DOI:
10.1039/C4RA16903D
(Paper)
RSC Adv., 2015,
5, 14095-14102
Discovery of novel 1,3,5-triazine-thiazolidine-2,4-diones as dipeptidyl peptidase-4 inhibitors with antibacterial activity targeting the S1 pocket for the treatment of type 2 diabetes
Received
23rd December 2014
, Accepted 16th January 2015
First published on 16th January 2015
Abstract
A novel series of 1,3,5-triazine-thiazolidine-2,4-diones was synthesized and characterized by a number of analytical and spectroscopic techniques. The molecules were screened for the in vitro inhibition of dipeptidyl peptidase-4 and compound 7a showed the most prominent inhibition with IC50 = 6.25 μM. The other compounds showed considerable inhibition (IC50 = 12.11–49.21 μM). Docking studies indicated that the lipophilic thiazolidine-2,4-dione fragment of ligand 7a was oriented towards the tight lipophilic cavity of the S1 pocket of the active site formed by residues such as Tyr631, Val656, Trp659, Tyr662, Tyr666 and Val711 via the formation of H-bonds with Tyr547. One of the amines present on the wings of the triazine formed a hydrogen bond with Glu205, a vital residue for the N-terminal recognition site with an efficient CDOCKER interaction energy. In a bacterial inhibition study, the entire set of compounds showed excellent activity and, in some instances, were found to be equipotent to the cefixime used as a standard.
Introduction
Diabetes mellitus is classified into type 1 (previously known as insulin-dependent) diabetes and type 2 (formerly called non-insulin-dependent) diabetes. Diabetes is a group of metabolic disorders in which either the pancreas does not make adequate insulin or the body cannot effectively use the insulin it produces, or both.1 The burden of this disease is increasing globally, especially in developing nations, which contribute more than 80% of the deaths due to diabetes.2 As an estimate, 1.5 million deaths in 2012 were caused by diabetes and, by 2030, it is expected to be the seventh leading cause of morbidity and death worldwide.3
In patients with type 2 diabetes, in addition to classical insulin-based treatment, inhibition of the serine protease dipeptidyl peptidase 4 (DPP-4) has been proved to be an effective treatment for improving glycemic control.4 DPP-4 is also known as CD26 (cluster of differentiation 26 or T-cell activation antigen CD26) or adenosine deaminase complexing protein 2, which selectively cleaves the first two amino acids of glucose-dependent insulinotropic polypeptide (GIP) and glucagon-like peptide (GLP-1), thereby making them inactive. Therefore, the inhibition of DPP-4 by a chemotherapeutic agent may lead to an increase in the levels of circulating endogenous GLP-1 by prolonging its half-life and consequently enhancing the beneficial effects of GLP-1 in glucose-dependent insulin secretion and β-cell restoration. The inhibition of DPP-4 results in higher circulating concentrations of endogenous GLP-1 and a subsequent decrease in plasma glucose by enhancing β cell glucose-stimulated insulin release and promoting insulin gene expression and biosynthesis (Fig. 1).5
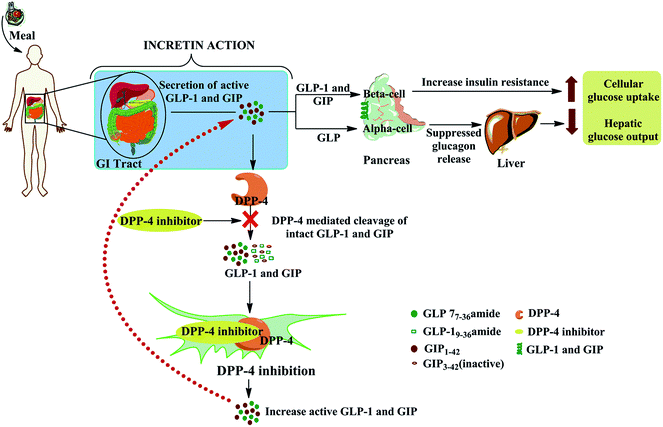 |
| Fig. 1 Classical role of DPP-4 and its inhibition. | |
Imeglimin [(6R)-(+)-4-dimethylamino-2-imino-6-methyl-1,2,5,6-tetrahydro-1,3,5-triazine hydrochloride] is the first of a new tetrahydrotriazine-containing class of oral drugs used to treat diabetes: the glimins. It is currently in phase 2b clinical development.6 In preclinical studies, imeglimin has been shown to reduce excessive hepatic glucose production, increase glucose uptake in skeletal muscle and improve insulin secretion in response to glucose by acting on the liver, muscles and pancreas, three key organs involved in the pathophysiology of type 2 diabetes. Imeglimin offers a unique mechanism of action that targets the mitochondria and is compatible with drugs that counter insulin resistance or enhance insulin secretion and β cell protection.7
Thiazolidin-2,4-diones, a class of oral insulin-sensitizing agents that improve insulin resistance, are agonists of proxisome proliferator activated receptor-γ (PPAR-γ). These molecules amplify PPAR-γ expression in the adipose tissue, increasing adipocytes and subcutaneous adipose tissue mass. Increased PPAR-γ expression in adipose tissue results in increased fatty acid uptake and storage by increasing the transcription of fatty acid transport protein-1 and acyl-coenzyme A synthetase. Decreased circulating free fatty acid levels protect β cells, the liver and skeletal muscle from their toxic effects, thus improving insulin sensitivity.8 Of the thiazolidin-2,4-diones, ciglitazone, pioglitazone, rosiglitazone, troglitazone (approved in 1997) and englitazone have been examined clinically.9
According to the American Diabetes Association, combinations of oral drugs rather than monotherapy can be used to attack the pathophysiology of diabetes at multiple points in patients in whom insulin secretion is still moderate.10 Combination therapy includes treatment with two or more drugs with different complementary mechanisms of action – for example, the combination of a thiazolidinedione and a biguanide improves insulin sensitivity and lowers blood glucose through complementary pathways and therefore produces an additive effect. Nevertheless, this strategy sometimes fails because of the incomplete and altered pharmacokinetics of the combined drugs that affect their bioavailibity.11 Molecular hybridization, an innovative approach in which two diverse pharmacophoric groups are joined, is currently attracting attention from medicinal chemists. These drugs can be more powerful than either of their precursors as a result of dual drug targeting at more than one site and privileged activity compared with the individual agent.12
Considering the clinical implications of DPP-4 inhibitors, the advantages of molecular hybridization and the potential of 1,3,5-triazine and thiazolidine-2,4-diones to treat diabetes, we report here the design, synthesis and biological evaluation of a series of novel hybrid 1,3,5-triazine-thiazolidine-2,4-diones as DPP-4 inhibitors with antibacterial activity.
Results and discussion
Chemistry
The novel hybrid conjugates were synthesized via a multi-step reaction as outlined in Scheme 1. The synthesis of thiazolidin-2,4-dione (3) was achieved by reacting chloroacetic acid (1) and thiourea (2) in the presence of water via the formation of the thiouronium salt, which later cyclizes in the presence of an acid to give the corresponding product 3 in good yield. Reaction of 2,4,6-trichloro-1,3,5-triazine (4) with the amines 5a–k in the presence of an activating base yielded the mono-chloro-di-substituted-1,3,5-triazine 6a–k on stirring for the desired time (step 2). The combination of previously obtained products from two different steps (3 and 6a–k) leads to the generation of the novel target hybrid conjugates 7a–k via a nucleophilic reaction in the presence of K2CO3 as a base.
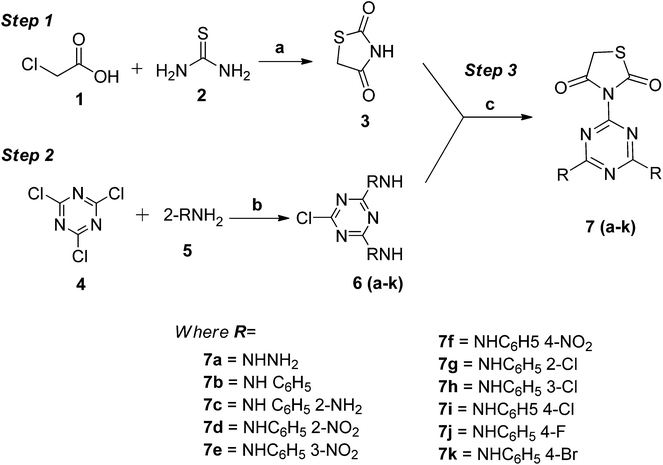 |
| Scheme 1 Synthesis of novel 1,3,5-triazine-thiazolidine-2,4-diones: reagents and condition: (a) concentrated H2SO4, water, reflux; (b) NaOH, stirring, 40–45 °C; and (c) K2CO3, reflux, 120–135 °C. | |
Inhibitory activity against DPP-4 in vitro
The synthesized 1,3,5-triazine-thiazolidine-2,4-diones derivatives 7a–k were evaluated in vitro for DPP-4 enzyme inhibitory activity and the results obtained are reported as the micromolar inhibitory concentration, IC50 (μM) along with the standard (P32/98) in Table 1. In a comparison test, compound 7a containing hydrazine as a substituent was identified as a very potent analogue in this series (IC50 = 6.25 μM), whereas a two-fold decrease in activity was reported on the introduction of the bulky aromatic substitution compound 7b (IC50 = 12.11 μM). A marginal progress in activity was indicated by compound 7c having o-NH2 as a substituent on the phenyl moiety. The introduction of NO2 (compound 7d) in place of NH2 rendered the compound less potent and a greater decrease in activity was seen on the insertion of Cl (compound 7g). The optimization strategy was then focused on studying the optimum placement of these functional groups. Compounds 7e and 7f with m- and p-NO2 led to a further decrease in activity. The same pattern of activity was not observed for compounds 7h and 7i containing m- and p-Cl, which showed a comparatively weak inhibition. A significant decrease in activity was seen for compounds 7j and 7k with a p-F and a p-Br substituent, respectively (IC50 = 38.37 μM and 49.21 μM) (Table 2).
Table 1 Inhibitory effect of novel 1,3,5-triazine-thiazolidine-2,4-dione derivatives on DPP-4
Compound |
IC50a (μM) |
IC50 represents inhibitory concentration determined by non-linear regression analysis using GRAPHPAD PRISM software. Values are expressed as the mean of three independent experiments. |
7a |
6.35 |
7b |
12.11 |
7c |
10.76 |
7d |
14.64 |
7e |
15.38 |
7f |
17.85 |
7g |
27.32 |
7h |
23.25 |
7i |
29.53 |
7j |
38.37 |
7k |
49.21 |
P32/98 (standard) |
2.5 |
Table 2 Docking interaction and scoring of compounds 7a (most active) and 7k (least active)
Compound |
CDOCKER interaction energy |
CDOCKER energy |
H-bonding residues |
π-cation interaction |
7a |
31.90 |
44.31 |
Glu205, Tyr547 |
Arg125 |
7k |
44.27 |
43.51 |
Arg358, Arg669, Glu205 |
Arg125 |
A structure–activity relationship study suggested that the presence of a substituent had a substantial influence on the inhibitory activity. It was clearly seen that the presence of a bulky substituent decreased the activity and a further decrease was observed on the introduction of the substituent. The pattern of the structure–activity relationship is clearly shown in Fig. 2.
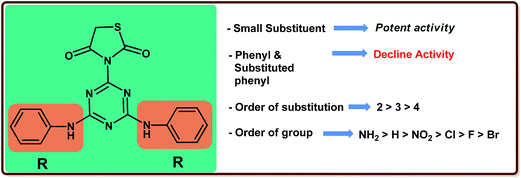 |
| Fig. 2 Structure–activity relationships of target analogues as DPP-4 inhibitors. | |
Molecular docking studies
Dipeptidyl peptidase-4 enzyme consists of two domains: a eight-stranded β-propeller domain at the N-terminus and an α/β-hydrolase domain at the C-terminus. The X-ray crystallography study of various inhibitors in complex with DPP-4 showed that the binding site of DPP-4 mainly consists of three parts: an S1 pocket, the N-terminal recognition region and an S2 pocket.13 The S1 hydrophobic pocket consists of residues of Tyr631, Val656, Trp659, Tyr662, Tyr666 and Val711 adjacent to the catalytic triad of the active site. The active site is situated in the hydrolase domain and consists of the catalytic triad residues Ser630, Asp708 and His740. The N-terminal recognition region is formed by Glu205, Glu206 and Tyr662, whereas the hydrophobic S2 pocket, which is larger than the S1 pocket, is surrounded by the residues Phe357, Arg358, Ser209 and Tyr666.
Based on these in vitro results, it was worthwhile performing a molecular docking study of the most active compound 7a with the DPP-4 inhibitor to explore the key molecular interactions (2FJP.pdb). Fig. 3 shows that one of the amines present on the wings of the triazine forms a hydrogen bond with Glu205, a vital residue for the N-terminal recognition site. In this new orientation, the amine present on the other wing protrudes towards the Ser630 and Tyr631 of the S1 pocket by creating a hydrogen bond with the neighboring Tyr547. The lipophilic thiazolidine-2,4-dione fragment of the ligand was oriented towards the S1 pocket of the active site formed by residues such as Tyr631, Val656, Trp659, Tyr662, Tyr666 and Val711. In addition, the creation of one π-cation interaction was also reported between the 1,3,5-triazine and the Arg105 of the S1 pouch. Scoring parameters indicate that compound 7a binds more firmly to the S1 pocket of the DPP-4 by the virtue of the efficient CDOCKER interaction energy and CDOCKER energy. As a consequence of this tight binding, the residence time of 7a increases in DPP-4, which may transform into a higher inhibitory activity in vitro. The engulfment of compound 7a in the S1 pocket of DPP-4 was found in accordance with earlier studies which reported that small fragments target the tight lipophilic cavity of the S1 pocket.14
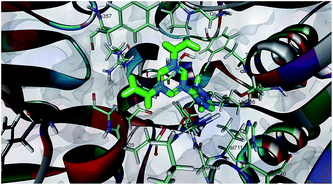 |
| Fig. 3 Docked complex of compound 7a in the binding pocket of DPP-4. | |
The docking of the least active compound 7k in the DPP-4 binding site revealed the reason for its non-activity. The thiazolidine-2,4-dione fragment of ligand 7k was positioned towards the S2 pocket of the binding site by making H-bonds with Arg358 and Arg669 as a result of its extensive hydrophobic character (Fig. 4). It also formed H-bonds with the Glu205 of the N-terminal recognition site at different CDOCKER energies.
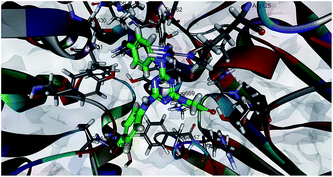 |
| Fig. 4 Docked complex of compound 7k in the binding pocket of DPP-4. | |
The docking study suggested that an optimum balance between the hydrophobic–hydrophilic character favours an increase in bioactivity (7a), whereas an extensive hydrophobic character, as seen in compound 7k, leads to a loss of activity. Molecules small enough to fit into the S1 pocket have better bioactivity than larger molecules.
Antibacterial activity
The immune dysfunction seen in patients with diabetes as a result of the hyperglycemic environment leads to more frequent and/or serious infectious diseases, which potentially increases their morbidity and mortality.15 However, the efficacy of the antibiotic drugs used to treat these infections has been seriously compromised by the development of resistance and the discovery of newer agents is now required.16 In a continuation of our research towards the discovery of novel antibacterial agents from 1,3,5-triazine derivatives,17 these constitutive derivatives were tested against a panel of diverse microorganisms.
The newly prepared compounds were screened for to determine their minimum inhibitory concentration (MIC) against selected Gram-positive [Bacillus subtilis (NCIM-2063), B. cereus (NCIM-2156), Staphylococcus aureus (NCIM-2079)] and Gram-negative [Escherichia coli (NCIM-2065), Proteus vulgaris (NCIM-2027) and Pseudomonas aeruginosa (NCIM-2036)] organisms using the broth microdilution (in tubes) method of the Clinical and Laboratory Standards Institute with minor modifications using cefixime as a standard (Table 3).
Table 3 Antibacterial activity of compound 7a–k
Compound |
Minimum inhibitory concentration (μg mL−1) |
P. vulgaris |
E. coli |
P. aeruginosa |
B. subtilis |
S. aureus |
B. cerus |
7a |
31.25 |
62.5 |
62.5 |
125 |
62.5 |
62.5 |
7b |
15.62 |
15.62 |
15.62 |
31.25 |
62.5 |
62.5 |
7c |
125 |
125 |
3.91 |
15.62 |
3.91 |
125 |
7d |
32.25 |
15.62 |
15.62 |
7.81 |
7.81 |
15.62 |
7e |
15.62 |
15.62 |
3.91 |
3.91 |
31.25 |
3.91 |
7f |
62.5 |
62.5 |
15.62 |
125 |
15.62 |
7.81 |
7g |
7.81 |
62.5 |
125 |
7.81 |
125 |
31.25 |
7h |
3.91 |
62.5 |
15.62 |
62.5 |
7.81 |
62.5 |
7i |
15.62 |
62.5 |
31.25 |
31.25 |
31.25 |
125 |
7j |
125 |
125 |
62.5 |
31.25 |
31.25 |
7.81 |
7k |
62.5 |
31.25 |
31.25 |
62.5 |
31.25 |
31.25 |
Cefixime (standard) |
7.81 |
15.62 |
3.91 |
31.25 |
7.81 |
31.25 |
In a comparison test, the entire set of hybrid derivatives showed moderate to excellent activities against the tested Gram-positive and Gram-negative microorganisms compared with a standard. Compound 7a showed moderate to no activity against the tested organisms. Compound 7b showed an equipotent activity to the standard against E. coli and was found to be moderately active against the other strains. Compound 7c showed improved activity compared with the standard against E. coli, B. subtilis and S. aureus, with moderate activity against the rest of the strains. The presence of NO2 (7d) renders the compounds more active against all the strains except P. aeruginosa and P. vulgaris, against which it displayed moderate activity compared with the previous analogue. The isomeric replacement of NO2, as seen in compounds 7e and 7f, does not show any significant change in the activity profile except in the case of B. subtilis (for 7f), where it showed almost no activity. For P. vulgaris, compounds 7g–i showed considerable activity, whereas mild to no activity was reported by compounds 7j and 7k. Moreover, compounds 7g–j showed moderate activities against E. coli except for 7k. In the case of P. aeruginosa, the m-chloro-substituted analogue (7h) showed more prominent inhibition than the isomeric counterparts 7g and 7i. Compounds 7j and 7k showed mild and no activity against P. aeruginosa, respectively. The halogen-substituted compounds (7g–k) showed mild to moderate activity against all the tested Gram-positive microorganisms.
Experimental
The melting points of the synthesized compounds were determined in an open capillary tube Hicon melting point apparatus and are uncorrected. Thin-layer chromatography on silica gel-G coated plates was used to detect the completion of the reaction. The diverse mobile phase was selected in different proportions according to the assumed polarity of the products. The spots were visualized by exposure to iodine vapour. IR spectra were recorded in KBr on a Biored FTs spectrophotometer and the reported wave numbers are given in cm−1. 1H-NMR spectra were recorded in DMSO on a Bruker Model D9RX-300 MHz spectrometer. The chemical shifts were reported as δ (ppm) relative to TMS as an internal standard. Mass spectra were obtained on a Waters Q-TOF MICROMA SS LC mass spectrometer.
General procedure
Step 1: The synthesis of thiazolidinone (3) was performed according to a previously published procedure.18
Step 2: The synthesis of the di-substituted 1,3,5-triazines 6(a–k) was performed in accordance with an earlier reported procedure.17a
Step 3: 6-Chloro-N2,N4-bis(substituted phenyl)-1,3,5-triazine-2,4-diamine 6(a–k) (0.1 mol) was added into 50 mL of dioxane at a temperature of 40–45 °C. A solution of thiazolidine-2,4-dione (3) (0.1 mol) in 35 mL of dioxane was added slowly to this solution and stirred for 2 h, followed by the dropwise addition of K2CO3 (0.1 mol). The reaction mixture was then refluxed at 120–135 °C for 3–6 h. The completion of the reaction was monitored by thin-layer chromatography using benzene and ethyl acetate (9
:
1) as the mobile phase. The reaction mixture was washed thoroughly with water, filtered and further purified by column chromatography to give the pure products 7(a–k).
3-(4,6-Dihydrazinyl-1,3,5-triazin-2-yl)thiazolidine-2,5-dione, 7a. Dark brown powder; MP > 300 °C. FTIR (νmax; cm−1, in KBr): 3259.03 (–NH– stretching), 1613.67 (–C
O stretching), 1599.12–1445.77 (–NH2 bending), 1385.41–1222.59 (C–N stretching), 687.11 (C–S stretching). 1H-NMR (300 MHz, CDCl3-d6, TMS) δ ppm: 2.52–2.54 (d, 4H, 2 × –NH2 – hydrazine), 3.32–3.34 (d, 4H, 2 × –NH– hydrazine), 3.59 (s, 2H, –CH2– thiazolidine). 13C-NMR (400 MHz, CDCl3) δ ppm: 163.84 (C
O, thiazolidine), 138.27 (C, triazine), 128.24 (C, triazine), 123.22 (C
O, thiazolidine), 78.34 (–CH2–, thiazolidine). Mass spectra (TOF MS ES+, m/z): calculated 256.05; observed 257.20 (M + 1).
3-[4,6-Bis(phenylamino)-1,3,5-triazin-2-yl]thiazolidine-2,5-dione, 7b. White powder; MP > 300 °C. FTIR (νmax; cm−1, in KBr): 3366.40 (–NH– stretching), 1605.32 (–C
O stretching), 1573.76–1426.76 (–NH2 bending), 1338.47–1238.92 (C–N stretching), 667.75 (C–S stretching). 1H-NMR (300 MHz, CDCl3-d6, TMS) δ ppm: 3.58 (s, 2H, 2 × –NH–Ar), 3.88 (s, 2H, –CH2– thiazolidine), 6.59–6.61 (d, 4H, J = 6 Hz, 2 × Ar–H), 6.96–7.02 (m, 2H, 2 × Ar–H), 7.49–7.58 (m, 4H, 2 × Ar–H). 13C-NMR (400 MHz, CDCl3) δ ppm: 163.99 (C
O, thiazolidine), 147.91 (C, triazine), 140.81 (C, triazine), 135.37 (C
O, thiazolidine), 129.48 (2 × –NH–C, Ar), 126.17 (2 × C, Ar), 119.80 (4 × C, Ar), 116.47 (4 × C, Ar), 109.67 (2 × C, Ar), 66.33 (–CH2–, thiazolidine). Mass spectra (TOF MS, m/z): calculated 378.09; observed 139.1 (100%), 85.0 (35.86%), 379.3 (20.03%, M + 1), 93.1 (17.69%).
3-[4,6-Bis(2-aminophenylamino)-1,3,5-triazin-2-yl]thiazolidine-2,5-dione, 7c. Dark brown powder; MP > 300 °C. FTIR (νmax; cm−1, in KBr): 3230.15 (–NH– stretching), 1611.78 (–C
O stretching), 1566.21–1434.62 (–NH2 bending), 1348.65–1249.96 (C–N stretching), 684.20 (C–S stretching). 1H-NMR (300 MHz, CDCl3-d6, TMS) δ ppm: 3.36 (s, 4H, 4 × –NH2), 3.56 (s, 2H, –CH2– thiazolidine), 6.79–6.81 (m, 4H, 2 × Ar–H), 7.01–7.07 (m, 4H, 2 × Ar–H). 13C-NMR (400 MHz, CDCl3) δ ppm: 163.65 (C
O, thiazolidine), 162.76 (C, triazine), 133.42 (2 × C, triazine), 126.67 (C
O, thiazolidine), 126.03 (2 × –NH–C, Ar), 124.43 (2 × NH2–C, Ar), 117.29 (4 × C, Ar), 112.15 (4 × C, Ar), 78.50 (2 × C, Ar), 65.38 (–CH2–, thiazolidine). Mass spectra (TOF MS, m/z): calculated 408.11; observed 133.1 (100%), 189.2 (69.81%), 409.20 (09.17%, M + 1), 134.1 (9.08%).
3-[4,6-Bis(2-nitrophenylamino)-1,3,5-triazin-2-yl]thiazolidine-2,5-dione, 7d. Yellow powder; MP 121–123 °C. FTIR (νmax; cm−1, in KBr): 3271.48 (–NH– stretching), 1616.55 (–C
O stretching), 1566.12–1422.45 (–NH2 bending), 1398.80 (N
O stretching, Ar–NO2), 1386.64–1115.86 (C–N stretching), 819.61 (C–N stretching, Ar–NO2), 617.06 (C–S stretching). 1H-NMR (300 MHz, CDCl3-d6, TMS) δ ppm: 3.58 (s, 2H, 2 × –NH–Ar), 3.80 (s, 2H, –CH2– thiazolidine), 7.36–7.38 (d, 2H, J = 6 Hz, 2 × Ar–H), 7.40–7.43 (t, 2H, J = 9 Hz, 2 × Ar–H), 7.47–7.49 (t, 2H, J = 6 Hz, 2 × Ar–H), 7.59–7.61 (d, 2H, J = 6 Hz, 2 × Ar–H). 13C-NMR (400 MHz, CDCl3) δ ppm: 163.75 (C
O, thiazolidine), 162.16 (C, triazine), 131.00 (C, triazine), 122.67 (C
O, thiazolidine), 120.03 (2 × NO2–C, Ar), 113.15 (2 × –NH–C, Ar), 109.24 (2 × C, Ar), 107.29 (2 × C, Ar), 84.15 (2 × C, Ar), 78.50 (2 × C, Ar), 66.33 (–CH2–, thiazolidine). Mass spectra (TOF MS, m/z): calculated 468.06; observed 454.1 (100%), 469.10 (66.89%, M + 1), 510.1 (64.90%), 113.0 (24.88%).
3-[4,6-Bis(3-nitrophenylamino)-1,3,5-triazin-2-yl]thiazolidine-2,5-dione, 7e. Yellow powder; MP > 300 °C. FTIR (νmax; cm−1, in KBr): 3198.08 (–NH– stretching), 1646.10 (–C
O stretching), 1558.62–1456.79 (–NH2 bending), 1329.40 (N
O stretching, Ar–NO2), 1287.17–1195.20 (C–N stretching), 782.96 (C–N stretching, Ar–NO2), 615.40 (C–S stretching). 1H-NMR (300 MHz, CDCl3-d6, TMS) δ ppm: 3.34 (s, 2H, 2 × –NH–Ar), 3.36 (s, 2H, –CH2– thiazolidine), 6.82–6.83 (d, 2H, J = 3 Hz, 2 × Ar–H), 7.23–7.25 (t, 2H, J = 6 Hz, 2 × Ar–H), 7.39 (s, 2H, 2 × Ar–H), 8.25–8.27 (d, 2H, J = 6 Hz, 2 × Ar–H). 13C-NMR (400 MHz, CDCl3) δ ppm: 142.43 (C
O, thiazolidine), 134.35 (C, triazine), 131.52 (C, triazine), 123.25 (C
O, thiazolidine), 119.00 (2 × NO2–C, Ar), 112.25 (2 × –NH–C, Ar), 110.14 (2 × C, Ar), 108.25 (2 × C, Ar), 82.12 (2 × C, Ar), 78.40 (2 × C, Ar), 42.36 (–CH2–, thiazolidine). Mass spectra (TOF MS, m/z): calculated 468.06; observed 175.0 (100%), 469.10 (40.85%, M + 1), 259.0 (15.55%), 113.0 (14.55%).
3-[4,6-Bis(4-nitrophenylamino)-1,3,5-triazin-2-yl]thiazolidine-2,5-dione, 7f. Yellow powder; MP 221–223 °C. FTIR (νmax; cm−1, in KBr): 3341.72 (–NH– stretching), 1622.48 (–C
O stretching), 1592.65–1426.44 (–NH2 bending), 1343.29 (N
O stretching, Ar–NO2), 1281.37–1008.75 (C–N stretching), 870.58 (C–N stretching, Ar–NO2), 729.41 (C–S stretching). 1H-NMR (300 MHz, CDCl3-d6, TMS) δ ppm: 3.33 (s, 2H, 2 × –NH–Ar), 3.36 (s, 2H, –CH2– thiazolidine), 6.57–6.61 (t, 4H, J = 12 Hz, 2 × Ar–H), 6.99–7.02 (d, 4H, J = 12 Hz, 2 × Ar-H). 13C-NMR (400 MHz, CDCl3) δ ppm: 146.13 (C
O, thiazolidine), 135.35 (C, triazine), 130.32 (C, triazine), 125.24 (C
O, thiazolidine), 119.04 (2 × –NH–C, Ar), 115.23 (2 × NO2–C, Ar), 79.12 (2 × C, Ar), 78.46 (2 × C, Ar), 40.16 (–CH2–, thiazolidine). Mass spectra (TOF MS, m/z): calculated 468.06; observed 121.1 (100%), 469.10 (91.11%, M + 1), 85.0 (45.54%), 91.1 (29.93%).
3-[4,6-Bis(2-chlorophenylamino)-1,3,5-triazin-2-yl]thiazolidine-2,5-dione, 7g. White powder; MP 286–288 °C. FTIR (νmax; cm−1, in KBr): 328.34 (–NH– stretching), 1622.12 (–C
O stretching), 1586.58–1416.81 (–NH2 bending), 1298.13–1180.52 (C–N stretching), 1007.74 (C–Cl stretching), 685.97 (C–S stretching). 1H-NMR (300 MHz, CDCl3-d6, TMS) δ ppm: 3.39 (s, 2H, 2 × –NH–Ar), 3.90 (s, 2H, –CH2– thiazolidine), 6.58–6.62 (m, 2H, 2 × Ar–H), 6.99–7.02 (d, 2H, J = 12 Hz, 2 × Ar–H), 7.33–7.41 (m, 2H, 2 × Ar–H), 7.91–7.95 (m, 2H, 2 × Ar–H). 13C-NMR (400 MHz, CDCl3) δ ppm: 164.95 (C
O, thiazolidine), 163.73 (C, triazine), 155.68 (C, triazine), 146.13 (C
O, thiazolidine), 135.65 (2 × –NH–C, Ar), 130.20 (2 × C, Ar), 126.35 (2 × Cl–C, Ar), 125.32 (2 × C, Ar), 124.67 (2 × C, Ar), 119.33 (2 × C, Ar), 112.32 (–CH2–, thiazolidine). Mass spectra (TOF MS, m/z): calculated 446.01; observed 386.2 (100%), 368.2 (90.99%), 447.20 (32.45%, M + 1), 258.2 (25.36%).
3-(4,6-Bis(3-chlorophenylamino)-1,3,5-triazin-2-yl)thiazolidine-2,5-dione, 7h. White crystal; MP 245–247 °C. FTIR (νmax; cm−1, in KBr): 3374.84 (–NH– stretching), 1613.45 (–C
O stretching), 1569.83–1486.44 (–NH2 bending), 1423.52–1178.53 (C–N stretching), 1090.62 (C–Cl stretching), 795.46 (C–S stretching). 1H-NMR (300 MHz, CDCl3-d6, TMS) δ ppm: 3.88 (s, 2H, –CH2– thiazolidine), 3.77 (s, 2H, 2 × –NH–Ar), 7.19 (s, 2H, 2 × Ar–H), 7.21–7.22 (d, 2H, J = 3 Hz, 2 × Ar–H), 7.24–7.25 (d, 2H, J = 3 Hz, 2 × Ar–H), 7.27–7.29 (d, 2H, J = 6 Hz, 2 × Ar–H). 13C-NMR (400 MHz, CDCl3) δ ppm: 163.73 (C
O, thiazolidine), 162.16 (C, triazine), 128.07 (C, triazine), 127.90 (C
O, thiazolidine), 122.16 (2 × –NH–C, Ar), 78.70 (2 × C, Ar), 78.57 (2 × C, Ar), 78.37 (2 × C, Ar), 78.04 (2 × C, Ar), 40.21–39.16 (2 × Cl–C, Ar), 38.85 (–CH2–, thiazolidine). Mass spectra (TOF MS, m/z): calculated 446.01; observed 347.2 (100%), 349.2 (63.92%), 447.10 (40.67%, M + 1), 368.1 (37.74%).
3-[4,6-Bis(4-chlorophenylamino)-1,3,5-triazin-2-yl]thiazolidine-2,5-dione, 7i. Light pink powder; MP 281–283 °C. FTIR (νmax; cm−1, in KBr): 3399.59 (–NH– stretching), 1620.96 (–C
O stretching), 1580.88–1495.08 (–NH2 bending), 1431.00–1155.52 (C–N stretching), 986.80 (C–Cl stretching), 792.14 (C–S stretching). 1H-NMR (300 MHz, CDCl3-d6, TMS) δ ppm: 3.83 (s, 2H, 2 × –NH–Ar), 3.77 (s, 2H, –CH2– thiazolidine), 6.99 (m, 4H, 2 × Ar–H), 7.60–7.75 (d, 4H, J = 4.5 Hz, 2 × Ar–H). 13C-NMR (400 MHz, CDCl3) δ ppm: 163.83 (C
O, thiazolidine), 162.23 (C, triazine), 135.58 (C, triazine), 134.54 (C
O, thiazolidine), 122.81–121.98 (2 × –NH–C, Ar), 115.23–114.63 (4 × C, Ar), 79.16–78.50 (2 × Cl–C, Ar), 40.12–38.87 (4 × C, Ar), 35.95 (–CH2–, thiazolidine). Mass spectra (TOF MS, m/z): calculated 446.01; observed 316.2 (100%), 390.2 (51.65%), 315.2 (40.57%), 447.10 (30.09%, M + 1), 372.2 (26.03%).
3-[4,6-Bis(4-fluorophenylamino)-1,3,5-triazin-2-yl]thiazolidine-2,5-dione, 7j. White powder; MP 123–125 °C. FTIR (νmax; cm−1, in KBr): 3297.56 (–NH– stretching), 1619.16–1600.60 (–C
O stretching), 1568.73–1480.86 (–NH2 bending), 1426.75–1169.27 (C–N stretching), 988.78 (C–F stretching), 714.56 (C–S stretching). 1H-NMR (300 MHz, CDCl3-d6, TMS) δ ppm: 3.35 (s, 2H, 2 × –NH–Ar), 3.58 (s, 2H, –CH2– thiazolidine), 7.08–7.11 (d, 4H, J = 9 Hz, 2 × Ar–H), 7.31–7.35 (d, 4H, J = 12 Hz, 2 × Ar–H). 13C-NMR (400 MHz, CDCl3) δ ppm: 163.84 (C
O, thiazolidine), 139.69 (C, triazine), 133.15 (C
O, thiazolidine), 130.01 (C, triazine), 123.07 (2 × F–C, Ar), 120.29–119.06 (2 × –NH–C, Ar), 79.16–78.50 (4 × C, Ar), 63.33 (4 × C, Ar), 40.15 (–CH2–, thiazolidine). Mass spectra (TOF MS, m/z): calculated 414.07; observed 85.0 (100%), 366.1 (88.11%), 415.1 (74.86%, M + 1), 475.5 (54.79%).
3-[6-Bis(4-bromophenylamino)-1,3,5-triazin-2-yl]thiazolidine-2,5-dione, 7k. Off-white crystals; MP 167–169 °C; FTIR (νmax; cm−1, in KBr): 3388.40 (–NH– stretching), 1586.84 (–C
O stretching), 1556.09–1434.25 (–NH2 bending), 1372.76–1129.67 (C–N stretching), 794.78 (C–Br stretching), 729.98 (C–S stretching). 1H-NMR (300 MHz, CDCl3-d6, TMS) δ ppm: 3.45 (s, 2H, 2 × –NH–Ar), 3.68 (s, 2H, –CH2– thiazolidine), 7.42–7.44 (d, 4H, J = 6 Hz, 2 × Ar–H), 7.73–7.75 (d, 4H, J = 6 Hz, 2 × Ar–H). 13C-NMR (400 MHz, CDCl3) δ ppm: 181.18 (C
O, thiazolidine), 170.31 (C, triazine), 162.96 (C
O, thiazolidine), 135.17 (C, triazine), 129.13–129.00 (2 × –NH–C, Ar), 127.39–126.73 (4 × C, Ar), 79.21–78.55 (4 × C, Ar), 40.13–38.88 (2 × Br–C, Ar), 36.90 (–CH2–, thiazolidine). Mass spectra (TOF MS, m/z): calculated 535.91; observed 347.2 (100%), 348.2 (74.59%), 537.10 (70.91%, M + 1), 349.2 (70.83%).
Biological activity
In vitro DPP-4 inhibitory activity
The synthesized compounds were evaluated for in vitro DPP-4 enzyme inhibition. The enzyme assay was performed using a DPP-4 drug discovery kit (BML-AK 499, Enzo Life Sciences, Plymouth Meeting, PA, USA). The activity of the test compounds was assayed using human recombinant DPP-4 enzyme, chromogenic substrate (H-Gly-Pro-AMC, Km 114 μM), DPP-4 inhibitor (P32/98), assay buffer and the calibration standard provided in the kit. The assay was performed using a 96-well flat-bottomed microtitre plate and was followed by the addition of the assay buffer, DPP-4 enzyme and chromogenic substrate (HGly-Pro-pNA). The assay principle and procedure were followed as per the manufacturer's guidelines. Solutions of the test compounds were made in DMSO at concentrations of 25, 50, 100 and 200 μg mL−1; 20 μL were added to each well after further dilution. The plate was incubated at 37 °C for 10 min to allow the enzyme-inhibitor reaction and was read continuously at A405 nm using a Bio-Rad ELISA plate reader. IC50 values were determined using non-linear regression analysis.
Antibacterial activity
All the synthesized compounds were screened for their MIC (μg mL−1) against selected Gram-positive [B. subtilis (NCIM-2063), B. cereus (NCIM-2156), S. aureus (NCIM-2079)] and Gram-negative [E. coli (NCIM-2065), P. vulgaris (NCIM-2027) and P. aeruginosa (NCIM-2036)] organisms by the broth dilution method with minor modifications as recommended by the National Committee for Clinical Laboratory Standards.19 Cefixime was used as a standard antibacterial agent. Solutions of the test compounds and reference drug were prepared in DMSO at concentrations of 125, 62.5, 31.25, 15.62, 7.81, 3.91, 1.95 and 0.97 μg mL−1. Ten tubes were made in parallel with the second set used as MIC reference controls (16–24 h visual). After sample preparation, the controls were placed in a 37 °C incubator and read for macroscopic growth (clear or turbid) the next day. A 0.8 mL volume of nutrient broth was pipetted into tubes 2–7; tube 1 (negative control) received 1.0 mL of nutrient broth and tube 10 (positive control) received 0.9 mL of nutrient. Tube 1, the negative control, did not contain any bacteria or antibiotic drug. The positive control, tube 10, received 0.9 mL of nutrient broth and contained bacteria but no antibiotic drug. The test compound was dissolved in DMSO (125 μg mL−1) and 0.1 mL of the prepared test compounds were serially diluted from tube 2 to tube 9 (tubes 2–9 contained 125, 62.5, 31.25, 15.62, 7.81, 3.91, 1.95, 0.97 μg mL−1, respectively). After this process, each tube was inoculated with 0.1 mL of the bacterial suspension with a concentration corresponding to 0.5 on the McFarland scale (9 × 108 cells per mL) and each suspension was incubated at 37 °C for 24 h at 150 rpm. The final volume in each tube was 1.0 mL. The incubation chamber was kept humid. At the end of the incubation period, the MIC values were recorded as the lowest concentration of the substance that gave no visible turbidity, i.e. no growth of inoculated bacteria.
Docking study
The computational studies were carried out with the X-ray crystal structure of DPP-4 (PDB ID: 2FJP, Chain A) in complex with (2S,3S)-3-amino-4-(3,3-difluoropyrrolidin-1-yl)-N,N-dimethyl-4-oxo-2-(4-[1,2,4]triazolo[1,5-a]-pyridin-6-ylphenyl)butanamide: a selective alpha-amino amide dipeptidyl peptidase IV inhibitor for the treatment of type 2 diabetes. Docking of the ligand 7a was carried out in the binding pocket of DPP-4 with the CDOCKER protocol of DS 2.5 following the protocol reported elsewhere.20 CDOCKER is a grid-based MD-simulated, annealing-based algorithm that uses CHARMm. The receptor (protein) was held rigid, whereas the ligands were flexible during the refinement.21,22 The basic strategy involves the generation of several initial ligand orientations in the allosteric site of the target protein followed by MD-based simulated annealing and final refinement by minimization.
Acknowledgements
The authors are grateful to SHIATS for providing the necessary infrastructure facilities. The support of SAIF, Punjab University, India is highly appreciated for providing the spectral data of the compounds synthesized herein.
References
- American Diabetes Association, Report of the Expert Committee on the diagnosis and classification of diabetes mellitus, Diabetes Care, 1997, 20, 1183–1201 CrossRef.
- Institute of Medicine (US) Committee on Preventing the Global Epidemic of Cardiovascular Disease: Meeting the Challenges in Developing Countries, ed. Fuster V, Kelly B. B, Promoting Cardiovascular Health in the Developing World: A Critical Challenge to Achieve Global Health, Washington (DC), National Academies Press (US), 2010, 2, Epidemiology of Cardiovascular Disease, http://www.ncbi.nlm.nih.gov/books/NBK45688/.
- J. E. Shaw, R. A. Sicree and P. Z. Zimmet, Diabetes Res. Clin. Pract., 2010, 87, 4–14 CrossRef CAS PubMed.
- C. H. S. McIntosh, Front. Biosci., Landmark Ed., 2008, 13, 1753–1773 CrossRef CAS PubMed.
-
(a) J. J. Holst and C. F. Deacon, Diabetes, 1998, 47, 1663–1670 CrossRef CAS;
(b) M. Zander, S. Madsbad and J. L. Madsen, Lancet, 2002, 359, 824–830 CrossRef CAS;
(c) H. J. Mest and R. Mentlein, Diabetologia, 2005, 48, 616–620 CrossRef CAS PubMed.
- US/EU EudraCT number 2012-004045-33.
- P. Fouqueray, X. Leverve, E. Fontaine, M. Baquié, C. Wollheim, H. Lebovitz and S. Bozec, J. Diabetes Metab., 2011, 2, 126 Search PubMed.
- V. S. Jain, D. K. Vora and C. S. Ramaa, Bioorg. Med. Chem., 2013, 21, 1599–1620 CrossRef CAS PubMed.
- B. B. Lohray, V. B. Lohray, in Drug Discovery and Development, ed. M. S. Chorghade, Wiley-Interscience, 2007, vol. 2, pp. 91–120 Search PubMed.
- American Diabetes Association, Standards of medical care in diabetes, Diabetes Care, 2014, 37, S14–S80 CrossRef PubMed.
- G. M. Shenfield, Drugs, 1982, 23, 462–480 CrossRef CAS PubMed.
- B. Meunier, Acc. Chem. Res., 2008, 41, 69–77 CrossRef CAS PubMed.
- D. Kim, L. Wang, M. Beconi, G. J. Eiermann, M. H. Fisher, H. He, G. J. Hickey, J. E. Kowalchick, B. Leiting, K. Lyons, F. Marsilio, M. E. McCann, R. A. Patel, A. Petrov, G. Scapin, S. B. Patel, R. S. Roy, J. K. Wu, M. J. Wyvratt, B. B. Zhang, L. Zhu, N. A. Thornberry and A. E. Weber, J. Med. Chem., 2005, 48, 141–151 CrossRef CAS PubMed.
- C. Rummey, S. Nordhoff, M. Thiemann and G. Metz, Bioorg. Med. Chem. Lett., 2006, 16, 1405–1409 CrossRef CAS PubMed.
- J. Casqueiro and C. Alves, Indian J. Endocrinol. Metab., 2012, 16, 27–36 CrossRef PubMed.
- J. Davies and D. Davies, Microbiol. Mol. Biol. Rev., 2010, 74, 417–433 CrossRef CAS PubMed.
-
(a) B. Singh, H. R. Bhat, M. K. Kumawat and U. P. Singh, Bioorg. Med. Chem. Lett., 2014, 24, 3321–3325 CrossRef CAS PubMed;
(b) H. R. Bhat, U. P. Singh, P. Gahtori, S. K. Ghosh, K. Gogoi, A. Prakash and R. K. Singh, New J. Chem., 2013, 37, 2654–2662 RSC;
(c) S. Kumar, H. R. Bhat, M. K. Kumawat and U. P. Singh, New J. Chem., 2013, 37, 581–584 RSC;
(d) H. R. Bhat, U. P. Singh, P. Gahtori, S. K. Ghosh, K. Gogoi, A. Prakash and R. K. Singh, RSC Adv., 2013, 3, 2942–2952 RSC;
(e) U. P. Singh, M. Pathak, V. Dubey, H. R. Bhat and R. K. Singh, Chem. Biol. Drug Des., 2012, 80, 572–583 CrossRef CAS PubMed;
(f) V. Dubey, M. Pathak, H. R. Bhat and U. P. Singh, Chem. Biol. Drug Des., 2012, 80, 598–604 CrossRef CAS PubMed;
(g) P. Gahtori, S. K. Ghosh, P. Pratap, A. Prakash, K. Gogoi, H. R. Bhat and U. P. Singh, Exp. Parasitol., 2012, 130, 292–299 CrossRef CAS PubMed;
(h) U. P. Singh, H. R. Bhat and P. Gahtori, J. Mycol. Med., 2012, 22, 134–144 CrossRef CAS PubMed.
- K. R. Alagawadi and S. G. Alegaon, Arabian J. Chem., 2010 DOI:10.1016/j.arabjc.2010.07.012 , in press.
- National Committee for Clinical Laboratory Standards, Standard Methods for Dilution Antimicrobial Susceptibility Test for Bacteria Which Grow Aerobically, NCCLS, Villanova, 1982, p. 242.
- G. Kumari, Nutan, M. Modi, S. K. Gupta and R. K. Singh, Eur. J. Med. Chem., 2011, 46, 1181–1188 CrossRef CAS PubMed.
- F. A. Momany and R. Rone, Comput. Chem., 1992, 13, 888–900 CrossRef CAS.
- G. Wu, D. H. Robertson, C. L. Brooks and M. Vieth, J. Comput. Chem., 2003, 24, 1549–1562 CrossRef CAS PubMed.
|
This journal is © The Royal Society of Chemistry 2015 |
Click here to see how this site uses Cookies. View our privacy policy here.