DOI:
10.1039/C4RA00010B
(Paper)
RSC Adv., 2014,
4, 12849-12856
8-TQEN (N,N,N′,N′-tetrakis(8-quinolylmethyl)ethylenediamine) analogs as fluorescent cadmium sensors: strategies to enhance Cd2+-induced fluorescence and Cd2+/Zn2+ selectivity†
Received
1st January 2014
, Accepted 20th February 2014
First published on 24th February 2014
Abstract
In order to exploit the untapped sensing potential of the TQEN (N,N,N′,N′-tetrakis(2-quinolylmethyl)ethylenediamine) family of fluorescent probes, 8-TQEN (N,N,N′,N′-tetrakis(8-quinolylmethyl)ethylenediamine) analogs were designed and characterized. Although 8-TQEN lacks practicality owing to poor solubility in both aqueous media and organic solvents, 6-MeO-8-TQEN (N,N,N′,N′-tetrakis(6-methoxy-8-quinolylmethyl)ethylenediamine, 1b) exhibits Cd2+-selective fluorescence enhancement at 395 nm in DMF–HEPES buffer (1
:
1), (ICd/I0 = 25, λex = 332 nm, ϕCd = 0.025). Zn2+ induces weaker fluorescence (IZn/I0 = 9, IZn/ICd = 0.35). In contrast, both the parent probes TQEN (IZn/I0 = 23, ICd/IZn = 0.64) and 6-MeOTQEN (IZn/I0 = 11, ICd/IZn = 1.29 exhibit higher sensitivity toward Zn2+. When the quinoline groups were replaced with 8-hydroxyquinoline different responses were observed. The propanediamine derivative, 8-TQOEPN (N,N,N′,N′-tetrakis(8-quinolyloxyethylene)propanediamine, 2c) exhibits significant fluorescence enhancement at 423 nm upon Cd2+ binding (λex = 325 nm, ICd/I0 = 19, ϕCd = 0.31). Fluorescence enhancement is Cd2+-specific as Zn2+ induces only more modest emission increases (IZn/I0 = 8.8, IZn/ICd = 0.45).
Introduction
Techniques to visualize specific metal ions are necessary to investigate inorganic biochemistry and environmental pollutants.1–6 Since metal ions perform indispensable tasks in biological systems, metal ion concentration in organs and/or whole cells is tightly regulated. To maintain optimal function, cells must acquire and excrete metal ions efficiently to prevent both deficiencies and excesses in concentrations. Despite the essentiality of regulation, the exact metal ion homeostasis mechanisms are still being elucidated. Fluorescent probes are an attractive approach to visualize metal ions for numerous applications.2,7 Fluorescent Zn2+ sensors have been investigated extensively to interrogate the biological roles of zinc.8–11 The heavy elements from group 12, Hg2+ and Cd2+, are also potential targets for fluorescent sensor development. New Hg2+ and Cd2+ probes could facilitate investigating the mechanisms of toxicity as well as therapeutic strategies.12–17 Since Zn2+ and Cd2+ possess similar coordination chemistry, discriminating between the two metal ions is a significant and important challenge in sensor development.6,18–22
One of our long-standing goals is to develop structurally simple, Cd2+-specific fluorescent sensors that can be made readily available to the scientific community. Previously, we reported that TQEN (N,N,N′,N′-tetrakis(2-quinolylmethyl)ethylenediamine), a simple analog of TPEN (N,N,N′,N′-tetrakis(2-pyridylmethyl)ethylenediamine), exhibits emission enhancement upon Zn2+ complexation (Chart 1).23 The emission enhancement observed for TQEN results from a combination of the PET (photo-induced electron transfer) and the CHEF (chelation enhanced fluorescence) mechanisms of fluorescence switching.1,6 In addition to Zn-induced emission enhancement, TQEN also responds to Cd2+ (ICd/IZn = 0.64). The introduction of 6-methoxy group onto quinoline ring of TQEN increases the Cd2+ sensitivity in 6-MeOTQEN (ICd/IZn = 1.29).24 Since the substitution of quinoline for the isoquinoline chromophore25,26 and the modification of molecular scaffold21,26 reduced the Cd2+ response significantly, we sought an alternative strategy to increase Cd2+-selectivity.
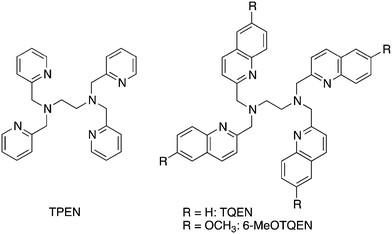 |
| Chart 1 | |
In this paper, we describe the Cd2+-induced fluorescence enhancement of 6-MeO-8-TQEN (N,N,N′,N′-tetrakis(6-methoxy-8-quinolylmethyl)ethylenediamine, 1b, Chart 2) and 8-TQOEPN (N,N,N′,N′-tetrakis(2-(8-quinolyloxy)ethyl)propanediamine), 2c, Chart 2). Isomers with different chain lengths also were investigated to further examine the structural requirements need to obtain an efficient fluorescence response.
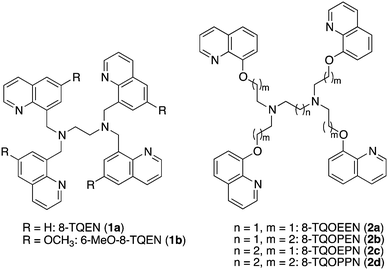 |
| Chart 2 | |
Experimental
General
All reagents and solvents used for synthesis were from commercial sources and used as received. Compound 2a is a known compound27 and was synthesized from a chloride precursor.28,29 The other compounds were prepared as outlined in Scheme 1 from reported compounds.29,30 N,N-Dimethylformamide (DMF, Dojin) was spectral grade (Spectrosol). All aqueous solution was prepared using Milli-Q water (Millipore). 1H NMR (300 Hz) and 13C NMR (75.5 Hz) spectra were recorded on a Varian GEMINI 2000 spectrometer and referenced to internal Si(CH3)4 or solvent signals. UV-vis and fluorescence spectra were measured on a Jasco V-660 spectrophotometer and Jasco FP-6300 spectrofluorometer, respectively. Fluorescence quantum yields were measured on a HAMAMATSU photonics C9920-02 absolute PL quantum yield measurement system.
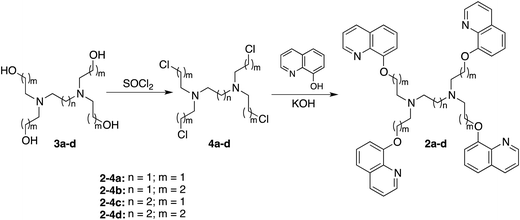 |
| Scheme 1 | |
N,N,N′,N′-Tetrakis(8-quinolylmethyl)ethylenediamine (8-TQEN, 1a)
To a dry CH3CN solution (30 mL) of 8-bromomethylquinoline (1.72 g, 7.75 mmol) and ethylenediamine (0.13 mL, 1.94 mmol) was added potassium carbonate (1.74 g, 12.6 mmol) and potassium iodide (573 mg, 3.45 mmol). The resulting reaction mixture was refluxed for 2 days under N2. The resultant solution was cooled to room temperature and the solvent was evaporated. The residue was washed with CHCl3 and water to give compound 1a as a white solid (450 mg, 0.72 mmol, 37%). This material is insoluble to water, ethanol, chloroform, DMF, DMSO and other common organic solvents. Anal. calcd for C42H36N6 (1a·0.2H2O): C, 80.29; H, 5.84; N, 13.37. Found: C, 80.07; H, 5.78; N, 13.28.
N,N,N′,N′-Tetrakis(6-methoxy-8-quinolylmethyl)ethylenediamine (6-MeO-8-TQEN, 1b)
To a dry CH3CN solution (30 mL) of 6-methoxy-8-bromomethylquinoline (118 mg, 0.56 mmol) and ethylenediamine (9.5 μL, 0.14 mmol) was added potassium carbonate (196 mg, 1.40 mmol) and potassium iodide (236 mg, 1.40 mmol). The resulting reaction mixture was refluxed for 2 days under N2, cooled to room temperature and the solvent was evaporated. The residue was extracted with CHCl3–water and the organic layer was dried and evaporated to give compound 1b as a white solid (109 mg, 0.14 mmol, quant.). 1H NMR (DMSO-d6): δ (ppm) 8.60 (dd, 4H, J = 1.7, 4.1 Hz), 8.17 (dd, 4H, J = 1.7, 8.1 Hz), 7.61 (d, 4H, J = 2.4 Hz), 7.40 (dd, 4H, J = 4.1, 8.1 Hz), 7.12 (d, 4H, J = 2.4 Hz), 4.32 (s, 8H), 3.74 (s, 12H), 2.93 (s, 4H). 13C NMR (DMSO-d6): δ (ppm) 156.7, 146.4, 142.0, 139.1, 134.8, 129.0, 121.3, 119.9, 103.8, 87.7, 55.2, 54.1. HRMS (ESI-MS) m/z: [M + Na]+ calcd for C46H44N6O4Na 745.3502; found 745.3567. Anal. calcd for C46H45N6O4.5 (1b·0.5H2O): C, 73.28; H, 6.01; N, 11.14. Found: C, 73.23; H, 6.04; N, 11.17.
N,N,N′,N′-Tetrakis(2-(8-quinolyloxy)ethyl)ethylenediamine (8-TQOEEN, 2a)
To an agitated mixture of 8-quinolinol (2.18 g, 14.8 mmol) and powdered potassium hydroxide (830 mg, 14.8 mmol) in dry ethanol (30 mL) was added N,N,N′,N′-tetrakis(2-chloroethyl)ethylenediamine (4a) (460 mg, 1.48 mmol) in 3 mL of ethanol. The resulting reaction mixture was refluxed overnight under N2, cooled to room temperature and the solvent was evaporated. The residue was extracted with CHCl3–water and the organic layer was washed with 3 N NaOH, dried and evaporated. The residue was purified by column chromatography (alumina, CHCl3/CH3OH 99.5/0.5, Rf = 0.35) to give compound 2a as a yellow oil (226 mg, 0.30 mmol, 20%). 1H NMR (CDCl3): δ (ppm) 8.91 (dd, J = 4.3, 1.8 Hz, 4H), 8.14 (dd, J = 8.2, 1.5 Hz, 4H), 7.40–7.45 (m, 12H), 7.06 (dd, J = 7.3, 1.5 Hz, 4H), 4.34 (t, J = 5.8 Hz, 8H), 3.05 (t, J = 6.0 Hz, 8H), 2.76 (s, 4H). 13C NMR (CDCl3): δ (ppm) 154.0, 148.6, 139.5, 136.0, 129.3, 126.5, 121.6, 119.5, 64.2, 56.8, 52.5. HRMS (ESI-MS) m/z: [M + Na]+ calcd for C46H44N6O4Na 767.3322; found 767.3434.
N,N,N′,N′-Tetrakis(3-(8-quinolyloxy)propyl)ethylenediamine (8-TQOPEN, 2b)
To an agitated mixture of 8-quinolinol (1.88 g, 13.0 mmol) and powdered potassium hydroxide (788 mg, 14.0 mmol) in dry ethanol (30 mL) was added N,N,N′,N′-tetrakis(3-chloropropyl)ethylenediamine (4b) (397 mg, 1.08 mmol) in 3 mL of ethanol. The resulting reaction mixture was refluxed overnight under N2, cooled to room temperature and the solvent was evaporated. The residue was extracted with CHCl3–water and the organic layer was washed with 3 N NaOH, dried and evaporated. The residue was purified by column chromatography (alumina, CHCl3/CH3OH 99.5/0.5, Rf = 0.28) to give a yellow oil. This material was dissolved in small portions of acetonitrile and conc. hydrochloric acid was added to induce precipitation. After filtration, the obtained hydrochloride salt was dissolved in water and 6 N NaOH was added to adjust the pH > 12. The product was extracted into chloroform, dried and evaporated to give compound 2b as a yellow oil (130 mg, 0.162 mmol, 15%). 1H NMR (CDCl3): δ (ppm) 8.89 (dd, J = 4.3, 1.5 Hz, 4H), 8.05 (dd, J = 8.5, 1.5 Hz, 4H), 7.27–7.38 (m, 12H), 6.87 (dd, J = 7.3, 1.5 Hz, 4H), 4.06 (t, J = 6.6 Hz, 8H), 2.55 (t, J = 6.6 Hz, 8H), 2.43 (s, 4H), 1.88 (quint, J = 6.5 Hz, 8H). 13C NMR (CDCl3): δ (ppm) 154.5, 148.9, 140.1, 135.5, 129.2, 126.5, 121.3, 119.1, 108.5, 66.7, 52.6, 50.7, 26.9. HRMS (ESI-MS) m/z: [M + Na]+ calcd for C50H52N6O4Na 823.3948; found 823.3985. Anal. calcd for C50H57Cl2N6O5.5 (2b·2HCl·1.5H2O): C, 66.66; H, 6.38; N, 9.33. Found: C, 66.77; H, 5.99; N, 9.23.
N,N,N′,N′-Tetrakis(2-(8-quinolyloxy)ethyl)propanediamine (8-TQOEPN, 2c)
To an agitated mixture of 8-quinolinol (1.53 g, 10.6 mmol) and powdered potassium hydroxide (592 mg, 10.6 mmol) in dry ethanol (30 mL) was added N,N,N′,N′-tetrakis(2-chloroethyl)propanediamine (4c) (285 mg, 1.06 mmol) in 3 mL of ethanol. The resulting reaction mixture was refluxed overnight under N2, cooled to room temperature and the solvent was evaporated. The residue was extracted with CHCl3–water and the organic layer was washed with 3 N NaOH, dried and evaporated. The residue was purified by column chromatography (alumina, CHCl3/CH3OH 99.5/0.5, Rf = 0.3) to give compound 2c as a yellow oil (88 mg, 0.12 mmol, 9%). 1H NMR (CDCl3): δ (ppm) 8.88 (dd, J = 4.3, 1.8 Hz, 4H), 8.06 (dd, J = 8.2, 1.5 Hz, 4H), 7.29–7.40 (m, 12H), 7.02 (dd, J = 6.4, 2.7 Hz, 4H), 4.34 (t, J = 6.6 Hz, 8H), 4.34 (t, J = 6.7 Hz, 8H), 3.24 (t, J = 6.6 Hz, 8H), 2.82 (t, J = 7.2 Hz, 8H), 2.61 (s, 4H), 1.85 (br, 2H). 13C NMR (CDCl3): δ (ppm) 154.2, 148.9, 140.0, 135.5, 129.2, 126.5, 121.3, 119.3, 108.7, 77.2, 67.2, 53.6, 25.4. HRMS (ESI-MS) m/z: [M + Na]+ calcd for C47H46N6O4Na 781.3398; found 781.3478. Anal. calcd for C47H50N6O6 (2c·2H2O): C, 71.01; H, 6.34; N, 10.57. Found: C, 70.77; H, 6.35; N, 10.88.
N,N,N′,N′-Tetrakis(3-(8-quinolyloxy)propyl)propanediamine (8-TQOPPN, 2d)
To an agitated mixture of 8-quinolinol (2.67 g, 18.4 mmol) and powdered potassium hydroxide (1.03 g, 18.4 mmol) in dry ethanol (30 mL) was added N,N,N′,N′-tetrakis(3-chloropropyl)propanediamine (4d) (700 mg, 1.84 mmol) in 3 mL of ethanol. The resulting reaction mixture was refluxed overnight under N2. The resultant solution was cooled to room temperature and the solvent was evaporated. The residue was extracted with CHCl3–water and the organic layer was washed with 3 N NaOH, dried and evaporated. The residue was purified by column chromatography (alumina, AcOEt/MeOH 98/2, Rf = 0.6) to give yellow oil. This material was dissolved in a small portion of ethanol and conc. hydrochloric acid was added to induce precipitation. After filtration, the hydrochloride salt was dissolved in water and 6 N NaOH was added to adjust the pH > 12. The product was extracted into chloroform, dried and evaporated to give compound 2d as a yellow oil (195 mg, 0.239 mmol, 13%). 1H NMR (CDCl3): δ (ppm) 8.90 (dd, J = 4.3, 1.8 Hz, 4H), 8.04 (dd, J = 8.2, 1.8 Hz, 4H), 7.26–7.38 (m, 12H), 6.89 (dd, J = 7.3, 1.5 Hz, 4H), 4.10 (t, J = 6.7 Hz, 8H), 2.57 (br, 8H), 2.40 (br, 4H), 2.06 (quint, J = 6.6 Hz, 8H), 1.54 (br, 2H). 13C NMR (CDCl3): δ (ppm) 154.5, 148.8, 140.0, 135.5, 129.1, 126.4, 121.2, 119.0, 108.4, 66.9, 52.3, 50.1, 26.8, 24.5. HRMS (ESI-MS) m/z: [M + H]+ calcd for C51H55N6O4 815.4286; found 815.4285. Anal. calcd for C51H60ClN2O6.5 (2d·HCl·2.5H2O): C, 68.32; H, 6.75; N, 9.37. Found: C, 68.51; H, 6.35; N, 9.26.
Results and discussion
Ligand synthesis
Compounds 1a and 1b were synthesized from corresponding 8-bromomethylquinolines and ethylenediamine. Ligands 2b–d were synthesized using the procedures outlined in Scheme 1. The final product yields are relatively low due to difficult purifications. No obvious correlations between the chain lengths and yields were observed. All new compounds were characterized by 1H and 13C NMR, and the purity was verified by elemental analysis.
UV-vis and fluorescence spectral changes of 6-MeO-8-TQEN (1b) induced by Cd2+
Since compound 8-TQEN (1a) exhibited poor solubility in common organic solvents, spectroscopic investigations could not be executed. Spectral measurements of 6-MeO-8-TQEN (1b) were performed in DMF–HEPES buffer (1
:
1 (v/v), HEPES buffer contains 50 mM HEPES, 100 mM KCl, pH = 8.0) at 25 °C (Fig. 1). Upon addition of Cd2+, the UV-vis absorption of 1b changed slightly, however, no distinct isosbestic points were detected. Fig. 1b shows the emission changes in 1b after the addition of Cd2+. Upon excitation at 332 nm, apo-1b exhibits negligible fluorescence. After the addition of Cd2+, the fluorescence increases at 395 nm. The fluorescence quantum yield of [Cd(1b)]2+ (ϕ = 0.025) is similar to [Zn(6-MeOTQEN)]2+ (ϕ = 0.032) and larger than [Zn(TQEN)]2+ (ϕ = 0.007). From the fluorescence titration curve shown in Fig. 1c, the dissociation constant of [Cd(1b)]2+ complex was calculated to be Kd = (3.4 ± 0.1) × 10−5 M using nonlinear curve fitting (Fig. S1†).24 The binding affinity is significantly weaker than that of the [Zn(TQEN)]2+ complex (Kd = ∼10−6 M). TQEN binds Cd2+ with higher affinity than Zn2+.23 The reduction in absolute Cd2+ affinity of 1b can be attributed to the formation of 6-membered chelate rings rather than the 5-membered chelate rings in the [Cd(TQEN)]2+ complex. The increase in chelate ring size decreases the binding affinity for large metal ions.31
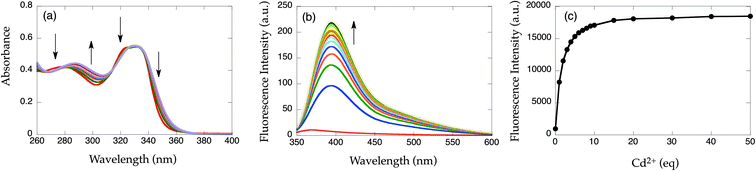 |
| Fig. 1 (a) UV-vis absorption and (b) fluorescence spectra of 34 μM 6-MeO-8-TQEN (1b) (λex = 332 nm) in DMF–HEPES buffer (1 : 1) at 25 °C with Cd2+ between 0 and 1.7 mM. (c) Plot of integrated fluorescence intensity from 350 to 600 nm with increasing [Cd2+]. | |
Fluorescence spectral change of 1b induced by other metal ions
Fig. 2 shows the fluorescence response of 1b to 20 equiv. of various metal ions. The fluorescence enhancement is Cd2+-specific although Zn2+ induces small fluorescence changes at 395 nm (IZn/ICd = 0.35). The lack of a fluorescence response to Pb2+ and Hg2+ enhances the value of 1b as a fluorescent probe for toxic metal ions. As shown in Fig. 2a, the addition of Zn2+ leads to an emission enhancement in the bands at 405 and 490 nm, the latter indicates the formation of an intramolecular excimer.25 Due to this lower-energy fluorescence, the comparison based on the integrated emission is less specific to Cd2+ (FZn/FCd = 0.72). Since many Zn2+ probes respond to Cd2+, we are interested in developing a general probe design strategy to discriminate effectively between the two metal ions. In contrast to TQEN and 6-MeOTQEN, which exhibit a Zn2+-induced fluorescence enhancement of IZn/I0 = 23 (ICd/IZn = 0.64) and IZn/I0 = 11 (ICd/I0 = 14, ICd/IZn = 1.29) respectively, the response of 1b is Cd2+-selective (IZn/I0 = 9, IZn/ICd = 0.35). The fluorescence response suggests that six-membered chelate ring formation allows 1b to accommodate the larger Cd2+ ion. The alkoxy substitution further enhances the emission signal. The binding constant of Zn2+ with 1b was estimated to be Kd = (3.7 ± 0.3) × 10−4 M, which is significantly weaker binding than the Cd2+ binding. This difference is the plausible explanation for the Cd/Zn discrimination ability of 1b.
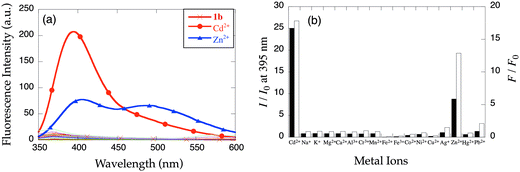 |
| Fig. 2 (a) Fluorescence spectra and (b) the relative fluorescence intensity of 6-MeO-8-TQEN (1b) at 395 nm (I/I0, filled bars) and integrated region from 350 to 600 nm (F/F0, open bars) upon the addition of various metal ions. Emission was recorded with 20 equiv. of metal ion in DMF–HEPES buffer (1 : 1) at 25 °C. I0 and F0 are the emission intensity of free ligand. | |
UV-vis and fluorescence spectral changes of 2a–d induced by Cd2+
Since a larger binding pocket appears to enhance Cd2+-selectivity in 1b, we examined extending the alkyl linkers in 8-TQEN. In 8-TQOPEN (2b), the receptor forms four, six-membered chelate rings upon metal ion binding. The 8-quinolinol fluorophore was chosen to increase the intensity of fluorescence signal and because evidence suggests alkoxy linkers increase Cd2+-selectivity.15 Unfortunately, apo-2b in DMF–H2O (1
:
1) emits intensely (Fig. 3, λex = 321 nm; λem = 408 nm), due to inefficient PET quenching. The inefficient PET is probably related to the distance between the quinoline flurophores and the quenching ethylenediamine nitrogen lone pairs. Metal ions that typically quench fluorescent sensors such as Hg2+, Fe3+, Ag+ and Cu2+ also extinguish the emission of 2b in DMF–H2O (1
:
1).
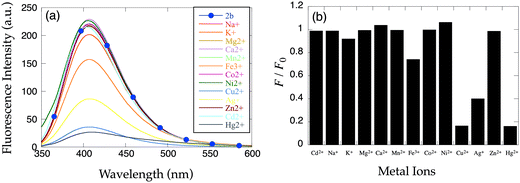 |
| Fig. 3 (a) Fluorescence spectra of 34 μM 8-TQOPEN (2b) (λex = 324 nm) in DMF–H2O (1 : 1) at 25 °C in the presence of 2 equiv. of various metal ions. (b) The relative fluorescence intensity of 2b integrated from 350 to 600 nm upon the addition of 2 equiv. of various metal ions. I0 is the emission intensity of free ligand. | |
To test the hypothesis that linker length leads to inefficient PET quenching in apo-8-TQOPEN (2b), 8-TQOEEN (2a) was prepared. The two probes are identical except 2b has a propylene linkage between the nitrogen atom and the quinoline, while 2a possesses an ethylene spacer. In contrast to the previous report,15 the emission of apo-2a is even greater than that of apo-2b (λex = 321 nm; λem = 409 nm). Upon addition of 2 equiv. of metal ions, only an emission decrease analogous to the one observed with 2b was measured with quenching metal ions (Fig. 4). No significant response to Zn2+ or Cd2+ could be detected with this metal dose (2 equiv.).
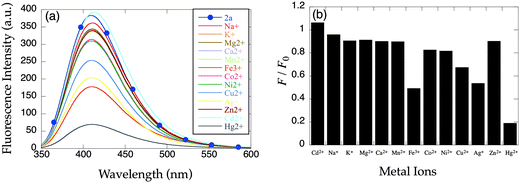 |
| Fig. 4 (a) Fluorescence spectra of 34 μM 8-TQOEEN (2a) (λex = 324 nm) in DMF–H2O (1 : 1) at 25 °C in the presence of 2 equiv. of various metal ions. (b) The relative fluorescence intensity of 2a integrated from 350 to 600 nm upon the addition of 2 equiv. of various metal ions. I0 is the emission intensity of free ligand. | |
To further investigate the impact of six-membered chelate rings on sensor properties, we synthesized the propylenediamine derivatives 8-TQOEPN (2c) and 8-TQOPPN (2d). The propyl linker on the central diamine moiety introduces a six-membered chelate ring at the core of the complex. Although 2d, that possesses four propyleneoxy chains, exhibits considerable basal fluorescence intensity and responds to quenching metal ions like 2a and 2b (Fig. 5), the emission of 2c is reduced compared to the other structurally related derivatives (Fig. 6a). These optical properties suggest that the PET quenching is reasonably efficient. More importantly, 2c fluorescence increases in the presence of 2 equiv. of Cd2+ (Fig. 6b, gray bars) unlike related probes. Although a large excess of Cd2+ can induce a moderate fluorescence enhancement in 2a, the fluorescent intensity of [Cd(2c)]2+ is greater than that of apo-2a, 2b and 2d (Fig. 6b, filled bars). The composite results indicate that a combination of the PET and CHEF mechanisms is responsible for the signaling behavior. Furthermore, alkyl chain length impacts the PET efficiency of the apo-probe and the fluorescent response toward Cd2+.
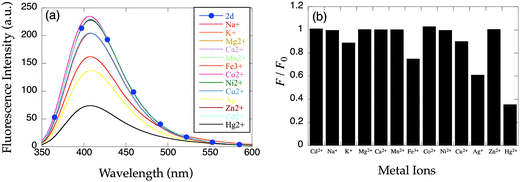 |
| Fig. 5 (a) Fluorescence spectra of 34 μM 8-TQOPPN (2d) (λex = 324 nm) in DMF–H2O (1 : 1) at 25 °C in the presence of 2 equiv. of various metal ions. (b) The relative fluorescence intensity of 2d integrated from 350 to 600 nm upon the addition of 2 equiv. of various metal ions. I0 is the emission intensity of free ligand. | |
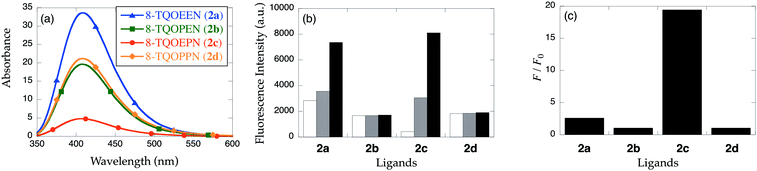 |
| Fig. 6 Comparison of fluorescence properties of 2a–d. (a) Fluorescence spectra of 34 μM 2a–d in DMF–HEPES buffer (1 : 1) at 25 °C in the absence of metal ion. (b) Comparison of fluorescence intensity of 2a–d integrated from 350 to 600 nm in the absence (open bars), presence of 2 equiv. (gray bars), and presence of 20 equiv. of Cd2+ (filled bars). (c) Comparison of fluorescence enhancement factor (F/F0) of 2a–d in the presence of 20 equiv. of Cd2+. F0 is the integrated emission intensity of free ligand. | |
The Cd2+ binding of 2c was investigated further (Fig. 7). Upon excitation at 325 nm, emission at 423 nm increases gradually upon the addition of Cd2+. The fluorescence signal saturates at approximately 20 equiv. of metal ion with 19-fold emission enhancement (Fig. 7c). The fluorescence quantum yield of [Cd(2c)]2+ complex (ϕ = 0.31) is significantly higher than that of [Cd(1b)]2+. Assuming that 2c binds a single Cd2+ ion, the dissociation constant of 2c (Kd = 1.4 ± 0.2 × 10−4 M, Fig. S3†) indicates the lower affinity of 2c than 1b.
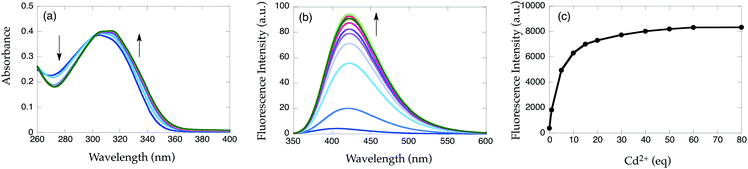 |
| Fig. 7 (a) UV-vis absorption and (b) fluorescence spectra of 34 μM 8-TQOEPN (2c) (λex = 325 nm) in DMF–HEPES buffer (1 : 1) at 25 °C with Cd2+ between 0 and 2.7 mM. (c) Plot of integrated fluorescence intensity from 350 to 600 nm with increasing [Cd2+]. | |
Fluorescence spectral change of 2c induced by other metal ions
The fluorescence enhancement of 2c is Cd2+-specific. While Zn2+ induces a modest response (FZn/F0 = 8.8 and FZn/FCd = 0.45), other metal ions do not modulate the emission (Fig. 8). No long wavelength emission upon Zn2+ binding is detected (Fig. 8a). The ability to discriminate Zn2+ and Cd2+ is comparable to probes with related structures.14,15 The metal ion selectivity profiles of 2c and 1b (Fig. 2) are similar, despite the structural differences. From the Zn2+ titration curve, the binding constant of 2c with Zn2+ was estimated to be Kd = (4.0 ± 0.2) × 10−4 M (Fig. S4†). The binding affinity of 2c for Zn2+ and Cd2+ is nearly identical, although there is a slight preference for Cd2+. Although a slight difference in binding affinity could be partially responsible for the Cd/Zn selectivity of 2c, the fluorescence intensity of fully metalated 2c is metal-ion dependent. The difference in the emission intensity of the Zn2+ and Cd2+ complexes suggests a different coordination environment impacts sensor emission switching mechanism.
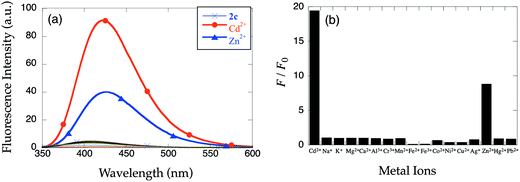 |
| Fig. 8 (a) Fluorescence spectra and (b) the relative fluorescence intensity of 8-TQOEPN (2c) integrated from 350 to 600 nm upon the addition of various metal ions. Emission recorded with 20 equiv. of metal ion in DMF–HEPES buffer (1 : 1) at 25 °C. F0 is the integrated emission intensity of free ligand. | |
pH effect on fluorescence intensity of 1b and 2c
The proton-induced emission changes of 1b and 2c were investigated in aqueous DMF solution (1
:
1) in the presence and absence of 5 equiv. of Cd2+ by adding either HCl or NaOH (Fig. 9). Due to the limited solubility of Cd(OH)2, the complex was formed prior to the addition of NaOH solution by mixing 5 equiv. of CdCl2 and 34 μM of the corresponding sensor. Both compounds show very narrow Cd2+ detection range (pH = 7–9 for 1b and pH = 7–8 for 2c) compared to related probes15 due to the weak metal binding affinity. Protonation of the ligand at the low pH, and the formation of Cd(OH)2 at the high pH leads to demetalation of the sensor. No proton-induced fluorescence enhancement was observed for either 1b or 2c.
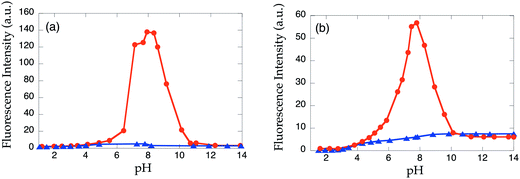 |
| Fig. 9 Effect of pH on fluorescence intensity of 34 μM (a) 6-MeO-8-TQEN (1b) at 395 nm and (b) 8-TQOEPN (2c) at 423 nm in the absence (blue marks) and presence (red marks) of 5 equiv. of Cd2+ in DMF–H2O (1 : 1) at 25 °C. | |
Conclusions
Two fluorescent sensors for Cd2+ inspired by TQEN, 6-MeO-8-TQEN (1b) and 8-TQOEPN (2c), have been developed. From the comparative analysis of 1b and the structurally related sensor 6-MeOTQEN, substituting an 8-quinolyl fluorophore for a 2-quinolyl one provides Cd2+ selectivity because of the receptor can accommodate larger metal ions more efficiently. Introducing 6-methoxy groups enhances the solubility of these compounds and red-shifts the excitation and emission wavelengths compared to TQEN. Of the four alkoxy-8-quinoline derivatives investigated, only 2c exhibited effective Cd2+-induced fluorescence enhancement (ϕCd = 0.31). The other three compounds exhibited moderate fluorescence in their free forms and exhibited minimal responses to Cd2+; however, Hg2+, Ag+ and Cu2+ quench the emission. Since the weak metal binding affinity and narrow pH window for Cd2+ detection for 1b and 2c need to be improved, future work will focus on developing sensors with properties more suitable for cellular imaging using the general design strategies elucidated here.
Acknowledgements
This work was supported by the Research for Promoting Technological Seeds, JST, Adaptable and Seamless Technology Transfer Program through Target-driven R&D, JST, Grant-in Aid for Scientific Research from the MEXT, Japan and the Nara Women's University Intramural Grant for Project Research.
References
- A. P. de Silva, H. Q. N. Gunaratne, T. Gunnlaugsson, A. J. M. Huxley, C. P. McCoy, J. T. Rademacher and T. E. Rice, Chem. Rev., 1997, 97, 1515–1566 CrossRef CAS PubMed.
- A. P. de Silva, D. B. Fox, A. J. M. Huxley, N. D. McClenaghan and J. Roiron, Coord. Chem. Rev., 1999, 185–186, 297–306 CrossRef CAS.
- E. L. Que, D. W. Domaille and C. J. Chang, Chem. Rev., 2008, 108, 1517–1549 CrossRef CAS PubMed.
- L. M. Hyman and K. J. Franz, Coord. Chem. Rev., 2012, 256, 2333–2356 CrossRef CAS PubMed.
- Z. Liu, W. He and Z. Guo, Chem. Soc. Rev., 2013, 42, 1568–1600 RSC.
- R. D. Hancock, Chem. Soc. Rev., 2013, 42, 1500–1524 RSC.
- B. Valeur and I. Leray, Coord. Chem. Rev., 2000, 205, 3–40 CrossRef CAS.
- C. J. Frederickson, E. J. Kasarskis, D. Ringo and R. E. Frederickson, J. Neurosci. Methods, 1987, 20, 91–103 CrossRef CAS.
- M. S. Nasir, C. J. Fahrni, D. A. Suhy, K. J. Kolodsick, C. P. Singer and T. V. O'Halloran, J. Bioinorg. Chem., 1999, 4, 775–783 CAS.
- C. J. Fahrni and T. V. O'Halloran, J. Am. Chem. Soc., 1999, 121, 11448–11458 CrossRef CAS.
- E. Tomat and S. J. Lippard, Curr. Opin. Chem. Biol., 2010, 14, 225–230 CrossRef CAS PubMed.
- X. Peng, J. Du, J. Fan, J. Wang, Y. Wu, J. Zhao, S. Sun and T. Xu, J. Am. Chem. Soc., 2007, 129, 1500–1501 CrossRef CAS PubMed.
- T. Cheng, Y. Xu, S. Zhang, W. Zhu, X. Qian and L. Duan, J. Am. Chem. Soc., 2008, 130, 16160–16161 CrossRef CAS PubMed.
- X.-L. Tang, X.-H. Peng, W. Dou, J. Mao, J.-R. Zheng, W.-W. Qin, W.-S. Liu, J. Chang and X.-J. Yao, Org. Lett., 2008, 10, 3653–3656 CrossRef CAS PubMed.
- Z. Li, P. Xi, L. Huang, G. Xie, Y. Shi, H. Liu, M. Xu, F. Chen and Z. Zeng, Inorg. Chem. Commun., 2011, 14, 1241–1244 CrossRef CAS PubMed.
- L. Xue, G. Li, Q. Liu, H. Wang, X. Liu, C. Ding, S. He and H. Jiang, Inorg. Chem., 2011, 50, 3680–3690 CrossRef CAS PubMed.
- S. Goswami, K. Aich, S. Das, A. K. Das, A. Manna and S. Halder, Analyst, 2013, 138, 1903–1907 RSC.
- A. M. Costero, S. Gil, J. Sanchis, S. Peransí, V. Sanz and J. A. G. Williams, Tetrahedron, 2004, 60, 6327–6334 CrossRef CAS PubMed.
- C. Lu, Z. Xu, J. Cui, R. Zhang and X. Qian, J. Org. Chem., 2007, 72, 3554–3557 CrossRef CAS PubMed.
- X. Zhou, P. Li, Z. Shi, X. Tang, C. Chen and W. Liu, Inorg. Chem., 2012, 51, 9226–9231 CrossRef CAS PubMed.
- Y. Mikata, Y. Sato, S. Takeuchi, Y. Kuroda, H. Konno and S. Iwatsuki, Dalton Trans., 2013, 42, 9688–9698 RSC.
- Y. Mikata, Y. Nodomi, A. Kizu and H. Konno, Dalton Trans., 2014, 43, 1684–1690 RSC.
- Y. Mikata, M. Wakamatsu and S. Yano, Dalton Trans., 2005, 545–550 RSC.
- Y. Mikata, M. Wakamatsu, A. Kawamura, N. Yamanaka, S. Yano, A. Odani, K. Morihiro and S. Tamotsu, Inorg. Chem., 2006, 45, 9262–9268 CrossRef CAS PubMed.
- Y. Mikata, A. Yamanaka, A. Yamashita and S. Yano, Inorg. Chem., 2008, 47, 7295–7301 CrossRef CAS PubMed.
- Y. Mikata, K. Kawata, S. Iwatsuki and H. Konno, Inorg. Chem., 2012, 51, 1859–1865 CrossRef CAS PubMed.
- G. Weber, J. Riecke, W. Saenger and G. M. Sheldrick, Inorg. Chim. Acta, 1981, 54, L185–L186 CrossRef CAS.
- R. Bartzatt and L. Donigan, Lett. Drug Des. Discovery, 2004, 1, 78–83 CrossRef CAS.
- Y. Sakurai and M. Izumi, Pharm. Bull., 1953, 1, 297–301 CrossRef CAS.
- K. Schlögl and R. Schlögl, Monatsh. Chem., 1964, 95, 922–941 CrossRef.
- W. Gan, S. B. Jones, J. H. Reibenspies and R. D. Hancock, Inorg. Chim. Acta, 2005, 358, 3958–3966 CrossRef CAS PubMed.
Footnote |
† Electronic supplementary information (ESI) available: Experimental details for preparation of new compounds, characterization data and Fig. S1–S26. See DOI: 10.1039/c4ra00010b |
|
This journal is © The Royal Society of Chemistry 2014 |
Click here to see how this site uses Cookies. View our privacy policy here.