DOI:
10.1039/C8AN01811A
(Paper)
Analyst, 2019,
144, 536-542
Two aggregation-induced emission (AIE)-active reaction-type probes: for real-time detecting and imaging of superoxide anions†
Received
19th September 2018
, Accepted 23rd October 2018
First published on 24th October 2018
Abstract
Fluorescent probes are powerful tools for investigating reactive oxygen species (ROS) in living organisms. The overproduced “primary” ROS of superoxide anions (O2˙−) cause a chain of oxidative damage. In order to monitor O2˙− level fluctuations in living cells, we synthesized two reaction-type probes of TPA-DHP-1,2,3 and TPA-PPA-1,2,3, which were composed of an electron-rich triphenylamine (TPA) and the very active functional groups of dihydropyridine (DHP) and pyridinium (PPA). Intriguingly, DHP and PPA were able to carry out easy proton abstractions and nucleophilic reactions in the presence of O2˙−, resulting in the corresponding products with sharp wavelength shifts, and elevated fluorescence intensities. Therefore, undesirable background fluorescence interference can be reduced during the monitoring and imaging process. Meanwhile, the developed dual-channel monitoring strategy not only provides observations of the O2˙− level fluctuations, but could also be employed to image the dynamic accumulation process of probes in the different cell organelles. Therefore, the design could provide a simple, accurate and universal platform for biological applications in future research work.
Introduction
Intracellular redox homeostasis is closely related to the function and health of cells;1 The disruption of redox homeostasis is associated with some severe human diseases.2 Cells are able to maintain their redox balance spontaneously depending on a serious elegant self-regulation system.3 Among them, redox-active molecules play an important role in buffering significant redox state changes appropriately.4 These molecules live through inter-reaction and inter-conversion to maintain the dynamic balance of the intracellular redox state,5 among which one class of important molecules that should be figured out is of reactive oxygen species (ROS).6 ROS are normally produced intracellularly during the metabolism process. ROS mainly include several oxygen-containing species, such as the superoxide anion (O2˙−), hydrogen peroxide (H2O2), singlet oxygen (1O2), hydroxyl radical (˙OH), lipid peroxy radical (ROO˙), ozone (O3), hypochlorous acid/hypochlorite (HOCl/ClO−).7 It is well known that O2˙− is the “primary” ROS in living systems, therefore, it can serve as a precursor for many ROS. Correspondingly, overproduction of O2˙− causes oxidative stress and disruption of the redox balance.8 In this way, having a clear understanding of the relationship between the disease and changes in the concentration of O2˙− is of practical importance for physiological and pathological fields. Furthermore, unraveling the location of O2˙− in subcellular compartments can lead to advances in elucidating its chemistry and biology.
Compared with reported sensing methods such as electron spin resonance (ESR) and mass spectrometry (MS), molecular fluorescent probes have advantages of quick response, excellent sensitivity and are non-invasive.9 As usual, redox mechanism (oxidation ability of O2˙−) and non-redox mechanism (nucleophilicity or other reactive nature of O2˙−) are employed to explore reaction-based fluorescence probes for O2˙− detection.10 Representative studies are as follows: in 2017, Tang et al. reported aggregation-induced emission (AIE)-active probes with two-channel signal output, which could be used as a fluorescent probe for selective imaging of superoxide anions in living cells.11 Meanwhile, a series of extraordinary works could be referred from Tang et al.12 (for instance, probes of TPE-CLA, MF-DBZH, ER-BZT, TCA, FO-PTe). However, several limitations still exist in these O2˙− fluorescent probes. For instance, some of them often suffer from interference from other ROS, delayed response time, and errors from the detection environment, and background fluorescence interference. Thus, alternative O2˙− fluorogenic probes with distinct advantages, such as high specificity, instantaneous response, and off-on optical sensing performance, remain in urgent demand for bioimaging in cells and in vitro.13 In our previous studies, we synthesized a series of AIE-active materials; all of them demonstrated excellent response behaviours toward external stimulus, and also the cell imaging applications were explored.14 Now, our group has been looking forward to synthesize some fluorescent materials with the ability to monitor biological samples; in particular, the “primary” ROS, O2˙−, in living systems. Normally, the monitoring sensitivity and selectivity depend on two important facts: firstly, the probes are able to carry out easy chemical transformations with the assistance of O2˙−. For instance, phenols have been oxidized into benzoquinones in the presence of O2˙−,12a which results in an intense fluorescence resonance energy transfer (FRET) and strong fluorescence emission, in addition, it also serves as a powerful fluorescence imaging tool for dynamic tracking of O2˙− in live cells and in vivo. Secondly, the easy transformation could bring out very different emission signal changes.8,11 In particular, a large emission wavelength shift or/and multiple emission intensity enhancement (off-on) are especially desirable.15 Following these points, we designed and synthesized two different interaction models for O2˙− detection (Scheme 1). 1. It is well known that the half-Hantzsch intermediate of dihydropyridine (DHP) can be easily converted into pyridine in the presence of some mild oxidants.16 Thus, O2˙− is able to facilitate this transformation, leading to the formation of more electron-accepting groups of pyridine. 2. Pyridinium decomposition reactions can be promoted by nucleophilic O2˙−, which result in the transformation of pyridinium to pyridine. The totally different electron-accepting and donating systems lead to sharp optical signals expressions. As a conclusion, O2˙− detection could be logically realized.
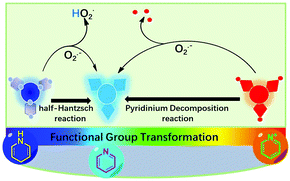 |
| Scheme 1 Schematic illustration of the probe and its working mechanism for the selective reaction with superoxide anion generated by an exogenous inducer or some endogenous processes. | |
Materials and methods
General procedures
Triphenylamine (TPA, 99%), tris(4-bromophenyl)amine (98%), phosphorus oxychloride (POCl3), N,N-dimethylformamide (DMF, AR), NH3·H2O (25%, AR), ethyl acetoacetate (98%), 4-bromotriphenylamine (99%), tris(4-iodophenyl)amine (98%), pyridine-4-boronic acid (99%), tetrakis(triphenylphosphine)palladium(0) (Pb(PPh3)4 98%), potassium carbonate (K2CO3, 99%), bis(4-bromophenyl)amine, (99%), iodobenzene (98%), benzyl bromide (98%), 4-bromomethylphenylboronic acid (98%), 1,10-phenanthroline (99%) were purchased from Aladdin Co. Nitrogen with a purity of 99.99% was provided from a commercial source. Other reagents, such as toluene, dichloromethane (DCM), methanol, ethanol, tetrahydrofuran (THF), ethyl acetate, and chloroform were purchased from Energy Chemical Company.
Instrumentation
1H NMR (600 MHz), 13C NMR (150 MHz) spectra were recorded on MERCURY spectrometer at 25 °C, and all NMR spectra were referenced to the solvent. UV-visible absorption spectra (UV) were recorded on a TU-1901 spectrometer. Fluorescence spectra were measured using a Fluoro Sens 2003 Fluorescence Spectrophotometer. Fluorescent microscopy images of HeLa cells were taken on DM5000B (Leica, Germany), co-staining fluorescent microscopy images of HeLa cells were taken on IX71 (Leica, Germany). All the samples were prepared according to the standard methods.
Reactive species
O2˙− was prepared by dissolving KO2 in DMSO solution. The concentration of O2˙− was determined by a TU-1901 spectrometer; NaOCl solution was diluted appropriately in 0.1 M NaOHaq. The concentration of OCl− was determined based on the molar extinction coefficient at 292 nm (350 M−1 cm−1); H2O2 was diluted appropriately in water. The concentration of H2O2 was determined based on the molar extinction coefficient at 240 nm (43.6 M−1 cm−1); hydroxyl radical (˙OH) was produced by the Fenton reaction (Fe2+/H2O2 = 1
:
10), and the concentration of ˙OH was equal to the Fe(II) concentration; 1O2 was generated by the addition of NaOCl and H2O2; peroxynitrite (ONOO−) was chemically provided by H2O2 and NaNO2. The concentration of ONOO− was estimated using an extinction coefficient of 1670 M−1 cm−1 (302 nm); ascorbic acid (Vc), glutathione (GSH) and cysteine (Cys) solutions were prepared from commercially obtained solids.
Theoretical calculation
All the calculations were implemented using the Gaussian 09 program package. The geometry of TPA-DHP-1, TPA-DHP-2 and TPA-DHP-3 were optimized using the three-parameter hybrid B3LYP density method, and a basis set of 6-31G*. The excited states of all the compounds were investigated via time-dependent density functional theory (DFT).
Cell fluorescence imaging
Human cervical cancer cells (HeLa) (1.0 × 105 per well) were cultured overnight and then stained in a Petri dish at 50% confluence with TPA-DHP-1 and TPA-PPA-3 in the DMSO solution (20 μM), an image was taken after 30 min incubation. Cells were first stained in the desired concentration by following the previous staining procedure. They were then incubated at 37 °C in 5% CO2 for 12 h and imaged at ambient temperature in the medium. Sensing of the generated O2˙− under oxidative stress was accomplished by adding Zymosan A and SOD (50 mg mL−1, 10 μL). To verify the production of O2˙−, cells were treated with Zymosan A and SOD for 10 min before adding TPA-DHP-1 and TPA-PPA-3.
Results and discussion
Synthesis of TPA-CHOs, TPA-DHPs, and TPA-PPAs and their structural characterization
Triphenylamine (TPA) is a highly electron-rich molecule; its characteristics originate from the sp3 hybrid nitrogen atom,17 which can donate electron clouds to the surrounding benzene rings.18 As a viable chemical structure design from the point of fluorescent materials, withdrawing substituents could facilitate the formation of desirable long wavelength emissive materials, even with small groups, such as –CHO,19 –C
C–COOCH3.20 As another method, TPA has been verified as an ACQ-active compound,21 and also has a very low fluorescence quantum yield. However, chemical modification can transform TPA into AIEgens and can also elevate the emission intensity through rational design.22 Therefore, in the present study,14a we synthesized three types of decorating group substituted TPA compounds (Scheme 2). Firstly, a formyl group was selected, this tiny withdrawing group could be introduced easily by the Vilsmeier–Haack reaction, and could also be easily oxidized with some soft oxidative species. Correspondingly, TPA-CHO-1, 2, and 3 were obtained in the gram scale by controlling the reaction conditions. Then, employing TPA-CHO-1, 2, and 3 as precursors, we synthesized dihydropyridine (DHP) substituted TPA compounds, TPA-DHP-1, 2, and 3 by the half-Hantzsch reaction (Fig. S5 ESI†). It was postulated that the half-Hantzsch reaction product (DHP) can be oxidized into the more stable pyridine (Py) compound by a specific ROS. It is likely that after dehydrogenating, the products of TPA-Py will be red-shifted and have intensified emission due to the formation of an electron D–A system. Finally, TPA-PPA-1, 2, and 3 were synthesized and designed for the ROS sensing. Three of them demonstrated long wavelength emission colours of more than 500 nm (λem = 546, 598, and 597 nm respectively) (Fig. S7 ESI†). Especially, the pyridinium, after decompounding by nucleophilic ROS, was able to output signal by the fluorescence channel, this possibility could be referred from several reports. As a result, TPA-CHO-(1–3), TPA-DHP-(1–3), and TPA-PPA-(1–3) were obtained successfully, their structures were confirmed by 1H and 13C NMR (Fig. S1–S6 ESI†).
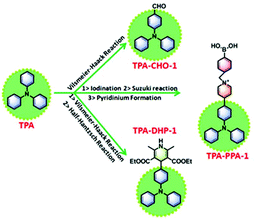 |
| Scheme 2 The general synthetic process to TPA-CHOs, TPA-DHPs and TPA-PPAs (taking TPA-CHO-1, TPA-DHP-1 and TPA-PPA-1 as the representative instance). | |
Fluorescence behaviour and the orbital energies of TPA-CHOs, TPA-DHPs, and TPA-PPAs
The chemical structures of all the compounds and the corresponding optical values, including the maximum emission peak (λem) and fluorescence quantum yield (Φ), as well as the powder images under 365 nm UV lamp irradiation are displayed in Fig. 1. Generally, after decorating the TPA core with the three different groups, the emission peaks are located from the blue region (λem = 448 nm) to the almost red region (λem = 597 nm), such properties give great opportunities for TPA-based materials with tunable emission colours. We studied the AIE property of all compounds in solvent mixtures according to the documented method (Table S1, Fig. S8 ESI†). Among all the synthesized compounds, TPA-CHO-1 has the highest fluorescence quantum yield of 31.36%, but the shortest emission wavelength of 448 nm. With a very sharp contrast, the mono-dihydropyridine substituted TPA-DHP-1 possessed the lowest fluorescence quantum yield of 0.55% over all of the fluorescent members. Potentially, this characteristic could be employed to design off-on type fluorescence sensors. It should be pointed out that the closest red emission compound is TPA-PPA-3, which could facilitate the bioassay in the most harmless manner.
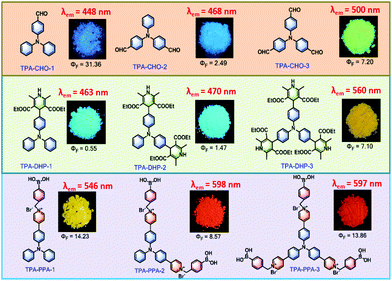 |
| Fig. 1 The chemical structures of all compounds and the corresponding optical values (λem and Φ), as well as the powder images under 365 nm UV lamp illumination. | |
Meanwhile, these synthesized compounds were optimized by density functional theory (DFT) calculations carried out using Gaussian 09 using the B3LYP modification with the 6-31G* basis set. The calculated energy levels of the highest occupied molecular orbital (HOMO) and the lowest unoccupied molecular orbital (LUMO) are illustrated in Fig. 2 and Fig. S10 ESI.† As it is well known, electron withdrawing substituents are able to lower the HOMO and LUMO levels through the formation of an electron donating and accepting (D–A) system.18 Accordingly, the more electron withdrawing groups the TPA core is substituted with, the more decreased the HOMO and LUMO levels are. Thus, TPA-CHO-3, TPA-DHP-3 and TPA-PPA-3 possessed the lowest orbital energy. Accordingly, three of them had the longest emission wavelength (500 nm, 560 nm, and 597 nm respectively) amongst the TPA-CHOs, TPA-DHPs and TPA-PPAs derivatives.
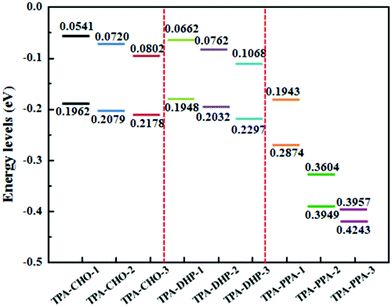 |
| Fig. 2 HOMO–LUMO energy levels of TPA-CHO-(1–3), TPA-DHP-(1–3), and TPA-PPA-(1–3), as estimated in Gaussian 09 using the B3LYP modification with the 6-31G* basis set. | |
Detection of O2˙− based on TPA-DHPs and TPA-PPAs
In order to inspect whether TPA-CHOs, TPA-DHPs and TPA-PPAs derivatives could specifically monitor O2˙−in vitro, we tested its ability to discriminate O2˙− and various bio analytes, such as competing ROS, RNS, and relevant biological substances. The comprehensive measurements exhibited that TPA-DHPs and TPA-PPAs demonstrate the most sensitive and selective sensing ability toward O2˙−. As shown in Fig. 3a distinct red shift and enhanced fluorescence intensity could be witnessed in the case of the TPA-DHPs. Especially, TPA-DHP-1 demonstrated an almost 90 nm wavelength shift and a nearly 14-fold intensity enhancement (Fig. 3a). In addition, the TPA-PPAs also displayed a distinct blue shift in the presence of O2˙−. Especially, TPA-PPA-3 gave rise to a 114 nm wavelength shift and a 1.8-fold intensity enhancement (Fig. 3f).
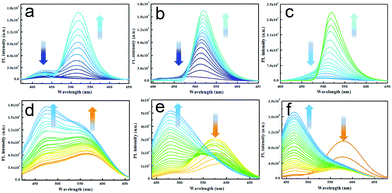 |
| Fig. 3 PL spectra of TPA-DHP-1–3 (a–c) (20 μM) and TPA-PPA-1–3 (d–f) (20 μM) incubated with increasing amounts of O2˙− from 0 to 100 μM. | |
As we expected, the TPA-DHPs readily carries out dehydrogenation in the presence of O2˙−, which then converses into the aromatized pyridines (Py) compound TPA-Pys. From the theoretical simulation results (Fig. 4), we can see that after Hantzsch reaction takes place, (taking TPA-DHP-1 as an example), the dihedral angle between the heterocycle with benzene ring increases from 101.9° to 179.8°. In other words, in the structure of TPA-Py-1, a more electron delocalized conjugation system formed. Additionally, an electron D–A system was also produced due to the more electron withdrawing pyridine segment. Therefore, the corresponding energy band gap between the LUMO and HOMO decreased from 0.13124 to 0.12576 eV. Correspondingly, the PL wavelength shifted from 411 nm to 513 nm, indicating a distinct emission change in colour. Moreover, the signal output with almost no overlap was not only beneficial for quick detection in vitro, but also in vivo.
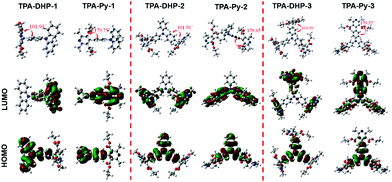 |
| Fig. 4 Optimized structures and HOMO–LUMO energy levels of TPA-DHP-1, TPA-DHP-2, TPA-DHP-3 and corresponding aromatization products as estimated by Gaussian 09 using the B3LYP modification with the 6-31G* basis set. | |
As shown in Fig. 5, taking TPA-DHP-1 and TPA-PPA-3 as examples, we determined the O2˙− monitoring specificity. We can see that TPA-DHP-1 displays negligible fluorescence changes in the presence of other ROS or relevant substances, including hydrogen peroxide (H2O2), singlet oxygen (1O2), nitric oxide (NO), TBHP, hypochlorite (OCl−), hydroxyl radical (˙OH), peroxynitrite (ONOO−), glutathione (GSH), and ascorbic acid (Vc). Nevertheless, after O2˙− was added to the above-mentioned solutions, a significant increase of fluorescence intensity was witnessed. Thus, these results strongly suggest that TPA-DHP-1 is highly selective for O2˙−.
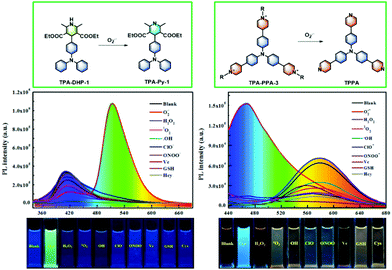 |
| Fig. 5 PL spectra of TPA-PPA-3 (20 μM) upon addition of O2˙−, H2O2, 1O2, ˙OH, ClO−, ONOO−, Vc, GSH, Hcy (100 μM) in an MeCN solution; the corresponding photographs taken under irradiation with 365 nm UV light. | |
In addition, as several reports disclosed, fluorescent pyridiniums can be utilized as O2˙− sensors; this is possibly due to the nucleophilicity of O2˙−.23 Therefore, we postulated that TPA-PPAs’ derivatives could be transformed into TPPA in the presence of O2˙−. We demonstrated that TPPA has the advantages of higher fluorescence quantum yield and long fluorescence lifetimes, which is beneficial for the sensing of O2˙−in vitro and in vivo. Initially, detailed analysis verified that the TPA-PPA-3 compound possesses the most sensitive and selective O2˙− sensing performance (Fig. 3f). From the selectivity experiments (Fig. 5), the most dominant sensing behaviour toward O2˙− could be seen in the case of TPA-PPA-3. Upon adding the O2˙− solutions, a quick response occurred accompanied by an apparent blue-shift from 580 nm to 466 nm. Meanwhile, the PL intensity of the solution was elevated nearly two-fold and the fluorescence off-on sensing process was confirmed to be very effective in this application.
Cell cytotoxicity assessment and cellular imaging applications
In order to test applications in cell imaging, the cytotoxicity of TPA-DHP-1 and TPA-PPA-3 were evaluated by 3-(4,5-dimethyl-2-thiazolyl)-2,5-diphenyltetrazolium bromide (MTT) assay. The results show that the HeLa cell viability is not significantly altered when 0, 10, 20, 30, 40, and 50 μg mL−1 probes are added into the culture medium after a long incubating time of 96 h. The HeLa cells were then stained by TPA-DHP-1 (20 μg mL−1) with different concentrations of Zymosan A. In this experiment, Zymosan A, which is an effective O2˙− producing agent, was used to enhance the O2˙− concentration. Fig. 6 illustrated that the HeLa cells treated with Zymosan A (50–400 μg mL−1) exhibit an elevated blue fluorescence in comparison to the control without Zymosan A. These images indicate significant elevations in O2˙− concentrations. Overall, the PL intensity increased almost 9-fold when the Zymosan A concentration was maintained at 400 μg mL−1. This result clearly demonstrated that TPA-DHP-1 has promising potential for monitoring the fluctuating concentrations of O2˙− in living cells.
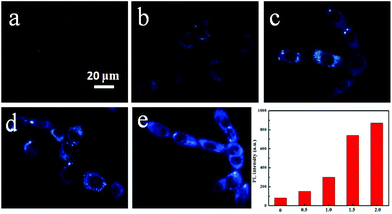 |
| Fig. 6 Fluorescence micrographs of HeLa cells incubated with Zymosan A (0, 50, 100, 200, 400 μg mL−1) and TPA-DHP-1 (20 μM) for 30 min. Inset: The corresponding fluorescence levels of the cells were quantified by Image J. | |
Next, confocal fluorescence microscopy images of the HeLa cells with different treatment methods were evaluated. The HeLa cells were initially incubated with TPA-DHP-1 (20 μg mL−1) only, and both channels expressed blue and green emission colours, (Fig. 7) which demonstrated the presence of TPA-DHP-1 and TPA-Py-1 in the HeLa cells. From this result, we can also postulate that the transformation of TPA-DHP-1 into TPA-Py-1 can be realized by O2˙− even at cellular level concentration. Evidently, TPA-DHP-1 could be used in O2˙− monitoring even at the cellular level concentration. Superoxide dismutase (SOD) was widely distributed as a natural killer of free oxygen radicals in the biological community; it accelerated the conversion of superoxide anion to hydrogen peroxide, which was further converted to water by catalase and glutathione peroxidase. Then, SOD was used to inhibit superoxide anion accumulation in living cells. As can be seen from Fig. 7, the green fluorescence intensity decreased compared to the control samples, this result also indicates the less efficient transformation of TPA-DHP-1 into TPA-Py-1. Evidently, this was due to the decreased O2˙− concentrations. Meanwhile, in the presence of the O2˙− producing agent, Zymosan A, the elevated superoxide anion level triggered an increase of green fluorescence intensity and diminished the blue fluorescence intensity. Most estimates suggested that the majority of intracellular ROS production is derived from the mitochondria.5 Therefore, we are convinced that the TPA-DHP-1 probe predominantly accumulates in the mitochondria.
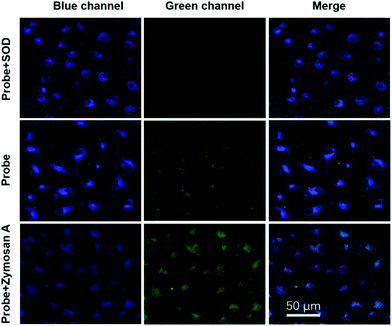 |
| Fig. 7 Confocal fluorescence microscopic images of HeLa cells with different treatments. Top: HeLa cells treated with the SOD (50 μg mL−1) for 10 min at 37 °C, then treated with the TPA-DHP-1 (20 μM) for 30 min. Middle: HeLa cells treated with the TPA-DHP-1 (20 μM) for 30 min. Bottom: HeLa cells treated with the Zymosan A (50 μg mL−1) for 10 min at 37 °C, then treated with the TPA-DHP-1 (20 μM) for 30 min. | |
In the next step, we employed TPA-PPA-3 as a probe to monitor O2˙− in HeLa cells (Fig. 8). As we previously reported, TPA-PPA-3 is a cell nucleus targeted probe by co-staining with DAPI.14a In the control samples, the highest emitted red fluorescence demonstrated the cell nucleus imaging. Particularly, in the presence of the inhibitors of SOD, the blue emission was suppressed and the red emission was enhanced. These changes evidenced the lower formation of TPPA from TPA-PPA-3, which is also due to the decreased O2˙− concentration. Interestingly, after incubating TPA-PPA-3 with Zymosan A, the O2˙− concentrations were elevated, and the different cell's locations could be seen from both optical channels. In the red one, the cell nucleus was stained clearly. Meanwhile, in the other channels, blue emissive mitochondria could be visualized. This result demonstrated the dynamic accumulation process and the chemical transformation of TPA-PPA-3 during the nucleus targeted staining. We postulated in this experiment that during the accumulation process of TPA-PPA-3 into the cell nucleus, a part of them transformed into the blue emissive TPPA in the presence of concentrated O2˙−. Then, the multiple positive charges are reduced, and the ability to penetrate into the nuclear membrane is lost. Finally, the mitochondria could be visualized with a blue emission colour. A part of the TPA-PPA-3 also successfully penetrated into the cell nucleus, which can be seen from the red channel.
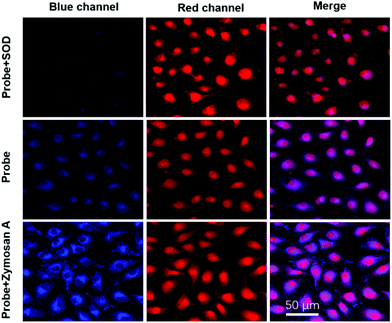 |
| Fig. 8 Confocal fluorescence microscopic images of the HeLa cells with different treatments. Top: HeLa cells treated with the SOD (50 μg mL−1) for 10 min at 37 °C, then treated with the TPA-PPA-3 (20 μM) for 30 min. Middle: HeLa cells treated with the TPA-PPA-3 (20 μM) for 30 min. Bottom: HeLa cells treated with the Zymosan A (50 μg mL−1) for 10 min at 37 °C, then treated with the TPA-PPA-3 (20 μM) for 30 min. | |
Conclusions
In summary, we have synthesized two types of AIE-active fluorescent probes (TPA-DHP-1,2,3 and TPA-PPA-1,2,3) by reconstructing and modifying a commonly used molecule of triphenylamine (TPA), and investigated their optical properties in the solid, solution, and aggregate states. Among all the investigated fluorescent materials, TPA-DHP-1 and TPA-PPA-3 demonstrated high selectivity towards the superoxide anion (O2˙−) over other common intracellular ROSs, reductants and amino acids. Meanwhile, both probes exhibited three remarkable features: (1) Due to the sharp wavelength shift, elevated fluorescence intensity, and irreversible chemical transformation, undesirable background fluorescence interference can be diminished clearly. (2) The rapid, sensitive and dynamic monitoring of O2˙− in living cells and in vitro from different optical channels could successfully be realized. (3) The dual-channel monitoring strategy not only provided real-time observation of the generation of O2˙− in mitochondria but could also be employed for imaging both the cell nucleus and mitochondria simultaneously. This study provides a simple, accurate, and feasible method for the detection of bioactive small molecules in living cells.
Conflicts of interest
There are no conflicts to declare.
Acknowledgements
This work is supported by the National Natural Science Foundation of China (No. 21764012). We thank the Key Laboratory of Eco-Environment-Related Polymer Materials (Northwest Normal University) and the Ministry of Education Scholars Innovation Team (IRT 1177) for financial support.
Notes and references
- T. Finke and N. J. Holbrook, Nature, 2000, 408, 239 CrossRef PubMed.
-
(a) M. Pasparakis and P. Vandenabeele, Nature, 2015, 517, 311 CrossRef CAS PubMed;
(b) P. J. Murray and T. A. Wynn, Nat. Rev. Immunol., 2011, 11, 723 CrossRef CAS PubMed;
(c) C. Szabó, H. Ischiropoulos and R. Radi, Nat. Rev. Drug Discovery, 2007, 6, 662 CrossRef PubMed.
-
(a) D. C. Wallace, Science, 1999, 283, 1482 CrossRef CAS PubMed;
(b) W. Wang, H. Fang, L. Groom, A. Cheng, W. Zhang, J. Liu and J. Yin, Cell, 2008, 134, 279 CrossRef CAS PubMed.
- M. H. Lee, J. S. Kim and J. L. Sessler, Chem. Soc. Rev., 2015, 44, 4185 RSC.
- W. Wang, H. Fang, L. Groom, A. Cheng, W. Zhang, J. Liu and J. Yin, Cell, 2008, 134, 279 CrossRef CAS PubMed.
-
(a) J. Zielonka, J. Joseph, A. Sikora, M. Hardy, O. Ouari, J. Vasquez-Vivar and B. Kalyanaraman, Chem. Rev., 2017, 117, 10043 CrossRef CAS PubMed;
(b) Y. L. Pak, S. J. Park, D. Wu, B. Cheon, H. M. Kim, J. Bouffard and J. Yoon, Angew. Chem., Int. Ed., 2018, 130, 1583 CrossRef.
-
(a) Z. Liu, X. Zhou, Y. Miao, Y. Hu, N. Kwon, X. Wu and J. Yoon, Angew. Chem., Int. Ed., 2017, 56, 5812 CrossRef CAS PubMed;
(b) X. Chen, K. A. Lee, E. M. Ma, K. M. Lee, Y. Y. Seo, H. K. Choi, W. J. Lee and J. Yoon, Chem. Commun., 2011, 47, 4373 RSC;
(c) H. Zhu, J. Fan, J. Wang, H. Mu and X. Peng, J. Am. Chem. Soc., 2014, 136, 12820 CrossRef CAS PubMed;
(d) G. Cheng, J. Fan, W. Sun, J. Cao, C. Hu and X. Peng, Chem. Commun., 2014, 50, 1018 RSC;
(e) D. Cheng, Y. Pan, L. Wang, Z. Zeng, L. Yuan, X. Zhang and Y. T. Chang, J. Am. Chem. Soc., 2016, 139, 285 CrossRef PubMed;
(f) L. Yuan, L. Wang, B. K. Agrawalla, S. J. Park, H. Zhu, B. Sivaraman and Y. T. Chang, J. Am. Chem. Soc., 2015, 137, 5930 CrossRef CAS PubMed;
(g) Y. Sheng, I. A. Abreu, D. E. Cabelli, M. J. Maroney, A. F. Miller, M. Teixeira and J. S. Valentine, Chem. Rev., 2014, 114, 3854 CrossRef CAS PubMed;
(h) X. Jiao, Y. Li, J. Niu, X. Xie, X. Wang and B. Tang, Anal. Chem., 2017, 90, 533 CrossRef PubMed.
- R. Zhang, J. Zhao, G. Han, Z. Liu, C. Liu, C. Zhang and M. Y. Han, J. Am. Chem. Soc., 2016, 138, 3769 CrossRef CAS PubMed.
-
(a) M. Yamato, T. Egashira and H. Utsumi, Free Radical Biol. Med., 2003, 35, 1619 CrossRef CAS;
(b) H. Yasui and H. Sakurai, Biochem. Biophys. Res. Commun., 2000, 269, 131 CrossRef CAS PubMed;
(c) Y. Koide, Y. Urano, S. Kenmoku, H. Kojima and T. Nagano, J. Am. Chem. Soc., 2007, 129, 10324 CrossRef CAS PubMed.
-
(a) P. Li, W. Zhang, K. Li, X. Liu, H. Xiao, W. Zhang and B. Tang, Anal. Chem., 2013, 85, 9877 CrossRef CAS PubMed;
(b) R. Zhang, J. Zhao, G. Han, Z. Liu, C. Liu, C. Zhang and M. Y. Han, J. Am. Chem. Soc., 2016, 138, 3769 CrossRef CAS PubMed;
(c) J. J. Hu, N. K. Wong, S. Ye, X. Chen, M. Y. Lu, A. Q. Zhao and D. Yang, J. Am. Chem. Soc., 2015, 137, 6837 CrossRef CAS PubMed.
- X. Gao, G. Feng, P. N. Manghnani, F. Hu, N. Jiang, J. Liu and B. Z. Tang, Chem. Commun., 2017, 53, 1653 RSC.
-
(a) W. Zhang, P. Li, F. Yang, X. Hu, C. Sun, W. Zhang and B. Tang, J. Am. Chem. Soc., 2013, 135, 14956 CrossRef CAS PubMed;
(b) P. Li, W. Zhang, K. Li, X. Liu, H. Xiao, W. Zhang and B. Tang, Anal. Chem., 2013, 85, 9877 CrossRef CAS PubMed;
(c) J. Niu, J. Fan, X. Wang, Y. Xiao, X. Xie, X. Jiao and B. Tang, Anal. Chem., 2017, 89, 7210 CrossRef CAS PubMed.
-
(a) L. L. Bronsart, C. Stokes and C. H. Contag, Mol. Imaging Biol., 2016, 18, 166 CrossRef CAS PubMed;
(b) F. Si, Y. Liu, K. Yan and W. Zhong, Chem. Commun., 2015, 51, 7931 RSC;
(c) Z. Zhang, J. Fan, Y. Zhao, Y. Kang, J. Du and X. Peng, ACS Sens., 2018, 3, 735 CrossRef CAS PubMed;
(d) J. J. Hu, N. K. Wong, S. Ye, X. Chen, M. Y. Lu, A. Q. Zhao and D. Yang, J. Am. Chem. Soc., 2015, 137, 6837 CrossRef CAS PubMed;
(e) Y. Zhou, J. Ding, T. Liang, E. S. Abdel-Halim, L. Jiang and J. J. Zhu, ACS Appl. Mater. Interfaces, 2016, 8, 6423 CrossRef CAS PubMed;
(f) R. Q. Li, Z. Q. Mao, L. Rong, N. Wu, Q. Lei, J. Y. Zhu and Z. H. Liu, Biosens. Bioelectron., 2017, 87, 73 CrossRef CAS PubMed;
(g) H. Xiao, X. Liu, C. Wu, Y. Wu, P. Li, X. Guo and B. Tang, Biosens. Bioelectron., 2017, 91, 449 CrossRef CAS PubMed.
-
(a) H. Ma, M. Yang, C. Zhang, Y. Ma, Y. Qin, Z. Lei and Y. Yang, J. Mater. Chem. B, 2017, 5, 8525 RSC;
(b) H. Ma, Z. Yang, H. Cao, L. Lei, L. Chang, Y. Ma and Z. Lei, J. Mater. Chem. B, 2017, 5, 655 RSC.
- J. Yang, X. Liu, H. Wang, H. Tan, X. Xie, X. Zhang and J. Hua, Analyst, 2018, 143, 1242 RSC.
-
(a) J. Chen and A. J. McNeil, J. Am. Chem. Soc., 2008, 130, 16496 CrossRef CAS PubMed;
(b) A. J. Jimenez, M. Fagnoni, M. Mella and A. Albini, J. Org. Chem., 2009, 74, 6615 CrossRef CAS PubMed.
-
(a) T. Geng, Z. Zhu, W. Zhang and Y. Wang, J. Mater. Chem. A, 2017, 5, 7612 RSC;
(b) A. Labrunie, J. Gorenflot, M. Babics, O. Aleveque, S. Dabos-Seignon, A. H. Balawi and P. M. Beaujuge, Chem. Mater., 2018, 30, 3474 CrossRef CAS.
- M. Jiang, X. Gu, R. T. Kwok, Y. Li, H. H. Sung, X. Zheng and K. S. Wong, Adv. Funct. Mater., 2018, 28, 1704589 CrossRef.
- P. S. Hariharan, V. K. Prasad, S. Nandi, A. Anoop, D. Moon and S. P. Anthony, Cryst. Growth Des., 2016, 17, 146 CrossRef.
- Y. Ma, H. Ma, Z. Yang, J. Ma, Y. Su, W. Li and Z. Lei, Langmuir, 2015, 31, 4916 CrossRef CAS PubMed.
- Y. Hong, J. W. Lam and B. Z. Tang, Chem. Soc. Rev., 2011, 40, 5361 RSC.
-
(a) J. Mei, N. L. Leung, R. T. Kwok, J. W. Lam and B. Z. Tang, Chem. Rev., 2015, 115, 11718 CrossRef CAS PubMed;
(b) R. Hu, N. L. Leung and B. Z. Tang, Chem. Soc. Rev., 2014, 43, 4494 RSC.
-
(a) Z. H. Guo, Z. X. Jin, J. Y. Wang and J. Pei, Chem. Commun., 2014, 50, 6088 RSC;
(b) J. Wu, H. Wang, S. Xu and W. Xu, J. Phys. Chem. A, 2015, 119, 1303 CrossRef CAS PubMed.
Footnote |
† Electronic supplementary information (ESI) available. See DOI: 10.1039/c8an01811a |
|
This journal is © The Royal Society of Chemistry 2019 |