including the species concentration, ratio and resultant distance, provides an opportunity to manipulate the photoinduced charge separation between neighbouring guests, the framework or media reactants. In an example of immersing a CO2 reduction catalyst [ReBr(CO)3(4,4′-dcbpy)] and a photosensitizer [Ru-(bpy)2(5,5′-dcbpy)]Cl2 inside the cavities of UiO-68 and on the surface of UiO-66 (Fig. 8), the elongating activity but decreasing reaction rate in ReRu-68 compared with ReRu-66 suggested the limited electron communication between a photosensitizer and a catalyst randomly residing within MOF hosts.68
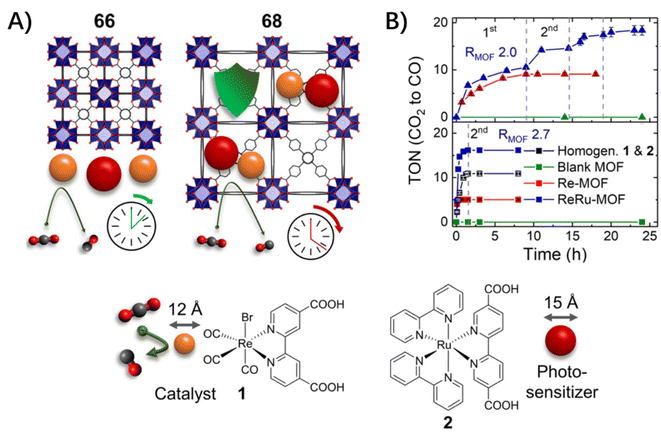 |
| Fig. 8 (A) A schematic of the concept behind the differences in the catalytic performance. (B) Accumulated TON vs. time plot for 66 (bottom) and 68 (top) with pristine MOFs (green), Re–MOF (red), ReRuMOF (blue) with the best-performing RMOF shown, homogeneous 1 and 2 (black). Adapted from ref. 68. | |
3.2.3 Molecular device assembly.
In comparison with incorporating a photosensitizer and a catalyst separately, it is anticipated that a PMD can be encapsulated into MOF hosts, where the photosensitizer and catalyst are covalently linked as a single-component photocatalyst. An intramolecular electron transfer from the excited state of the photosensitizer unit to the catalytic centre through the bridging ligand is expected to enhance the communication between them. The developed PMD could be a bimetallic assembly paired with a covalently linked photosensitizer and catalyst, and a multi-metallic assembly comprised of multiple chromophores connected through bridging ligands to a catalytic site or multiple catalytic units.24,69,70
In previous works, a metal–organic cage (MOC) was explored for H2 evolution by assembling multiple photosensitive ruthenium units and catalytic palladium units within an octahedral structure, which engendered the spatially separated but mutually equivalent multi-channel electron transfers from Ru sites to Pd sites (Fig. 9A).50,51,71,72 After encapsulating this MOC-16 into ZIF-8 and UiO-66 matrixes,48,49 the heterogenous host–guest assemblies inherited the distinctive feature of efficient and directional electron transfer in the picosecond domain of MOC-16, while, the lifetimes of the 3MLCT excited-state were significantly elongated to the microsecond scale due to the inhibition of non-radiative vibrational relaxation by the rigid MOF-matrixes (Fig. 9B). As a result, extra high activities for H2 generation were achieved.
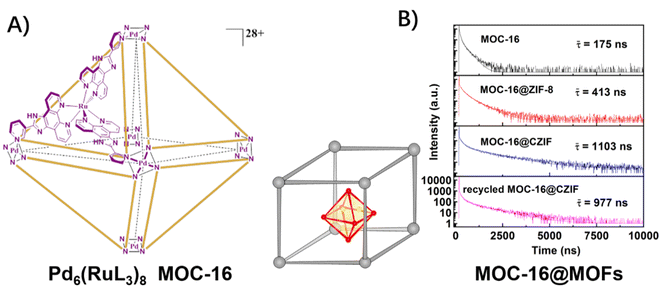 |
| Fig. 9 (A) The structure of a PMD of the Pd6(RuL3)8 metal–organic cage (MOC-16); (B) time-resolved emission decay of the 3MLCT excited-state of MOC-16 and MOC-16@MOFs. Reprinted with permission from ref. 49. Copyright 2019 American Chemical Society. | |
4. The spatial confinement and mass transport in the host–guest assembly
Following the photoexcitation and a series of photophysical events, the dark process of catalytic reaction was considered for host–guest assemblies. Accompanied by the accommodation of functional units or the inclusion of guest species, MOF hosts will serve as a “nanoreactor” providing spatial confinement, while simultaneously allowing for small-molecule permeability by virtue of the permanent porosity, reminiscent of natural enzymes. Therefore, the spatial confinement and the resultant mass transfer in the host–guest assembly are specifically discussed in this section.
4.1 Spatial confinement
The host–guest effects exert physical confinement to isolate and protect the chromophores and redox catalysts, along with chemical confinement to stabilize the high-energy intermediate species via host–guest noncovalent interactions that are difficult to access in the bulk solution.73 Thereby, the reaction pathway could be diverted to achieve unprecedented reaction activities or rate enhancements, even though there are only a few literature precedents of such host–guest assemblies for MOFs.
An example is post-metalating organic linkers of MOF-253 to immobilize Pt(bpy)(Cl)2 moieties with both light-absorbing and catalytic functions (Fig. 10).42 The photocatalytic hydrogen evolution was ascribed to the cooperation of the paired Pt(bpy)(Cl)2 catalyst presumably via a Pt(II)–Pt(III)-hydride intermediate, a mechanism akin to that described in photocatalytically active diplatinum(II) complexes.3,23,42 In this case, the square channels of MOF-253 with closely spaced dcbpy units provide a space for pairs of dichloroplatinum(II) complexes to engender heterogeneous catalytic activity.
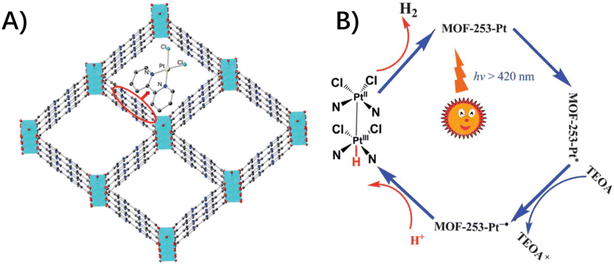 |
| Fig. 10 (A) A model structure of MOF-253–Pt through the post-synthetic modification of MOF-253 with PtCl2. (B) A proposed reaction mechanism via pairs of dichloroplatinum(II) complexes for the photocatalytic H2 evolution. Reproduced from ref. 42 with permission from the Royal Society of Chemistry. | |
4.2 Mass transport
The MOF host could supply the spatial confinement with its rigid frameworks; in the meantime, the restricted diffusion (reactants, sacrificial reagents and products) by its numerous apertures (window, channel and cavity sizes) potentially results in kinetic bottlenecks, in comparison with the bulk solution phase. As for the host–guest assembly, the aperture blockage, accompanied by guest incorporations, could further increase the limitation on mass transport as a result of steric hindrance.74,75 On the other hand, the porosity and hydrophilic/hydrophobic properties of MOF scaffolds could regulate the ionic and molecular transport and provide an environment that facilitates proton transfer, and deliver substrates to catalytic active species nearby.11
In our previous works,48,49 MOC-16 was incorporated into three matrixes of ZIF-8, UiO-66 and ZIF-8-derived-carbonate CZIF to compare the mass transfer based on the wettability and porous structure (Fig. 11). During the H2O-splitting reaction for H2 evolution, no activity appeared in the hydrophobic MOC-16@ZIF-8, but high activities were observed at both hydrophilic MOC-16@UiO-66 and MOC-16@CZIF. The quantitative analysis indicated that a sacrificial reagent could permeate the porous UiO-66 to serve as a two-electron donor, in sharp contrast to acting as a one-electron donor in the nonporous CZIF matrix.
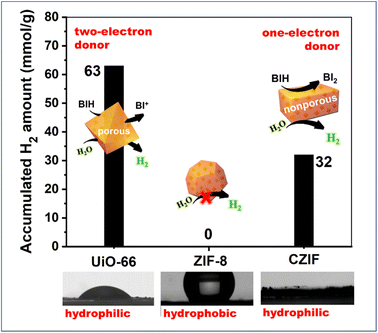 |
| Fig. 11 The host–guest effects on mass transfer based on the wettability and porous structure of MOC-16 incorporated into three matrixes of ZIF-8, UiO-66 and ZIF-8-derived-carbonate CZIF. Reproduced from ref. 48 with permission from Wiley-VCH. | |
Notably, the stability of MOF hosts highly relies on the strength of the coordination between metal-based nodes and organic struts, especially in the presence of sizeable concentrations of aqueous hydroxide.11 In our cases of MOC-16@ZIF-8 and Ru(bda)L2@UiO-66 (bda: 2,2′-bipyridine-6,6′-dicarboxylic acid), the structural derivations of ZIF-8 and UiO-66 hosts with ligand replacements were concomitant with uptakes of carbonate and phosphate, respectively, in the presence of H2O and CO2 and phosphate-buffered saline (PBS).49,76 The isolation and integrity of guest molecules remained, while presenting the outstanding activity of H2 evolution and the impressive turn-over number of O2 evolution, respectively, in the presence of an indispensable CO2 atmosphere and PBS. An enzyme-like microenvironment close to catalytic sites, which is favorable for the proton delivery, was proposed, where carbonate and phosphate act as a proton mediator/relay to assist the proton and/or proton-coupled electron transfer through a hydrogen-bond network of water molecules (Fig. 12).
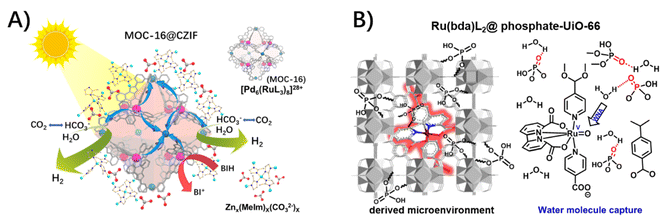 |
| Fig. 12 The uptake of (A) carbonate in MOC-16@ZIF-8 and (B) phosphate in Ru(bda)L2@UiO-66 (reproduced from ref. 76 with permission from Elsevier), as a proton mediator/relay to assist the proton delivery through a hydrogen-bond network of water molecules. | |
5. Conclusion and perspectives
This review highlights the integration of molecular guests with MOF architectures, enabling enzyme-like efficacy to isolate or preorganize photo-active and redox-active sites and manipulate the photophysical and photocatalytic properties. As an emergent field of heterogeneous host–guest assembly, the understanding and modeling of the intertwined host–guest effects physically and chemically are still a great challenge. The precise synthesis to regulate the periodic population of guests throughout frameworks and govern the attainable guest capacity within a porous host, and the specific characterization to determine the distances and angles between guest molecules as well as their molecular conformations, are indispensable for unraveling the structure–property relationships. The highly sensitive techniques and theoretical predictions are valuable in these investigations. The attempts to take advantage of host–guest assemblies for efficiently implementing artificial photosynthesis, are underway.
Conflicts of interest
There are no conflicts to declare.
Acknowledgements
We gratefully acknowledge the financial support from the NKRD Program of China (2021YFA1500401) and the NSFC Project (21875293, 22075332, 21890380).
References
- B. Zhang and L. Sun, Artificial photosynthesis: opportunities and challenges of molecular catalysts, Chem. Soc. Rev., 2019, 48(7), 2216–2264 RSC.
- Y. Yang, J.-S. Chen, J.-Y. Liu, G.-J. Zhao, L. Liu, K.-L. Han, T. R. Cook and P. J. Stang, Photophysical Properties of a Post-Self-Assembly Host/Guest Coordination Cage: Visible Light Driven Core-to-Cage Charge Transfer, J. Phys. Chem. Lett., 2015, 6(10), 1942–1947 CrossRef CAS PubMed.
- M. B. Majewski, A. W. Peters, M. R. Wasielewski, J. T. Hupp and O. K. Farha, Metal–Organic Frameworks as Platform Materials for Solar Fuels Catalysis, ACS Energy Lett., 2018, 3(3), 598–611 CrossRef CAS.
- H. Zhang, J. Nai, L. Yu and X. W. Lou, Metal-Organic-Framework-Based Materials as Platforms for Renewable Energy and Environmental Applications, Joule, 2017, 1(1), 77–107 CrossRef CAS.
- H. Fei, M. D. Sampson, Y. Lee, C. P. Kubiak and S. M. Cohen, Photocatalytic CO2 Reduction to Formate Using a Mn(I) Molecular Catalyst in a Robust Metal–Organic Framework, Inorg. Chem., 2015, 54(14), 6821–6828 CrossRef CAS.
- T. Zhang and W. Lin, Metal–organic frameworks for artificial photosynthesis and photocatalysis, Chem. Soc. Rev., 2014, 43(16), 5982–5993 RSC.
- H. Yamashita, K. Mori, Y. Kuwahara, T. Kamegawa, M. Wen, P. Verma and M. Che, Single-site and nano-confined photocatalysts designed in porous materials for environmental uses and solar fuels, Chem. Soc. Rev., 2018, 47(22), 8072–8096 RSC.
- X. Jing, C. He, L. Zhao and C. Duan, Photochemical Properties of Host–Guest Supramolecular Systems with Structurally Confined Metal–Organic Capsules, Acc. Chem. Res., 2019, 52(1), 100–109 CrossRef CAS PubMed.
- K. Mori and H. Yamashita, Metal Complexes Supported on Solid Matrices for Visible-Light-Driven Molecular Transformations, Chem.–Eur. J., 2016, 22(32), 11122–11137 CrossRef CAS PubMed.
- D. Li, H.-Q. Xu, L. Jiao and H.-L. Jiang, Metal-organic frameworks for catalysis: State of the art, challenges, and opportunities, EnergyChem, 2019, 1(1), 100005 CrossRef.
- S. Banerjee, R. I. Anayah, C. S. Gerke and V. S. Thoi, From Molecules to Porous Materials: Integrating Discrete Electrocatalytic Active Sites into Extended Frameworks, ACS Cent. Sci., 2020, 6(10), 1671–1684 CrossRef CAS PubMed.
- M. Sohmiya, K. Saito and M. Ogawa, Host–guest chemistry of mesoporous silicas: precise design of location, density and orientation of molecular guests in mesopores, Sci. Technol. Adv. Mater., 2015, 16(5), 054201 CrossRef.
- E. A. Dolgopolova, A. M. Rice, C. R. Martin and N. B. Shustova, Photochemistry and photophysics of MOFs: steps towards MOF-based sensing enhancements, Chem. Soc. Rev., 2018, 47(13), 4710–4728 RSC.
- L. Zeng, X. Guo, C. He and C. Duan, Metal–Organic Frameworks: Versatile Materials for Heterogeneous Photocatalysis, ACS Catal., 2016, 6(11), 7935–7947 CrossRef CAS.
- S. Wang and X. Wang, Multifunctional Metal–Organic Frameworks for Photocatalysis, Small, 2015, 11(26), 3097–3112 CrossRef CAS PubMed.
- B. Gibbons, M. Cai and A. J. Morris, A Potential Roadmap to Integrated Metal Organic Framework Artificial Photosynthetic Arrays, J. Am. Chem. Soc., 2022, 144(39), 17723–17736 CrossRef CAS PubMed.
- W. A. Maza and A. J. Morris, Photophysical Characterization of a Ruthenium(II) Tris(2,2'-bipyridine)-Doped Zirconium UiO-67 Metal-Organic Framework, J. Phys. Chem. C, 2014, 118(17), 8803–8817 CrossRef CAS.
- A. Dhakshinamoorthy, A. M. Asiri and H. García, Metal–Organic Framework (MOF) Compounds: Photocatalysts for Redox Reactions and Solar Fuel Production, Angew. Chem., Int. Ed., 2016, 55(18), 5414–5445 CrossRef CAS PubMed.
- M. C. So, G. P. Wiederrecht, J. E. Mondloch, J. T. Hupp and O. K. Farha, Metal–organic framework materials for light-harvesting and energy transfer, Chem. Commun., 2015, 51(17), 3501–3510 RSC.
- J. Liu, L. Chen, H. Cui, J. Zhang, L. Zhang and C.-Y. Su, Applications of metal–organic frameworks in heterogeneous supramolecular catalysis, Chem. Soc. Rev., 2014, 43(16), 6011–6061 RSC.
- Y. Jin, Q. Zhang, Y. Zhang and C. Duan, Electron transfer in the confined environments of metal–organic coordination supramolecular systems, Chem. Soc. Rev., 2020, 49(15), 5561–5600 RSC.
- L. Zhao, X. Jing, X. Li, X. Guo, L. Zeng, C. He and C. Duan, Catalytic properties of chemical transformation within the confined pockets of Werner-type capsules, Coord. Chem. Rev., 2019, 378, 151–187 CrossRef CAS.
- H. Ozawa and K. Sakai, Photo-hydrogen-evolving molecular devices driving visible-light-induced water reduction into molecular hydrogen: structure-activity relationship and reaction mechanism, Chem. Commun., 2011, 47(8), 2227–2242 RSC.
- G. F. Manbeck, E. Fujita and K. J. Brewer, Tetra- and Heptametallic Ru(II),Rh(III) Supramolecular Hydrogen Production Photocatalysts, J. Am. Chem. Soc., 2017, 139(23), 7843–7854 CrossRef CAS PubMed.
- P. M. Stanley, J. Haimerl, N. B. Shustova, R. A. Fischer and J. Warnan, Merging molecular catalysts and metal–organic frameworks for photocatalytic fuel production, Nat. Chem., 2022, 14, 1342–1356 CrossRef CAS PubMed.
- Y. Kuramochi, M. Sekine, K. Kitamura, Y. Maegawa, Y. Goto, S. Shirai, S. Inagaki and H. Ishida, Photocatalytic CO2 Reduction by Periodic Mesoporous Organosilica (PMO) Containing Two Different Ruthenium Complexes as Photosensitizing and Catalytic Sites, Chemistry, 2017, 23(43), 10301–10309 CrossRef CAS PubMed.
- K. M. Choi, D. Kim, B. Rungtaweevoranit, C. A. Trickett, J. T. Barmanbek, A. S. Alshammari, P. Yang and O. M. Yaghi, Plasmon-Enhanced Photocatalytic CO2 Conversion within Metal−Organic Frameworks under Visible Light, J. Am. Chem. Soc., 2017, 139(1), 356–362 CrossRef CAS PubMed.
- B. Yu, L. Li, S. Liu, H. Wang, H. Liu, C. Lin, C. Liu, H. Wu, W. Zhou, X. Li, T. Wang, B. Chen and J. Jiang, Robust Biological Hydrogen-Bonded Organic Framework with Post-Functionalized Rhenium(I) Sites for Efficient Heterogeneous Visible-Light-Driven CO2 Reduction, Angew. Chem., Int. Ed. Engl., 2021, 60(16), 8983–8989 CrossRef CAS PubMed.
- M. A. Nasalevich, R. Becker, E. V. Ramos-Fernandez, S. Castellanos, S. L. Veber, M. V. Fedin, F. Kapteijn, J. N. H. Reek, J. I. van der Vlugt and J. Gascon, Co@NH2-MIL-125(Ti): cobaloxime-derived metal–organic framework-based composite for light-driven H2 production, Energy Environ. Sci., 2015, 8(1), 364–375 RSC.
- G. Lan, Y.-Y. Zhu, S. S. Veroneau, Z. Xu, D. Micheroni and W. Lin, Electron Injection from Photoexcited Metal–Organic Framework Ligands to Ru2 Secondary Building Units for Visible-Light-Driven Hydrogen Evolution, J. Am. Chem. Soc., 2018, 140(16), 5326–5329 CrossRef CAS PubMed.
- K. Sasan, Q. Lin, C. Mao and P. Feng, Incorporation of iron hydrogenase active sites into a highly stable metal–organic framework for photocatalytic hydrogen generation, Chem. Commun., 2014, 50(72), 10390–10393 RSC.
- S. Pullen, H. Fei, A. Orthaber, S. M. Cohen and S. Ott, Enhanced Photochemical Hydrogen Production by a Molecular Diiron Catalyst Incorporated into a Metal–Organic Framework, J. Am. Chem. Soc., 2013, 135(45), 16997–17003 CrossRef CAS PubMed.
- D. Kim, D. R. Whang and S. Y. Park, Self-Healing of Molecular Catalyst and Photosensitizer on Metal–Organic Framework: Robust Molecular System for Photocatalytic H2 Evolution from Water, J. Am. Chem. Soc., 2016, 138(28), 8698–8701 CrossRef CAS PubMed.
- M. Rimoldi, A. J. Howarth, M. R. DeStefano, L. Lin, S. Goswami, P. Li, J. T. Hupp and O. K. Farha, Catalytic Zirconium/Hafnium-Based Metal–Organic Frameworks, ACS Catal., 2017, 7(2), 997–1014 CrossRef CAS.
- C. Wang, K. E. deKrafft and W. Lin, Pt Nanoparticles@Photoactive Metal–Organic Frameworks: Efficient Hydrogen Evolution via Synergistic Photoexcitation and Electron Injection, J. Am. Chem. Soc., 2012, 134(17), 7211–7214 CrossRef CAS.
- C. Wang, Z. Xie, K. E. deKrafft and W. Lin, Doping Metal–Organic Frameworks for Water Oxidation, Carbon Dioxide Reduction, and Organic Photocatalysis, J. Am. Chem. Soc., 2011, 133(34), 13445–13454 CrossRef CAS PubMed.
- Y. Song, Z. Li, Y. Zhu, X. Feng, J. S. Chen, M. Kaufmann, C. Wang and W. Lin, Titanium Hydroxide Secondary Building Units in Metal-Organic Frameworks Catalyze Hydrogen Evolution under Visible Light, J. Am. Chem. Soc., 2019, 141(31), 12219–12223 CrossRef CAS PubMed.
- T. C. Zhuo, Y. Song, G. L. Zhuang, L. P. Chang, S. Yao, W. Zhang, Y. Wang, P. Wang, W. Lin, T. B. Lu and Z. M. Zhang, H-Bond-Mediated Selectivity Control of Formate versus CO during CO(2) Photoreduction with Two Cooperative Cu/X Sites, J. Am. Chem. Soc., 2021, 143(16), 6114–6122 CrossRef CAS PubMed.
- S. Lin, A. K. Ravari, J. Zhu, P. M. Usov, M. Cai, S. R. Ahrenholtz, Y. Pushkar and A. J. Morris, Insight into Metal–Organic Framework Reactivity: Chemical Water Oxidation Catalyzed by a [Ru(tpy)(dcbpy)(OH2)]2+-Modified UiO-67, ChemSusChem, 2018, 11(2), 464–471 CrossRef CAS PubMed.
- T. Liseev, A. Howe, M. A. Hoque, C. Gimbert-Suriñach, A. Llobet and S. Ott, Synthetic strategies to incorporate Ru-terpyridyl water oxidation catalysts into MOFs: direct synthesis vs. post-synthetic approach, Dalton Trans., 2020, 49(39), 13753–13759 RSC.
- R. Ezhov, A. Karbakhsh Ravari, A. Page and Y. Pushkar, Water Oxidation Catalyst cis-[Ru(bpy)(5,5'-dcbpy)(H2O)2]2+ and Its Stabilization in Metal-Organic Framework, ACS Catal., 2020, 10(9), 5299–5308 CrossRef CAS.
- T. Zhou, Y. Du, A. Borgna, J. Hong, Y. Wang, J. Han, W. Zhang and R. Xu, Post-synthesis modification of a metal–organic framework to construct a bifunctional photocatalyst for hydrogen production, Energy Environ. Sci., 2013, 6(11), 3229–3234 RSC.
- B. An, L. Zeng, M. Jia, Z. Li, Z. Lin, Y. Song, Y. Zhou, J. Cheng, C. Wang and W. Lin, Molecular Iridium Complexes in Metal-Organic Frameworks Catalyze CO(2) Hydrogenation via Concerted Proton and Hydride Transfer, J. Am. Chem. Soc., 2017, 139(49), 17747–17750 CrossRef CAS.
- B. Nepal and S. Das, Sustained Water Oxidation by a Catalyst Cage-Isolated in a Metal–Organic Framework, Angew. Chem., Int. Ed., 2013, 52(28), 7224–7227 CrossRef CAS PubMed.
- X. Liang, S. Yang, J. Yang, W. Sun, X. Li, B. Ma, J. Huang, J. Zhang, L. Duan and Y. Ding, Covalent immobilization of molecular complexes on metal-organic frameworks towards robust and highly efficient heterogeneous water oxidation catalysts, Appl. Catal., B, 2021, 291, 120070 CrossRef CAS.
- Z. Li, T. M. Rayder, L. Luo, J. A. Byers and C.-K. Tsung, Aperture-Opening Encapsulation of a Transition Metal Catalyst in a Metal–Organic
Framework for CO2 Hydrogenation, J. Am. Chem. Soc., 2018, 140(26), 8082–8085 CrossRef CAS PubMed.
- Y. Luo, S. Fan, W. Yu, Z. Wu, D. A. Cullen, C. Liang, J. Shi and C. Su, Fabrication of Au-25(SG)(18)-ZIF-8 Nanocomposites: A Facile Strategy to Position Au-25(SG)(18) Nanoclusters Inside and Outside ZIF-8, Adv. Mater., 2018, 30(6), 1704576 CrossRef PubMed.
- D. Wu, Y. Luo, X. Li, Z. Su, J. Shi and C. Su, Revisiting the environment effect on mass transfer for heterogenized Ru6Pd8 metal-organic cage photocatalyst confined within 3D matrix, Chemistry, 2022, e202200310 CAS.
- Y.-C. Luo, K.-L. Chu, J.-Y. Shi, D.-J. Wu, X.-D. Wang, M. Mayor and C.-Y. Su, Heterogenization of Photochemical Molecular Devices: Embedding a Metal-Organic Cage into a ZIF-8-Derived Matrix To Promote Proton and Electron Transfer, J. Am. Chem. Soc., 2019, 141(33), 13057–13065 CrossRef CAS PubMed.
- K. Li, L.-Y. Zhang, C. Yan, S.-C. Wei, M. Pan, L. Zhang and C.-Y. Su, Stepwise Assembly of Pd-6(RuL3)(8) Nanoscale Rhombododecahedral Metal-Organic Cages via Metalloligand Strategy for Guest Trapping and Protection, J. Am. Chem. Soc., 2014, 136(12), 4456–4459 CrossRef CAS PubMed.
- S. Chen, K. Li, F. Zhao, L. Zhang, M. Pan, Y.-Z. Fan, J. Guo, J. Shi and C.-Y. Su, A metal-organic cage incorporating multiple light harvesting and catalytic centres for photochemical hydrogen production, Nat. Commun., 2016, 7, 13169 CrossRef CAS PubMed.
- B. V. Kramar, N. C. Flanders, W. Helweh, W. R. Dichtel, J. T. Hupp and L. X. Chen, Light Harvesting Antenna Properties of Framework Solids, Acc. Mater. Res., 2022, 3(11), 1149–1159 CrossRef CAS.
- S. M. Shaikh, S. Ilic, B. J. Gibbons, X. Yang, E. Jakubikova and A. J. Morris, Role of a 3D Structure in Energy Transfer in Mixed-Ligand Metal–Organic Frameworks, J. Phys. Chem. C, 2021, 125(42), 22998–23010 CrossRef CAS.
- A. Chakraborty, S. Ilic, M. Cai, B. J. Gibbons, X. Yang, C. C. Slamowitz and A. J. Morris, Role of Spin–Orbit Coupling in Long Range Energy Transfer in Metal–Organic Frameworks, J. Am. Chem. Soc., 2020, 142(48), 20434–20443 CrossRef CAS PubMed.
- C. A. Kent, B. P. Mehl, L. Ma, J. M. Papanikolas, T. J. Meyer and W. Lin, Energy Transfer Dynamics in Metal−Organic Frameworks, J. Am. Chem. Soc., 2010, 132(37), 12767–12769 CrossRef CAS PubMed.
- C. A. Kent, D. Liu, T. J. Meyer and W. Lin, Amplified Luminescence Quenching of Phosphorescent Metal–Organic Frameworks, J. Am. Chem. Soc., 2012, 134(9), 3991–3994 CrossRef CAS PubMed.
- H.-J. Son, S. Jin, S. Patwardhan, S. J. Wezenberg, N. C. Jeong, M. So, C. E. Wilmer, A. A. Sarjeant, G. C. Schatz, R. Q. Snurr, O. K. Farha, G. P. Wiederrecht and J. T. Hupp, Light-Harvesting and Ultrafast Energy Migration in Porphyrin-Based Metal–Organic Frameworks, J. Am. Chem. Soc., 2013, 135(2), 862–869 CrossRef CAS PubMed.
- X. Zhao, X. Song, Y. Li, Z. Chang and L. Chen, Targeted Construction of Light-Harvesting Metal–Organic Frameworks Featuring Efficient Host–Guest Energy Transfer, ACS Appl. Mater. Interfaces, 2018, 10(6), 5633–5640 CrossRef CAS.
- Q. Zhang, C. Zhang, L. Cao, Z. Wang, B. An, Z. Lin, R. Huang, Z. Zhang, C. Wang and W. Lin, Förster Energy Transport in Metal–Organic Frameworks Is Beyond Step-by-Step Hopping, J. Am. Chem. Soc., 2016, 138(16), 5308–5315 CrossRef CAS PubMed.
- S. Yang, D. Fan, W. Hu, B. Pattengale, C. Liu, X. Zhang and J. Huang, Elucidating Charge Separation Dynamics in a Hybrid Metal–Organic Framework Photocatalyst for Light-Driven H2 Evolution, J. Phys. Chem. C, 2018, 122(6), 3305–3311 CrossRef CAS.
- S. Yang, B. Pattengale, S. Lee and J. Huang, Real-Time Visualization of Active Species in a Single-Site Metal–Organic Framework Photocatalyst, ACS Energy Lett., 2018, 3(3), 532–539 CrossRef CAS.
- X. Deng, J. Albero, L. Xu, H. Garcia and Z. Li, Construction of a Stable Ru-Re Hybrid System Based on Multifunctional MOF-253 for Efficient Photocatalytic CO2 Reduction, Inorg. Chem., 2018, 57(14), 8276–8286 CrossRef CAS PubMed.
- S. Karmakar, S. Barman, F. A. Rahimi and T. K. Maji, Covalent grafting of molecular photosensitizer and catalyst on MOF-808: effect of pore confinement toward visible light-driven CO2 reduction in water, Energy Environ. Sci., 2021, 14(4), 2429–2440 RSC.
- Z.-H. Yan, M.-H. Du, J. Liu, S. Jin, C. Wang, G.-L. Zhuang, X.-J. Kong, L.-S. Long and L.-S. Zheng, Photo-generated dinuclear {Eu(II)}2 active sites for selective CO2 reduction in a photosensitizing metal-organic framework, Nat. Commun., 2018, 9(1), 3353 CrossRef PubMed.
- Z.-M. Zhang, T. Zhang, C. Wang, Z. Lin, L.-S. Long and W. Lin, Photosensitizing Metal–Organic Framework Enabling Visible-Light-Driven Proton Reduction by a Wells–Dawson-Type Polyoxometalate, J. Am. Chem. Soc., 2015, 137(9), 3197–3200 CrossRef CAS PubMed.
- L. Zeng, Z. Wang, Y. Wang, J. Wang, Y. Guo, H. Hu, X. He, C. Wang and W. Lin, Photoactivation of Cu Centers in Metal–Organic Frameworks for Selective CO2 Conversion to Ethanol, J. Am. Chem. Soc., 2020, 142(1), 75–79 CrossRef CAS PubMed.
- I. H. Choi, S. Yoon, S. Huh, S. J. Kim and Y. Kim, Photophysical Properties and Heterogeneous Photoredox Catalytic Activities of Ru(bpy)3@InBTB Metal–Organic Framework (MOF), Chem.–Eur. J., 2020, 26(64), 14580–14584 CrossRef CAS PubMed.
- P. Stanley, J. Haimerl, C. Thomas, A. Urstoeger, M. Schuster, N. Shustova, A. Casini, B. Rieger, J. Warnan and R. Fischer, Host-Guest Interactions in Metal-Organic Framework Isoreticular Series for Molecular Photocatalytic CO2 Reduction, Angew. Chem., Int. Ed., 2021, 60, 17854–17860 CrossRef CAS PubMed.
- M. G. Pfeffer, B. Schaefer, G. Smolentsev, J. Uhlig, E. Nazarenko, J. Guthmuller, C. Kuhnt, M. Waechtler, B. Dietzek, V. Sundstroem and S. Rau, Palladium versus Platinum: The Metal in the Catalytic Center of a Molecular Photocatalyst Determines the Mechanism of the Hydrogen Production with Visible Light, Angew. Chem., Int. Ed., 2015, 54(17), 5044–5048 CrossRef CAS PubMed.
- M. G. Pfeffer, T. Kowacs, M. Waechtler, J. Guthmuller, B. Dietzek, J. G. Vos and S. Rau, Optimization of Hydrogen-Evolving Photochemical Molecular Devices, Angew. Chem., Int. Ed., 2015, 54(22), 6627–6631 CrossRef CAS.
- J. Guo, Y.-W. Xu, K. Li, L.-M. Xiao, S. Chen, K. Wu, X.-D. Chen, Y.-Z. Fan, J.-M. Liu and C.-Y. Su, Regio- and Enantioselective Photodimerization within the Confined Space of a Homochiral Ruthenium/Palladium Heterometallic Coordination Cage, Angew. Chem., Int. Ed., 2017, 56(14), 3852–3856 CrossRef CAS PubMed.
- K. Wu, K. Li, S. Chen, Y.-J. Hou, Y.-L. Lu, J.-S. Wang, M.-J. Wei, M. Pan and C.-Y. Su, The Redox Coupling Effect in a Photocatalytic RuII-PdII Cage with TTF Guest as Electron Relay Mediator for Visible-Light Hydrogen-Evolving Promotion, Angew. Chem., Int. Ed., 2020, 59(7), 2639–2643 CrossRef CAS PubMed.
- A. B. Grommet, M. Feller and R. Klajn, Chemical reactivity under nanoconfinement, Nat. Nanotechnol., 2020, 15(4), 256–271 CrossRef CAS PubMed.
- B. A. Johnson, A. M. Beiler, B. D. McCarthy and S. Ott, Transport Phenomena: Challenges and Opportunities for Molecular Catalysis in Metal–Organic Frameworks, J. Am. Chem. Soc., 2020, 142(28), 11941–11956 CrossRef CAS PubMed.
- C. H. Sharp, B. C. Bukowski, H. Li, E. M. Johnson, S. Ilic, A. J. Morris, D. Gersappe, R. Q. Snurr and J. R. Morris, Nanoconfinement and mass transport in metal–organic frameworks, Chem. Soc. Rev., 2021, 50(20), 11530–11558 RSC.
- J. Feng, X. Li, Y. Luo, Z. Su, M. Zhong, B. Yu and J. Shi, Regulating the Microenvironment of Ru(bda)L2 Catalyst Incorporated in Metal-organic Framework for Effective Photo-driven Water Oxidation, Chin. J. Catal., 2023 DOI:10.1016/S1872-2067(23)64411-0.
|
This journal is © The Royal Society of Chemistry 2023 |
Click here to see how this site uses Cookies. View our privacy policy here.