DOI:
10.1039/D0RA09072G
(Paper)
RSC Adv., 2020,
10, 42221-42234
Dihydropyrimidinones: efficient one-pot green synthesis using Montmorillonite-KSF and evaluation of their cytotoxic activity†
Received
24th October 2020
, Accepted 13th November 2020
First published on 23rd November 2020
Abstract
A simple, efficient, cost-effective, recyclable and green approach has been developed for the synthesis of new dihydropyrimidinone analogs via the Biginelli reaction. The methodology involves a multicomponent reaction catalyzed by “HPA-Montmorillonite-KSF” as a reusable and heterogeneous catalyst. This method gives an efficient and much improved modification of the original Biginelli reaction, in terms of yield and short reaction times under solvent free conditions. All the derivatives were subjected to cytotoxicity screening against a panel of four different human cancer cell lines viz. colon (Colo-205), prostate (PC-3), leukemia (THP-1) and lung (A549) to check their effect on percentage growth. MTT [3-(4,5-dimethylthiazol-yl)-diphenyl tetrazoliumbromide] cytotoxicity assay was employed to check IC50 values. Of the synthesized analogs, 16a showed the best activity with IC50 of 7.1 ± 0.8, 13.1 ± 1.4, 13.8 ± 0.9 and 14.7 ± 1.1 μM against lung (A549), leukemia (THP-1), prostate (PC-3) and colon (Colo-205) cancer lines, respectively. The 16a analog was further checked for its effect on cancer cell properties through clonogenic (colony formation) and scratch motility (wound healing) assays and thereby was found that it reduced both the colony formation and migratory properties of the lung cancer cell line (A549). Further, molecular docking studies were performed with 16a to show its binding mode.
1. Introduction
In modern methods of drug discovery processes, design and synthesis of drugs based on biological targets is of the great interest to medicinal chemists. Additionally, semi-synthetic processes for new compounds, obtained by molecular modification of the functional groups of lead compounds, are able to generate structural analogs with greater pharmacological activity and with fewer side effects.1 A constant enrichment in the science of organic synthesis through improvement of the synthetic methodologies is observed, driven by the needs to improve the capability to synthesize molecules in more facile, efficient and in economical ways.2 The paradigms of organic synthesis have shifted from the traditional concept of efficiency in terms of chemical yield to one that also considers economic and ecological values. The significance of a particular reaction can be judged on its capability to form products with dimensions of high yield, chemo-, regio-, stereo- or enantio-selectivity.3 In last one decade, there has been an incredible increase on the accessibility of Multi-Component Reactions (MCRs), and much still remains to be accomplished.4–9 MCRs are important cornerstones in the diversity-oriented construction of molecular complexity due to their ability to incorporate, in a fast and efficient manner, three or more components into a single product.10–14 Reactions that build up carbon–carbon bonds and at the same time introduce nitrogen-containing functionalities into the structural framework are especially attractive for the rapid construction of organic molecules. As MCRs are one-pot processes with simpler experimental conditions that do not require the isolation of intermediates, they are perfect candidates for combinatorial, and generation of products in a single synthetic operation15,16 and are of increasing importance in organic and medicinal chemistry.17–19 Since rapidity and diversity are considered as key factors in modern drug discovery, MCR strategies offer significant advantages over conventional linear-type syntheses, owing to their exceptional synthetic efficiency.20 MCRs contribute to the requirements of an environmentally benign process by reducing the number of synthetic steps, energy consumption and waste production.21 Functionalized nitrogen-heterocycles play a prominent role in medicinal chemistry and therefore they have been intensively used as scaffolds in the field of drug development.22–26 Despite several reports on fused heterocycles, there is a continuing demand for development of new methods for synthesis of novel fused heterocycles due to their plethora of medicinal applications.27
In this context pyrimidine derivatives are of particular interest because of their interesting pharmacological profile.28–30 Dihydropyrimidin-2(1H)-ones (DHPMs), also known as Biginelli compounds are easily accessible via a multicomponent condensation process, first reported more than a century ago.31 The synthesis of 3,4-dihydropyrimidin-2(1H)-ones (Biginelli compounds) and their derivatives is of great importance due to the biological investigation of these molecules via molecular manipulation which have shown several activities such as calcium channel blockers,32–35 α1a-adrenergic receptor antagonists,35 mitotic kinesin inhibitors35 along with anti-bacterial and anti-tubercular activities.36,37 Monastrol, a structurally simple DHPM has been found as a novel cell-permeable molecule that blocks normal bipolar mitotic spindle assembly in mammalian cells by specifically inhibiting the motor activity of the mitotic kinesin Eg5, a motor protein required for spindle bipolarity, and therefore, causes cell cycle arrest.38 There are only a limited number of cell-permeable molecules currently known to specifically inhibit mitotic kinesin Eg5 and such molecules having DHPM scaffolds can therefore be considered as leads for the development of new anticancer drugs.
Based on the above cited findings and inspiration from the potential anticancer activity of DHPMs,39–44 we directed this work towards the synthesis of a diverse series of novel DHPM derivatives of biological interest using a simple, efficient, cost-effective, recyclable and green approach via Biginelli reaction involving the one-pot multicomponent reaction catalyzed by novel “HPA-Montmorillonite-KSF” as a reusable and heterogeneous catalyst. All the newly synthesized compounds were subjected to MTT cytotoxicity screening against a panel of four different human cancer cell lines viz. colon (Colo-205), prostate (PC-3), leukemia (THP-1) and lung (A549) along with normal epithelial cell line (fR-2) followed by clonogenic (colony formation) and scratch motility (wound healing) assays of most potent molecule to analyze any effect on certain cancer properties. Molecular docking studies were carried out to establish the binding capabilities of the same derivative.
2. Results and discussion
2.1. Chemistry
From the literature scan it is evident that DHPM moiety is pharmacologically important and many researchers are engaged to prepare their libraries by applying various modifications using different synthetic strategies to shorten the reaction time and increase the yield using a variation of different catalysts. Some of the reported catalysts used include Cu(OTf)2/MWI,45 Yb(PFO)3,46 HCl/EtOH,47 H3PO3/Pd-Cat, TMSCl/CAN, silica immobilized Ni(II), Ca(OCl)2, Mg(NO3)2, PPA-SiO2, sulfated tungstate, melamine trisulfonic acid, Cu(NO3)2·3H2O, acidic ionic liquids, organocatalysts, H3PMo12O40, H3PW12O40, H6P2W18O62·24H2O, H4PMo11VO40 (ref. 48) and other catalysts. These chemical methods in spite of their potential utility, involve expensive/toxic reagents and adverse reaction conditions like, strong acids, long reaction times, high temperature, stoichiometric amount of catalysts, environmental concerns and poor yields. Therefore, in order to overcome these limitations, the discovery of a new and efficient catalyst with high catalytic activity, short reaction time, recyclability and simple work-up procedures for the preparation of 3,4-dihydropyrimidin-2(1H)-ones under neutral, mild and practical conditions is of prime interest. Biginelli reaction catalysed by heteropolyacids (HPAs) can be one of the sources for the synthesis of large number of new molecules. HPA catalysts are widely exploited for the production of fine organic chemicals, health care products, pharmaceuticals and agrochemical products.49 HPAs are more reactive catalysts than conventional inorganic and organic acids for reactions in solution50 and have been used in organic transformations, such as synthesis of acylals, tetrahydropyranilation of phenols, thioacetalization and transacetalization reactions. They are also used as industrial catalysts for several liquid-phase reactions, including alcohol dehydration, alkylation and esterification.51 HPAs that contain super acidic properties have found numerous applications during last three decades, as useful and versatile acid catalysts for some acid-catalyzed reactions. They are usually solids that are insoluble in non-polar solvents while as highly soluble in polar ones. They can be used in bulk or in supported forms, in both homogeneous and heterogeneous system. Furthermore, HPAs have several advantages, including high flexibility in modification of the acid strength, ease of handling, environmental compatibility, non-toxicity, and experimental simplicity.52
Inspired by above mentioned facts, a novel green methodology has been developed which involves the synthesis of DHPM analogs by Biginelli reaction and their in vitro screening against different human cancer cell lines. The methodology involves the multicomponent reaction catalyzed by HPA-Montmorillonite-KSF (H5PV2W10O40) as a reusable and heterogeneous catalyst. The strategy involves a three-component one-pot Biginelli-type reaction for the condensation of urea, ethylacetoacetate and different aldehydes to corresponding pyrimidinones in presence of a catalytic amount of HPA-Montmorillonite-KSF. The best conditions were achieved under solvent free conditions (neat) using 2 mol% of HPA, 1.5 equivalents of urea/thiourea and ethyl acetoacetate and 1 equivalent of aldehyde under reflux conditions for 1 h, affording the desired product in good yields (Table 1).
Table 1 H5PV2W10O40 catalysed synthesis of DHPM in different solvents and under solvent free conditionsa
S. no. |
Solvent |
Time (h) |
Amount of catalyst (mol%) |
Yield (%) |
Temperature (°C) |
Para-Methoxy benzaldehyde : urea : ethylacetoacetate in the ratio of 1 : 1.5 : 1.5. |
1 |
1,4-Dioxane |
6 h |
10 |
65 |
Reflux |
2 |
Acetonitrile |
5 h |
10 |
77 |
Reflux |
3 |
Toluene |
7 h |
10 |
71 |
Reflux |
4 |
Ethanol |
2 h |
10 |
87 |
Reflux |
5 |
Solvent free |
1 h |
10 |
92 |
Reflux |
6 |
Solvent free |
1 h |
2 |
96 |
Reflux |
7 |
Solvent free |
5 minutes |
2 |
70 |
Microwave at 50 °C |
8 |
Solvent free |
30 minutes |
2 |
74 |
Sonication at 35 °C |
9 |
Ethanol |
18 h |
2 |
40 |
rt (25) |
10 |
Solvent free |
14 h |
2 |
52 |
rt |
2.1.1. Optimization of reaction. The model reaction was carried out by using para-methoxy benzaldehyde (1 eq.), urea (1.5 eq.) and ethylacetoacetate (1.5) in solvent ethanol and catalyst (H5PV2W10O40) under reflux conditions for the generation of corresponding DHPM in good yield (Scheme 1), which was identified by spectral analysis in light of literature.53 Encouraged by the initial success with regard to the formation of 4f, a comprehensive optimization study was performed with the objective of reducing the reaction time and space, enhancing the yields, minimizing the temperature range and exploration study towards the use of other solvents and catalysts for better yields due to the biological and synthetic importance of the DHPMs as described earlier. In the first set of optimization experiments, the reaction was studied in different solvents that included acetonitrile, 1,4-dioxane, toluene, ethanol at reflux conditions. The investigation was also extended for solvent free reaction. The use of solvent free conditions had profound effect in terms of time saving as the reaction was complete in 1 h and the yields were excellent (Table 1). The reaction was also optimized with respect to the use of appropriate/optimum amount of catalyst (H5PV2W10O40) and the best yields at minimum time were obtained by using 2 mol% of HPA. In addition to this, the reaction was monitored at different temperature conditions in microwave; moreover sonication methods were also tried. Microwave assisted reaction adsorbed on silica gel (mesh 60–120) for 5–6 minutes with H5PV2W10O40 as catalyst furnished product 4 in low yield. However, under ultrasonication at 35 °C with reaction time of 30 minutes yields were low as compared to that at reflux. Reaction was also performed at room temperature for stirring, it takes long time for the completion of the reaction and yields were comparatively poor. From the above results, it may be concluded that the temperature affects not only yield of the products, but also the reaction time. The results of the optimization of the reaction conditions are summarized in (Table 1). This methodology is effective with a variety of substituted aliphatic and aromatic aldehydes independent of the nature of substituents (electron donating or electron withdrawing) in the aromatic ring, representing an improvement to the classical Biginelli's methodology as shown in Table 2.
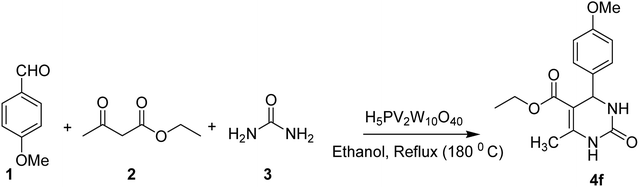 |
| Scheme 1 The model for preparation of DHPMs. | |
Table 2 Synthesis of 3,4-dihydropyrimidin-2(1H)-ones
Entry |
Product |
R |
X |
Yield (%) |
Mp (°C) |
Found |
Reported |
1 |
4a |
C6H5 |
O |
97 |
201–203 |
202–204 |
2 |
4b |
C6H5 |
S |
95 |
206–208 |
205–207 |
3 |
4c |
4-(Cl)–C6H4 |
O |
93 |
209–211 |
210–212 |
4 |
4d |
2-(Cl)–C6H4 |
O |
89 |
213–215 |
214 |
5 |
4e |
4-(Br)–C6H4 |
O |
93 |
211–214 |
213–215 |
6 |
4f |
4-(MeO)–C6H4 |
O |
96 |
201–204 |
201–203 |
7 |
4g |
2,3-(OMe)2–C6H3 |
O |
94 |
176–177 |
178 |
8 |
4h |
2,4-(OMe)2–C6H3 |
O |
95 |
157–160 |
158–160 |
9 |
4i |
3,4,5-(OMe)3–C6H2 |
O |
97 |
|
— |
10 |
4j |
4-(NO2)–C6H4 |
O |
79 |
207–208 |
209–211 |
11 |
4k |
4-(F)–C6H4 |
O |
83 |
|
— |
12 |
4l |
2-(Br)-5-(OMe)–C6H3 |
O |
87 |
|
— |
13 |
4m |
Piperanal |
O |
94 |
|
— |
14 |
4n |
2-(NO2)–C6H4 |
O |
81 |
217–219 |
218–220 |
15 |
4o |
4-(OH)–C6H4 |
O |
78 |
226–228 |
227–228 |
16 |
4p |
3-(OH)-4-(OMe)–C6H3 |
O |
93 |
98–99 |
98–100 |
17 |
4q |
5-(Br)-2-(OMe)–C6H3 |
O |
94 |
|
— |
The next part of the optimization study was to assess the recycled use of catalyst. It may be stated that due to the constantly rising environmental concerns in the field of chemistry, it is sensible to use easily recovered and recycled catalysts, especially expensive or toxic metallic ones for the next use,54 as only few of them meet the criterion of green chemistry. For example, the recovery of ytterbium triflate from water seems cumbersome since water must be removed through heating and then drying under vacuum at 100 °C for 2 hours,55 and in the case of polymer-supported Yb(III) resin, the activity of recycled resin is much lower than that of the original one thus limiting the recyclability.56 Therefore, there is still room for further search for recyclable catalysts to be used in the Biginelli reaction that can convert a variety of aldehydes to pyrimidinones in high yields under mild reaction conditions. In order to show the merit of the present work in comparison with some reported protocols, we compared the results of the synthesis of 5-ethoxycarbonyl-4-phenyl-6-methyl-3,4-dihydropyrimidin-2(1H)-one in the presence of montmorillonite KSF, sulfuric acid, zeolite, silica-sulfuric acid, BF3–OEt2/CuCl, H3PMo12O40 with HPA-Montmorillonite-KSF with respect to the reaction times (Table 3). The yield of product in presence HPA-Montmorillonite-KSF is comparable with these catalysts. However, the reaction in presence of these catalysts required longer reaction times than HPA-Montmorillonite-KSF. The catalyst could be reused several times (six times) and did not show any significant negative influence on the overall yields of the reaction (Fig. 1).
Table 3 Comparison the results of the synthesis of 4-aryl-3,4-dihydropyrimidin-2(1H)-one using different catalysts
Entry |
Catalyst |
Time (h) |
Yield (%) |
1 |
Montmorillonite KSF |
48 |
82 |
2 |
Sulphuric acid |
18 |
71 |
3 |
Zeolite |
12 |
80 |
4 |
Silica sulphur acid |
16 |
91 |
5 |
BF3·OEt2/CuCl |
18 |
71 |
6 |
H3PMo12O40 |
5 |
80 |
7 |
HPA-Montmorilonite-KSF (present work) |
1 |
96 |
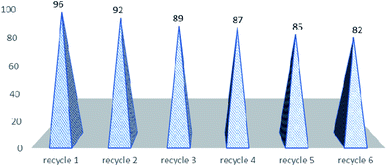 |
| Fig. 1 Recyclability of the catalyst. | |
2.2. Biology
2.2.1. In vitro screening of the novel analogs against human cancer cell lines. 3-(4,5-Dimethylthiazol-2-yl)-2,5-diphenyltetrazolium bromide (MTT) assay was used to screen the newly synthesized analogs against a panel of four different human cancer cell lines viz. colon (Colo-205), prostate (PC-3), leukemia (THP-1) and lung (A549) along with normal epithelial cell line (fR-2). The newly synthesized analogs were screened for their effect on cell growth/viability at the concentrations of 0.07, 0.7, 7.0 and 70.0 μM and concentration dependent growth curves were drawn to determine the IC50 values (ESI Fig. S6† and Table 5). The values are the average of triplicate analysis. The range of 0.07–70 μM concentration of analogs was chosen based on our initial IC50 values determination experiment wherein we found most potent analog has IC50 around 7 μM; so maximum IC50 we seeked to determine in repeat experiment discussed below was in 10-fold range viz 70 μM. Furthermore, 5-fluorouracil, paclitaxel and doxorubicin are known chemotherapy agents used against several cancer types particularly colon and breast; pancreatic and lung; and leukemia, respectively. Also, several studies have been already done by other groups where these anti-cancer molecules have been used in in vitro studies in different cancer cells.58–61 We have in our lab used 5-fluorouracil, paclitaxel and doxorubicin as experimental positive controls for Colo-205 and fR-2; PC-3 and A549; and THP-1, respectively in MTT assay. Most of the synthesized analogs displayed broad spectrum cytotoxic effect in a dose dependent manner. The cytotoxicity profile indicates that the osthol derived DHPM derivative 5a demonstrated cytotoxic effect against colon (Colo-205), prostate (PC-3), leukemia (THP-1) and lung (A549) cancer cell lines with IC50 of 37.6 ± 1.1, 40.8 ± 1.7, 65.8 ± 1.4 and 67.8 ± 1.7 μM, respectively; however, it was also toxic against normal cell line fR-2 with IC50 of 68.8 ± 1.1 μM.
Table 5 IC50 values of analogs for 24 h treatment against colon (Colo-205), prostate (PC-3), leukemia (THP-1) and lung (A549) cancer cell lines using MTT assay. Normal epithelial cell line (fR-2) was used as control. n = 3. mean ± SDa
Compound |
Colon (Colo-205) IC50 |
Prostate (PC-3) IC50 |
Leukemia (THP-1) IC50 |
Lung (A549) IC50 |
Normal (fR-2) IC50 |
IC50 values are expressed in μM concentration. 5-Fluorouracil (Colo-205, fR-2), paclitaxel (PC-3, A549) and doxorubicin (THP-1) were used as positive controls. |
5a |
37.6 ± 1.1 |
40.8 ± 1.7 |
65.8 ± 1.4 |
67.8 ± 1.7 |
68.8 ± 1.1 |
6a |
>70.0 |
>70.0 |
>70.0 |
>70.0 |
>70.0 |
7a |
>70.0 |
>70.0 |
>70.0 |
>70.0 |
>70.0 |
8a |
18.6 ± 1.0 |
22.2 ± 1.6 |
43.8 ± 1.4 |
63.4 ± 1.1 |
67.2 ± 1.5 |
9a |
>70.0 |
>70.0 |
>70.0 |
>70.0 |
>70.0 |
10a |
>70.0 |
29.4 ± 1.2 |
42.1 ± 1.7 |
>70.0 |
>70.0 |
11a |
>70.0 |
29.3 ± 1.3 |
>70.0 |
>70.0 |
>70.0 |
12a |
>70.0 |
>70.0 |
>70.0 |
>70.0 |
>70.0 |
13a |
59.4 ± 1.6 |
35.4 ± 2.2 |
23.2 ± 1.4 |
30.3 ± 2.9 |
67.6 ± 2.5 |
14a |
>70.0 |
>70.0 |
>70.0 |
>70.0 |
>70.0 |
15a |
27.3 ± 1.8 |
>70.0 |
>70.0 |
>70.0 |
>70.0 |
16a |
14.7 ± 1.1 |
13.8 ± 0.9 |
13.1 ± 1.4 |
7.1 ± 0.8 |
>70.0 |
17a |
38.2 ± 1.4 |
47.9 ± 2.1 |
>70.0 |
26.4 ± 1.1 |
>70.0 |
18a |
>70.0 |
>70.0 |
>70.0 |
>70.0 |
>70.0 |
19a |
33.7 ± 1.8 |
>70.0 |
>70.0 |
47.8 ± 2.4 |
>70.0 |
20a |
23.4 ± 1.4 |
>70.0 |
>70.0 |
>70.0 |
68.7 ± 1.1 |
21a |
48.1 ± 2.1 |
>70.0 |
>70.0 |
>70.0 |
>70.0 |
5-Fluorouracil |
33.9 ± 1.9 |
— |
— |
— |
334.7 ± 3.8 |
Paclitaxel |
— |
0.048 ± 0.008 |
— |
5.1 ± 0.4 |
— |
Doxorubicin |
— |
— |
0.018 ± 0.004 |
— |
— |
DHPM derivatives with varying carbon chain length of R group showed interesting results wherein compound 8a bearing n-pentyl chain showed improved cytotoxic effect against colon (Colo-205), prostate (PC-3) leukemia (THP-1) and lung (A549) cancer cell lines with IC50 of 18.6 ± 1.0, 22.2 ± 1.6, 43.8 ± 1.4 and 63.4 ± 1.1 μM, respectively; however, still toxic against normal cell line (fR-2) being with IC50 of 67.2 ± 1.5 μM. On the other hand compounds 6a, 7a and 9a bearing n-propyl, n-butyl and n-hexyl R groups lost their activity with IC50 values not in the range of ≤70 μM, thereby demonstrating the optimum chain length responsible for cytotoxicity. Compound 10a having hydroxyl-styryl R group showed activity against PC-3 and THP-1 with IC50 of 29.4 ± 1.2, and 42.1 ± 1.7 μM, respectively. Compound 11a demonstrated selective cytotoxicity against prostate (PC-3) cancer cell line with IC50 of 29.3 ± 1.3 μM while as its corresponding chloro-derivative (12a) totally lost its activity with IC50 values not in the range of ≤70 μM. Compound 13a showed broad spectrum cytotoxic effect against colon (Colo-205), prostate (PC-3), leukemia (THP-1) and lung (A549) cancer cell line with IC50 of 59.4 ± 1.6, 35.4 ± 2.2, 23.2 ± 1.4 and 30.3 ± 2.9 μM, respectively while toxicity against normal cell line (fR-2) determined with IC50 of 67.6 ± 2.5 μM. The role of an extra ethylene moiety in 15a as compared 14a is highlighted in terms of its selective cytotoxic activity against colon (Colo-205) cancer cell line with IC50 of 27.3 ± 1.8 μM, thereby making provision for synthesis of similar DHPM analogs having more activity and less toxicity. This observation is further supported in 20a and 21a wherein an extra ethylene group in 20a enhances the activity (23.4 ± 1.4 μM) by two fold as compared to 21a (48.1 ± 2.1 μM).
Among all the tested DHPM analogs, 16a bearing a p-methoxy phenyl moiety displayed the most potent cytotoxic effect with IC50 of 14.7 ± 1.1, 13.8 ± 0.9, 13.1 ± 1.4 and 7.1 ± 0.8 μM against colon (Colo-205), prostate (PC-3), leukemia (THP-1) and lung (A549) cancer lines respectively. 17a and 19a with dimethoxy phenyl moieties showed moderate cytotoxicity against lung (A549), prostate (PC-3) and colon (Colo-205) cancer lines, while as, 18a with trimethoxy phenyl moiety lost its activity with IC50 values not in the range of ≤70 μM. These observations demonstrate that the activity of such DHPM analogs increases as the number of methoxy groups decreases in the phenyl moiety of R group. All these results suggest that the structural features have profound influence on the biological profile of a compound. It was concluded that the compound 16a is the most potent derivative with minimum IC50 of 7.1 ± 0.8 μM against lung cancer line (A549). Additionally the derivative had least toxicity against normal epithelial cell line (fR-2) with IC50 value in the range of >70 μM.
One of the hallmark traits of cancer cells is their ability to undergo isolated clonal growth. Clonogenic assay was performed to test the colony formation property of single cell to grow into a colony. This assay confirmed that most potent compound 16a inhibits clonogenic property of A549 cells significantly at 7 and 15 μM when compared with vehicle DMSO; staurosporine is already known to have effect on mobility and invasiveness of A549 cells,58 hence was used as positive control (Fig. 2). To check the anti-metastatic activity, 16a was further evaluated by scratch motility (wound healing) assay against A549 cell line. After A549 cells got attached overnight incubation, the cell monolayers were wounded and PBS washed. This was followed by treatment with 16a/DMSO in culture media. The vehicle DMSO treated cells had almost completely filled in the cleared area, whereas treatment with 7 and 15 μM of compound 16a significantly abrogates motility and invasion potential of A549 cells (Fig. 3). Again staurosporine was used as positive control.62
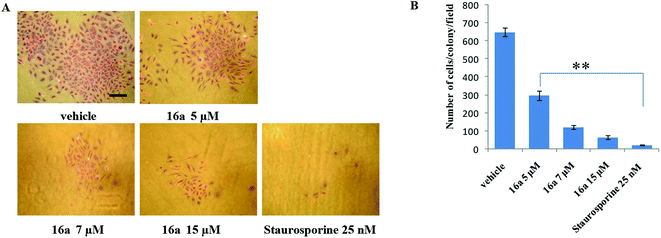 |
| Fig. 2 (A) A549 cells (1 × 103 cells per well) were cultured and treated with various concentration of compound 16a for 24 h at 37 °C and then stained with crystal violet (for details see Result section), number of stained colonies were counted, photographed (magnification, 100×; scale bar, 200 μm) and (B) data was calculated from three independent experiments. Columns mean; bars SD of three independent experiments. **P < 0.01 compared with untreated control. | |
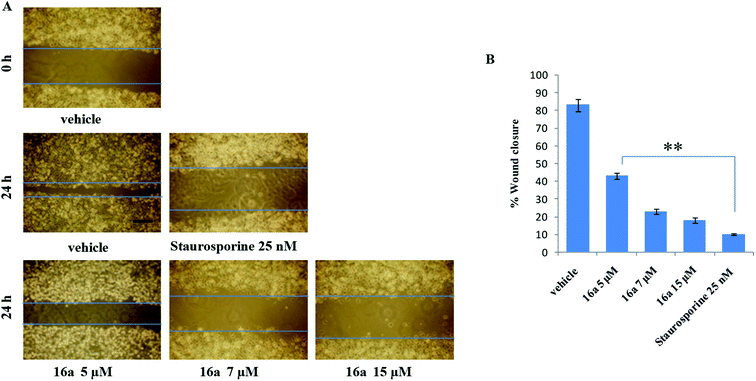 |
| Fig. 3 (A) A549 cells (0.5 × 105 cells per well) were grown to confluence in six well tissue culture plate and scratched with sterile tip, compound 16a was added to cultures as indicated. Scratched areas were photographed (magnification, 100×; scale bar, 200 μm) at zero hour and then subsequently again 24 h later to assess the degree of wound healing. (B) The scratched areas were quantified in three random fields in each treatment, and data were calculated from three independent experiments. Data were calculated from three independent experiments. Columns mean; bars SD of three independent experiments. **P < 0.01 compared with untreated control. | |
2.3. Molecular docking studies
In order to rationalize the obtained biological data and understand the probable mode of binding, the most potent compound (16a) were docked into the crystal structure. On analysis, 16a was found to bind with better affinity on CDK2 and PI3Kγ which supports the in vitro cytotoxic activity data. The interaction figure analysis of compound 16a with CDK2 reveals that the dihydropyrimidinone moiety of 16a is involved in bidentate H-bonding with Leu83 (hydrophobic) amino acid residue and methoxy-benzene moiety is forming a pi–pi stacking with Phe80 (hydrophobic) which favors more affinity of the molecules for CDK2. Additionally, the “O” of methoxy-benzene moiety is for forming third H-bond with Asp-145 of CDK-2. Further the interaction figure analysis of compound 16a with PI3Kγ shows that the dihydropyrimidinone moiety of 16a is involved in bidentate H-bonding with Tyr867 (hydrophobic) and Asp964 (acidic) amino acid residue and esteric carbonyl oxygen is forming H-bonding with the hinge residue Val882 (hydrophobic) (Fig. 4). The three H-bondings enhance the affinity of the 16a molecule for both CDK-2 and PI3Kγ.
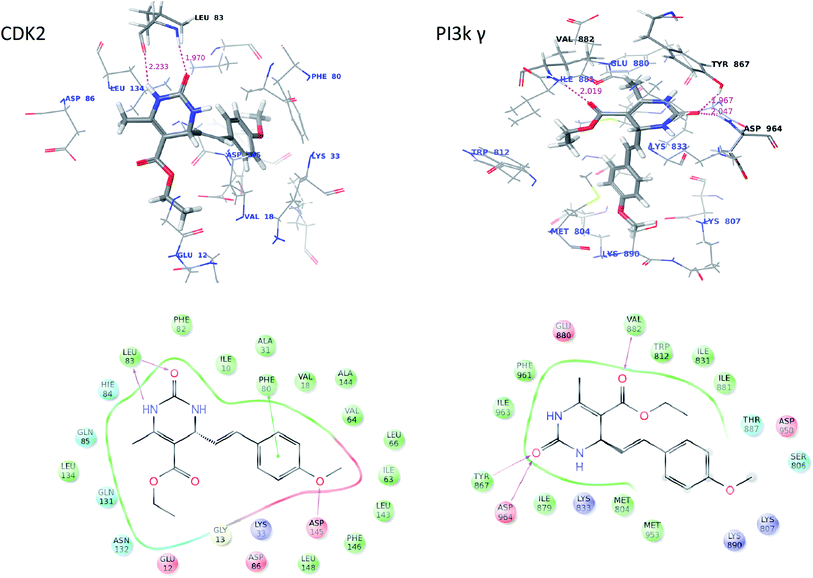 |
| Fig. 4 Molecular docking studies showing probable binding mode of 16a. | |
We concluded earlier from SAR analysis that phenyl moiety as side chain of dihydropyrimidone increased the specificity and cytotoxicity of 16a. So, we did docking studies with 6a too which has one of the simplest aliphatic (propyl) as side chain for comparison. The docking data revealed that only two H-bonding were being formed between 6a and the enzymes, with Leu83 in case of CDK-2 and with Val882 and Glu880 in case of PI3Kγ compared to three in case of 16a. Further the absence of methoxy-benzene moiety in 6a leads to loss of pi–pi stacking. So, this docking comparison explains partially why phenyl moiety containing 16a molecule has increased cytotoxicity.
3. Conclusions
We have reported a catalytic method for the synthesis of dihydropyrimidinones using HPA-Montmorillonite-KSF as an efficient and eco-friendly heterogeneous inorganic catalyst. It is noteworthy to mention that the catalyst is reusable and even after five runs; the catalytic activity of H5PV2W10O40·10H2O was retained as that of the freshly used catalyst. The short reaction times, simple work-up and isolation of the products in high yields with high purity, and mild reaction conditions, are some of the highlights of current procedure. A diverse library of DHPM analogs have been synthesized by using this catalyst and most of the derivatives displayed impressive anti-proliferative effect at 50 μM, with best cytotoxic effect observed for 16a exhibiting the inhibition against all the tested cancer cell lines with most potency against lung (A549) cancer cells as 7.1 ± 0.8 μM while the toxicity against the normal epithelial cells was apparently more than 10 fold higher (>70 μM). However, in vivo studies are warranted to investigate the mechanisms of action responsible for the cytotoxic activity of the most active derivatives.
4. Experimental
4.1. Chemistry
All reagents for chemical synthesis were obtained from Sigma Aldrich and the solvents used in reactions were distilled and dried prior to use. All the chemical reactions were monitored by TLC on 0.25 mm silica gel 60 F254 plates (E. Merck) using 2% ceric ammonium sulphate solution for detection of the spots. Purification of compounds was carried out by column chromatography using silica gel 60–120 mesh stationary phase. 1H NMR and 13C NMR spectra (with chemical shifts expressed in δ and coupling constants in hertz) were recorded on Bruker DPX 200, 400 and DPX 500 instruments using CDCl3 or CD3OD as the solvents with TMS as internal standard. High resolution mass spectra (HRMS) were recorded on Agilent Technologies 6540 instrument and IR recorded on an FT-IR Bruker (270-30) spectrophotometer. Melting points of compounds were recorded on Buchi melting point apparatus B-542.
4.1.1. Preparation of catalyst H5PV2W10O40·10H2O (HPA). Disodium hydrogen phosphate (Na2HPO4, 1.775 g, 25 mmol) was dissolved in 50 mL of water and mixed with vanadium pentoxide (V2O5, 6.1 g, 100 mmol) dissolved in 20 mL of 1 M Na2CO3 and the solution so formed was boiled for 30 min (green colour) and then cooled to room temperature. To this solution, sodium tungstate dihydrate (Na2WO4·2H2O, 41.25 g, 250 mmol) dissolved in 20 mL of water (black colour) was added. This solution was kept at 90 °C for 30 min (bluish green colour), cooled and to it was added 50% sulfuric acid (50 mL) drop wise, a wine red solution (pH = 2) was obtained. Extraction of the solution with diethyl ether (100 mL) afforded the orange-red H5PV2W10O40·10H2O product.The HPA so formed was dissolved in 150 mL water and added dropwise to 500 mL aqueous suspension of 10 g Montmorillonite KSF. This mixture was stirred for 5 h and the water was evaporated over water bath to get a dry powder, which was kept overnight in hot air oven at 110 °C. A portion of this solid was then calcined at 425 °C for 3 h to get a heteropoly acid clay nano hybrid and was named as HCNH. The catalyst was characterized using X-ray powder diffraction (XRD) and scanning electron microscopy as discussed in ESI.†
4.1.2. Preparation of aldehyde 5 (Scheme 2). Compound 5 was prepared by adding selenium dioxide (1.3 mmol) to a solution of osthol (1.3 mmol) dissolved in glacial acetic acid (5 mL) and stirred for about 3 h. On completion of the reaction (monitored by TLC), the contents were poured into crushed ice and extracted with dichloromethane (3 × 50 mL), the organic layer was washed with water (2 × 10 mL) dried over sodium sulfate and concentrated under vacuo to give crude product, which on silica gel column chromatography yielded pure aldehyde in 50% yield. 1H NMR (CDCl3, 400 MHz): δ 9.4 (s, 1H), 7.6 (1H, d, J = 9.5 Hz), 7.3 (1H, d, J = 8.6 Hz), 6.8 (1H, d, J = 8.6 Hz), 6.5 (1H, t, J = 7.3 Hz), 6.2 (1H, d, J = 9.4 Hz), 3.8 (2H, d, J = 7.3 Hz), 3.9 (3H, s), 1.9 (3H, s). 13C NMR (CDCl3, 100 MHz): δ 195.4, 160.79, 160.30, 153.0, 150.74, 143.64, 139.81, 127.38, 114.2, 113.3, 113.1, 107.4, 56.1, 22.8, 9.2. IR (KBr) νmax cm−1: 2923, 1728, 1608, 1497, 1280, 1117, 1093. HR-ESIMS m/z: 259.0793 (calculated for C15H14O4, 258.0892).
4.1.3. Preparation aldehydes 15–20 (Scheme 3). To the ethereal solution of substituted aldehyde (110 mmol), freshly prepared Gigrand's reagent (110 mmol) was added at −5 °C and the contents were stirred for 2 h at 0–5 °C. After the completion of reaction, saturated solution of ammonium chloride (10 mL) was added followed by dilution with water. The product was extracted with ether (2 × 50 mL) and the combined organic layer was washed with water (2 × 20 mL), dried over anhydrous sodium sulphate and concentrated under vacuum to give the corresponding secondary alcohol as a gummy mass (95% yield). To a chilled solution of Scheme 3 products in DMF, phosphoryl chloride was added slowly at 0 °C for 1 hour. The contents were stirred further for 2 hours and then allowed to attain room temperature followed by heating on a water bath for 3 hours. The reaction mixture was cooled and a saturated solution of sodium acetate (15 mL) was added followed by dilution with water. The contents of the reaction mixture were extracted with ethyl acetate (4 × 25 mL), the organic layer was washed with water (3 × 20 mL) and dried over sodium sulfate, filtered and concentrated under vacuo to give crude product, which on silica gel column chromatography yielded the required aldehydes57 (Scheme 3).
4.1.4. General procedure for the synthesis of DHPM derivatives (Scheme 4). A mixture of aldehyde (10 mmol), 1,3-dicarbonyl compound (15 mmol), urea or thiourea (15 mmol) and HPA-Montmorillonite-KSF (2 mol%) was refluxed for 1 h using solvent free conditions and the progress of the reaction was monitored by TLC. Upon completion of the reaction, the mixture was cooled to room temperature and the catalyst was subsequently removed by filtration and the solution was poured on to ice-water (30 mL). The resulting solid product was filtered and recrystalized from ethanol to give the pure products. After removing the reaction products by filtration, the solid catalyst was washed with ethanol and reused for the second run of the Biginelli reaction.
4.1.4.1. Ethyl 4-(4-(6-methoxy-2-oxo-2H-chromen-7-yl)but-2-en-2-yl)-6-methyl-2-oxo-1,2,3,4-tetrahydropyrimidine-5-carboxylate 5a. Yellowish solid, (mp: 144–146 °C). 1H NMR (400 MHz, CDCl3): δ 7.6 (1H, d, J = 9.5 Hz), 7.3 (1H, d, J = 8.6 Hz), 7.2 (1H, s), 6.8 (1H, d, J = 8.6 Hz), 6.5 (1H, t, J = 7.3 Hz), 6.2 (1H, d, J = 9.4 Hz), 4.9 (1H, s), 4.1 (2H, q, J = 8 Hz), 3.9 (3H, s), 3.8 (2H, d, J = 7.6 Hz), 2.3 (3H, s), 1.9 (3H, s), 1.2 (3H, t, J = 8 Hz). 13C NMR (100 MHz, CDCl3): δ 169.4, 161.8, 155.7, 154.6, 151.5, 149.3, 142.8, 134.5, 133.2, 126.1, 119.8, 119.3, 114.7, 106.8, 92.8, 61.5, 56.7, 48.9, 30.3, 18.9, 16.2, 14.6. IR (KBr) νmax cm−1: 3340, 3012, 1728, 1681, 1072, 832. HR-ESIMS m/z: 413.4424 (calculated for C22H24N2O6, 412.4358).
4.1.4.2. Ethyl 1,2,3,4-tetrahydro-6-methyl-2-oxo-4-propylpyrimidine-5-carboxylate 6a. White solid, (mp: 156–158 °C). 1H NMR (400 MHz, CDCl3): δ 4.2 (1H, m), 4.2 (2H, q, J = 6.7 Hz), 2.2 (3H, s), 1.4 (2H, m), 1.28 (4H, m), 0.9 (3H, t, J = 6.9 Hz). 13C NMR (100 MHz, CDCl3): δ 167.8, 156, 149.2, 102.2, 61.1, 51.9, 40.3, 18.4, 18.1, 14.6, 14.2. IR (KBr) νmax cm−1: 1744, 1717, 1637, 1440, 1215. HR-ESIMS m/z: 227.1393 (calculated for C11H18N2O3, 226.1317).
4.1.4.3. Ethyl 4-butyl-1,2,3,4-tetrahydro-6-methyl-2-oxopyrimidine-5-carboxylate 7a. White solid, (mp: 146–149 °C). 1H NMR (400 MHz, CDCl3): δ 4.2 (1H, m), 4.1 (2H, q, J = 7.1 Hz), 2.2 (3H, s), 1.4 (2H, m), 1.2 (7H, m), 0.9 (3H, t, J = 7.4 Hz). 13C NMR (100 MHz, CDCl3): δ 167.4, 154.8, 146.8, 101.5, 59.9, 51.4, 36.8, 31.4, 24, 22.5, 14.3, 14. IR (KBr) νmax cm−1: 1744, 1717, 1705, 1637, 1440, 1107. HR-ESIMS m/z: 241.1546 (calculated for C12H20N2O3, 240.1474).
4.1.4.4. Ethyl-methyl-2-oxo-4-pentyl-1,2,3,4-tetrahydropyrimidine-5-carboxylate 8a. White solid, (mp: 150–152 °C). 1H NMR (400 MHz, CDCl3): δ 4.8 (1H, s), 4.1 (1H, m), 4 (2H, q, J = 7.3 Hz), 3.2 (1H, s), 2.1 (3H, s), 1.4 (2H, m), 1.9 (6H, m), 0.8 (3H, t, J = 7.6 Hz). 13C NMR (100 MHz, CDCl3): δ 167.4, 154.8, 146.8, 101.5, 59.9, 51.4, 31.4, 37.4, 24, 22.5, 18.5, 14.3, 14. IR (KBr) νmax cm−1: 1744, 1705, 1637, 1617, 1440. HR-ESIMS m/z: 255.1703 (calculated for C13H22N2O3, 254.1630).
4.1.4.5. Ethyl-4-hexyl-1,2,3,4-tetrahydro-6-methyl-2-oxo-pyrimidine-5-carboxylate 9a. White solid, (mp: 153–156 °C). 1H NMR (400 MHz, CDCl3): δ 4.6 (1H, s), 4.1 (1H, m), 4 (2H, q, J = 7.2 Hz), 3 (1H, s), 2.1 (3H, s), 1.4 (2H, m), 1.2 (8H, m), 0.8 (3H, t, J = 7.3 Hz). 13C NMR (100 MHz, CDCl3): δ 166.7, 153.2, 145.8, 100.3, 59.5, 50.4, 32.2, 24.3, 23.1, 19.29, 15.3, 14.2, 13.9. IR (KBr) νmax cm−1: 1744, 1705, 1637, 1617, 1440. HR-ESIMS m/z: 269.1853 (calculated for C14H24N2O3, 268.1787).
4.1.4.6. Ethyl-4-(3-hydroxystyryl)-1,2,3,4-tetrahydro-6-methyl-2-oxopyrimidine-5-carboxylate 10a. Yellowish powder solid, (mp: 135–137 °C). 1H NMR (400 MHz, CDCl3): δ 7.3 (1H, m), 7.3 (1H, m), 7.2 (1H, m), 7.2 (1H, m), 6.5 (1H, d, J = 16 Hz), 6.2 (1H, dd, J = 3.9, 16 Hz), 4.2 (1H, m), 4.1 (2H, q, J = 7.4 Hz), 2.3 (3H, s), 1.3 (3H, t, J = 7.3 Hz). 13C NMR (100 MHz, CDCl3): δ 167.6, 158.4, 154.5, 144.6, 130.6, 130.1, 129.7, 128.8, 127.6, 118.3, 109.7, 101.8, 59.7, 53.7, 18.4, 14.9. IR (KBr) νmax cm−1: 3240, 3117, 2960, 1693, 1514, 1462. HR-ESIMS m/z: 303.1339 (calculated for C16H18N2O4, 302.1267).
4.1.4.7. Ethyl-4-(3,4-dihydronaphthalen-2-yl)-6-methyl-2-oxo-1,2,3,4-tetrahydropyrimidine-5-carboxylate 11a. Pale yellow solid, (mp: 181–183 °C). 1H NMR (400 MHz, CDCl3): δ 7.1–7.2 (4H, m), 6.3 (1H, s), 5.3 (1H, s), 5 (1H, s), 4.1 (2H, q, J = 7.6 Hz), 2.8 (2H, t, J = 7.6 Hz), 2.3 (3H, s), 2.2 (2H, m), 1.2 (3H, t, J = 7.5 Hz). 13C NMR (100 MHz, CDCl3): δ 167.7, 155.7, 149.5, 142.3, 136.1, 135.2, 128.2, 128.4, 127.5, 125, 99.8, 61.1, 29.2, 23.9, 18.3, 14.8. IR (KBr) νmax cm−1: 3240, 3117, 2960, 1693, 1728, 1681, 1608, 1514. HR-ESIMS m/z: 313.1546 (calculated for C18H20N2O3, 312.1474).
4.1.4.8. Ethyl-4-(1-chloro-3,4-dihydronaphthalen-2-yl)-6-methyl-2-oxo-1,2,3,4-tetrahydropyrimidine-5-carboxylate 12a. White solid, (mp: 162–164 °C). 1H NMR (400 MHz, CDCl3): δ 7.6 (1H, m), 7.2 (2H, m), 7.1 (1H, m), 5.8 (1H, s), 5.3 (1H, s), 5.2 (1H, s), 4.1 (2H, q, J = 6.7 Hz), 2.8 (2H, m), 2.3 (3H, s), 2.4 (2H, m), 1.1 (3H, t, J = 7.3 Hz). 13C NMR (100 MHz, CDCl3): δ 167.5, 154.8, 150.3, 137.7, 137.4, 134.14, 129.5, 128.3, 127.9, 127.4, 126.2, 98.6, 61.4, 29.2, 25.1, 18.9, 15.2. IR (KBr) νmax cm−1: 3235, 2936, 1693, 1700, 1681, 1596. HR-ESIMS m/z: 347.1156 (calculated for C18H19ClN2O3, 346.1084).
4.1.4.9. Ethyl-4-((Z)-3-(methoxycarbonyl)-1-chloro-1-(3,4-dimethoxyphenyl)prop-1-en-2-yl)-1,2,3,4-tetrahydro-6-methyl-2-oxopyrimidine-5-carboxylate 13a. Brown powder solid, (mp: 126–128 °C). 1H NMR (400 MHz, CDCl3): δ 7.1 (1H, d, J = 2.7 Hz), 7 (1H, dd, J = 2.3 & 8.3 Hz), 6.9 (1H, d, J = 8.3 Hz), 4.5 (1H, s), 4.2 (2H, q, J = 7.2 Hz), 3.6 (6H, s), 3.6 (3H, s), 2.7 (2H, s), 2.2 (3H, s), 1.3 (3H, t, J = 6.9 Hz). 13C NMR (100 MHz, CDCl3): δ 174, 169.9, 155.7, 151.3, 150.1, 148.5, 143.4, 134.3, 123.1, 121.6, 113.8, 113, 95.1, 61.5, 57.6, 56.7, 51.8, 36.3, 16.3, 14.7. IR (KBr) νmax cm−1: 3195, 2860, 1693, 1514, 1462. HR-ESIMS m/z: 453.1423 (calculated for C21H25ClN2O7, 452.1350).
4.1.4.10. Ethyl-1,2,3,4-tetrahydro-6-methyl-4-(2,2-dimethylchroman-6-yl)-2-oxo-pyrimidine-5-carboxylate 14a. Yellowish solid, (mp: 134–137 °C). 1H NMR (400 MHz, CDCl3): δ 7.5 (1H, s), 7 (2H, m), 6.6 (1H, d, J = 2 Hz), 5.4 (1H, m), 5.3 (1H, s), 4.1 (2H, q, J = 7.6 Hz), 2.7 (2H, t, J = 7.5 Hz), 2.3 (3H, s), 1.7 (2H, t, J = 7.5 Hz), 1.3 (6H, s), 1.2 (3H, t, J = 7.6 Hz). 13C NMR (100 MHz, CDCl3): δ 165.8, 153.7, 145.9, 135, 127.7, 125.7, 120.9, 117.2, 101.6, 74.3, 59.9, 55.2, 54, 32.6, 30.2, 26.8, 22.5, 18.6, 14.1. IR (KBr) νmax cm−1: 3335, 3156, 1693, 1700, 1608, 1576, 1488. HR-ESIMS m/z: 345.1822 (calculated for C19H24N2O4, 344.1736).
4.1.4.11. Ethyl-1,2,3,4-tetrahydro-6-methyl-4-((Z)-2-(2,2-dimethylchroman-6-yl)vinyl)-2-oxo-pyrimidine-5-carboxylate 15a. Yellowish solid, (mp: 120–123 °C) 1H NMR (400 MHz, CDCl3): δ 7.3 (1H, s), 7.2 (2H, m), 6.7 (1H, dd, J = 8.5 & 2.4 Hz), 6.2 (1H, d, J = 16 Hz), 5.9 (1H, dd, J = 3.2 & 16.0 Hz), 4.5 (1H, d, J = 4.0 Hz), 4.1 (2H, q, J = 7.3 Hz), 2.4 (3H, s), 2.7 (2H, t, J = 7.0 Hz) 1.7 (2H, t, J = 7.0 Hz), 1.2 (3H, t, J = 7.1 Hz), 1.2 (6H, s). 13C NMR (100 MHz, CDCl3): δ 168.6, 155.9, 154.6, 152.4, 130.5, 129, 128, 125.8, 122.8, 115.1, 100.7, 78.3, 61.5, 34.4, 27.4, 25, 19.5, 14.4. IR (KBr) νmax cm−1: 3240, 3117, 2960, 1693, 1514, 1097. HR-ESIMS m/z: 371.11979 (calculated for C21H26N2O4, 370.1893).
4.1.4.12. Ethyl-4-(4-methoxystyryl)-6-methyl-2-oxo-1,2,3,4-tetrahydropyrimidine-5-carboxyl-ate 16a. Colourless liquid. 1H NMR (400 MHz, CDCl3): δ 7.2 (1H, s), 7 (2H, d, J = 7.9 Hz), 6.8 (2H, d, J = 8 Hz), 6.2 (1H, d, J = 16 Hz), 5.9 (1H, dd, J = 3.2, 16 Hz), 5.5 (1H, s), 4.5 (1H, d, J = 4 Hz), 4.1 (2H, q, J = 7.3 Hz), 3.7 (3H, s), 2.4 (3H, s), 1.2 (3H, t, J = 7.1 Hz). 13C NMR (100 MHz, CDCl3): δ 167.6, 154.5, 144.6, 132.9, 129.7, 129.3, 127.4, 127.3, 118.3, 109.7, 101.8, 85.3, 59.7, 55.2, 36.4, 19.5, 14.4. IR (KBr) νmax cm−1: 3240, 2960, 1693, 1514, 1462, 1097. HR-ESIMS m/z: 317.1495 (calculated for C17H20N2O4, 316.1423).
4.1.4.13. Ethyl 4-(2,3-dimethoxystyryl)-1,2,3,4-tetrahydro-6-methyl-2-oxopyrimidine-5-carboxylate 17a. Yellowish liquid, 1H NMR (400 MHz, CDCl3): δ 7.2 (1H, s), 6.8 (1H, d, J = 16 Hz), 6.5 (3H, m), 5.9 (1H, dd, J = 3.2, 16 Hz), 5.5 (1H, s), 4.5 (1H, d, J = 4 Hz), 4.1 (2H, q, J = 7.4 Hz), 3.7 (6H, s), 2.4 (3H, s), 1.2 (3H, t, J = 7.1 Hz). 13C NMR (100 MHz, CDCl3): δ 167.7, 156.8, 155.7, 151.2, 144.6, 130, 127.1, 122.5, 119.9, 104.5, 101.9, 93.8, 59.7, (2C, 55.3), 36.4, 19.5, 14.3. IR (KBr) νmax cm−1: 3240, 2960, 1693, 1514. HR-ESIMS m/z: 347.1601 (calculated for C18H22N2O5, 346.1529).
4.1.4.14. Ethyl 4-(3,4,5-trimethoxystyryl)-1,2,3,4-tetrahydro-6-methyl-2-oxopyrimidine-5-carboxylate 18a. Greyish liquid. 1H NMR (400 MHz, CDCl3): δ 7.2 (1H, s), 6.5 (2H, d, J = 16 Hz), 6.2 (2H, s), 5.9 (1H, dd, J = 3.2, 16 Hz), 5.5 (1H, s), 4.5 (1H, d, J = 3.4 Hz), 4.1 (2H, q, J = 7.6 Hz), 3.7 (9H, s), 2.4 (3H, s), 1.2 (3H, t, J = 7.1 Hz). 13C NMR (100 MHz, CDCl3): δ 167.8, 156.8, (2C, 155.7), 150.2, 144.6, 130, 127.1, 122.5, (2C, 104.5), 101.9, 59.7, 56.3, (2C, 55.3), 36.4, 19.5, 14.34. IR (KBr) νmax cm−1: 3240, 2960, 1693, 1514, 1462. HR-ESIMS m/z: 377.1822 (calculated for C19H24N2O6, 376.1634).
4.1.4.15. Ethyl-4-(2,4-dimethoxystyryl)-1,2,3,4-tetrahydro-6-methyl-2-oxopyrimidine-5-carboxylate 19a. Yellowish liquid. 1H NMR (400 MHz, CDCl3): δ 7.2 (1H, s), 7 (1H, m), 6.8 (1H, d, J = 16 Hz), 6.2 (2H, m), 5.96 (1H, dd, J = 3.2, 16 Hz), 5.5 (1H, s), 4.5 (1H, d, J = 4 Hz), 4.1 (2H, q, J = 7.3 Hz), 3.7 (6H, s), 2.4 (3H, s), 1.2 (3H, t, J = 7.1 Hz). 13C NMR (100 MHz, CDCl3): δ 167.7, 159.8, 156.7, 154.2, 144.6, 130, 127.1, 122.5, 119.9, 104.5, 101.9, 93.8, 59.7, (2C, 55.3), 36.4, 19.5, 14.3. IR (KBr) νmax cm−1: 3240, 2960, 1693, 1514, 1097. HR-ESIMS m/z: 347.1611 (calculated for C18H22N2O5, 346.1529).
4.1.4.16. Ethyl-4-((E)-2-(benzo[d][1,3]dioxol-6-yl)vinyl)-1,2,3,4-tetrahydro-6-methyl-2-oxo pyrimidine-5-carboxylate 20a. White solid, (mp: 181–184 °C). 1H NMR (200 MHz, CDCl3): δ 7.8 (1H, s), 6.9 (2H, m), 6.7 (1H, m), 6.6 (1H, d, J = 2.2 Hz), 6.3 (1H, d, J = 16.0 Hz), 6.1 (2H, s), 5.9 (1H, dd, J = 3.4 & 16.0 Hz), 5.6 (1H, s), 4.61 (1H, d, J = 3.4 Hz), 4.1 (2H, q, J = 7.2 Hz), 2.3 (3H, s), 1.2 (3H, t, J = 7.2 Hz). 13C NMR (100 MHz, CDCl3): δ 165.7, 154.2, 148.8, 146.9, 145.3, 136.8, 129.2, 124.3, 120.8, 109.1, 107.2, 102, 101.8, 60.2, 55.6, 19.4, 14.5. IR (KBr) νmax cm−1: 3230, 2930, 1710, 1655, 1520, 1107. HR-ESIMS m/z: 331.1309 (calculated for C18H22N2O5, 330.1216).
4.1.4.17. Ethyl-4-(benzo[d][1,3]dioxol-6-yl)-1,2,3,4-tetrahydro-6-methyl-2-oxopyrimidine-5-carboxylate 21a. White solid, (mp: 176–178 °C). 1H NMR (200 MHz, CDCl3): δ 7.9 (1H, s), 6.8 (1H, d, J = 8.7 Hz), 6.7 (1H, m), 6.7 (1H, d, J = 1.6 Hz), 5.9 (2H, s), 5.6 (1H, s), 5.3 (1H, s), 4 (2H, q, J = 7 Hz), 2.3 (3H, s), 1.18 (3H, t, J = 7 Hz). 13C NMR (100 MHz, CDCl3): δ 165.6, 153.6, (2C, 147.2), 146.3, 137.9, 120, 108.1, 107, (2C, 101.1), 60, 55.4, 18.5, 14.2. IR (KBr) νmax cm−1: 3225, 2928, 1710, 1651, 1514, 1097. HR-ESIMS m/z: 305.1131 (calculated for C18H22N2O5, 304.1059).
4.2. Biology
RPMI-1640 medium, Dulbecco's modified eagle's medium, penicillin, streptomycin, fetal calf serum, sodium bicarbonate, phosphate buffer saline, trypsin, gentamycin sulphate, tryphan blue, ethanol, DMSO, paraformaldehyde were purchased from Sigma Chemicals Co. Glacial acetic acid from Fischer scientific, PBS and trichloroacetic acid (TCA) from Merck specialties private limited. All the human cancer cell lines (Colo-205, PC-3, THP-1, and A549) as well as normal epithelial cell line (fR-2) were obtained from National Center for Cell Science, Ganeshkhind, Pune-4111007 (India) and National Cancer Institute, Biological Testing Branch DTP/DCTD/NCI, Frederick Cancer Research and Development Center, Fairview Center, Suite 205, 1003 West 7th Street, Frederick, MD 21701-8527 (USA).
4.2.1. MTT [3-(4,5-dimethylthiazolyl-2)-2,5-diphenyltetrazolium bromide] assay. To determine IC50 value, all the derivatives were further evaluated against colon (Colo-205), prostate (PC-3), leukemia (THP-1) and lung (A549) cancer cell lines including normal epithelial cell line (fR-2) using MTT assay. Briefly, Colo-205, PC-3, THP-1, A549 and fR-2 cells (3 × 103 cells per mL per 100 μL per well) were plated into a 96 well tissue culture plate. Cells were incubated either in RPMI-1640 or Dulbecco's modified eagle's media containing 10% fetal calf serum, supplemented with 100 units per mL penicillin, 100 mg L−1 streptomycin in a humidified atmosphere in 5.0% CO2 at 37 °C. After overnight cell attachment, the cells were treated with test materials at concentrations of 0.07, 0.7, 7.0 and 70.0 μM for 24 h and then further treated with MTT (250 μg mL−1) for 3–4 h. Inhibition of formation of colored MTT formazan was taken as an index of cytotoxicity activity. The amount of colored formazan derivative was determined by measuring optical density (OD) using TECAN microplate reader (Infinite M200 PRO) at 570 nm and percentage cell growth was calculated by the formula (test OD/non-treated OD) × 100. Further the graphs and IC50 values of different analogs and controls in cancer cells of different tissue origin used for screening were determined on excel.63
4.2.2. Clonogenic (colony formation) assay. To evaluate whether compound 16a inhibits clonogenic property of cancer cells, colony formation assay was performed in A549 cells. Briefly, A549 cells were plated at a seeding density of (1 × 103 cells per well) in 6 well tissue culture grade plates. After 24 h the culture medium was changed and new medium was added and cells were exposed to various concentrations of 16a (5, 7 and 15 μM) along with vehicle DMSO for 24 h at 37 °C incubator in 5% CO2. After the treatment, the plates were placed in an incubator with fresh media only for a time equivalent to at least six potential cell divisions (to give colonies of >50 cells). Later on, the obtained colonies were fixed with 4% paraformaldehyde and were stained with 0.5% crystal violet solution. The colonies from the plates were counted and averaged from the observed fields randomly (n = 3) and photographed with Olympus C-7070 wide 700M inverted microscope camera (Fig. 2). Staurosporine (25 nM) was used as positive control while as DMSO was used as vehicle.
4.2.3. Scratch motility (wound healing) assay. A549 cells were seeded in a 6 well plate at a concentration of (5.5 × 105 cells per well) and allowed to form a confluent monolayer for 24 h, it was then serum starved for 24 h. After that the monolayer was scratched with a sterile pipette tip (200 μL), washed with serum free medium to remove floated and detached cells and photographed (time 0 h). Cells were successively treated in medium containing low serum (1.0%) in presence of different concentrations of 16a (5, 7 and 15 μM) along with vehicle DMSO for 24 h. Staurosporine (25 nM) was used as positive control while as DMSO was used as vehicle.Wounded areas were progressively photographed with Olympus C-7070 with 700M camera (100× magnification). The percentage of wound closure was estimated by the following equation:
Wound closure% = [1 − (wound area at t1/wound area at t0) × 100] |
where
t1 is the time after wounding when cells were treated for 24 h and
t0 is the time immediately after wounding.
4.3. Molecular docking studies
All the computational studies were carried out in the Schrodinger suite 2012 molecular modeling software. The 2D structure of the ligands was built in the maestro window. The structures were then converted to their respective 3D structures, with various conformers, tautomers and ionization states using the Ligprep and Confgen modules.64 The three dimensional structure of CDK2 and PI3Kγ i.e., PDB ID 2DUV and PDB ID 1E7U respectively were downloaded from Protein Data Bank.65 The receptor was prepared for docking using the Protein Preparation wizard. Extra precision (XP) scoring function of Glide was used for carrying out the docking studies.66
Conflicts of interest
There are no conflicts to declare.
Acknowledgements
The author (SF) is grateful to DST SERB, India for providing research funding bearing the Project No: TAR/2018/000088. The authors (FAA, AA) are also grateful to King Saud University, Riyadh, Saudi Arabia for providing research funding bearing the Researchers Supporting Project Number RSP-2020/160.
References
- M. Gordaliza, Clin. Transl. Oncol., 2007, 9, 767–780 CrossRef CAS.
- A. J. V. Wangelin, H. Neumann, D. Grdes, S. Klaus, D. Strübing and M. Beller, Chem. – Eur. J., 2003, 9, 4286–4294 CrossRef.
- P. A. Wender, S. T. Handy and D. L. Wright, Chem. Ind., 1997, 6, 765 Search PubMed.
- J. Zhu and H. Bienaymee, Multicomponent Reactions, Willey-VCH, Weinheim, Germany, 2005 Search PubMed.
- A. Domling, Chem. Rev., 2006, 106, 17 CrossRef.
- D. J. Ramon and M. Yus, Angew. Chem., 2005, 44, 1602 CrossRef CAS.
- C. Simon, T. Constantieux and J. Rodriguez, Eur. J. Org. Chem., 2004, 24, 4957–4980 CrossRef.
- J. Zhu, Eur. J. Org. Chem., 2003, 7, 1133 CrossRef.
- R. V. A. Orru and M. de. Greef, Synthesis, 2003, 10, 1471 CrossRef.
- L. F. Tietze, F. Haunert, M. Shibasaki, J. F. Stoddart and F. Vgtle, Stimulating Concepts in Chemistry, Wiley-VCH, Weinhei, 2000, vol. 39, pp. 3168–3210 Search PubMed.
- H. Bienayme, C. Hulme, G. Oddon and P. Schmitt, Chem. – Eur. J., 2000, 6, 3321 CrossRef CAS.
- L. F. Tietze and A. Modi, Med. Res. Rev., 2000, 20, 304–322 CrossRef CAS.
- M. Bell, K. Frisch and K. A. Jorgensen, J. Org. Chem., 2006, 71, 5407–5410 CrossRef CAS.
- R. W. Armstrong, A. P. Combs, P. A. Tempest, S. D. Brown and T. A. Keating, Acc. Chem. Res., 1996, 29, 123 CrossRef CAS.
- P. Eilbracht, L. Barfacker, C. Buss, C. Hollmann, B. E. Kitsos-Rzychon, C. L. Kranemann, T. Rische, R. Roggenbuck and A. Schimdt, Chem. Rev., 1999, 99, 3329 CrossRef CAS.
- U. Bora, A. Saikia and R. C. Boruah, Org. Lett., 2003, 5, 435–438 CrossRef CAS.
- C. O. Kappe, Acc. Chem. Res., 2000, 33, 879–888 CrossRef CAS.
- I. Ugi, Pure Appl. Chem., 2001, 73, 187–191 CAS.
- D. J. Ramon and M. Yus, Angew. Chem., Int. Ed., 2005, 44, 1602 CrossRef CAS.
- A. Fayol and J. Zhu, Org. Lett., 2005, 7, 239–242 CrossRef CAS.
- E. Rajanarendar, S. Raju, M. N. Reddy, S. R. Krishna, L. H. Kiran, A. R. N. Reddy and Y. N. Reddy, Eur. J. Med. Chem., 2012, 50, 274–279 CrossRef CAS.
- J. B. Sperry and D. L. Wright, Curr. Opin. Drug Discov. Dev., 2005, 8, 723–740 CAS.
- R. E. Ziegert, J. Toraeng, K. Knepper and S. Braese, J. Comb. Chem., 2005, 7, 147–169 CrossRef CAS.
- C. Hulme, in Multicomponent Reactions, ed. J. Zhu and H. Bienaymé, Wiley-VCH, Weinheim, 2005, p. 311 Search PubMed.
- L. Garuti, M. Roberti and D. Pizzirani, Med. Chem., 2007, 7, 481–490 CAS.
- C. Gil and S. J. Braese, Comb. Chem., 2009, 11, 175–197 CrossRef CAS.
- W. A. Loughlin, J. D. A. Tyndall, M. P. Glenn and D. P. Fairlie, Chem. Rev., 2004, 104, 6085–6117 CrossRef CAS.
- P. G. Baraldi, M. A. Tabrizi, S. Gessi and P. A. Borea, Chem. Rev., 2008, 108, 238–263 CrossRef CAS.
- E. Petricci, C. Mugnaini, M. Radi, A. Togninelli, C. Bernardini, F. Manetti, M. C. Parlato, M. L. Renzulli, M. Alongi, C. Falciani, F. Corelli and M. Botta, Arkivoc, 2006, 7, 452 Search PubMed.
- I. M. Lagoja, Chem. Biodiversity, 2005, 2, 1 CrossRef CAS.
- P. Biginelli, Gazz. Chim. Ital., 1893, 23, 360–461 Search PubMed.
- J. Lloyd, H. J. Finlay, K. Atwal, A. Kover, J. Prol, L. Yan, R. Bhandaru, W. Vaccaro, T. Huynh, C. S. Huang, M. Conder, T. Jenkins, H. Sun, D. Li and P. Levesque, Bioorg. Med. Chem. Lett., 2009, 19, 5469 CrossRef CAS.
- W. Vacarro, T. Huynh, J. Lloyd, K. S. Atwal, H. J. Finlay, P. C. Levesque, M. L. Conder, T. Jenkins-West, H. Shi and L. Sun, Bioorg. Med. Chem. Lett., 2008, 18, 6381 CrossRef.
- J. Lloyd, H. J. Finlay, W. Vacarro, T. Hyunh, A. Kover, R. Bhandaru, L. Yan, K. Atwal, M. L. Conder, T. Jenkins-West, H. Shi, C. Huang, D. Li, H. Sun and P. Levesque, Bioorg. Med. Chem. Lett., 2010, 20, 1436–1439 CrossRef CAS.
- C. O. Kappe, Eur. J. Med. Chem., 2000, 35, 1043–1052 CrossRef CAS.
- S. Chitra, D. Devanathan and K. Pandiarajan, Eur. J. Med. Chem., 2010, 45, 367–371 CrossRef CAS.
- M. B. Deshmukh, S. M. Salunkhe, D. R. Patil and P. V. Anbhule, Eur. J. Med. Chem., 2009, 44, 2651–2654 CrossRef CAS.
- T. U. Mayer, T. M. Kapoor, S. J. Haggarty, R. W. King, S. L. Schreiber and T. J. Mitchison, Science, 1999, 286, 971–974 CrossRef CAS.
- L. Ye, J. Liu, R. Zhang, Y. Guo, H. Wang, Q. Meng, Y. Sun and Z. Liu, Molecules, 2019, 24, 891 CrossRef.
- H. Y. K. Kaan, V. Ulaganathan, O. Rath, H. Prokopcov, D. Dallinger, C. O. Kappe and F. Kozielski, J. Med. Chem., 2010, 53, 5676–5683 CrossRef CAS.
- C. M. Wright, R. J. Chovatiya, N. E. Jameson, D. M. Turner, G. Zhu, S. Werner, D. Huryn, M. Pipas, J. M. Billy, W. Day, P. Wip and J. L. Brodskya, Bioorg. Med. Chem., 2008, 16, 3291 CrossRef CAS.
- O. C. Agbaje, O. O. Fadeyi, S. A. Fadeyi, L. E. Myles and C. O. Okoro, Bioorg. Med. Chem. Lett., 2011, 21, 989 CrossRef CAS.
- B. R. P. Kumar, G. Sankar, R. B. N. Baig and S. Chandrashekaran, Eur. J. Med. Chem., 2009, 44, 4192–4198 CrossRef.
- D. A. Ibrahim and A. M. El-Metwally, Eur. J. Med. Chem., 2010, 45, 1158–1166 CrossRef CAS.
- K. K. Pasunooti, H. Chai, C. N. Jensen, B. K. Gorityala, S. Wang and X. W. Liu, Tetrahedron Lett., 2011, 52, 80–82 CrossRef CAS.
- M. Wu, J. Yu, W. Zhao, J. Wu and S. Cao, J. Fluorine Chem., 2011, 132, 155–159 CrossRef CAS.
- J. Svetlik and V. Kettmann, Tetrahedron Lett., 2011, 52, 1062–1066 CrossRef CAS.
- P. M. Kumar, K. S. Kumar, S. R. Poreddy, P. K. Mohakhud, K. Mukkanti and M. Pal, Tetrahedron Lett., 2011, 52, 1187–1191 CrossRef CAS.
- T. Okuhara, N. Mizuno and M. Misono, Adv. Catal., 1996, 41, 113–252 CAS.
- M. M. Heravi and S. Sadjadi, J. Iran. Chem. Soc., 2009, 6, 1–54 CrossRef CAS.
- M. M. Heravi, K. Bakhtiari and F. F. Bamoharram, Catal. Commun., 2006, 7, 373–376 CrossRef CAS.
- I. V. Kozhevnikov, Chem. Rev., 1998, 98, 171–198 CrossRef CAS.
- A. Shaabani, A. Bazgir and F. Teimouri, Tetrahedron Lett., 2003, 44, 857–859 CrossRef CAS.
- P. T. Anastas and J. C. Warner, Green Chemistry, 1998 Search PubMed.
- Y. Ma, C. Qian, L. Wang and M. Yang, J. Org. Chem., 2000, 65, 3864–3868 CrossRef CAS.
- A. Dondoni and A. Massi, Tetrahedron Lett., 2001, 42, 7975–7978 CrossRef CAS.
- S. Koul, J. L. Koul, S. C. Taneja, K. L. Dhar, D. S. Jamwal, K. Singh, R. K. Reen and J. Singh, Bioorg. Med. Chem., 2000, 8, 251–268 CrossRef CAS.
- P. Y. Chen, J. D. Wu, K. Y. Tang, C. C. Yu, Y. H. Kuo, W. B. Zhong and C. K. Lee, Molecules, 2013, 18, 7600–7608 CrossRef CAS.
- R. Danesi, W. D. Figg, E. Reed and C. E. Myers, Mol. Pharmacol., 1995, 47, 1106–1111 CAS.
- J. E. Liebmann, J. A. Cook, C. Lipschultz, D. Teague, J. Fisher and J. B. Mitchell, Br. J. Cancer, 1993, 68, 1104–1109 CrossRef CAS.
- L. Zhang, Z. Wang, T. Khishignyam, T. Chen, C. Zhou, Z. Zhang, M. Jin, R. Wang, Y. Qiu and D. Kong, Biomed. Pharmacother., 2018, 103, 1069–1078 CrossRef CAS.
- Y. Wang, H. Yang, H. Liu, J. Huang and X. Song, BMC Cancer, 2009, 9(174), 1–12 Search PubMed.
- S. Farooq, S. U. Rehman, A. Hussain, A. Hamid, M. A. Qurishi and S. Koul, Eur. J. Med. Chem., 2014, 84, 545–554 CrossRef CAS.
- K. S. Watts, P. Dalal, R. B. Murphy, W. Sherman, R. A. Friesner and J. C. Shelley, J. Chem. Inf. Model., 2010, 50, 534–546 CrossRef CAS.
- J. Lee, T. Park, S. Jeong, K. H. Kim and C. Hong, Bioorg. Med. Chem. Lett., 2007, 17, 1284–1287 CrossRef CAS.
- T. A. Halgren, R. B. Murphy, R. A. Friesner, H. S. Beard, L. L. Frye, W. T. Pollard and J. L. Banks, J. Med. Chem., 2004, 47, 1750–1759 CrossRef CAS.
Footnote |
† Electronic supplementary information (ESI) available. See DOI: 10.1039/d0ra09072g |
|
This journal is © The Royal Society of Chemistry 2020 |
Click here to see how this site uses Cookies. View our privacy policy here.