Antimony mobility in soils: current understanding and future research directions
Received
2nd December 2024
, Accepted 24th February 2025
First published on 25th February 2025
Abstract
Antimony (Sb) has gained increased attention over the past few decades due to its possible detrimental effects on biota and its potential to leach and disperse from contaminated soils. The fate of Sb in the environment is largely controlled by its chemical speciation, as well as the speciation of solid phases (e.g. Mn/Fe-oxyhydroxides) that interact with Sb in soils. Microbes have the capacity to facilitate a multitude of oxidation and reduction reactions in soils. Therefore, they exert control over the reactivity of Sb in the environment, either directly and/or indirectly, by changing Sb speciation and/or affecting the redox state of soil solid phases. Here, we outline processes that determine the behaviour of Sb in soils. We conclude that based on laboratory studies there is a good theoretical understanding of pure soil components interacting with Sb species. However, comparatively little is known concerning the contribution of these interactions in complex natural systems that are dynamic in terms of biogeochemical conditions and that can hardly be simulated using laboratory incubations. We note that important biochemical foundations of microbially driven Sb conversions (i.e. molecular constraints on organisms, genes and enzymes involved) have emerged recently. Again, these are based on laboratory incubations and investigations in environments high in Sb. In this regard, an important remaining question is which microorganisms actively impact Sb speciation under real-world conditions, in particular where Sb concentrations are low. Multiple dissolved Sb species have been described in the literature. We note that more analytical development is needed to identify and quantify possible key Sb species in natural systems, as well as anthropogenically impacted environments with only moderate Sb concentrations. With these research needs addressed, we believe that the Sb fate in the environment can be more accurately assessed, and remediation options can be developed.
Environmental significance
Sb has gained significant attention due to its potential to cause harm to ecosystems and human health, especially in contaminated soils. Sb, both naturally occurring and introduced through anthropogenic activities such as mining, industrial processes, and military operations, can migrate through the environment, posing risks to water quality and biodiversity. Its mobility and toxicity are largely controlled by its chemical speciation and interactions with various soil components, including microbial communities. However, much of the existing research has been based on simplified laboratory conditions, and there remains a gap of Sb behavior under dynamic, real-world environmental conditions. This review highlights the current state of knowledge on Sb biogeochemistry and identifies key research areas essential for enhancing environmental management and remediation efforts.
|
1. Introduction
Antimony (Sb) is a naturally occurring element with metallic and non-metallic characteristics. In its elemental form, it is a white, brittle, crystalline metalloid positioned within group 15 of the periodic table, with an atomic number of 51 and an atomic mass of 122.1 Sb is relatively rare in the Earth's crust, with concentrations ranging from 0.2 μg g−1 to 0.3 μg g−1.2 In the natural environment, Sb is often associated with sulphur (e.g. Sb2S3, stibnite) or oxygen (e.g. Sb2O3, valentinite).2 Sb can be found at elevated concentrations in anthropogenically impacted soils and sediments because of metal mining, military training, smelting and pharmaceutical and pesticide manufacturing.2 The world's Sb reserves amount to 1.8 million tonnes, and are concentrated in China (48%), Russia (18%) and Bolivia (16%).3 Due to its economic importance and potential risks and vulnerabilities related to future supply, the European Commission included Sb in its latest critical raw material list.4
The use of Sb can be categorised into three main domains: metal production applications, non-metal applications, and as a crucial component in flame-retardant materials. The largest share (43%) of Sb is utilised in flame retardants.4 Here, antimony trioxide (Sb2O3) is used because of its synergistic capacity to interact with halogenated compounds for effective flame suppression.5 Most of the metallic Sb is used as a hardening agent in lead (Pb) alloys, such as Pb electrodes in Pb-acid batteries [32% of total,4], as well as in lead bullets used in small-arms shooting ranges.6 Such bullets typically contain Sb at concentration levels between 2% and 8%,7 so large amounts of Sb enter the pedosphere because of shooting activities. In addition to its established role as a catalyst in the plastic and ceramics industry, emerging applications of Sb include its use in data storage technologies and as a component in semiconductors, including those used in photovoltaics.8 In the pharmaceutical/medical industry, Sb-containing medications serve as emetics and laxatives.9
In natural systems, Sb occurs mostly in four oxidation states: (−III), (0), (III) and (V). Sb(−III) and Sb(0) may be found, for instance, in solid phases, such as in ores (as sulphides or native Sb). In the aqueous phase, Sb(III) occurs as water-soluble Sb(OH)3 across a relatively large pH range (from very acidic to alkaline) in anoxic environments10 (Fig. 1). In contrast, Sb(V) can form water-soluble Sb(OH)6−, which is predominant in more oxic environments across a large pH spectrum.10 Various processes drive the transformation of natural Sb, including oxidation,11–14 reduction15–18 and methylation reaction.19,20 In most of these reactions, microorganisms are the primary agents that facilitate Sb turnover.21–24 However, information on microbial identity (strains and community composition) and the enzymatic pathways involved is rare.
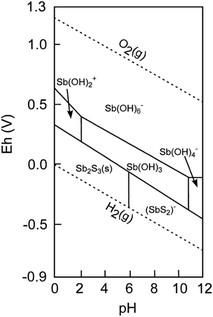 |
| Fig. 1 Eh–pH diagram of antimony in the Sb–S–H2O system at a dissolved antimony concentration of 10−8 mol L−1 and a dissolved sulfur concentration of 10−3 mol L−1 (reproduced from Filella et al., 2002; with permission from Elsevier).10 | |
Considering the potential detrimental effects of Sb on human health, and in the context of stringent environmental protection regulations, it is of utmost importance to understand the processes underlying the mobilisation and immobilisation of Sb in the environment. In this review, we present the fundamental biogeochemical mechanisms that underlie the possible transformation of Sb in the environment. We review existing knowledge of possible redox reactions with common soil phases [Fe/Mn oxyhydroxides, clay minerals, soil organic matter and dissolved sulphide]. We elucidate the complex interplay between these phases, Sb and soil microbiota, as well as the interactive effects involving plants. With this, we identify knowledge gaps that need to be addressed to transfer our solid fundamental understanding of simplified, static laboratory systems to complex, dynamic natural environments. Addressing them will lay the basis for successful remediation measures in Sb-contaminated ecosystems in the future.
1.1. Detrimental effects of antimony
Despite beneficial uses (e.g. treatment of parasitic disease leishmaniasis), there are several adverse health effects documented following exposure due to oral ingestion, inhalation or dermal contact with Sb. These include respiratory, myocardial, gastrointestinal, developmental and metabolic (impact on blood glucose levels) effects25 as well as genotoxicity26 which has been the focus of several reviews (e.g. ref. 27 and 28). Besides the negative impact on human health, wildlife toxicity of antimony has been documented.29 Due to its possible adverse effects and widespread occurrence, Sb has been classified as a priority pollutant by the US Environmental Protection Agency (USEPA);30 that is, it belongs to the group of substances that are identified as being particularly harmful to human health and the environment, and that require careful monitoring and management to mitigate their detrimental effects. According to the USEPA, both the maximum contamination level goal and the maximum contaminant level are set at 0.006 mg L−1.31 The European Union established a threshold of 0.01 mg L−1 Sb in drinking water.32,33 According to the World Health Organization, the guideline value for Sb in drinking water is 0.02 mg L−1.34
Several geogenic sources contribute to global Sb emissions in the order of wind-borne dust < volcanic activity < sea salt spray < biogenic sources < forest fires.35 A distinct rise of Sb in arctic ice cores starting approximately 900 years ago suggests that anthropogenic sources were contributing to atmospheric Sb emissions already in pre-industrial times.36 Nowadays, anthropogenic activities result in locally and/or regionally elevated Sb concentrations in freshwaters, soils and sediments. For instance, Sb contamination in China has been related to mining activities, smelting and coal burning.37,38 In other countries that do not mine or refine primary Sb, the possible origins of locally elevated Sb concentrations include shooting activities. In Switzerland, for instance, up to ∼25 tons of Sb enter the pedosphere each year because of shooting activities.39,40 As a result, target areas of shooting ranges are often heavily contaminated with high concentrations Sb and thus require remediation. In “moderately” contaminated soils (i.e., those with increased Sb concentrations compared to the background), studying the fate of Sb is particularly relevant, as such soils may not require remediation under current laws. Various mechanisms exist that act to mobilise Sb, increasing the likelihood of dispersal in the environment. This can pose severe threats to ecosystems, potentially affecting humans through food chain exposure.41
1.2. Geochemistry of antimony
As is also the case for other elements, the ‘chemical speciation’ determines the fate of Sb. Speciation refers to the specific forms of an element defined by its isotopic composition, electronic or oxidation state and/or complex or molecular structure.42 Temperature, pH, redox potential (Eh) and Sb concentrations are the key factors that determine the ‘expected’ (i.e. thermodynamically favoured) Sb speciation and thus its solubility and mobility in soils. In the environment, thermodynamic equilibrium is often not reached; thus, several Sb species are found at once.10 Sb exhibits a complex chemistry in the environment, which has been reviewed in several publications43,44 and is only briefly presented here.
In general, geochemical processes that control Sb mobility in soils or sediments are sorption–desorption reactions, Sb-mineral phase formation and dissolution and redox transformations. Furthermore, chelation by biogenic compounds, as well as biologically mediated alkylation reactions, can occur. In nature, Sb may be associated with oxygen and hydroxide, resulting in oxides, hydroxides or oxyanions, either in the pentavalent oxidation state or in the trivalent oxidation state under more reduced conditions. Solid Sb(OH)3 is not known to occur naturally, and the common convention is to represent the hydrolytic species of Sb(III) as hydroxide complexes, while the representation as oxyanions is also common.45 Pentavalent antimony is presented as Sb(OH)6− and is the predominant form of Sb in the aqueous phases under oxidising conditions (Fig. 1).10 Furthermore, Sb associates with sulphur, forming either insoluble (e.g. stibnite, Sb2S3) or soluble sulphides (mostly anionic Sb–S complexes46) (Fig. 2). Sb can also undergo biologically catalysed alkylation, leading to the formation of volatile Sb species, such as monomethyl Sb, dimethyl Sb and trimethyl Sb, in the environment.19 Biomethylation has long been suggested as a mechanism for the detoxification of different elements.19 Sb methylation appears to be a ubiquitous process in terms of organisms involved (fungi, archaea and bacteria), as well as environments, where methylated Sb compounds were detected (e.g. soil, sediment, sewage, freshwater, seawater and landfill gas).19
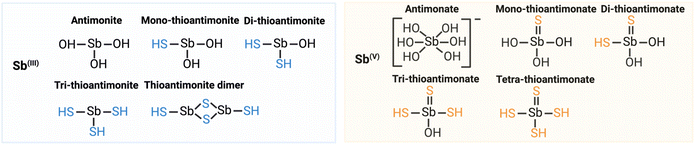 |
| Fig. 2 Thioantimony transformation cycle in the environment. Adapted from (Ye & Jing, 2021).47 | |
The environmental interactions between Sb and mineral phases and/or organic matter particles have been intensively researched and are briefly summarised below. Notably, Fe and Mn (hydr)oxides are well-known sorbents, as well as redox-catalysts, for Sb,48–51 and soil organic matter (SOM) is recognised as an important complexing agent for Sb.16,46,52,53 Possible interactions with other mineral phases, such as clay minerals or sulphides, have been acknowledged but have received much less attention in previous studies.54,55
1.2.1. Reaction with iron (hydr-)oxides.
In natural soils and sediments, both crystalline minerals and amorphous iron (hydr)oxide minerals can exist as e.g. hematite (Fe2O3), ferrihydrite [(Fe10O14(OH)2)], goethite (α-FeOOH) and magnetite (Fe3O4), which all represent possible sorbents for Sb.56–58 Many studies have quantitatively and mechanistically studied the interaction of Sb species with such phases.59–62 Fe (hydr)oxides have amphoteric properties and can steadily form complexes with cations and anions. Accordingly, one may expect that pentavalent Sb [being present as anionic Sb(OH)6− across the entire pH range; [see Fig. 1] will exhibit some sorption at pH values below the point of zero charge (PZC) of the respective iron (hydr-)oxide. Indeed, nearly complete Sb(V) sorption was observed, for example, on goethite at pH < 7 (average reported PZC = ∼8;63). However, assessing the mobility of Sb is not simply a question of the ionic sorption of Sb species on pure mineral phases. For instance, in the natural environment, neither Sb(III) nor Sb(V) will occur as the only dissolved species but will be present together with other ions; hence, competition for sorption sites on Fe oxyhydroxides is likely (e.g. competition with the arsenite oxyanion64). Furthermore, in simultaneous adsorption experiments with ferrihydrite, Sb(III) adsorption was not affected by the presence of Sb(V), while Sb(III) had an inhibitory effect on the adsorption of Sb(V).65 This study points to another complication in the context of assessing Sb mobility: Sb species are prone to undergo redox reactions upon contact with certain solid surfaces. Ferrihydrite, for instance, can catalyse the oxidation of Sb(III) to Sb(V), which, in turn, influences Sb adsorption.65 The oxidation of Sb(III) has also been shown, for instance, on goethite49 and hydrous ferric oxide.66 On the other hand, the reduction of Sb(V) has been demonstrated, for example, on mackinawite and magnetite (Kirsch et al. 2008),60 as well as “green rust” [various redox active Fe(II)/Fe(III) hydroxides].44,67 Recently, Peiffer et al. emphasised the universal importance of such redox-active mineral phases for the fate of contaminants.68 Metastable mineral phases – including those of Fe (e.g. ferrihydrite or goethite; eventually transforming to haematite or magnetite) – are considered highly reactive surfaces, delivering or consuming electrons in redox reactions. Redox-active mineral phases obtain their specific redox-catalytic properties upon interaction with other redox-active constituents in solution and require activation by fluctuating redox conditions to maintain their high reactivity (Peiffer et al. 2021). The corresponding redox reactions of Sb often occur fast (i.e. from hours to a few days),48,65 thus controlling Sb mobility on diurnal or weekly time scales (e.g. upon periodic flooding and waterlogging after rain). This is certainly a challenge in understanding the mobility of Sb (and other redox-active elements such as As, Se and Te) in the environment. Moreover, the reaction with the Fe phases represents just one of the many possible reactions that Sb can undergo in the environment. Nevertheless, it is crucial to understand the reactivity of pure Fe phases with Sb, considering that such phases may be applied in natural environments for in situ remediation to decrease metal mobility in general (e.g. ref. 69), and specifically for Sb(V) and Pb from shooting range activities.70,71
1.2.2. Reaction with manganese (hydr)oxides.
Many Mn oxide/hydroxide phases are known to exist near the Earth's surface72 yet birnessite, pyrolusite, manganite and amorphous Mn oxides represent some of the most common solid Mn(III/V) phases in soils. Sorption of Sb(III) and Sb(V) has been studied using several of such pure Mn phases. Sb(V) sorption on birnessite,73 pyrolusite and manganite74 showed the typical behaviour of an anionic species, with increased sorption with decreasing pH. The high adsorptive capacity of Mn oxides has been exploited in several functional materials (e.g. nanofibers of α-MnO2, biogenic manganese oxides or Fe-doped birnessite) for Sb removal from waters.75 When Sb(III) is brought into contact with solid manganese oxides, it gets oxidised due to the high oxidative power of these mineral phases. Reduced Mn(II)/(III) is either incorporated into the remaining solid or released as dissolved Mn2+, as shown for several phases76,77.48 showed a more efficient oxidation of Sb(III) by Mn oxides compared to Fe oxides, with the oxidation completed within a few days. Even faster oxidation of Sb(III) was observed on birnessite on a timescale of minutes by.78 The oxidation capacity of Mn oxides seems to be positively correlated with the Mn(III) content and surface-adsorbed oxygen but appears to be negatively correlated with its level of crystallinity.76,79 More precisely, it was demonstrated that the edge sites of birnessite represent key loci of Sb(III) oxidation and that, in turn, poorly crystalline birnessite exhibits higher oxidation and sorption capacities when compared with crystalline birnessite due to the availability of these edge sites.76
During periods of waterlogging, soils can become anoxic, and solid Mn(IV) oxides can undergo reductive dissolution reactions, either through abiotic interactions with reducing compounds or microbial dissimilatory Mn reduction.80 When such reduced soil solutions become oxidised again, solid Mn phases form upon dissolved Mn2+ oxidation. The products can be either amorphous or, to some degree, crystalline (mostly in the form of birnessite, rhodochrosite or bixbyite81), which impacts their sorption capacity.82 During redox transition periods, dissolved reduced Mn2+ and solid oxidised Mn may coexist (i.e. during the transition from reducing to oxidising conditions, and vice versa). The interaction with Mn2+ affects Mn-oxide phase transformations and thus impacts Sb mobility indirectly. For instance, Sb(V)-bearing birnessite can undergo rapid (<1 day) transformation at circumneutral pH to manganite and hausmannite in the presence of Mn2+, effectively sequestering Sb.83 In contrast, the absence of Mn2+ did not result in phase transformation but in the release of Sb(V) to the aqueous phase.83
In the environment, the oxidation of dissolved Mn to form particulate Mn phases is mainly microbially driven (e.g. see the review by ref. 84 and 85), and the products are thus of a ‘biogenic’ nature. Biogenic Mn oxides appear to display a considerably (sometimes orders of magnitude) higher sorption potential than their ‘synthetic’ counterparts. For instance,86 demonstrated that commercially available crystalline MnO2 minerals showed a Pb sorption capacity between ∼0.01 and 1 mmol Pb per mol Mn, whereas MnO2 that was produced through microbial Mn2+ oxidation by Leptothrix displayed a much higher sorption capacity of around 550 mmol of Pb per mol Mn. This effect also seems to be pertinent to Sb sorption.87 In addition,86 demonstrated that sorption capacity is not constant over time. For example, as fresh synthetic Mn oxide phases age, their sorption capacity decreases.86 Therefore, studies that rely solely on experiments with commercially chemically synthesised Mn phases that have possibly undergone ageing may not fully capture the true potential of these phase sorbents for Sb in natural environments. In soils and sediments prone to waterlogging and/or fluctuating redox conditions, complex redox reactions involving Mn and Sb will determine the mobility and speciation of Sb to a large extent. The impact of the biogenic origin and ageing of MnO2 phases on the sorption of Sb remains to be systematically studied.
1.2.3. Association with clay minerals.
Clay minerals play important roles in soil structure and function. They directly influence the availability of plant nutrients, control water retention/storage and contribute to the structural stability of soils.88,89 Sb mobility can be affected by the clay mineral content in soils, primarily due to their high surface area with abundant sorption sites. Certain phyllosilicates (for instance smectite- and montmorillonite-type clays) are well known for their capacity to sorb cations. This is because isomorphic substitution can occur,90 which gives these minerals a permanent negative charge under most soil pH conditions. Therefore, next to sorption on the external plane, cations can sorb as interlayer complexes as well.91 Considering that dissolved Sb(V) occurs mostly as a charged anion, one can expect repulsion, and thus little retention on such phyllosilicates. Indeed, montmorillonite shows the lowest sorption affinity for Sb(V) in the sequence of montmorillonite < δ-MnO2 < ferrihydrite at pH 5.5.92 Not all phyllosilicates undergo extensive isomorphic substitution. Kaolinite, for instance, a common variable-charge clay mineral, has a low capacity to adsorb cations, both because of little isomorphic substitution and due to its comparatively low surface area.89 Indeed, Sb(V) sorption on kaolinite is high at a low pH.93 Unlike anionic Sb(V), trivalent Sb(III) remains neutral over a broad pH range (Fig. 1) and should therefore be less susceptible to ion exchange reactions on positively charged minerals. However, only a handful of studies actually investigated Sb(III) sorption on clay minerals and other Al-rich phases. An early study by94 investigated Sb(III) sorption on different synthetic hydrous oxides, showing that trace concentrations of Sb(III) can effectively be sorbed by the synthesised AI(OH)3 gel at a pH of 6–7. Later,95 compared the sorption of Sb(III) and Sb(V) by bentonite.94 The bentonite used had a low PZC of 2.6 due to isomorphic substitution, confirming the greater adsorption of neutral Sb(III) compared to anionic Sb(V) at pH 6. Similarly,96 observed greater sorption of Sb(III) compared to Sb(V) on kaolinite at a similar pH (pH = 5.5). Additionally, they found that Sb(V) sorption was enhanced at pH 5.5 compared to pH 8.0. Besides this expected general behaviour of Sb, further interesting observations could be made:95 noted some desorption of Sb following Sb(III) adsorption, and they assigned this to the oxidation of Sb(III) on the bentonite surface and subsequent desorption of Sb(V).96 used oxidised and reduced nontronite, an Fe-containing phyllosilicate, and found that for nontronite in the reduced state, sorption capacity towards Sb(III) was slightly decreased, whereas Sb(V) uptake was dramatically increased compared to oxidised nontronite. A certain Fe content in natural clay minerals is very common (as structural Fe in the lattice of clays, in the interlayer space and/or adsorbed on the external surface), and this Fe can cycle between its Fe(II) and Fe(III) oxidation states.97 Therefore, one may speculate that Fe-containing clays can also act as redox catalysts similar to Fe oxyhydroxides (see above in Section 1.2.3). Despite this possible role, it is fair to say that, in contrast to the Fe and Mn solid phases, these reactions have remained almost entirely unstudied thus far.
1.2.4. Interaction of Sb with soil organic matter.
Soil organic matter (SOM) comprises components from the breakdown of plant materials, faunal remains and microbial biomass, consisting of humic acids (HA), fulvic acids (FA) and humins.98 In addition to its generally important role in the context of nutrient supply, soil structure, water storage and carbon sequestration, SOM also influences the biogeochemical cycle of Sb in different ways. SOM promotes electron flow and can serve as an electron donor and/or electron shuttle for microbial respiration in anaerobic environments, either reducing Sb(V) to Sb(III)99 or reducing Sb-bearing solid phases of e.g. Fe100,101 and Mn.102 SOM may also interact with Sb through complexation and binding. In general, Sb reactivity with natural organic matter will depend on the type of organic matter (HA, FA or humins) and the type of reactive functional groups present. It has been shown that the decreased availability of Sb upon the ‘ageing’ of soils may be largely determined by Sb binding to organic matter.103 The interaction between SOM and Sb will depend on both Sb speciation and the presence of certain functional groups: cationic species (such as Sb(III) in the form of SbOH2+, Fig. 1) may interact with carboxylate functional groups.104 Anionic Sb species [such as Sb(V) in the form of Sb(OH)6−] might involve amine-type functional groups.105 For neutral species [such as Sb(III) in the form of Sb(OH)3], the presence of thiol moieties appears to impact Sb retention. In peatlands, Sb(III) may complex with carboxylic or phenol groups when the thiol content is low. However, under high-thiol conditions, Sb(III) sorption to SOM and thiol groups tends to increase.10,46 Under highly acidic conditions (pH < 2), there is notable binding between both Sb species and SOM, with Sb(V) displaying a stronger preference over Sb(III) (present in cationic form, whereas Sb(V) is partially present as an anion).105,106 In contrast, under near-neutral conditions (pH > 4), the affinity to complex Sb(V) is reduced, which can be attributed to the negative charge of Sb(V) and SOM at neutral pH.105,106 In soil solutions, the disposition and availability of dissolved organic matter (DOM) determines the fate of Sb.46,99 In this regard, complexation of Sb with DOM has been shown to increase Sb mobility.99 Fan et al. evaluated the effects of DOM on the sorption of Sb(V) in soils, and demonstrated that the sorption of Sb(V) can be considerably reduced in soil regions where DOM has been removed experimentally (by extraction with CaCl2 solution; see ref. 107). The ability of Sb to form complexes with smaller carboxylic organic acids, potentially from biogenic sources in soils, is well established (e.g. in the form of citric acid;108) and may increase Sb mobility as well.
In summary, a plethora of possible interactions between Sb and SOM exist, and a generally valid statement about whether SOM increases or decreases Sb mobility and bioavailability in soils seems almost impossible. Several fundamental reactions between SOM and Sb species have been quantitatively and spectroscopically examined. However, considering that SOM in healthy soils is continuously synthesised and decomposed,109 it is crucial to deepen our understanding of these dynamic interactions.
1.2.5. Formation of dissolved thioantimony compounds.
Recently, particularly in sulphide-rich environments, such as geothermal systems, the natural formation of thioantimony (Sb–S) species has been noted. As a chalcophile element, the geochemistry of Sb is often coupled with the cycling of sulphur (S). A good example in this context is the formation of stibnite (Sb2S3), the primary Sb ore on Earth.43,110 Dissolved inorganic thioantimony compounds are formed by formally replacing the hydroxyl groups in Sb(III) and Sb(V) oxyanions with sulphydryl groups, thus generating thioantimonates and/or thioantimonites (Fig. 2).111,112 The natural occurrence of Sb(V)–S species was reported for geothermal waters, hot springs and sulphidic landfills.55,113–115 Sb(III)–S species were, for instance, formed during the dissolution of Sb2S3 in a sulphide solution.116,117 This may occur in the environment, when the sulphide solution is of biogenic nature.118 However, in natural systems Sb(III)–S species are hard to detect due to inherent challenges linked to their analysis in real matrices. On the one hand, Sb(III)–S species are redox labile55,119 rendering sample preparation/conservation problematic. On the other hand, when using hyphenated techniques such as Liquid Chromatography Inductively Coupled Plasma Mass Spectrometry (LC-ICP-MS) for quantifying individual species, the chromatographic separation of multiple co-existing Sb–S species is difficult.
In terms of mobility, the repulsion of typically negatively charged thioantimonates and thioantimonites by negatively charged mineral (hydr)oxides of Fe and Mn may generally enhance overall Sb mobility in sulphide-rich natural environments.120 Limited information, however, is available on the occurrence of thioantimony in natural waters outside of geothermal and hot springs (see ref. 47 for more details). Future research should therefore focus on exploring the potential existence and fate of thioantimonates and/or thioantimonites in other ecosystems containing reduced sulphur. Considering the widespread presence and activity of sulphate-reducing bacteria that produce biogenic sulphide,121 one can expect that such Sb–S species will be discovered in Sb contaminated anoxic environments once the analytical challenges associated with the conservation of their speciation and quantification of individual species during analysis are addressed.
1.3. Role of microbial dissimilatory iron reduction in Sb mobility
The reduction of Fe(III) in the environment is to a large degree catalysed by Fe(III)-reducing microorganisms. This trait is phylogenetically widespread among bacteria and archaea and can occur by two different metabolic mechanisms: dissimilatory Fe-reducing microorganisms are strict or facultative anaerobes that conserve energy from the electron transfer to Fe(III) at their respiratory chain. Others, mostly fermenting bacteria, can dump electrons onto Fe(III) independently of a respiratory chain and improve thereby their growth yield.100,122 When soils become anoxic due to water saturation Fe minerals may undergo reductive dissolution promoted by the activity of Fe(III)-reducing microorganisms, potentially releasing previously adsorbed metals and metalloids.53 In this regard, some studies have used pure strains of Fe-reducing bacteria to demonstrate the mobilisation of Sb to the aqueous phase (e.g. ref. 123). Other studies involving natural soils and sediments and their associated microbial consortia have observed that the release of dissolved Fe and Sb is closely linked. For instance,124 could relate the release of Sb and Fe from different paddy soils of the Xikuangshan Sb mine (China) in simulated flooding conditions.125 showed that Sb(III) formed as a result of the microbial reduction of Sb, which was first sorbed on iron (hydr)oxides before it was released as a result of reductive Fe dissolution in a shooting range soil.
While there seems to be some potential for increased Sb mobility under Fe-reducing conditions, evidence exists that the opposite may also be true. Microbial Fe(III) reduction can cause phase transformations and/or secondary mineral formation in/from metastable iron oxides. For instance, traces of Fe2+ can induce the rapid transformation of ferrihydrite into more stable forms such as goethite, lepidocrocite and magnetite.126–128 All these phases have a different metal-sorption capacity and a differential tendency to incorporate elements in their mineral structure compared to ferrihydrite. Thus, the transformation of metastable Fe phases into more stable phases can influence the mobility of the elements originally associated. For instance, using Shewanella putrefaciens123 showed that Fe(II) can catalyse the rapid transformation of Sb(V)-bearing ferrihydrite to feroxyhyte and goethite, incorporating Sb(V) via substitution for Fe(III). Moreover, a study on the microbial reduction of Sb(V)-bearing ferrihydrite by Geobacter sulfurreducens129 demonstrated microbially induced phase Fe transformations immobilising Sb. While, in general, microbial control over Fe cycling is very well understood (e.g. ref. 130), the information on the impact of microbial Fe reduction on Sb mobilisation is still limited. The question of whether microbial Fe reduction ultimately results in increased or decreased mobility of Sb in nature cannot be fully answered to date.
1.4. Role of dissimilatory manganese reduction in Sb mobilisation
The reduction of Mn(IV) and Mn(III) in soils and freshwater sediments is primarily catalysed by microorganisms. As for Fe(III), Mn(IV) reduction is linked to the anaerobic oxidation of organic carbon100 and Mn(IV) can serve as the terminal electron acceptor for dissimilatory reduction. Various microorganisms possess the capacity to reduce Mn oxides to support their growth (see e.g. ref. 131–133), and many microbes that can reduce Fe(III) can also reduce Mn(IV), and vice versa. Since Mn phases represent potent sorbents for Sb (see Section 1.2.2), their reductive dissolution may result in Sb mobilisation. For instance, column experiments using moderately contaminated shooting range soils showed that Sb(V) and Mn2+ effluent concentrations were correlated, providing evidence that Sb(V) was associated with these phases and suggesting that Sb was released by reductive dissolution.57 Similarly, using an experimental setup with controlled redox conditions that suppress Fe and sulphate reduction134 showed that the reductive dissolution of Sb-hosting Mn phases conferred Sb release. A relatively large number of studies exist that exploit the potential of Mn phases as sorbents and/or oxidants for Sb(III) (see Section 1.2.2). Only a few fundamental studies, however, have investigated the interplay among Mn oxyhydroxides, Mn dissimilatory microbial activity, and its impact on Sb mobilisation, despite the evidence that this may control the Sb fate in soils and sediments to a large degree.
2. Microbially catalysed redox reactions of Sb compounds
Microbial transformations directly involving Sb compounds can be broadly classified into oxidative and reductive reactions including the reduction of Sb(V) as well as hydride generation and alkylation reactions, see ref. 19. Since the first isolations back in the 1970s135 there are currently well over 70 Sb oxidisers known.136 Sb oxidisers can be divided into chemoautotrophs and heterotrophs. Chemoautotrophic oxidisers conserve energy from the oxidation of Sb(III) to Sb(V) and use CO2 as a carbon source for biomass formation. Some examples of isolated pure cultures include Variovax paradoxus IDSBO-4, Sulfobacillus sp., Leptospirillum sp., Ferroplasma sp., Sulfobacillus thermotolerans Sb–K, Sulfobacillus sibiricus Sb–F and Sulfobacillus thermosulfidooxidans.11,12,137 Heterotrophic Sb(III) oxidisers cannot conserve energy from this reaction and use organic compounds (e.g. tartrate;11) as the carbon and energy source. Here, the oxidation of Sb(III) may represent a detoxification mechanism. Most isolated Sb oxidisers known so far use O2 as a terminal electron acceptor (Biswas and Sarkar 2022), although nitrate appears to be used in some environments as well.138
As and Sb are both elements of group 15 of the periodic table, and hence share some chemical properties, such as similar oxidation states and the tendency to associate with oxygen and sulphur in nature. This suggests that the two elements also share similar biochemistries.139 recently pointed out, however, that the biochemistry of As and Sb may be very distinct. Still, it has been observed that some Sb-oxidising strains can concomitantly oxidise As, whereas others cannot, which has implications regarding their proposed use in bioremediation.140 The autotroph V. paradoxus can concurrently oxidise both Sb(III) and As(III), whereas in the heterotroph Hydrogenophaga taeniospiralis, As(III) inhibits Sb(III) oxidation.11 Arsenic and Sb often co-occur in environments contaminated by mining activities.141,142 Here, microbial Sb(III) oxidation may thus be competitively hindered by the simultaneous presence of As; thus, the proposed bioremediation attempts using organisms such as Hydrogenophaga taeniospiralis might be unsuccessful in mitigating Sb contamination.
In contrast to microbial oxidation, the isolation of microorganisms that mediate the reduction of Sb(V) to Sb(III) is comparatively recent. The first reports of dissimilatory Sb(V)-reducing bacteria (DSbRB) date only from 10 years ago.143 This is surprising, considering that the dissimilatory reduction of other metals and metalloids (such as As) has been known for a long time.144–146 DSbRB use Sb(V) as the final electron acceptor in its respiratory chain to conserve energy. The electron donors can be organic, but H2 as the sole inorganic electron donor is also possible.17
Interestingly, Yamamura et al. showed that certain DSbRB can only reduce Sb(V) – but not As(V) – underlining the biochemical differences between the two elements in the context of reductive reactions.18 For arsenate, many resistant microorganisms are known to reduce As(V) for detoxification, not conserving energy (as opposed to dissimilatory reduction).147 Whether such detox mechanisms, independent from energy conservation, may also exist for Sb(V) is not yet known.
Several studies have shown that the final product of Sb reduction is an Sb oxide, such as Sb trioxide (Sb2O3).17,18,143,148 Given the low solubility of Sb2O3,149 only minor amounts of Sb(III) will remain in aqueous solution. However, in sulphidic environments, the formation of dissolved thioantimony compounds (see Section 1.2.5) and/or precipitation as stibnite (Sb2S3) can occur.16,150–152 Sb2S3 is even less soluble in water compared to Sb2O3, resulting in significantly reduced dissolved Sb concentrations.153 Thus, the composition of the growth medium (i.e. presence or absence of sulphide) determines the final product of microbial Sb reduction in laboratory experiments. Whether other components of common buffered growth media (i.e. phosphate or carbonate) impact the final solid product formed in buffered laboratory media has not been studied systematically to date and is unknown. In the environment, concentrations of Sb, as well as those of sulphide, phosphate and/or carbonate are commonly/most often much lower than in laboratory incubations. Thus, the final product of Sb reduction in the environment remains to be elucidated. In industrial wastewater treatment, it is often necessary to reduce high initial concentrations of dissolved Sb to low residual levels before discharge154 Here, it is appealing to use dissimilatory Sb reducers in combination with sulphate reducers to produce sulphide for precipitation in situ. Using bench-scale anaerobic reactors,155 recently confirmed successful Sb(V) removal using sulphate-reducing microorganisms. In remediation, so-called ‘permeable reactive barriers’ are being used to treat contaminated waters cost-effectively, removing metals via metal sulphide precipitation. If dissimilatory Sb reducers can be successfully seeded into and/or enriched onto such permeable reactive barriers, this may represent a potential Sb remediation option for the future. However, one should avoid re-oxidation (chemical or microbially catalysed) by ensuring consistently reducing conditions.
Despite growing information on strains capable of catalysing oxidative and reductive reactions of Sb compounds, the question remains as to whether they are abundant in soils and actively involved in mediating these reactions in the environment. Also, it remains to be elucidated how the presence of Sb shapes the microbial community. It has been shown that Sb affects e.g. microbial biomass formation, soil respiration and rates of nitrification.74 Sb(III) decreased the bacterial diversity and abundance of specific bacterial phyla, whereas little or no effect was observed with Sb(V).74 This suggests that Sb species, particularly Sb(III), can have negative effects on microbial communities and the biogeochemical processes they catalyse. However, purely correlation-based studies are of limited value, as they do not prove a causal relationship. Studies that investigate the change in microbial community structures in soils (or another environmental system) using controlled and replicated experiments with additions of different Sb species and concentrations are clearly needed. Monitoring key biogeochemical processes along with the assessment of microbial community structures, or better, of the environmental transcriptome, will ultimately allow the effect of the degree of microbial control on the fate of Sb to be determined. It will also help to identify key responsive taxa indicating Sb stress or Sb tolerance, and RNAseq data will allow the identification of key responsive genes to Sb transformations or stress. This will allow us to develop genetic markers that may be used to demonstrate, for example, the presence of potentially Sb-metabolising organisms in the environment or even specific Sb-transforming pathways. The first promising steps in this direction have been undertaken (e.g. ref. 156). Genes involved in Sb redox reactions have only recently become known157 and can serve as genetic markers for microbial Sb transformations in the environment.
2.1. Interaction with plants
Bioavailable soil Sb poses a risk for both the animal and human food chains, as well as for crop yield. It has been shown that Sb concentrations in soils and plant-tissue concentrations show a strong positive correlation.158,159 Sb concentrations exceeding 150 mg kg−1 in soils and >5 mg kg−1 in plants can disrupt the normal functioning of plants.27,160 The adverse effects can manifest in different ways, some are growth deficiencies or retardation,161 biomass reduction,162 impaired photosynthesis,163,164 and generation and excessive accumulation of reactive oxygen species harmful to plants.164,165 Although the mechanism of Sb(III) uptake is relatively well known,166,167 the mechanisms responsible for the uptake of Sb(V) into plants remain incompletely understood. It has been suggested that Sb(V) enters plants through anionic transporters. For example, transporters for Cl− and NO3− are not very selective and they may also facilitate the uptake of other anions, such as Sb(V).168 Recent studies have suggested that the uptake of Sb(V) can also be affected by the concentration of phosphorus.167,169 The mechanism of uptake and the possible negative effect of other dissolved Sb species (i.e. sulphur-containing or thio-species) is entirely unknown.
Phytoremediation is frequently proposed as a cost-efficient and socially acceptable remediation technique for metals (see recent review by e.g. ref. 170). Certainly, some promising progress has been made in phytoremediation (e.g. the development of hyperaccumulating plants through genetic engineering and bioaugmentation with specific microbial strains;170). Nonetheless, several limitations remain to date and often render phytoremediation uneconomical and/or impractical. One of the most common limitations of phytoremediation is the insufficient uptake and concentration of metals in plant tissues. For instance,171 investigated the levels of Sb accumulation across >34 plant species in an area heavily polluted with Sb (∼6000 mg kg−1), observing tissue concentrations of max ∼143.7 mg kg−1. So-called ‘enhanced phytoremediation’ tries to overcome this limitation by using complexing agents to increase dissolved Sb concentration in the soil pore water and with this metal uptake in plants.172–174 However, the application of complexation comes at the risk of increased leaching from soils.175–177 Furthermore, for effective phytoremediation, Sb should be transferred to above-ground harvestable parts of the plant, which is quantified by the ‘translocation factor’ (ratio of shoot versus root concentration).172 This renders many plants with a low translocation factor unsuitable for phytoremediation (e.g. Arundo donax in the study of ref. 171). Accumulation of Sb within roots might in fact be a good strategy for plants to prevent Sb accumulation and transport into reproductive tissues in highly Sb-contaminated environments. For instance, some plants in an Sb mining area in Southwest China showed high Sb concentrations in the root tissue (>1000 mg kg−1), but translocation factors were well below 1.178 To extract a high total amount of metals from the soil, it is necessary to build up a large amount of harvestable biomass with increased metal concentrations within the above-ground biomass. Despite being cost-efficient and socially acceptable, it remains to be proven whether phytoremediation of Sb will ever prove to be an ‘effective’ method of remediation, allowing a high mass of Sb to be extracted in a relatively short period of time. A better understanding of the processes governing Sb mobilisation into soil pore water, as well as the molecular processes of uptake and translocation within the plant, will be imperative for effectively identifying appropriate plants for bioremediation.
3. Conclusions and outlook
The interactions between Sb and soil phases have been extensively studied. Iron and manganese (hydr)oxides are recognised as important sorbents for Sb, and SOM is known to complex with Sb. Although interactions with other phases, such as clay minerals and sulphides, are acknowledged, they have been less thoroughly explored despite their prevalence in nature. There is certainly a large body of literature covering the interaction of Sb with pure mineral phases. We believe that such research is crucial for understanding the behaviour of Sb in the frame of a potential treatment for Sb contamination when defined minerals are added, for instance, during in situ remediation or drinking water treatment. Most studies are, however, oversimplified regarding the behaviour of Sb in the natural environment, as they often do not account for its complexity and dynamic redox conditions.
Sb undergoes various redox reactions, and its fate is influenced by its speciation. Redox-active minerals, such as Fe/Mn oxides and phyllosilicates containing Fe, not only sorb and complex Sb but may also cause changes of its redox state. These minerals gain their redox properties and enhanced reactivity through interactions with redox-active constituents in solution and require fluctuating redox conditions to remain highly reactive. In soils subject to waterlogging and/or periodic flooding, such dynamic redox conditions are typical. In laboratory experiments, redox conditions are mostly much less dynamic (and the composition of media less complex). Many redox reactions in nature are catalysed by microbes, so laboratory experiments using pure strains and sterile conditions are considered too simplistic to accurately determine the ultimate environmental fate of Sb. We recommend conducting future studies with greater complexity that more closely mimics field/natural conditions. In this regard, one may, for instance, study the impact of natural microbial consortia in soils contaminated with Sb from the field in lysimeter experiments, capturing the impact of seasonal climatic changes.
While the microbial control of Fe and Mn cycling is well understood, information on how the microbial reduction of Fe and Mn affects Sb fate is still limited. In general, mobilisation in conjunction with the reductive dissolution of the host phases is expected, yet some studies have shown increased Sb sorption following the initial release. When reduced soil solutions become oxidised again, dissolved Fe and Mn form solid particles, often driven by microbial activity. The so-called ‘biogenic’ Mn oxides have a significantly higher sorption potential than chemically produced ones (the same may hold true for Fe phases). Moreover, the sorption capacities of these phases change over time due to ‘ageing’. This highlights the need for studies under biologically active and field-like conditions to realistically assess the true Sb environmental fate.
The interaction between Sb and SOM involves complexation by either DOM or organic matter associated with solid soil phases. These reactions are well studied, but it is still unclear whether SOM complexation generally increases or decreases the mobility and bioavailability of Sb. To resolve this uncertainty, we propose the use of species-specific methods, such as spectroscopic techniques (e.g. XAFS) and mass spectrometry (e.g. LC-ICP-MS), to quantify and identify Sb–SOM complexes and thus to provide more conclusive answers related to the impact of SOM on Sb mobility. Having species-specific methods at hand would also allow a better understanding of the toxicity of Sb, which is species-dependent. However, hyphenated methods such as LC-ICP-MS will face a particular challenge regarding proper sample preparation, preserving original Sb speciation and resolving various species with sufficient sensitivity.
Another important open research question that we identified here is whether the interaction of Sb with sulphide ultimately increases or decreases Sb solubility and mobility. In sulphide-rich environments, such as geothermal systems, dissolved thioantimony species form naturally. Several studies exist on Sb solubility and speciation in sulfidic solutions (e.g., ref. 179 and references therein). However, there is only limited information on the occurrence of thioantimony in natural waters beyond geothermal settings and hot springs. Biogenic sulphide, produced by sulphate-reducing bacteria, can also form these species, as demonstrated in laboratory experiments. Given the widespread presence and activity of these bacteria, thioantimony species are likely to be found in many environments. On the other hand, some studies suggest that under sulphide-rich conditions, stibnite (Sb2S3) may form as a product of dissimilatory Sb reduction, potentially replacing Sb trioxide (Sb2O3) precipitation when sulphide is present. Since Sb2S3 is practically insoluble in water, this reaction would result in significantly lower dissolved Sb concentrations in the environment.
Microbial reactions involving Sb compounds can be broadly classified into oxidative reactions and reductive reactions. The genes and enzymes, particularly those involved in Sb(V) reduction, were identified only recently. Before the identification of specific genes and enzymes conferring Sb oxidation and/or reduction, our understanding of microbial interactions with Sb was limited to high-level taxonomic classifications and correlations with the Sb species quantified. This provided only little insight into the actual metabolic activities of specific strains in the environment. With the identification of relevant genes and enzymes, we can now perform transcriptomic analyses and design specific probes (such as RT-PCR) to investigate which microbial strains are actively driving Sb redox reactions in complex consortia in the environment. We emphasize that laboratory incubations typically use high Sb concentrations to enrich pure microbial strains. Soils and sediments, however, are not necessarily heavily contaminated with Sb (but still pose a risk of Sb migration and uptake into the food chain). Therefore, it remains to be determined whether the pure strains isolated thus far are truly representative of communities in moderately contaminated areas. A deeper understanding of the microbial processes affecting Sb in such environments will hopefully enhance our ability to manage Sb-associated risks and to remediate Sb contamination effectively.
Data availability
No primary research results, software or code have been included and no new data were generated or analysed as part of this review.
Conflicts of interest
There are no conflicts to declare.
Acknowledgements
The financial support by ELECTRA H2020 project contract No. 826244 is gratefully appreciated.
References
- C. G. Anderson, The metallurgy of antimony, Chem. Erde, 2012, 72(SUPPL.4), 3–8, DOI:10.1016/j.chemer.2012.04.001.
- W. Zhuang, X. Lai, Q. Wang, Y. Liu, Q. Chen and C. Liu, Distribution characteristics, sources and ecological risk of antimony in the surface sediments of Changjiang Estuary and the adjacent sea, East China, Mar. Pollut. Bull., 2018, 137, 474–480 CrossRef CAS PubMed.
-
U.S. Geological Survey, Antimony, 2023 Search PubMed.
-
European Commission, Study on the Critical Raw Materials for the EU 2023 Final Report, 2023, http://www.europa.eu Search PubMed.
- D. Dupont, S. Arnout, P. Tom, J. Koen and P. T. Jones, Antimony Recovery from End-of-Life Products and Industrial Process Residues : A Critical Review, J. Sustain. Metall., 2016, 2(1), 79–103 CrossRef.
-
K. J. Schulz, J. H. DeYoung Jr, R. R. Seal II and D. Bradley, Critical mineral resources of the United States—An introduction, 2017, Professional Paper, Available from: http://periodic.lanl.gov/images/periodictable.pdf Search PubMed.
- J. Lewis, J. Sjöström, U. Skyllberg and L. Hägglund, Distribution, Chemical Speciation, and Mobility of Lead and Antimony Originating from Small Arms Ammunition in a Coarse-Grained Unsaturated Surface Sand, J. Environ. Qual., 2010, 39(3), 863–870, DOI:10.2134/jeq2009.0211.
- Z. Jin, Z. Zhang, J. Xiu, H. Song, T. Gatti and Z. He, A critical review on bismuth and antimony halide based perovskites and their derivatives for photovoltaic applications: recent advances and challenges, J. Mater. Chem. A, 2020, 8(32), 16166–16188 RSC . Available from: https://pubs.rsc.org/en/content/articlehtml/2020/ta/d0ta05433j.
- J. Li, B. H. Zheng, Y. He, Y. Zhou, X. Chen and S. Ruan,
et al., Antimony contamination, consequences and removal techniques: A review, Ecotoxicol. Environ. Saf., 2018, 156, 125–134 CrossRef CAS PubMed.
- M. Filella, N. Belzile and Y. W. Chen, Antimony in the environment: A review focused on natural waters II. Relevant solution chemistry, Earth Sci. Rev., 2002, 59(1–4), 265–285 CrossRef CAS.
- L. R. Terry, T. R. Kulp, H. Wiatrowski, L. G. Miller and R. S. Oremland, Microbiological oxidation of antimony(III) with oxygen or nitrate by bacteria isolated from contaminated mine sediments, Appl. Environ. Microbiol., 2015, 81(24), 8478–8488 CrossRef CAS PubMed.
- I. A. Tsaplina, V. V. Sorokin, A. E. Zhuravleva, V. S. Melamud, T. I. Bogdanova and T. F. Kondrat’eva, Oxidation of gold-antimony ores by a thermoacidophilic microbial consortium, Microbiology, 2013, 82(6), 680–689, DOI:10.1134/S0026261713060118.
- J. Li, Q. Wang, M. Li, B. Yang, M. Shi and W. Guo,
et al., Proteomics and genetics for identification of a bacterial antimonite oxidase in agrobacterium tumefaciens, Environ. Sci. Technol., 2015, 49(10), 5980–5989, DOI:10.1021/es506318b.
- J. Li, B. Yang, M. Shi, K. Yuan, W. Guo and Q. Wang,
et al., Abiotic and biotic factors responsible for antimonite oxidation in Agrobacterium tumefaciens GW4, Sci. Rep., 2017, 7, 1–11 CrossRef PubMed.
- V. K. Nguyen, W. Choi, Y. Park, J. Yu and T. Lee, Characterization of diversified Sb(V)-reducing bacterial communities by various organic or inorganic electron donors, Bioresour. Technol., 2018, 250, 239–246 CrossRef CAS PubMed.
- T. R. Kulp, L. G. Miller, F. Braiotta, S. M. Webb, B. D. Kocar and J. S. Blum,
et al., Microbiological reduction of Sb(V) in anoxic freshwater sediments, Environ. Sci. Technol., 2014, 48(1), 218–226 CrossRef CAS PubMed.
- C. Y. Lai, L. L. Wen, Y. Zhang, S. S. Luo, Q. Y. Wang and Y. H. Luo,
et al., Autotrophic antimonate bio-reduction using hydrogen as the electron donor, Water Res., 2016, 88, 467–474 CrossRef CAS PubMed.
- S. Yamamura, C. Iida, Y. Kobayashi, M. Watanabe and S. Amachi, Production of two morphologically different antimony trioxides by a novel antimonate-reducing bacterium, Geobacter sp. SVR, J. Hazard. Mater., 2021, 411, 125100 CrossRef CAS PubMed.
- R. Bentley and T. G. Chasteen, Microbial Methylation of Metalloids: Arsenic, Antimony, and Bismuth, Microbiol. Mol. Biol. Rev., 2002, 66(2), 250–271, DOI:10.1128/mmbr.66.2.250-271.2002.
- J. Meyer, A. Schmidt, K. Michalke and R. Hensel, Volatilisation of metals and metalloids by the microbial population of an alluvial soil, Syst. Appl. Microbiol., 2007, 30(3), 229–238 CrossRef CAS PubMed.
- M. C. Kasemodel, I. K. Sakamoto, M. B. A. Varesche and V. G. S. Rodrigues, Potentially toxic metal contamination and microbial community analysis in an abandoned Pb and Zn mining waste deposit, Sci. Total Environ., 2019, 675, 367–379 CAS.
- X. Pan, S. Zhang, Q. Zhong, G. Gong, G. Wang and X. Guo,
et al., Effects of soil chemical properties and fractions of Pb, Cd, and Zn on bacterial and fungal communities, Sci. Total Environ., 2020, 715, 136904 CrossRef CAS PubMed.
- P. G. S. A. Jeyasundar, A. Ali, M. Azeem, Y. Li, D. Guo and A. Sikdar,
et al., Green remediation of toxic metals contaminated mining soil using bacterial consortium and Brassica juncea, Environ. Pollut., 2021, 277 Search PubMed . Available from: https://pubmed.ncbi.nlm.nih.gov/33640810/.
- I. Sazykin, L. Khmelevtsova, T. Azhogina and M. Sazykina, Heavy Metals Influence on the Bacterial Community of Soils: A Review, Agriculture, 2023, 13(3), 653 CrossRef CAS . Available from: https://www.mdpi.com/2077-0472/13/3/653/htm.
- S. Sundar and J. Chakravarty, Antimony Toxicity, Int. Res. J. Publ. Environ. Health, 2010, 7(12), 4267–4277 CrossRef CAS PubMed . Available from: https://www.mdpi.com/1660-4601/7/12/4267/htm.
- R. Wysocki, J. I. Rodrigues, I. Litwin and M. J. Tamás, Mechanisms of genotoxicity and proteotoxicity induced by the metalloids arsenic and antimony, Cell. Mol. Life Sci., 2023, 80(11), 1–30, DOI:10.1007/s00018-023-04992-5.
- Natasha, M. Shahid, S. Khalid, C. Dumat, A. Pierart and N. K. Niazi, Biogeochemistry of antimony in soil-plant system: Ecotoxicology and human health, Appl. Geochem., 2019, 106, 45–59 CrossRef CAS.
- Z. Lai, M. He, C. Lin, W. Ouyang and X. Liu, Interactions of antimony with biomolecules and its effects on human health, Ecotoxicol. Environ. Saf., 2022, 233, 113317 CrossRef CAS PubMed.
-
B. E. Sample and C. A. Arenal, Wildlife Toxicity Assessment for Antimony, Wildlife Toxicity Assessments for Chemicals of Military Concern, 2015, pp. 325–348 Search PubMed.
-
USEPA. Priority Pollutant List [Internet]. 2014 [cited 2024 Nov 18]. Available from: https://www.epa.gov/sites/default/files/2015-09/documents/priority-pollutant-list-epa.pdf.
-
USEPA. National Primary Drinking Water Regulations [Internet]. 2023 [cited 2023 Jul 30]. Available from: https://www.epa.gov/ground-water-and-drinking-water/national-primary-drinking-water-regulations#Inorganic.
-
Official Journal of the European Union. DIRECTIVE (EU) 2020/2184 OF THE EUROPEAN PARLIAMENT AND OF THE COUNCIL of 16 December 2020 on the quality of water intended for human consumption [Internet]. 2020 [cited 2024 Jun 27]. Available from: https://eur-lex.europa.eu/eli/dir/2020/2184/oj.
-
European Union. European Union (Drinking Water) Regulations. 2023 [cited 2023 Aug 3]; Available from: chrome-extension://efaidnbmnnnibpcajpcglclefindmkaj/https://www.irishstatutebook.ie/eli/2023/si/99/made/en/pdf.
-
World Health Organization, Guidelines for Drinking-Water Quality: Fourth Edition Incorporating the First Addendum, Guidelines for Drinking-Water Quality: Fourth Edition Incorporating the First Addendum, World Health Organization, 2017, p. 541, https://www.ncbi.nlm.nih.gov/books/NBK442376/ Search PubMed.
- M. Filella, N. Belzile and Y. W. Chen, Antimony in the environment: A review focused on natural waters
I. Occurrence, Earth Sci. Rev., 2002, 57(1–2), 125–176 CrossRef CAS.
- M. Krachler, J. Zheng, D. Fisher and W. Shotyk, Atmospheric Sb in the Arctic during the past 16,000 years: Responses to climate change and human impacts, Global Biogeochem. Cycles, 2008, 22(1), 1015, DOI:10.1029/2007GB002998.
- J. Chen, G. Liu, Y. Kang, B. Wu, R. Sun and C. Zhou,
et al., Atmospheric emissions of F, As, Se, Hg, and Sb from coal-fired power and heat generation in China, Chemosphere, 2013, 90(6), 1925–1932 CrossRef CAS PubMed.
- M. Adnan, B. Xiao, M. U. Ali, P. Xiao, P. Zhao and H. Wang,
et al., Heavy metals pollution from smelting activities: A threat to soil and groundwater, Ecotoxicol. Environ. Saf., 2024, 274, 116189 CAS.
- C. A. Johnson, H. Moench, P. Wersin, P. Kugler and C. Wenger, Solubility of Antimony and Other Elements in Samples Taken from Shooting Ranges, J. Environ. Qual., 2005, 34(1), 248–254, DOI:10.2134/jeq2005.0248.
- B. H. Robinson, S. Bischofberger, A. Stoll, D. Schroer, G. Furrer and S. Roulier,
et al., Plant uptake of trace elements on a Swiss military shooting range: Uptake pathways and land management implications, Environ. Pollut., 2008, 153(3), 668–676 CrossRef CAS PubMed.
- V. Antoniadis, E. E. Golia, Y. T. Liu, S. L. Wang, S. M. Shaheen and J. Rinklebe, Soil and maize contamination by trace elements and associated health risk assessment in the industrial area of Volos, Greece, Environ. Int., 2019, 124, 79–88 CrossRef CAS PubMed.
- D. M. Templeton, F. Ariese, R. Cornelis, L. G. Danielsson, H. Muntau and H. P. Van Leeuwen,
et al., Guidelines for terms related to chemical speciation and fractionation of elements. Definitions, structural aspects, and methodological approaches (IUPAC recommendations 2000), Pure Appl. Chem., 2000, 72(8), 1453–1470, DOI:10.1351/pac200072081453/html.
- I. Herath, M. Vithanage and J. Bundschuh, Antimony as a global dilemma: Geochemistry, mobility, fate and transport, Environ. Pollut., 2017, 223, 545–559 Search PubMed . Available from: https://pubmed.ncbi.nlm.nih.gov/28190688/.
- Y. Zhang, E. J. O'Loughlin and M. J. Kwon, Antimony redox processes in the environment: A critical review of associated oxidants and reductants, J. Hazard. Mater., 2022, 431, 128607 Search PubMed.
- M. Filella, P. A. Williams and N. Belzile, Antimony in the environment: Knowns and unknowns, Environ. Chem., 2009, 6(2), 95–105 CAS.
- J. Besold, N. Kumar, A. C. Scheinost, J. Lezama Pacheco, S. Fendorf and B. Planer-Friedrich, Antimonite Complexation with Thiol and Carboxyl/Phenol Groups of Peat Organic Matter, Environ. Sci. Technol., 2019, 53(9), 5005–5015 CrossRef CAS PubMed.
- L. Ye and C. Jing, Environmental geochemistry of thioantimony: formation, structure and transformation as compared with thioarsenic, Environ. Sci.: Processes Impacts, 2021, 23(12), 1863–1872 CAS . Available from: https://pubs.rsc.org/en/content/articlehtml/2021/em/d1em00261a.
- N. Belzile, Y. W. Chen and Z. Wang, Oxidation of antimony (III) by amorphous iron and Manganese oxyhydroxides, Chem. Geol., 2001, 174(4), 379–387 CrossRef CAS.
- A. K. Leuz, H. Mönch and C. A. Johnson, Sorption of Sb(III) and Sb(V) to goethite: Influence on Sb(III) oxidation and mobilization, Environ. Sci. Technol., 2006, 40(23), 7277–7282 CrossRef CAS PubMed.
- S. Mitsunobu, Y. Takahashi, Y. Terada and M. Sakata, Antimony(V) incorporation into synthetic ferrihydrite, goethite, and natural iron oxyhydroxides, Environ. Sci. Technol., 2010, 44(10), 3712–3718, DOI:10.1021/es903901e.
- S. Rakshit, D. Sarkar, P. Punamiya and R. Datta, Antimony sorption at gibbsite–water interface, Chemosphere, 2011, 84(4), 480–483 CrossRef CAS PubMed.
- D. T. Scott, D. M. Mcknight, E. L. Blunt-Harris, S. E. Kolesar and D. R. Lovley, Quinone moieties act as electron acceptors in the reduction of humic substances by humics-reducing microorganisms, Environ. Sci. Technol., 1998, 32(19), 2984–2989, DOI:10.1021/es980272q.
- A. Kappler and P. V. Cappellen, Biogeochemical Redox Processes and their Impact on Contaminant Dynamics, Environ. Sci. Technol., 2010, 44(1), 15–23 CrossRef PubMed.
- J. Xi, M. He and C. Lin, Adsorption of antimony(V) on kaolinite as a function of pH, ionic strength and humic acid, Environ. Earth Sci., 2010, 60(4), 715–722, DOI:10.1007/s12665-009-0209-z.
- B. Planer-Friedrich and A. C. Scheinost, Formation and structural characterization of thioantimony species and their natural occurrence in geothermal waters, Environ. Sci. Technol., 2011, 45(16), 6855–6863, DOI:10.1021/es201003k.
- S. Denys, K. Tack, J. Caboche and P. Delalain, Bioaccessibility, solid phase distribution, and speciation of Sb in soils and in digestive fluids, Chemosphere, 2009, 74(5), 711–716 CrossRef CAS PubMed.
- K. Hockmann, S. Tandy, M. Lenz and R. Schulin, Antimony leaching from contaminated soil under manganese- and iron-reducing conditions: column experiments, Environ. Chem., 2014, 11(6), 624–631 CrossRef CAS . Available from: https://www.publish.csiro.au/en/EN14123.
- S. Tandy, K. Hockmann, M. Keller, B. Studer, A. Papritz and R. Schulin, Antimony mobility during prolonged waterlogging and reoxidation of shooting range soil: A field experiment, Sci. Total Environ., 2018, 624, 838–844 CrossRef CAS PubMed.
- R. Watkins, D. Weiss, W. Dubbin, K. Peel, B. Coles and T. Arnold, Investigations into the kinetics and thermodynamics of Sb(III) adsorption on goethite (α-FeOOH), J. Colloid Interface Sci., 2006, 303(2), 639–646 CrossRef CAS PubMed.
- R. Kirsch, A. C. Scheinost, A. Rossberg, D. Banerjee and L. Charlet, Reduction of antimony by nano-particulate magnetite and mackinawite, Mineral. Mag., 2008, 72(1), 185–189 CrossRef CAS . Available from: https://www.cambridge.org/core/journals/mineralogical-magazine/article/abs/reduction-of-antimony-by-nanoparticulate-magnetite-and-mackinawite/D2194571EF9AC9424DD48262BCE6FEA9.
- P. Qi and T. Pichler, Competitive adsorption of As(III), As(V), Sb(III) and Sb(V) onto ferrihydrite in multi-component systems: Implications for mobility and distribution, J. Hazard. Mater., 2017, 330, 142–148, DOI:10.1016/j.jhazmat.2017.02.016.
- L. Yan, T. Chan and C. Jing, Mechanistic Study for Antimony Adsorption and Precipitation on Hematite Facets, Environ. Sci. Technol., 2022, 56(5), 3138–3146, DOI:10.1021/acs.est.1c07801.
- M. Kosmulski, The pH dependent surface charging and points of zero charge. VIII. Update, Adv. Colloid Interface Sci., 2020, 275, 102064 CrossRef CAS PubMed.
- Z. Wu, Y. Zhao, Y. Zheng, M. Yin, J. Wang and N. Bolan,
et al., The fate of Sb(V) and As(V) during the aging of ferrihydrite, Chem. Eng. J., 2024, 479, 147671 CrossRef CAS.
- P. Qi and T. Pichler, Sequential and simultaneous adsorption of Sb(III) and Sb(V) on ferrihydrite: Implications for oxidation and competition, Chemosphere, 2016, 145, 55–60 CrossRef CAS PubMed.
- X. Guo, Z. Wu, M. He, X. Meng, X. Jin and N. Qiu,
et al., Adsorption of antimony onto iron oxyhydroxides: Adsorption behavior and surface structure, J. Hazard. Mater., 2014, 276, 339–345 CrossRef CAS PubMed.
- S. Mitsunobu, Y. Takahashi and Y. Sakai, Abiotic reduction of antimony(V) by green rust (Fe(4)(II)Fe(2)(III)(OH)(12)SO(4).3H(2)O), Chemosphere, 2008, 70(5), 942–947 CrossRef CAS PubMed . Available from: https://pubmed.ncbi.nlm.nih.gov/17761212/.
- S. Peiffer, A. Kappler, S. B. Haderlein, C. Schmidt, J. M. Byrne and S. Kleindienst,
et al., A biogeochemical–hydrological framework for the role of redox-active compounds in aquatic systems, Nat. Geosci., 2021, 14(5), 264–272 CrossRef CAS . Available from: https://www.nature.com/articles/s41561-021-00742-z.
- M. Komárek, A. Vaněk and V. Ettler, Chemical stabilization of metals and arsenic in contaminated soils using oxides – A review, Environ. Pollut., 2013, 172, 9–22 CrossRef PubMed.
- F. Kolbe, H. Weiss, R. Wennrich, W. G. Lorenz and B. Daus, Remobilization of pentavalent antimony and vanadium from a granular iron hydroxide material – A comparative study of different leaching systems, Talanta, 2011, 85(4), 2089–2093 CrossRef CAS PubMed.
- G. Okkenhaug, K. A. Grasshorn Gebhardt, K. Amstaetter, H. Lassen Bue, H. Herzel and E. Mariussen,
et al., Antimony (Sb) and lead (Pb) in contaminated shooting range soils: Sb and Pb mobility and immobilization by iron based sorbents, a field study, J. Hazard. Mater., 2016, 307, 336–343 CrossRef CAS PubMed . Available from: https://pubmed.ncbi.nlm.nih.gov/26799225/.
- J. E. Post, Manganese oxide minerals: Crystal structures and economic and environmental significance, Proc. Natl. Acad. Sci. U. S. A., 1999, 96(7), 3447 CrossRef CAS PubMed.
- M. E. Essington and K. A. Vergeer, Adsorption of Antimonate, Phosphate, and Sulfate by Manganese Dioxide: Competitive Effects and Surface Complexation Modeling, Soil Sci. Soc. Am. J., 2015, 79(3), 803–814, DOI:10.2136/sssaj2014.12.0482.
- X. Wang, Y. Yang, L. Tao and M. He, Antimonite oxidation and adsorption onto two tunnel-structured manganese oxides: Implications for antimony mobility, Chem. Geol., 2021, 579, 120336 CrossRef CAS.
- X. Zhang, N. Xie, Y. Guo, D. Niu, H. b. Sun and Y. Yang, Insights into adsorptive removal of antimony contaminants: Functional materials, evaluation and prospective, J. Hazard. Mater., 2021, 418, 126345 CrossRef CAS PubMed.
- Q. Sun, P. X. Cui, C. Liu, S. M. Peng, M. E. Alves and D. M. Zhou,
et al., Antimony oxidation and sorption behavior on birnessites with different properties (δ-MnO2 and triclinic birnessite), Environ. Pollut., 2019, 246, 990–998 CrossRef CAS PubMed.
- J. Nie, Z. Yao, P. Shao, Y. Jing, L. Bai and D. Xing,
et al., Revisiting the adsorption of antimony on manganese dioxide: The overlooked dissolution of manganese, Chem. Eng. J., 2022, 429, 132468 CrossRef CAS.
- Z. Shi, S. Peng, P. Wang, Q. Sun, Y. Wang and G. Lu,
et al., Modeling coupled kinetics of antimony adsorption/desorption and oxidation on manganese oxides, Environ. Sci.:Processes Impacts, 2018, 20(12), 1691–1696 RSC . Available from: https://pubs.rsc.org/en/content/articlehtml/2018/em/c8em00323h.
- J. Liu, W. Chen, X. Hu, H. Wang, Y. Zou and Q. He,
et al., Effects of MnO2 crystal structure on the sorption and oxidative reactivity toward thallium(I), Chem. Eng. J., 2021, 416, 127919 CrossRef CAS.
- H. Li, F. Santos, K. Butler and E. Herndon, A Critical Review on the Multiple Roles of Manganese in Stabilizing and Destabilizing Soil Organic Matter, Environ. Sci. Technol., 2021, 55(18), 12136–12152, DOI:10.1021/acs.est.1c00299.
- J. E. Johnson, P. Savalia, R. Davis, B. D. Kocar, S. M. Webb and K. H. Nealson,
et al., Real-Time Manganese Phase Dynamics during Biological and Abiotic Manganese Oxide Reduction, Environ. Sci. Technol., 2016, 50(8), 4248–4258 CrossRef CAS PubMed.
- L. Peng, N. Wang, T. Xiao, J. Wang, H. Quan and C. Fu,
et al., A critical review on adsorptive removal of antimony from waters: Adsorbent species, interface behavior and interaction mechanism, Chemosphere, 2023, 327, 138529 CrossRef CAS PubMed.
- N. Karimian, S. G. Johnston and E. D. Burton, Reductive transformation of birnessite and the mobility of co-associated antimony, J. Hazard. Mater., 2021, 404, 124227 CrossRef CAS PubMed.
- H. Zhou and C. Fu, Manganese-oxidizing microbes and biogenic manganese oxides: characterization, Mn(II) oxidation mechanism and environmental relevance, Rev. Environ. Sci. Biotechnol., 2020, 19(3), 489–507, DOI:10.1007/s11157-020-09541-1.
- Y. Cai, K. Yang, C. Qiu, Y. Bi, B. Tian and X. Bi, A Review of Manganese-Oxidizing Bacteria (MnOB): Applications, Future Concerns, and Challenges, Int. Res. J. Publ. Environ. Health, 2023, 20(2), 1272 CAS.
- Y. M. Nelson, L. W. Lion, W. C. Ghiorse and M. L. Shuler, Production of Biogenic Mn Oxides by Leptothrix discophora SS-1 in a Chemically Defined Growth Medium and Evaluation of Their Pb Adsorption Characteristics, Appl. Environ. Microbiol., 1999, 65(1), 175 CAS.
- Q. Li, Y. He, A. Yang, X. Hu, F. Liu and J. Mu,
et al., Antimony(III) removal by biogenic manganese oxides formed by Pseudomonas aeruginosa PA-1: kinetics and mechanisms, Environ. Sci. Pollut. Res., 2023, 30(43), 97102–97114, DOI:10.1007/s11356-023-29277-z.
- J. B. Dixon, Roles of clays in soils, Appl. Clay Sci., 1991, 5(5–6), 489–503 CAS.
-
I. Bibi, J. Icenhower, N. K. Niazi, T. Naz, M. Shahid and S. Bashir, Clay Minerals: Structure, Chemistry, and Significance in Contaminated Environments and Geological CO2 Sequestration, Environmental Materials and Waste: Resource Recovery and Pollution Prevention, 2016, pp. 543–567 Search PubMed.
- X. Liang, Y. Zang, Y. Xu, X. Tan, W. Hou and L. Wang,
et al., Sorption of metal cations on layered double hydroxides, Colloids Surf. A Physicochem. Eng. Asp., 2013, 433, 122–131 CAS.
- A. M. L. Kraepiel, K. Keller and F. M. M. Morel, A Model for Metal Adsorption on Montmorillonite, J. Colloid Interface Sci., 1999, 210(1), 43–54 CAS.
- C. M. van Genuchten and J. Peña, Antimonate and arsenate speciation on reactive soil minerals studied by differential pair distribution function analysis, Chem. Geol., 2016, 429, 1–9 CAS.
- S. Rakshit, D. Sarkar and R. Datta, Surface complexation of antimony on kaolinite, Chemosphere, 2015, 119, 349–354 CAS.
- P. Thanabalasingam and W. F. Pickering, Specific sorption of antimony (III) by the hydrous oxides of Mn, Fe, and Al, Water Air Soil Pollut., 1990, 49(1–2), 175–185, DOI:10.1007/BF00279519.
- J. Xi, M. He and C. Lin, Adsorption of antimony(III) and antimony(V) on bentonite: Kinetics, thermodynamics and anion competition, Microchem. J., 2011, 97(1), 85–91 CAS.
- A. G. Ilgen and T. P. Trainor, Sb(III) and Sb(V) sorption onto al-rich phases: Hydrous al oxide and the clay minerals kaolinite KGa-1b and oxidized and reduced nontronite NAu-1, Environ. Sci. Technol., 2012, 46(2), 843–851, DOI:10.1021/es203027v.
- Q. Fan, L. Wang, Y. Fu, Q. Li, Y. Liu and Z. Wang,
et al., Iron redox cycling in layered clay minerals and its impact on contaminant dynamics: A review, Sci. Total Environ., 2023, 855, 159003 CAS.
- G. Angst, K. E. Mueller, K. G. J. Nierop and M. J. Simpson, Plant- or microbial-derived? A review on the molecular composition of stabilized soil organic matter, Soil Biol. Biochem., 2021, 156, 108189 CAS.
- M. Verbeeck, Y. Thiry and E. Smolders, Soil organic matter affects arsenic and antimony sorption in anaerobic soils, Environ. Pollut., 2020, 257, 113566 CAS.
- D. R. Lovley and E. J. P. Phillips, Novel Mode of Microbial Energy Metabolism: Organic Carbon Oxidation Coupled to Dissimilatory Reduction of Iron or Manganese, Appl. Environ. Microbiol., 1988, 54(6), 1472 CAS.
- N. Stern, J. Mejia, S. He, Y. Yang, M. Ginder-Vogel and E. E. Roden, Dual Role of Humic Substances As Electron Donor and Shuttle for Dissimilatory Iron Reduction, Environ. Sci. Technol., 2018, 52(10), 5691–5699, DOI:10.1021/acs.est.7b06574.
- M. Xie, X. Zhang, S. Li, N. Maulani, F. Cai and Y. Zheng,
et al., Humic substances as electron acceptor for anaerobic oxidation of methane (AOM) and electron shuttle in Mn (IV)-dependent AOM, Sci. Total Environ., 2024, 912, 169576 CAS.
- S. Diquattro, P. Castaldi, S. Ritch, A. L. Juhasz, G. Brunetti and K. G. Scheckel,
et al., Insights into the fate of antimony (Sb) in contaminated soils: Ageing influence on Sb mobility, bioavailability, bioaccessibility and speciation, Sci. Total Environ., 2021, 770, 145354 CAS.
- J. Buschmann and L. Sigg, Antimony(III) binding to humic substances: Influence of pH and type of humic acid, Environ. Sci. Technol., 2004, 38(17), 4535–4541 CAS.
- M. Tella and G. S. Pokrovski, Stability and structure of pentavalent antimony complexes with aqueous organic ligands, Chem. Geol., 2012, 292–293, 57–68 CAS.
- M. Filella and P. A. Williams, Antimony interactions with heterogeneous complexants in waters, sediments and soils: A review of binding data for homologous compounds, Geochemistry, 2012, 72(SUPPL.4), 49–65 CAS.
- Y. Fan, C. Zheng, A. Huo, Q. Wang, Z. Shen and Z. Xue,
et al., Investigating the binding properties between antimony(V) and dissolved organic matter (DOM) under different pH conditions during the soil sorption process using fluorescence and FTIR spectroscopy, Ecotoxicol. Environ. Saf., 2019, 181, 34–42 CAS . Available from: https://pubmed.ncbi.nlm.nih.gov/31158721/.
- J. Zheng, A. Iijima and N. Furuta, Complexation effect of antimony compounds with citric acid and its application to the speciation of antimony(III) and antimony(V) using HPLC-ICP-MS, J. Anal. At. Spectrom., 2001, 16(8), 812–818 CAS . Available from: https://pubs.rsc.org/en/content/articlehtml/2001/ja/b101943k.
- H. Wu, H. Cui, C. Fu, R. Li, F. Qi and Z. Liu,
et al., Unveiling the crucial role of soil microorganisms in carbon cycling: A review, Sci. Total Environ., 2024, 909, 168627 CAS.
- C. L. Hollabaugh, Chapter 2 Modification of Goldschmidt's geochemical classification of the elements to include arsenic, mercury, and lead as biophile elements, Dev. Toxicol. Environ. Sci., 2007, 5, 9–31 CAS.
- J. F. W. Mosselmans, G. R. Helz, R. A. D. Pattrick, J. M. Charnock and D. J. Vaughan, A study of speciation of Sb in bisulfide solutions by X-ray absorption spectroscopy, Appl. Geochem., 2000, 15(6), 879–889 CrossRef CAS . Available from: https://research.manchester.ac.uk/en/publications/a-study-of-speciation-of-sb-in-bisulfide-solutions-by-x-ray-absor.
- W. W. Bennett, K. Hockmann, S. G. Johnston and E. D. Burton, Synchrotron X-ray absorption spectroscopy reveals antimony sequestration by reduced sulfur in a freshwater wetland sediment, Environ. Chem., 2017, 14(6), 345–349 CrossRef CAS . Available from: https://www.publish.csiro.au/en/EN16198.
- B. Planer-Friedrich, J. London, R. B. Mccleskey, D. K. Nordstrom and D. Wallschläger, Thioarsenates in geothermal waters of yellowstone National Park: Determination, preservation, and geochemical importance, Environ. Sci. Technol., 2007, 41(15), 5245–5251, DOI:10.1021/es070273v.
- B. Planer-Friedrich, J. Forberg, R. Lohmayer, C. F. Kerl, F. Boeing and H. Kaasalainen,
et al., Relative Abundance of Thiolated Species of As, Mo, W, and Sb in Hot Springs of Yellowstone National Park and Iceland, Environ. Sci. Technol., 2020, 54(7), 4295–4304, DOI:10.1021/acs.est.0c00668.
- Q. Guo, B. Planer-Friedrich, L. Luo, M. Liu, G. Wu and Y. Li,
et al., Speciation of antimony in representative sulfidic hot springs in the YST Geothermal Province (China) and its immobilization by spring sediments, Environ. Pollut., 2020, 266(Pt 1), 115221 CAS . Available from: https://pubmed.ncbi.nlm.nih.gov/32698057/.
- N. J. Olsen, B. W. Mountain and T. M. Seward, Antimony(III) Speciation in Hydrosulfide Solutions from 70 to 400 °c and up to 300 bar, ACS Earth Space Chem., 2019, 3(6), 1058–1072 CrossRef CAS . Available from: https://www.researchgate.net/publication/332337000_AntimonyIII_Speciation_in_Hydrosulfide_Solutions_from_70_to_400_C_and_up_to_300_bar.
- L. Yan, T. Chan and C. Jing, Mechanistic study for stibnite oxidative dissolution and sequestration on pyrite, Environ. Pollut., 2020, 262, 114309 CAS.
- L. Ye, W. Zhong, M. Zhang and C. Jing, New Mobilization Pathway of Antimonite: Thiolation and Oxidation by Dissimilatory Metal-Reducing Bacteria via Elemental Sulfur Respiration, Environ. Sci. Technol., 2022, 56(1), 652–659, DOI:10.1021/acs.est.1c05206.
- B. Planer-Friedrich and N. Wilson, The stability of tetrathioantimonate in the presence of oxygen, light, high temperature and arsenic, Chem. Geol., 2012, 322–323, 1–10 CAS.
- L. Ye, X. Meng and C. Jing, Influence of sulfur on the mobility of arsenic and antimony during oxic-anoxic cycles: Differences and competition, Geochim. Cosmochim. Acta, 2020, 288, 51–67 CAS.
- G. Muyzer and A. J. M. Stams, The ecology and biotechnology of sulphate-reducing bacteria, Nat. Rev. Microbiol., 2008, 6(6), 441–454 CAS . Available from: https://www.nature.com/articles/nrmicro1892.
- K. A. Weber, L. A. Achenbach and J. D. Coates, Microorganisms pumping iron: anaerobic microbial iron oxidation and reduction, Nat. Rev. Microbiol., 2006, 4(10), 752–764 CAS . Available from: https://www.nature.com/articles/nrmicro1490.
- E. D. Burton, K. Hockmann, N. Karimian and S. G. Johnston, Antimony mobility in reducing environments: The effect of microbial iron(III)-reduction and associated secondary mineralization, Geochim. Cosmochim. Acta, 2019, 245, 278–289 CAS.
- J. Long, D. Zhou, Y. Huang, Z. Yi, D. Bin and Y. Luo,
et al., Iron reduction process and antimony behavior change in paddy soils under stationary flooding conditions, Appl. Geochem., 2022, 142, 105311 CAS.
- K. Hockmann, M. Lenz, S. Tandy, M. Nachtegaal, M. Janousch and R. Schulin, Release of antimony from contaminated soil induced by redox changes, J. Hazard. Mater., 2014, 275, 215–221 CAS . Available from: https://pubmed.ncbi.nlm.nih.gov/24862348/.
- C. M. Hansel, S. G. Benner, J. Neiss, A. Dohnalkova, R. K. Kukkadapu and S. Fendorf, Secondary mineralization pathways induced by dissimilatory iron reduction of ferrihydrite under advective flow, Geochim. Cosmochim. Acta, 2003, 67(16), 2977–2992 CAS.
- H. Liu, P. Li, M. Zhu, Y. Wei and Y. Sun, Fe(II)-induced transformation from ferrihydrite to lepidocrocite and goethite, J. Solid State Chem., 2007, 180(7), 2121–2128 CAS.
- R. S. Cutting, V. S. Coker, J. W. Fellowes, J. R. Lloyd and D. J. Vaughan, Mineralogical and morphological constraints on the reduction of Fe(III) minerals by Geobacter sulfurreducens, Geochim. Cosmochim. Acta, 2009, 73(14), 4004–4022 CAS.
- J. Xie, V. S. Coker, B. O'Driscoll, R. Cai, S. J. Haigh and J. R. Lloyd, Microbial Reduction of Antimony(V)-Bearing Ferrihydrite by Geobacter sulfurreducens, Appl. Environ. Microbiol., 2023, 89(3), e0217522, DOI:10.1128/aem.02175-22.
- A. Kappler, C. Bryce, M. Mansor, U. Lueder, J. M. Byrne and E. D. Swanner, An evolving view on biogeochemical cycling of iron, Nat. Rev. Microbiol., 2021, 19(6), 360–374 CrossRef CAS PubMed . Available from: https://www.nature.com/articles/s41579-020-00502-7.
- J. F. Su, S. C. Shao, T. L. Huang, F. Ma, S. F. Yang and Z. M. Zhou,
et al., Anaerobic nitrate-dependent iron(II) oxidation by a novel autotrophic bacterium, Pseudomonas sp. SZF15, J. Environ. Chem. Eng., 2015, 3(3), 2187–2193 CrossRef CAS.
- X. Wang, G. J. Xie, N. Tian, C. C. Dang, C. Cai and J. Ding,
et al., Anaerobic microbial manganese oxidation and reduction: A critical review, Sci. Total Environ., 2022, 822, 153513 CrossRef CAS PubMed.
- L. Huang, X. Liu, C. Rensing, Y. Yuan, S. Zhou and K. H. Nealson, Light-independent anaerobic microbial oxidation of manganese driven by an electrosyntrophic coculture, ISME J., 2022, 17(1), 163–171 CrossRef PubMed . Available from: https://www.nature.com/articles/s41396-022-01335-3.
- L. Costa, M. Martinez, M. Suleiman, R. Keiser, M. Lehmann and M. Lenz, Manganese reductive dissolution coupled to Sb mobilization in contaminated shooting range soil, Appl. Microbiol. Biotechnol., 2024, 108(1), 295 CrossRef CAS PubMed . Available from: https://pubmed.ncbi.nlm.nih.gov/38598118/.
- N. N. Lialikova, [Stibiobacter senarmontii-a new microorganism oxidizing antimony], Mikrobiologiia, 1974, 43(6), 941–948 CAS . Available from: https://pubmed.ncbi.nlm.nih.gov/4449497/.
-
R. Biswas and A. Sarkar, Microbes: Key Players of the Arsenic Biogeochemical Cycle, in Microbial Metabolism of Metals and Metalloids, Advances in Environmental Microbiology, ed. C. J. Hurst, Springer Nature Switzerland AG, Cham, 2022, vol. 10, pp. 197–223, https://link.springer.com/10.1007/978-3-030-97185-4 Search PubMed.
- R. Deng, Y. Chen, X. Deng, Z. Huang, S. Zhou and B. Ren,
et al., A Critical Review of Resistance and Oxidation Mechanisms of Sb-Oxidizing Bacteria for the Bioremediation of Sb(III) Pollution, Front. Microbiol., 2021, 12, 738596 Search PubMed.
- M. Zhang, Z. Li, M. M. Häggblom, F. Li, G. Lu and R. Xu, Bacteria Responsible for Nitrate-dependent Antimonite Oxidation in Antimony-contaminated Paddy Soil Revealed by the Combination of DNA-SIP and Metagenomics, Soil Biol. Biochem., 2021, 156, 108194 CAS.
- D. Min and D. F. Liu, It Is Time to Explore Unique Mechanisms for Microbial Antimony Conversion Rather Than Relying on Analogies with Arsenic Bioconversion, Environ. Sci. Technol., 2023, 57(42), 15780–15781, DOI:10.1021/acs.est.3c08029.
- V. K. Nguyen and J. U. Lee, Antimony-Oxidizing Bacteria Isolated from Antimony-Contaminated Sediment – A Phylogenetic Study, Geomicrobiol. J., 2015, 32(1), 50–58 CAS , Available from: https://www.tandfonline.com/doi/abs/10.1080/01490451.2014.925009.
- E. Hiller, B. Lalinská, M. Chovan, Ľ. Jurkovič, T. Klimko and M. Jankulár,
et al., Arsenic and antimony contamination of waters, stream sediments and soils in the vicinity of abandoned antimony mines in the Western Carpathians, Slovakia, Appl. Geochem., 2012, 27(3), 598–614 CAS.
- W. Guo, Z. Zhang, H. Wang, H. Qin and Z. Fu, Exposure characteristics of antimony and coexisting arsenic from multi-path exposure in typical antimony mine area, J. Environ. Manage., 2021, 289, 112493 CAS.
- C. A. Abin and J. T. Hollibaugh, Dissimilatory antimonate reduction and production of antimony trioxide microcrystals by a novel microorganism, Environ. Sci. Technol., 2014, 48(1), 681–688, DOI:10.1021/es404098z.
- D. R. Lovley, Dissimilatory metal reduction, Annu. Rev. Microbiol., 1993, 47, 263–290 CAS . Available from: https://pubmed.ncbi.nlm.nih.gov/8257100/.
-
D. Lovley, Dissimilatory Fe(III)- and Mn(IV)-Reducing prokaryotes, The Prokaryotes: Prokaryotic Physiology and Biochemistry, 2013, pp. 287–308, https://link.springer.com/referenceworkentry/10.1007/978-3-642-30141-4_69 Search PubMed.
- G. M. Gadd, Metals, minerals and microbes: geomicrobiology and bioremediation, Microbiology (Read.), 2010, 156(Pt 3), 609–643 CAS . Available from: https://pubmed.ncbi.nlm.nih.gov/20019082/.
- R. S. Oremland and J. F. Stolz, The Ecology of Arsenic, Science, 1979, 300(5621), 939–944, DOI:10.1126/science.1081903.
- V. K. Nguyen and J. U. Lee, Isolation and Characterization of Antimony-Reducing Bacteria from Sediments Collected in the Vicinity of an Antimony Factory, Geomicrobiol. J., 2014, 31(10), 855–861 CAS , Available from: https://www.tandfonline.com/doi/abs/10.1080/01490451.2014.<?ccdc_no 901440?><?ccdc_no 901440?>901440<?ccdc END?><?ccdc END?>.
- K. Oorts, E. Smolders, F. Degryse, J. Buekers, G. Gascó and G. Cornelis,
et al., Solubility and toxicity of antimony trioxide (Sb2O3) in soil, Environ. Sci. Technol., 2008, 42(12), 4378–4383, DOI:10.1021/es703061t.
- N. Wang, S. Zhang and M. He, Springer #, Berlin V. Bacterial community profile of contaminated soils in a typical antimony mining site, Environ. Sci. Pollut. Res., 2016, 25(1), 141–152, DOI:10.1007/s11356-016-8159-y.
- H. Zhang and X. Hu, Bioadsorption and microbe-mediated reduction of Sb(V) by a marine bacterium in the presence of sulfite/thiosulfate and the mechanism study, Chem. Eng. J., 2019, 359, 755–764 CAS.
- J. Ramírez-Patiño, J. Pérez-Trevilla, F. J. Cervantes and I. Moreno-Andrade, Removal of antimony by dissimilatory and sulfate-reducing pathways in anaerobic packed bed bioreactors, J. Chem. Technol. Biotechnol., 2023, 98(4), 932–939, DOI:10.1002/jctb.7296.
- A. V. Zotov, N. D. Shikina and N. N. Akinfiev, Thermodynamic properties of the Sb(III) hydroxide complex Sb(OH)3(aq) at hydrothermal conditions, Geochim. Cosmochim. Acta, 2003, 67(10), 1821–1836 CrossRef CAS.
- L. Ye, H. Chen and C. Jing, Sulfate-Reducing Bacteria Mobilize Adsorbed Antimonate by Thioantimonate Formation, Environ. Sci. Technol. Lett., 2019, 6(7), 418–422, DOI:10.1021/acs.estlett.9b00353.
- J. Chen, G. Zhang, C. Ma and D. Li, Antimony removal from wastewater by sulfate-reducing bacteria in a bench-scale upflow anaerobic packed-bed reactor, Acta Geochem., 2020, 39(2), 203–215, DOI:10.1007/s11631-019-00382-6.
- W. Sun, X. Sun, M. M. Häggblom, M. Kolton, L. Lan and B. Li,
et al., Identification of Antimonate Reducing Bacteria and Their Potential Metabolic Traits by the Combination of Stable Isotope Probing and Metagenomic-Pangenomic Analysis, Environ. Sci. Technol., 2021, 55(20), 13902–13912, DOI:10.1021/acs.est.1c03967.
-
H. Liu, W. Sun and M. M. Häggblom, Microbial Transformations of Antimony, in Advances in Environmental Microbiology, 2022, vol. 10, pp. 223–54, Available from: https://link.springer.com/chapter/10.1007/978-3-030-97185-4_9 Search PubMed.
- J. Pratas, M. N. V. Prasad, H. Freitas and L. Conde, Plants growing in abandoned mines of Portugal are useful for biogeochemical exploration of arsenic, antimony, tungsten and mine reclamation, J. Geochem. Explor., 2005, 85(3), 99–107 CAS.
- M. Tschan, B. H. Robinson and R. Schulin, Antimony in the soil - Plant system - A review, Environ. Chem., 2009, 6(2), 106–115 CAS.
- R. W. Feng, L. Lei, J. M. Su, R. R. Zhang, Y. M. Zhu and W. X. Chen,
et al., Toxicity of different forms of antimony to rice plant: Effects on root exudates, cell wall components, endogenous hormones and antioxidant system, Sci. Total Environ., 2020, 711, 134589 CAS.
- F. Cai, J. Ren, S. Tao and X. Wang, Uptake, translocation and transformation of antimony in rice (Oryza sativa L.) seedlings, Environ. Pollut., 2016, 209, 169–176 CAS.
- J. Bech, I. Corrales, P. Tume, J. Barceló, P. Duran and N. Roca,
et al., Accumulation of antimony and other potentially toxic elements in plants around a former antimony mine located in the Ribes Valley (Eastern Pyrenees), J. Geochem. Explor., 2012, 113, 100–105 CAS.
- X. Pan, D. Zhang, X. Chen, A. Bao and L. Li, Antimony accumulation, growth performance, antioxidant defense system and photosynthesis of zea mays in response to antimony pollution in soil, Water Air Soil Pollut., 2011, 215(1–4), 517–523, DOI:10.1007/s11270-010-0496-8.
- C. S. N. Vidya, R. Shetty, M. Vaculíková and M. Vaculík, Antimony toxicity in soils and plants, and mechanisms of its alleviation, Environ. Exp. Bot., 2022, 202, 104996 CAS.
- A. Ortega, I. Garrido, I. Casimiro and F. Espinosa, Effects of antimony on redox activities and antioxidant defence systems in sunflower (Helianthus annuus L.) plants, PLoS One, 2017, 12(9), e0183991, DOI:10.1371/journal.pone.0183991.
- T. Kamiya and T. Fujiwara, Arabidopsis NIP1;1 Transports Antimonite and Determines Antimonite Sensitivity, Plant Cell Physiol., 2009, 50(11), 1977–1981 CrossRef CAS PubMed . Available from: https://academic.oup.com/pcp/article/50/11/1977/1846008.
- R. W. Feng, L. Lei, B. X. Liu, W. X. Chen, R. R. Zhang and L. Z. Wang,
et al., Effects of different inhibitors such as malonic acid, Na3PO4 and HgCl2 on uptake of different forms of antimony in rice plant, Plant Soil, 2019, 445(1–2), 259–271, DOI:10.1007/s11104-019-04296-3.
- R. Feng, C. Wei, S. Tu, Y. Ding, R. Wang and J. Guo, The uptake and detoxification of antimony by plants: A review, Environ. Exp. Bot., 2013, 96, 28–34 CrossRef CAS.
- R. Tisarum, Y. Chen, X. Dong, J. T. Lessl and L. Q. Ma, Uptake of antimonite and antimonate by arsenic hyperaccumulator Pteris vittata: Effects of chemical analogs and transporter inhibitor, Environ. Pollut., 2015, 206, 49–55 CrossRef CAS PubMed.
- H. Tang, M. U. Hassan, M. Nawaz, W. Yang, Y. Liu and B. Yang, A review on sources of soil antimony pollution and recent progress on remediation of antimony polluted soils, Ecotoxicol. Environ. Saf., 2023, 266, 115583 CrossRef CAS PubMed.
- C. Qi, F. Wu, Q. Deng, G. Liu, C. Mo and B. Liu,
et al., Distribution and accumulation of antimony in plants in the super-large Sb deposit areas, China, Microchem. J., 2011, 97(1), 44–51 CrossRef CAS.
- V. Antoniadis, S. M. Shaheen, H. J. Stärk, R. Wennrich, E. Levizou and I. Merbach,
et al., Phytoremediation potential of twelve wild plant species for toxic elements in a contaminated soil, Environ. Int., 2021, 146, 106233 CAS.
- M. Gavrilescu, Enhancing phytoremediation of soils polluted with heavy metals, Curr. Opin. Biotechnol., 2022, 74, 21–31 CAS.
- P. Seridou, K. Fyntrilakis, E. Syranidou and N. Kalogerakis, Hydroponic phytoremediation of antimony by Tamarix smyrnensis and Nerium oleander, J. Chem. Technol. Biotechnol., 2023, 98(9), 2214–2223, DOI:10.1002/jctb.7434.
- L. H. Wu, Y. M. Luo, X. R. Xing and P. Christie, EDTA-enhanced phytoremediation of heavy metal contaminated soil with Indian mustard and associated potential leaching risk, Agric. Ecosyst. Environ., 2004, 102(3), 307–318 CrossRef CAS.
- A. Yano, A. Yano, K. Iwasaki, Y. Kang, K. Sakurai and S. Tanaka, Influence of Chelating Agent Addition on Copper Distribution and Microbial Activity in Soil and Copper Uptake by Brown Mustard (Brassica juncea), Soil Sci. Plant Nutr., 2005, 51(2), 193–202, DOI:10.1111/j.1747-0765.2005.tb00023.x.
- Z. Wu, Q. Jiang, T. Yan, S. Xu, H. Shi and L. Peng,
et al., Antimony symplastic and apoplastic absorption, compartmentation, and xylem translocation in Brassica parachinensis L. under antimonate and antimonite, Ecotoxicol. Environ. Saf., 2020, 197, 110621 CAS.
- Z. Ning, T. Xiao and E. Xiao, Antimony in the Soil-Plant System in an Sb Mining/Smelting Area of Southwest China, Int. J. Phytorem., 2015, 17(11), 1081–1089 CAS , Available from: https://www.tandfonline.com/doi/abs/10.1080/15226514.2015.1021955.
- N. J. Olsen, B. W. Mountain and T. M. Seward, Antimony(III) sulfide complexes in aqueous solutions at 30 °C: A solubility and XAS study, Chem. Geol., 2018, 476, 233–247 CAS.
|
This journal is © The Royal Society of Chemistry 2025 |
Click here to see how this site uses Cookies. View our privacy policy here.