DOI:
10.1039/D3QI00925D
(Review Article)
Inorg. Chem. Front., 2023,
10, 5212-5224
Single-ion magnetism behaviors in lanthanide(III) based coordination frameworks
Received
18th May 2023
, Accepted 24th July 2023
First published on 25th July 2023
Abstract
Single-ion magnets (SIMs) and single-molecule magnets (SMMs) are typically made up of 3d and/or 4f complexes that possess significant energy barriers to prevent the spin reversal behavior at the molecular level. SMMs/SIMs have various promising applications, including high-density information storage, molecular spintronics, and quantum computers. This article offers an overview of single-ion magnetism in lanthanide(III)-based coordination frameworks that consist of self-assemblies in one-, two-, and three-dimensions. The large magnetic moments and magnetic anisotropy of lanthanide(III) ions make them potential candidates for SIMs/SMMs. Additionally, the coordination framework structure enables multi-functionalities such as responsiveness to external stimuli, proton conduction, high structural stability, and host–guest interactions. These multi-functionalities indicate that the lanthanide(III)-based magnetic metal coordination frameworks represent an exciting platform for the development of a new generation of SIMs/SMMs.
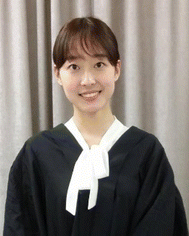 Qingyun Wan | Dr Wan received her Bachelor's degree in Materials Physics from the University of Science and Technology of China in 2014, under the guidance of Prof. Li-Zhu Wu and Prof. Chen-Ho Tung. In 2014, she joined the research group of Prof. Chi-Ming Che at HKU to pursue her PhD in Chemistry, which she completed in 2019. In 2017, she visited and studied at the Massachusetts Institute of Technology, under the guidance of Prof. Christopher C. Cummins. From 2020 to 2022, she visited Prof. Xiaodong Cui's group at the Department of Physics, HKU. In 2020–2023, she went to Tohoku University in Japan for a six-month visit and study, under the guidance of Prof. Masahiro Yamashita. |
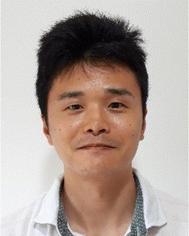 Masanori Wakizaka | Prof. Wakizaka received his Ph.D. degree from Hokkaido University in 2016 (Prof. Masako Kato lab.). After his postdoctoral experience at Tokyo Institute of Technology (Prof. Kimihisa Yamamoto lab.), he worked at Tohoku university at Prof. Masahiro Yamashita lab as well as at the Frontier Research Institute for Interdisciplinary Sciences as an assistant prof. Currently, he is working at Chitose Institute of Science and Technology as an associate prof and an independent principal investigator. |
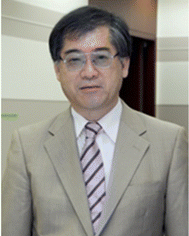 Masahiro Yamashita | Masahiro Yamashita received his B. Sc. degree in 1977, M. Sc. in 1979, and D. Sc. in 1982 from Kyushu University. After his graduation, he joined the Institute for Molecular Science (IMS). In 1983, he was appointed as an Assistant Professor at Kyushu University. In 1988, he was appointed as an Associate Professor at Nagoya University, and he was promoted to full Professor at the same university in 1998. He was a full Professor at Tokyo Metropolitan University from 2000 to 2004. He moved to Tohoku University as a full professor in 2004. He retired from Tohoku University in 2020 and is now an emeritus Professor of Tohoku University. He has been honored with the Inoue Scientific Award (2002), The Chemical Society of Japan Award for Creative Work (2005), the Award of Japan Society of Coordination Chemistry (2014), Mukai Award (2019), and The Chemical Society of Japan Award (2020). He is an Associate Member of Science Council of Japan. He is a Fellow of Royal Society of Chemistry (FRSC) with 517 original papers, 104 review articles, and 20 books. |
Introduction
The field of molecular magnetism has received considerable attention in recent decades,1,2 with the discovery of SMMs/SIMs opening up new avenues of research.1,3–6 These molecules exhibit magnetic behaviours at the molecular level and retain magnetization at low temperatures,4,7 making them promising for high-density information storage, quantum computing, and molecular spintronics.1,3,4 SIMs are considered a subclass of SMMs, where the electron spin term derives from a solitary magnetic center, instead of polynuclear metal complexes.8 In the past several years, molecular magnetism has shifted its attention from polynuclear complexes to SIMs, particularly for those containing f-block elements.8
Many reported lanthanide(III)-type SIMs/SMMs are based on isolated discrete complexes.4,9–34 The construction of Ln(III)-type framework structures enables new functionalities beyond SIMs/SMMs, such as responsiveness to external stimuli, proton conduction, high structural stability, and reversible switching of magnetization by guest molecule absorption.35–43 Slow magnetic relaxation in many framework compounds made by 3d metal ions is usually absent due to the existence of 3D ordered exchange interactions among metal ions, such as Ru and Co in the coordination framework.44–46 For lanthanide(III)-based coordination frameworks, the exchange interactions between lanthanide(III) ions are usually weak, due to the effective shielding effect of unpaired electrons in the 4f orbitals.47 Such weak interatomic exchange interactions in Ln(III)-frameworks can therefore be utilized to promote the appearance of slow magnetic relaxation behaviours, leading to the efficient construction of SIMs/SMMs based on stable multi-functional Ln(III)-frameworks.
The careful selection of bridging ligands can significantly tune the magnetic properties of the lanthanide(III) ion, making lanthanide(III)-type coordination framework compounds a promising class of materials for SIM/SMM development. This review summarizes the recent progress made in the past decade regarding lanthanide(III)-based framework SIMs/SMMs, classified by dimensionalities from 1D to 2D and 3D. A comprehensive review regarding both SIMs/SMMs and magnetocaloric behaviours of Ln(III)-coordination polymers has been given by Liu, Wen and coworkers recently.48 For examples of magnetic metal-coordination frameworks made by either 3d or 4f metal atoms, we recommend reviews by Kurmoo,49 Clérac50 and Harris51 which were reported in 2008, 2012 and 2020, respectively. We believe that the study of lanthanide(III)-type frameworks with SIM/SMM behaviours has the potential to lead to the development of new materials with important applications in fields such as data storage, quantum computing and molecular spintronics.
Background
In order for a material to be considered as an SMM, its magnetic moment must be bistable with an energy barrier between the up and down orientations of the moment, as shown in Fig. 1. The appearance of an energy barrier is closely related to magnetic anisotropy, which describes a molecule's tendency to be magnetized along one direction under a magnetic field. Compounds with orbital angular momentum, such as certain 3d metal complexes, exhibit anisotropy of magnetization and energy splitting of sublevels primarily due to spin–orbit coupling (SOC).9 The sublevels are separated by the energy following the equations: Ueff = |D|MS2 (integer spin) and Ueff = |D|(MS2–0.25) (noninteger total spin), where D is the axial anisotropy parameter. When D < 0, the low-lying energy levels are the ones with the highest |S| values, and when D > 0, the opposite situation shows up and the sublevels are inversely oriented.9,52,53 These two opposite forms of magnetic anisotropy are referred to as the easy axis (hard plane) and easy plane (hard axis), respectively. In the former situation, the lowest amount of energy is required for the magnetization process when the magnetic field is aligned with an axis, whereas in the latter case, it is achieved when the field is in a plane.54 In most situations, the bistable ground state requires a negative D value and easy axis magnetic anisotropy. Several reports have demonstrated slow magnetic relaxation behaviours for complexes with a positive D value,54–56 where spin–lattice relaxation in easy-plane systems occurs directly between the ground MS = ±1/2 levels.55,56
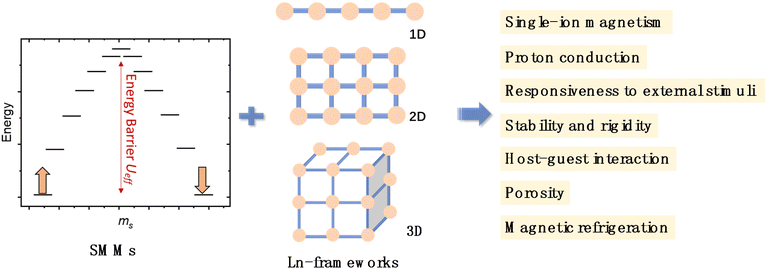 |
| Fig. 1 (Left) Schematic illustration of a potential energy surface as a function of the mS quantum number. (Middle) The construction Ln(III)-framework SIMs/SMMs in one-dimension (1D), two-dimensions (2D) and three-dimensions (3D). Balls and sticks: metal atoms and bridging ligands. (Right) Functionalities by incorporating Ln(III) atoms into the framework. | |
The electronic structures of 4f complexes are intrinsically different compared to those of 3d complexes. In 4f metal coordination compounds with orbital angular momentum, SOC arises from the interaction of the magnetic fields generated by S with the unquenched orbital motion (L), resulting in the angular momentum of J (see Fig. 2). SOC splits the 2S+1LJ ground state into multiplets with different values of J.52 In most cases, the multiplets are well separated in energy, and therefore only the lowest-lying state is populated at room temperature.57 As shown in Fig. 2, the energy difference among multiplets with different J values is on the order of ∼104 cm−1. The ligand field further splits the 2S+1LJ ground state multiplet into 2J + 1 sublevels with a quantum number MJ and an energy difference of ∼102 cm−1, where the magnetization relaxes through these levels. Due to the different SIM/SMM mechanisms between d-based and f-based SIMs/SMMs, the hysteresis temperatures and the potential barriers of f-based SIMs/SMMs are anticipated to be higher than those of d-based SIMs/SMMs. The relaxation of magnetization in single-ion magnets (SIMs) occurs through various mechanisms, as depicted in Fig. 3.8,53 The relaxation mechanisms of SIMs/SMMs are complex, and highly sensitive to numerous factors, such as temperature, magnetic field, hyperfine interactions, and intermolecular interactions. Relaxation of the magnetization occurring only by overcoming the energy barrier with Ueff ∼ 298 K is important to implement the SMM in a practical information storage device at room temperature. In such a situation, by keeping the temperature of the device below 298 K, it is ensured that the magnetization is not lost immediately, and a memory effect is retained.
|  | (1) |
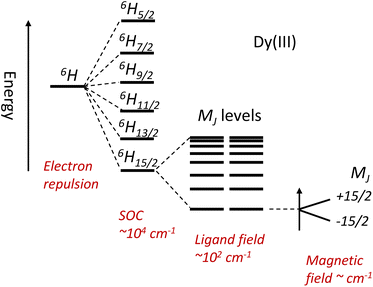 |
| Fig. 2 Electronic interactions in Dy(III) and their typical magnitudes. | |
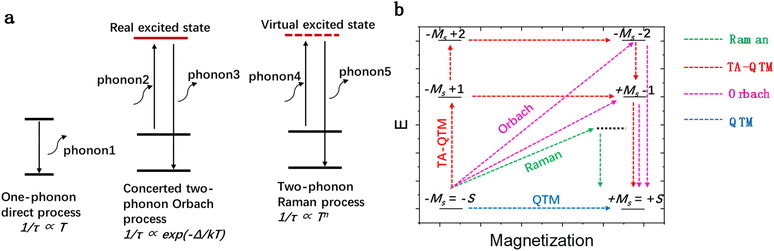 |
| Fig. 3 (a) Illustration of the three primary mechanisms for magnetization relaxation (spin–lattice relaxation) processes and their temperature dependence. (b) A combination of several relaxation mechanisms for a part of double-well energy potential. TA-QTM: Thermally Assisted Quantum Tunnelling of the Magnetization. | |
At low temperatures, a dominant relaxation mechanism of SMMs is usually QTM (quantum tunnelling of the magnetization), which is governed by environmental factors, such as the presence of nuclear spins and dipolar coupling. QTM between magnetic ground states is temperature independent. The magnetization process can also tunnel the energy barrier through excited states, by the thermally activated quantum tunnelling (TA-QTM) process, which is temperature dependent.58 For the design of single molecule magnets, QTM needs to be inefficient, which can be realized in compounds with strictly axial ligand field environments.
At higher temperatures, the coupling between the magnetic moment of SIMs/SMMs with lattice phonons is important for the magnetization process, and the spin–lattice relaxation includes direct, Orbach and Raman processes. These three processes can be differentiated by the mechanism for the absorption or emission of the phonon. In the direct process, the relaxation of the magnetization in SIMs/SMMs from the spin up to the spin down process occurs via the emission of a single phonon. The energy difference between the spin up and down states (+MJ and −MJ) in SIMs/SMMs is induced by Zeeman-splitting with a small value of several cm−1. There are not many phonon states available for an energy as small as ∼cm−1. Therefore, two-phonon processes become more important compared to the one-phonon processes for the magnetization relaxation of SIMs/SMMs. The Raman relaxation process can be understood as a two-phonon process that occurs through a virtual intermediate state of the lattice. The Orbach relaxation process consists of the absorption of a phonon that initially excites the spin system, followed by the relaxation of the spin system to the ground state with the emission of a phonon. The Orbach process can be considered as a concerted two-phonon process.
Notably, only the Orbach process has an exponential temperature dependence as shown in eqn (1), resulting in a straight line in the Arrhenius plot of ln
τ vs. 1/T. An important assumption in eqn (1) is that the phonon spectrum of the lattice can be approximated by the Debye model. This model assumes that the density of phonon states depends quadratically on the phonon frequency up to a maximum frequency, known as the Debye frequency.9
Experimental observations
1D Ln(III)-framework SIMs
The single-ion magnetism behavior in one-dimensional chain-type Ln(III) coordination structures has been extensively studied in the past several decades. Both the magnetic anisotropy of the lanthanide(III) ion and intrachain exchange interactions could influence the effective energy barrier of the spin reversal process. We have summarized recent developments and progress in the research field of single-ion magnet behavior in chain-type Ln(III) structures (Table 1). Among these examples, C.-M. Liu and colleagues reported an interesting one-dimensional chain of Dy(III) single-molecule magnets assembled from linear tetranuclear Dy(III) clusters (1, Table 1) in 2017 (Fig. 4).59 The Dy(III) linear chain structure (1) exhibits an enhanced energy barrier of 144.2 cm−1, compared to the tetranuclear Dy(III) building block clusters with a barrier of 45.4 cm−1. The authors proposed that the higher energy barrier in the Dy(III) chain structure is due to a more favourable crystal field for the Dy(III) ions, compared to the tetranuclear building blocks. This experimental result indicates that the construction of a chain structure could address considerable influence on the SMM properties of Ln(III)-based compounds.
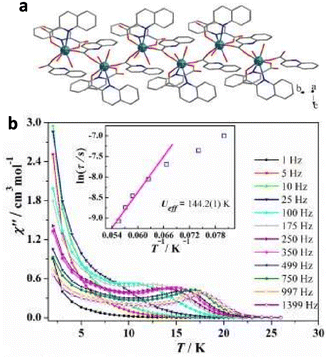 |
| Fig. 4 (a) X-ray crystal structure of compound 1. (b) Temperature dependence of out-of-phase ac susceptibility signals under zero dc field for 1. Reproduced from ref. 59 with permission from the Royal Society of Chemistry. | |
Table 1 Magnetic properties of the 1D Ln(III)-framework SIMs
Ln-framework |
U
eff (K) |
Ref. |
HQL1C: 2-quinolinecarboxylic acid; INNO = isonicotinate N-oxide, Bza = benzoic acid; 2,7-NDS = 2,7-naphthalenedisulfonate; IP = 1H-imidazo[4,5-f][1,10]-phenanthroline; DClQ = 5,7-dichloro-8-hydroxyquinoline; hfac = hexafluoroacetylacetonate; NITThienPh = 2-(5-phenyl-2-thienyl)-4,4,5,5-tetramethyl-imidazoline-1-oxyl-3-oxide; [H2L2] = N,N′-bis(3-methoxysalicylidene)-1,3-diaminopropane; POC- = pyridin-N-oxide-4-carboxylate; and [H2L3] = N,N′-bis(3-methoxysalicylidene)-1,2-diaminoethane. |
[Dy(QL1C)3(H2O)2]n (1) |
144.2(1) |
59
|
[Dy(INNO)(Bza)2(H2O)2]·(H2O) (2) |
— |
60
|
[Dy(μ-H2O)(phen)(μ-OH)(nb)2]n (3) |
88.7 |
61
|
[Dy0.05Y0.95(μ-H2O)(phen)(μ-OH)(nb)2]n (4) |
78.8 |
61
|
{[Dy(2,7-NDS)(IP)(OH)(H2O)2]·mH2O}n (5) |
82.39 |
62
|
[Dy(DClQ)2(N3)]n (6) |
45.9 |
63
|
[Dy2(hfac)6(NITThienPh)2]n (7) |
53/98 |
64
|
{[DyZn2(L2)2(POC)](OH)(ClO4)}·H2O·MeOH (8) |
235.3(3.1) |
65
|
{[Dy3Zn7(L3)6(POC)6](OH)3(ClO4)2}·9H2O (9) |
14.6(0.2) |
65
|
The magnetic moment of 4f elements has been observed to effectively tunnel under a barrier, which can be mitigated by applying a small external magnetic field.9 This field can be generated by a neighbouring spin on the ligand, thereby suppressing tunnelling in a zero-field environment. The examples of lanthanide(III)-radical-based SIMs include the azido-radical bridged lanthanide(III) dimer [{[(Me3Si)2N]2(THF)Tb}2(μ-γ2:γ2-N2)]−, which exhibits stable magnetization over extended periods of time with an energy barrier of 327 K,66 and complex Dy(hfac)3(boaDTDA) (hfac = 1,1,1,5,5,5-hexafluoroacetylacetonato; boaDTDA = 4-(benzoxazol-2′-yl)-1,2,3,5-dithiadiazolyl) where the boaDTDA ligand carries the radical.67 Such a radical strategy can also be utilized to construct Ln(III)-based chain structures, such as the ferromagnetic chain structure [Sm(hfac)3(boaDTDA)]n with the ordering transition at Tc = 3 K, reported by Preuss and co-workers in 2016.68,69 The dysprosium chain compound (6) is assembled by an azido-bridged (N3−) radical ligand, which was reported by Tian and co-workers in 2021. Compound 6 shows slow magnetic relaxation under zero-field,63 with an effective energy barrier of around 45.9 K and ferromagnetic coupling between the discrete Dy(III) ions (Fig. 5).
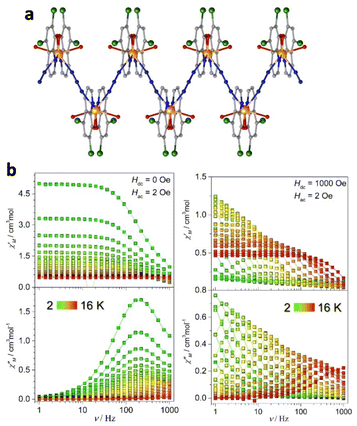 |
| Fig. 5 (a) X-ray crystal structure of compound 6. (b) Plot of the in-phase (χ′) and out-of-phase (χ′′) magnetic susceptibility from 2 to 16 K for 6 under zero and 1 kOe fields. Reproduced from ref. 63 with permission from the Wiley-VCH. | |
Besides the azido-type bridged ligands, other bridging ligands have been extensively used to construct Ln(III) 1D chain structures. Compound 1–2 and 8–9 were bridged by carboxylic-type ligands.59,60 Compound 5 was bridged by sulfonate ligands with an energy barrier of 82.39 K.62 Compounds 3 and 4 and compound 7 were bridged by a monoatomic oxygen atom and an imidazoline-type ligand, respectively.61,64 An intrachain ferromagnetic interaction with the coupling constant J = 3.04 cm−1 was observed in complexes 3 and 4. Compound 7 was reported to be antiferromagnetic at the ground state. These 1D Ln(III)-chain type structures with different bridging ligands show a wide range of Ueff, as summarized in Table 1.
2D Ln(III)-framework SIMs
The synthesis of two-dimensional (2D) dysprosium–organic complexes has garnered increasing interest in recent years for the study of SMM behaviours at the 2D limit. A summary of 2D Ln(III)-based SMM frameworks with their respective effective energy barriers is displayed in Table 2. A high Ueff value of approximately 160 K was observed in 2D Ln(III)-based coordination frameworks of compound 10 (Dy(L1)(HL1)(phen), Table 2) under a zero dc field.71 In 2021, a 2D Dy(III)-MOF compound 11 was synthesized using a microwave-assisted method, and it can be further exfoliated into stable 2D nanosheets by sonication (Fig. 6). Compound 11 does not show a frequency dependent out-of-phase χ′′ signal under H = 0. By applying a dc field, the quantum tunnelling of the magnetization (QTM) was effectively suppressed in 11, resulting in slow relaxation of the magnetization with an effective energy barrier of 15.3 K in the range 5–8 K.70 Compound 11 was reported to show ferromagnetic interactions between adjacent Dy(III) ions at low temperatures.
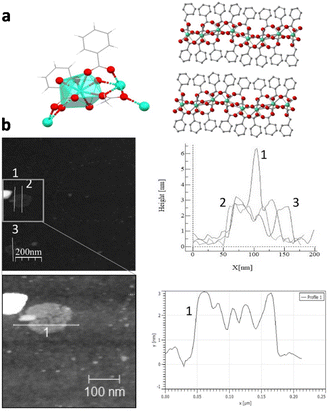 |
| Fig. 6 (a) X-ray crystal structure of compound 11. (b) AFM image of a thermally oxidized Si wafer on which two drops of exfoliated 11 in i-PrOH were deposited by spin coating. Reproduced from ref. 70 with permission from the Wiley-VCH. | |
Table 2 Magnetic properties of the 2D Ln(III)-framework SIMs
Ln-framework |
U
eff (K) |
Ref. |
H2L1: 3,5-dichloro-4-hydroxy benzoic acid; Cl2An: chloranilate (2,5-dihydroxy-1,4-benzoquinone dianion); Hm-dobdc: 4,6-dioxido-1,3-benzenedicarboxylate; H4L2: N′,N′′-((1E,1′E)-[2,2′-bipyridine]-6,6′-diylbis(methaneylylidene))bis(2-hydroxybenzohydrazide); H3L3 = (HO)2P(O)CH2CO2H; H3CAM = 4-hydroxypyridine-2,6-dicarboxylic acid; and H2L4: N′-(2-hydroxy-3-methoxybenzylidene)pyridine-N-oxidecarbohydrazide. |
Dy(L1)(HL1)(phen) (10) |
160 |
71
|
[Dy(MeCOO)-(PhCOO)2]n (11) |
15.3 |
70
|
[Dy(Cl2An)1.5(DMF)2]n (12) |
145(7) |
72
|
[Dy(Hm-dobdc) (H2O)2]·H2O (13) |
35.3 |
73
|
[Dy2(H2L2)2(H2O)(CH3OH)](ClO4)2H2O (14) |
91.6 |
74
|
[Dy(L3)(H2O)2]n (15) |
72 |
75
|
[Er(L3)(H2O)2]n (16) |
38.42 |
75
|
{[Dy2(HCAM)3(H2O)4]2H2O}n (17) |
63.5/57.1 |
76
|
[Dy(L4)(CH3COO)]·0.5DMF·H2O·2CH3OH (18) |
33.6 |
77
|
[Dy(L4)(CH3COO)]·0.5DMF (19) |
42 |
77
|
In addition to SMM properties, multifunctional 2D Dy-coordination polymer materials can be fabricated, such as compound 13 ([Dy(Hm-dobdc) (H2O)2]·H2O, Table 2) which exhibits magnetism, proton conduction, and luminescence simultaneously.73 The tuning of slow magnetic relaxation by a host–guest interaction has also been observed in compounds 18 and 19.77 Compound 19 was observed to undergo a reversible phase transition induced by the desorption and absorption of methanol and water molecules (Fig. 7). An antiferromagnetic interaction was observed between Dy(III) ions within the dinuclear core of compound 19.
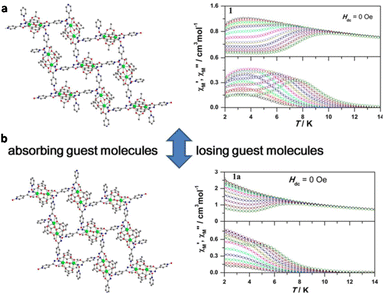 |
| Fig. 7 X-ray crystal structure and magnetic properties of compounds (a) 18 and (b) 19. Reproduced from ref. 77 with permission from the American Chemical Society. | |
Here, we provide a summary of the bridging ligands used to create 2D Ln(III)-coordination framework compounds, together with magnetic interactions among adjacent Ln(III) atoms. Carboxylic-type ligands have been utilized to construct compounds 10, 13 and 17.71,73,76 In compound 10, a weak ferromagnetic coupling between two Dy(III) ions within a Dy2 dimer was observed in the temperature range of 90–200 K. Magnetic investigations revealed the existence of intramolecular ferromagnetic interactions among Dy(III) ions in compounds 13 and 17. Compounds 11 and 12 were bridged by monoatomic oxygen atoms and a benzoquinone ligand, respectively,70,72 and an anti-ferromagnetic interaction has been observed between Dy(III) atoms in compound 12. Diacylhydrazone was used as the bridging ligand to create 2D structures of compound 14.74 Phosphonoacetic acid and a carbohydrazide ligand can also be utilized as a linker to make compounds 15 and 16
75 and compounds 18 and 19 respectively.77 The temperature dependence of the dc susceptibility of compound 16 shows antiferromagnetic couplings among Er(III) atoms, while ferromagnetic interaction was observed in compound 15 at low temperature.
3D Ln(III)-framework SIMs
Multitopic organic and inorganic linkers offer a means of organizing metal cluster nodes into a regular three-dimensional array. To create a 3D array of Ln(III) atoms, various types of bridging ligands can be utilized. Linkers such as carboxylic-type ligands have been used to create compounds 23, 26, 29–33, 35–41, 46, 49–50 and 52.35,42,78–88 Compounds 31 and 32 have been reported to show ferromagnetic coupling in 3D frameworks, and such a magnetic interaction makes a positive contribution to the energy barrier of SMM/SIM properties. Magnetic studies of compound 33 reveal the existence of weak antiferromagnetic interactions among Dy(III) ions. The connectivity of the Dy(III)-carboxylate chains leads to weak ferromagnetic interactions between the Dy(III) ions in compound 36.83 Compounds 38–41 are proposed to be paramagnetic based on the rectilinear drop of the χM−1vs. T plot.85 At low temperature (<4 K), a slight increase in the χMT value for compound 49 indicates weak ferromagnetic interactions between Dy(III) ions.86 An exchange of the coordinated and guest solvent molecules (acetone or DMF) was reported to result in different magnetic relaxation and ferroelectric properties of compound 50.87 Compound 22 was bridged by the benzoquinone ligand, where the neighbouring Dy(III) atoms show anti-ferromagnetic exchange interaction.72 Hydrazide and/or a 4,4′-bypyridyl-N,N′-dioxide ligand was employed to construct compounds 24, 25, 27 and 44–45.37,89,90 Complexes 24 and 25 show antiferromagnetic interactions with J values of −2.68 cm−1 and −2.39 cm−1, respectively. The ligand tetrathiafulvalene tetrabenzoate was used to link Ln(III) building blocks for the framework compound of 28.91 A phosphonate ligand was used to produce 3D coordination framework structures of compounds 34 and 51.92,93 Compound 51 was found to show antiferromagnetic coupling interactions among Dy(III) ions, as the χM−1vs. T plot fitted by the Curie–Weiss law [χM = C/(T − θ)] gives a negative θ value of −2.58 K.92 An oxalate-type ligand has been used to construct compounds 26 and 42–43.35,94 Compound 26 was reported to show ferromagnetic coupling in the 3D Dy(III) coordination framework structures. Here, we will present several 3D Ln(III)-based framework compounds for which SMM properties have been described (Table 3).
Table 3 Magnetic properties of the 3D Ln(III)-framework SIMs
Ln-framework |
U
eff (K) |
Ref. |
HINO = isonicotinic acid N-oxide; Cl2An: chloranilate (2,5-dihydroxy-1,4-benzoquinone dianion); A = pamoic acid, DMA = dimethylacetamide; H2L1 = N′-(2-hydroxybenzylidene)-picolinohydrazide; bpdo: 4,4′-bipyridine-N,N′-dioxide; TTFTB4−: tetrathiafulvalene tetrabenzoate; H3TCA = 4,4′,4′′-tricarboxytriphenylamine; DMA = N,N-dimethylacetamide; H2FDA: furan-2,5-dicarboxylic acid; dhbdc: 2,5-dihydroxyterephthalic acid; amp2H4 = prephotodimerized 9-anthrylmethylphosphonic acid; H2OBA = 4,4′-oxybis(benzoate) acid, Hatz = 3-amino-1,2,4-triazole; H2DMTDC = 3,4-dimethylthieno[2,3-b]thiophene-2,5-dicarboxylic acid; H2azdc = 4,4′-azobenzoic acid, DMA = N,N-dimethylacetamide; pmdc: pyrimidine-4,6-dicarboxylate; tpatox: N,N′,N′′-tris(4-phenyl)aminetris(oxamate); hfac = hexafluoro-acetylacetonate, PyNO = 4-pyridine-N-oxide; BTC = 1,3,5-benzenetricarboxylate; HIN = isonicotinic acid; H2DMTP-DC = 2′,5′-dimethoxytriphenyl-4,4′′-dicarboxylic acid; H2L2 = pamoic acid; H3L3: tris(4-carboxyphenyl)phosphane oxide; H3BTB: (4-carboxyphenyl)benzene; Na2H2L4 = disodium-2,2′-disulfonate-4,4′-oxydibenzoic acid; H2L5 = mucic acid; and OxH2 = oxalic acid. |
K4[Dy(NCS)4(H2O)4](NCS)3(H2O)2 (20) |
60 |
95
|
Dy2(INO)4(NO3)2·2CH3CN (21) |
109 |
43
|
[{Dy(Cl2An)1.5(CH3OH)}·4.5H2O]n (22) |
175(9) |
72
|
[Dy(PA)(NO3)(DMA)3]n (23) |
22.58 |
78
|
{[Dy(L1)(μ2-bpdo)0.5(μ4bpdo)0.5(CH3OH)]·ClO4·3CH3OH}n (24) |
393 |
89
|
{[Dy(L1)(μ2-bpdo)0.5(μ4-bpdo)0.5(CH3OH)]·ClO4}n (25) |
348 |
89
|
{[Dy(C2O4)1.5phen]·0.5H2O}n (26) |
35.5/32.6 |
35
|
[Ln(bipyNO)4](TfO)3·xsolvent (27) |
17.9 |
90
|
[Dy9(μ3-OH)13(μ3-O)(H2O)9(TTFTB)3] (28) |
3.4 |
91
|
{[Dy5(μ2-O)(μ3-OH)5(TCA)2(NO3)(CH3COO)(H2O)2]·NH(CH3)2·DMA·(H2O)3}n (29) |
1.7 |
79
|
{[Er2(μ2-OH)(μ3-OH)2(TCA)(H2O)]·DMA·H2O}n (30) |
8.7 |
79
|
{[Dy2(FDA)3(DMF)2]·1.5DMF}n (31) |
37.0 |
80
|
[Dy2(FDA)3(DMF)2(CH3OH)]n (32) |
46.0 |
80
|
{[Dy(dhbdc)1.5(DMF)2]·DMF} (33) |
31.6 |
81
|
Dy2(amp2H2)2(C2O4)(H2O)210H2O (34) |
41.5 |
93
|
[(Me2NH2)[Dy(OBA)2](Hatz)(H2O)1.5]n (35) |
15 |
82
|
{[Dy(DMTDC)1.5(H2O)2]·0.5DMF·0.5H2O}n (36) |
48.29 |
83
|
[Dy2(azdc)3(DMA)2]n·2n(DMA) (37) |
8.3 |
84
|
{[Dy(μ-pmdc)(μ-ox)0.5(H2O)3]·2H2O}n (38) |
35/41 |
85
|
{[Dy(μ3-pmdc)(μ-ox)0.5(H2O)2]·2.33H2O}n (39) |
18/23 |
85
|
{[Dy(μ3-pmdc)(μ-ox)0.5(H2O)2]·H2O}n (40) |
22/29 |
85
|
{[Dy(μ4-pmdc)(NO3)(H2O)]·H2O}n (41) |
44 |
85
|
{[Dy6(H2O)24[Co4(tpatox)4]](NO3)6}·53H2O (42) |
4.66 |
94
|
{[Er6(H2O)24[Co4(tpatox)4]](NO3)6}·58H2O (43) |
14.24 |
94
|
[(Dy(hfac)3)2(PyNO)3] (44) |
24.4 |
37
|
{[Dy(hfac)3]2(4,4′BipyNO)2]} (45) |
10.3 |
37
|
Dy(BTC) (46) |
45.88 |
42
|
[Dy2Cu4I3(IN)7(DMF)2]·DMF (47) |
2.11 |
100
|
[Dy2Cu4I3(IN)7(DMA)2]·DMA (48) |
11.6 |
100
|
{[Dy(DMTP-DC)1.5(H2O)3]·DMF}n (49) |
4.16 |
86
|
{[Dy2(L2)3(H2O)4]·(acetone)2·(H2O)3}n (50) |
1.91 |
87
|
{[Dy(L3)(H2O)]·2CH3CN}n (51) |
53.5 |
92
|
[Dy(BTB)H2O]n (52) |
17.8 |
88
|
{[Dy4(OH)4(L4)2(H2O)8]·4.6H2O·1.4CH3CN}n (53) |
30.4 |
101
|
{[Ln(L5)(Ox)(H2O)]n·xH2O} (54) |
36.5 |
102
|
Thiocyanate, with delocalized charge and a polarizable π system, is a very versatile inorganic ligand.96,97 Thiocyanate is composed of two donor atoms of nitrogen and sulfur, which can both coordinate to metals, resulting in the formation of homo- or hetero-metallic MOFs involving various combinations of metal ion node points.96–99 These combinations lead to the formation of materials with interesting solid-state structures and hetero-metallic arrays. In a recent work by Yamashita and coworkers, three new Dy3+, Tb3+ and Gd3+ based coordination frameworks were reported, which are linked by inorganic SCN ligands and K+ ions.95 By examining the magnetic properties (Fig. 8), compound 20 was observed to show SMM properties with slow magnetic relaxation behavior under zero dc field and a Ueff of 60 K.
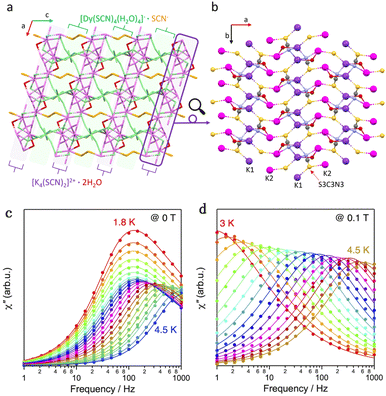 |
| Fig. 8 (a and b) X-ray crystal structure of compound 20, K4[Dy(NCS)4(H2O)4](NCS)3(H2O)2. Frequency dependence of the out-of-phase magnetic susceptibility χ′′ at (c) zero field in the temperature range of 1.8–4.5 K, and (d) 0.1 T in the temperature range of 3–4.5 K of compound 20.95 | |
The host–guest interactions in 3D Ln(III)-based coordination frameworks can address a significant influence on the magnetization relaxation process. In 2013, Li, Shi, and their colleagues reported a reversible sorption and desorption process between compound Dy(BTC) (46) and Dy(BTC)(H2O)DMF by coordinating water and DMF molecules (Fig. 9).42 Compound 46 shows SMM behaviour with an energy barrier of 45.88 K and a pre-exponential factor of τ0 = 2.43 × 10−6 s, while in compound Dy(BTC)(H2O)DMF with water and DMF molecule coordination, no peak in the out-of-phase ac susceptibility (χ′′) plot was observed. These experimental observations indicate that the host–guest interactions can effectively switch-on and -off the slow magnetic relaxation in a classic lanthanide(III) metal–organic framework system. Similarly, in 2015, Shi, Chibotaru, Gao, Long and co-workers reported a 3D MOF made with Dy2 single-molecule magnets as nodes (compound 21, Fig. 10). Guest solvent exchange between DMF and CH3CN induces a reversible single-crystal to single-crystal transformation of compound Dy2(INO)4(NO3)2·2CH3CN (21).43 A slow magnetic relaxation process was observed in 21 with an effective energy barrier of 109 K. In contrast, no slow magnetic relaxation process has been observed for the compound 21 with DMF as the guest solvent, in which the guest CH3CN molecule in compound 21 was replaced by a DMF molecule. The solvent guest-molecule effect observed in compound 21 was reported to be due to a slowing of the relaxation rate of incoherent quantum tunnelling of the magnetization.43
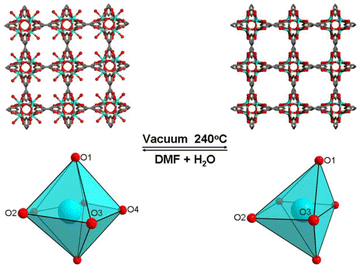 |
| Fig. 9 The structural changes of the framework and the coordination geometry of Dy(III) ions in compound 46. Reproduced from ref. 42 with permission from the Royal Society of Chemistry. | |
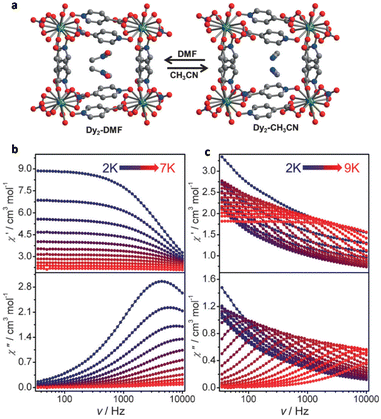 |
| Fig. 10 (a) X-ray crystal structure and magnetic properties of compound 21. (b and c) Frequency dependence of in-phase (χ′) and out-of-phase (χ′′) ac susceptibilities under zero dc field at indicated temperatures for Dy2-DMF (b) and Dy2-CH3CN (21, c) of compound 21. Reproduced from ref. 43 with permission from the Wiley-VCH. | |
Tunable proton conduction can also be realized in Ln(III) frameworks, together with the functionalities of magnetic refrigeration and slow magnetic relaxation. For example, 53 was reported to show field-induced slow magnetic relaxation properties with an effective energy barrier of 30.4 K and a proton conductivity of 2.96 × 10−6 S cm−1 under high temperature and humidity conditions.101 An interesting channel-mediated proton conduction functionality shows up in a 54 Dy(III) framework with field induced single-molecule-magnetic behavior.102
Tables 1–3 summarize the Ln(III)-frameworks exhibiting single-ion magnetism, with Dy(III) contributing the most cases. In contrast, other Ln(III)-frameworks, such as Gd(III) or Tb(III), typically exhibit multiple Debye relaxations and a lack of slow magnetization under a zero direct current (DC) field.95 As a result, structures of Dy(III)-frameworks have been reviewed and described in greater detail herein.
Conclusions
Magnetic metal coordination frameworks are an intriguing platform for developing the next generation of multi-functional magnets. Compared to inorganic solids, metal coordination frameworks offer several advantages, such as customizable structures with controllable porosity and post-synthetic chemical modification. This review summarizes recent progress in Ln(III)-based coordination frameworks that exhibit SMM/SIM properties. We believe that the study of Ln(III)-based coordination frameworks with SMM behaviours have the potential to lead to the development of new materials with applications in fields such as data storage and quantum computing.
Author contributions
The manuscript was written through contributions of all authors. All authors have given approval to the final version of the manuscript.
Conflicts of interest
There are no conflicts to declare.
Acknowledgements
This work was supported by the JSPS KAKENHI Grant Number JP19H05631 and the National Natural Science Foundation of China (NSFC, 22150710513). M. Y. Thanks the 111 projects (B18030) from China.
References
- R. Sessoli, D. Gatteschi, A. Caneschi and M. Novak, Magnetic bistability in a metal-ion cluster, Nature, 1993, 365, 141–143 CrossRef CAS
.
- R. Sessoli, H. L. Tsai, A. R. Schake, S. Wang, J. B. Vincent, K. Folting, D. Gatteschi, G. Christou and D. N. Hendrickson, High-spin molecules:[Mn12O12(O2CR)16(H2O)4], J. Am. Chem. Soc., 1993, 115, 1804–1816 CrossRef CAS
.
- D. Gatteschi and R. Sessoli, Quantum tunneling of magnetization and related phenomena in molecular materials, Angew. Chem., Int. Ed., 2003, 42, 268–297 CrossRef CAS PubMed
.
- D. N. Woodruff, R. E. Winpenny and R. A. Layfield, Lanthanide single-molecule magnets, Chem. Rev., 2013, 113, 5110–5148 CrossRef CAS PubMed
.
- M. Yamashita, Next generation multifunctional nano-science of advanced metal complexes with quantum effect and nonlinearity, Bull. Chem. Soc. Jpn., 2021, 94, 209–264 CrossRef CAS
.
- E. Coronado, Molecular magnetism: from chemical design to spin control in molecules, materials and devices, Nat. Rev. Mater., 2020, 5, 87–104 CrossRef
.
- P. Zhang, Y.-N. Guo and J. Tang, Recent advances in dysprosium-based single molecule magnets: Structural overview and synthetic strategies, Coord. Chem. Rev., 2013, 257, 1728–1763 CrossRef CAS
.
- S. G. McAdams, A.-M. Ariciu, A. K. Kostopoulos, J. P. Walsh and F. Tuna, Molecular single-ion magnets based on lanthanides and actinides: Design considerations and new advances in the context of quantum technologies, Coord. Chem. Rev., 2017, 346, 216–239 CrossRef CAS
.
- S. T. Liddle and J. van Slageren, Improving f-element single molecule magnets, Chem. Soc. Rev., 2015, 44, 6655–6669 RSC
.
- I. F. Diaz-Ortega, J. M. Herrera, D. Aravena, E. Ruiz, T. Gupta, G. Rajaraman, H. Nojiri and E. Colacio, Designing a Dy2 single-molecule magnet with two well-differentiated relaxation processes by using a nonsymmetric Bis-bidentate bipyrimidine-n-oxide ligand: a comparison with mononuclear counterparts, Inorg. Chem., 2018, 57, 6362–6375 CrossRef CAS PubMed
.
- L. Zhang, J. Jung, P. Zhang, M. Guo, L. Zhao, J. Tang and B. Le Guennic, Site-resolved two-step relaxation process in an asymmetric Dy2 single-molecule magnet, Chem. – Eur. J., 2016, 22, 1392–1398 CrossRef CAS PubMed
.
- E. Echenique-Errandonea, A. Zabala-Lekuona, J. Cepeda, A. Rodríguez-Diéguez, J. Seco, I. Oyarzabal and E. Colacio, Effect of the change of the ancillary carboxylate bridging ligand on the SMM and luminescence properties of a series of carboxylate-diphenoxido triply bridged dinuclear ZnLn and tetranuclear Zn2Ln2 complexes (Ln=Dy, Er), Dalton Trans., 2019, 48, 190–201 RSC
.
- C. Shi, R. Nie, X. Yao, S. Fan, G. An, Y. Dong and G. Li, Asymmetry-unit-dominated double slow-relaxation modes of 2, 6-dimethyl-3, 5-heptanedione dysprosium SMMs, RSC Adv., 2017, 7, 49701–49709 RSC
.
- J. Ruiz, A. J. Mota, A. Rodríguez-Diéguez, S. Titos, J. M. Herrera, E. Ruiz, E. Cremades, J. P. Costes and E. Colacio, Field and dilution effects on the slow relaxation of a luminescent DyO9 low-symmetry single-ion magnet, Chem. Commun., 2012, 48, 7916–7918 RSC
.
- A. Arauzo, A. Lazarescu, S. Shova, E. Bartolomé, R. Cases, J. Luzón, J. Bartolomé and C. Turta, Structural and magnetic properties of some lanthanide (Ln=Eu(III), Gd(III) and Nd(III)) cyanoacetate polymers: field-induced slow magnetic relaxation in the Gd and Nd substitutions, Dalton Trans., 2014, 43, 12342–12356 RSC
.
- N. Ishikawa, M. Sugita, T. Ishikawa, S.-Y. Koshihara and Y. Kaizu, Lanthanide double-decker complexes functioning as magnets at the single-molecular level, J. Am. Chem. Soc., 2003, 125, 8694–8695 CrossRef CAS PubMed
.
- N. Koike, H. Uekusa, Y. Ohashi, C. Harnoode, F. Kitamura, T. Ohsaka and K. Tokuda, Relationship between the skew angle and interplanar distance in four bis (phthalocyaninato) lanthanide (III) tetrabutylammonium salts ([NBun4][LnIIIPc2]; Ln=Nd, Gd, Ho, Lu), Inorg. Chem., 1996, 35, 5798–5804 CrossRef CAS
.
- F. Habib, G. Brunet, V. Vieru, I. Korobkov, L. F. Chibotaru and M. Murugesu, Significant enhancement of energy barriers in dinuclear dysprosium single-molecule magnets through electron-withdrawing effects, J. Am. Chem. Soc., 2013, 135, 13242–13245 CrossRef CAS PubMed
.
- J. Long, F. Habib, P.-H. Lin, I. Korobkov, G. Enright, L. Ungur, W. Wernsdorfer, L. F. Chibotaru and M. Murugesu, Single-molecule magnet behavior for an antiferromagnetically superexchange-coupled dinuclear dysprosium(III) complex, J. Am. Chem. Soc., 2011, 133, 5319–5328 CrossRef CAS PubMed
.
- F. Habib, P.-H. Lin, J. Long, I. Korobkov, W. Wernsdorfer and M. Murugesu, The use of magnetic dilution to elucidate the slow magnetic relaxation effects of a Dy2 single-molecule magnet, J. Am. Chem. Soc., 2011, 133, 8830–8833 CrossRef CAS PubMed
.
- P. Zhang, L. Zhang, C. Wang, S. Xue, S.-Y. Lin and J. Tang, Equatorially coordinated lanthanide single ion magnets, J. Am. Chem. Soc., 2014, 136, 4484–4487 CrossRef CAS PubMed
.
- I. Oyarzabal, J. Ruiz, J. M. Seco, M. Evangelisti, A. Camón, E. Ruiz, D. Aravena and E. Colacio, Rational Electrostatic Design of Easy-Axis Magnetic Anisotropy in a ZnII-DyIII-ZnII Single-Molecule Magnet with a High Energy Barrier, Chem. – Eur. J., 2014, 20, 14262–14269 CrossRef CAS PubMed
.
- Y. S. Ding, N. F. Chilton, R. E. Winpenny and Y. Z. Zheng, On approaching the limit of molecular magnetic anisotropy: a near-perfect pentagonal bipyramidal dysprosium(III) single-molecule magnet, Angew. Chem., Int. Ed., 2016, 55, 16071–16074 CrossRef CAS PubMed
.
- J. Liu, Y.-C. Chen, J.-L. Liu, V. Vieru, L. Ungur, J.-H. Jia, L. F. Chibotaru, Y. Lan, W. Wernsdorfer and S. Gao, A stable pentagonal bipyramidal Dy(III) single-ion magnet with a record magnetization reversal barrier over 1000 K, J. Am. Chem. Soc., 2016, 138, 5441–5450 CrossRef CAS PubMed
.
- A. B. Canaj, S. Dey, C. Wilson, O. Céspedes, G. Rajaraman and M. Murrie, Engineering macrocyclic high performance pentagonal bipyramidal Dy(III) single-ion magnets, Chem. Commun., 2020, 56, 12037–12040 RSC
.
- R. J. Blagg, L. Ungur, F. Tuna, J. Speak, P. Comar, D. Collison, W. Wernsdorfer, E. J. McInnes, L. F. Chibotaru and R. E. Winpenny, Magnetic relaxation pathways in lanthanide single-molecule magnets, Nat. Chem., 2013, 5, 673–678 CrossRef CAS PubMed
.
- F. Pointillart, K. Bernot, S. Golhen, B. Le Guennic, T. Guizouarn, L. Ouahab and O. Cador, Magnetic memory in an isotopically enriched and magnetically isolated mononuclear dysprosium complex, Angew. Chem., 2015, 127, 1524–1527 CrossRef
.
- S. K. Langley, D. P. Wielechowski, V. Vieru, N. F. Chilton, B. Moubaraki, B. F. Abrahams, L. F. Chibotaru and K. S. Murray, A {CrIII2DyIII2} Single-Molecule Magnet: Enhancing the Blocking Temperature through 3d Magnetic Exchange, Angew. Chem., Int. Ed., 2013, 52, 12014–12019 CrossRef CAS PubMed
.
- Y.-N. Guo, G.-F. Xu, P. Gamez, L. Zhao, S.-Y. Lin, R. Deng, J. Tang and H.-J. Zhang, Two-step relaxation in a linear tetranuclear dysprosium(III) aggregate showing single-molecule magnet behavior, J. Am. Chem. Soc., 2010, 132, 8538–8539 CrossRef CAS PubMed
.
- J. D. Hilgar, M. G. Bernbeck and J. D. Rinehart, Million-fold relaxation time enhancement across a series of phosphino-supported erbium single-molecule magnets, J. Am. Chem. Soc., 2019, 141, 1913–1917 CrossRef CAS PubMed
.
- F.-S. Guo, B. M. Day, Y.-C. Chen, M.-L. Tong, A. Mansikkamäki and R. A. Layfield, Magnetic hysteresis up to 80 kelvin in a dysprosium metallocene single-molecule magnet, Science, 2018, 362, 1400–1403 CrossRef CAS PubMed
.
- C. A. Goodwin, F. Ortu, D. Reta, N. F. Chilton and D. P. Mills, Molecular magnetic hysteresis at 60 kelvin in dysprosocenium, Nature, 2017, 548, 439–442 CrossRef CAS PubMed
.
- P. Evans, D. Reta, G. F. Whitehead, N. F. Chilton and D. P. Mills, Bis-monophospholyl dysprosium cation showing magnetic hysteresis at 48 K, J. Am. Chem. Soc., 2019, 141, 19935–19940 CrossRef CAS PubMed
.
- A. Borah and R. Murugavel, Magnetic relaxation in single-ion magnets formed by less-studied lanthanide ions Ce(III), Nd(III), Gd(III), Ho(III), Tm(II/III) and Yb(III), Coord. Chem. Rev., 2022, 453, 214288 CrossRef CAS
.
- C.-M. Liu, D.-Q. Zhang and D.-B. Zhu, A 3D MOF constructed from dysprosium(III) oxalate and capping ligands: ferromagnetic coupling and field-induced two-step magnetic relaxation, Chem. Commun., 2016, 52, 4804–4807 RSC
.
- M. Chen, E. C. Sañudo, E. Jimenez, S.-M. Fang, C.-S. Liu and M. Du, Lanthanide–organic coordination frameworks showing new 5-connected network topology and 3D ordered array of single-molecular magnet behavior in the Dy case, Inorg. Chem., 2014, 53, 6708–6714 CrossRef CAS PubMed
.
- X. Yi, G. Calvez, C. Daiguebonne, O. Guillou and K. Bernot, Rational organization of lanthanide-based SMM dimers into three-dimensional networks, Inorg. Chem., 2015, 54, 5213–5219 CrossRef CAS PubMed
.
- H. Huang, W. Gao, X.-M. Zhang, A.-M. Zhou and J.-P. Liu, 3D Ln III-MOFs: displaying slow magnetic relaxation and highly sensitive luminescence sensing of alkylamines, CrystEngComm, 2019, 21, 694–702 RSC
.
- F.-L. Hu, F.-L. Jiang, J. Zheng, M.-Y. Wu, J.-D. Pang and M.-C. Hong, Magnetic properties of 3D heptanuclear lanthanide frameworks supported by mixed ligands, Inorg. Chem., 2015, 54, 6081–6083 CrossRef CAS PubMed
.
- D. Savard, P.-H. Lin, T. J. Burchell, I. Korobkov, W. Wernsdorfer, R. Clérac and M. Murugesu, Two-dimensional networks of lanthanide cubane-shaped dumbbells, Inorg. Chem., 2009, 48, 11748–11754 CrossRef CAS PubMed
.
- D.-D. Yin, Q. Chen, Y.-S. Meng, H.-L. Sun, Y.-Q. Zhang and S. Gao, Slow magnetic relaxation in a novel carboxylate/oxalate/hydroxyl bridged dysprosium layer, Chem. Sci., 2015, 6, 3095–3101 RSC
.
- Q. Zhou, F. Yang, B. Xin, G. Zeng, X. Zhou, K. Liu, D. Ma, G. Li, Z. Shi and S. Feng, Reversible switching of slow magnetic relaxation in a classic lanthanide metal–organic framework system, Chem. Commun., 2013, 49, 8244–8246 RSC
.
- X. Zhang, V. Vieru, X. Feng, J. L. Liu, Z. Zhang, B. Na, W. Shi, B. W. Wang, A. K. Powell and L. F. Chibotaru, Influence of Guest Exchange on the Magnetization Dynamics of Dilanthanide Single-Molecule-Magnet Nodes within a Metal–Organic Framework, Angew. Chem., Int. Ed., 2015, 54, 9861–9865 CrossRef CAS PubMed
.
- N. Motokawa, S. Matsunaga, S. Takaishi, H. Miyasaka, M. Yamashita and K. R. Dunbar, Reversible magnetism between an antiferromagnet and a ferromagnet related
to solvation/desolvation in a robust layered [Ru2]2TCNQ charge-transfer system, J. Am. Chem. Soc., 2010, 132, 11943–11951 CrossRef CAS PubMed
.
- X.-N. Cheng, W.-X. Zhang and X.-M. Chen, Single crystal-to-single crystal transformation from ferromagnetic discrete molecules to a spin-canting antiferromagnetic layer, J. Am. Chem. Soc., 2007, 129, 15738–15739 CrossRef CAS PubMed
.
- G. M. Espallargas and E. Coronado, Magnetic functionalities in MOFs: from the framework to the pore, Chem. Soc. Rev., 2018, 47, 533–557 RSC
.
- N. Ishikawa, M. Sugita and W. Wernsdorfer, Nuclear spin driven quantum tunneling of magnetization in a new lanthanide single-molecule magnet: bis (phthalocyaninato) holmium anion, J. Am. Chem. Soc., 2005, 127, 3650–3651 CrossRef CAS PubMed
.
- J.-J. Hu, Y. Peng, S.-J. Liu and H.-R. Wen, Recent advances in lanthanide coordination polymers and clusters with magnetocaloric effect or single-molecule magnet behavior, Dalton Trans., 2021, 50, 15473–15487 RSC
.
- M. Kurmoo, Magnetic metal–organic frameworks, Chem. Soc. Rev., 2009, 38, 1353–1379 RSC
.
- I.-R. Jeon and R. Clérac, Controlled association of single-molecule magnets (SMMs) into coordination networks: towards a new generation of magnetic materials, Dalton Trans., 2012, 41, 9569–9586 RSC
.
- A. E. Thorarinsdottir and T. D. Harris, Metal–organic framework magnets, Chem. Rev., 2020, 120, 8716–8789 CrossRef CAS PubMed
.
- H. L. Feltham and S. Brooker, Review of purely 4f and mixed-metal nd-4f single-molecule magnets containing only one lanthanide ion, Coord. Chem. Rev., 2014, 276, 1–33 CrossRef CAS
.
- A. Zabala-Lekuona, J. M. Seco and E. Colacio, Single-Molecule Magnets: From Mn12-ac to dysprosium metallocenes, a travel in time, Coord. Chem. Rev., 2021, 441, 213984 CrossRef CAS
.
- M. Perfetti, M. A. Sørensen, U. B. Hansen, H. Bamberger, S. Lenz, P. P. Hallmen, T. Fennell, G. G. Simeoni, A. Arauzo and J. Bartolomé, Magnetic anisotropy switch: Easy axis to easy plane conversion and vice versa, Adv. Funct. Mater., 2018, 28, 1801846 CrossRef
.
- J. M. Zadrozny, J. Liu, N. A. Piro, C. J. Chang, S. Hill and J. R. Long, Slow magnetic relaxation in a pseudotetrahedral cobalt(II) complex with easy-plane anisotropy, Chem. Commun., 2012, 48, 3927–3929 RSC
.
- J.-L. Liu, K. Yuan, J.-D. Leng, L. Ungur, W. Wernsdorfer, F.-S. Guo, L. F. Chibotaru and M.-L. Tong, A six-coordinate ytterbium complex exhibiting easy-plane anisotropy and field-induced single-ion magnet behavior, Inorg. Chem., 2012, 51, 8538–8544 CrossRef CAS PubMed
.
- J. D. Rinehart and J. R. Long, Exploiting single-ion anisotropy in the design of f-element single-molecule magnets, Chem. Sci., 2011, 2, 2078–2085 RSC
.
- L. Ungur, M. Thewissen, J.-P. Costes, W. Wernsdorfer and L. F. Chibotaru, Interplay of strongly anisotropic metal ions in magnetic blocking of complexes, Inorg. Chem., 2013, 52, 6328–6337 CrossRef CAS PubMed
.
- R.-P. Li, Q.-Y. Liu, Y.-L. Wang, C.-M. Liu and S.-J. Liu, Evolution from linear tetranuclear clusters into one-dimensional chains of Dy(III) single-molecule magnets with an enhanced energy barrier, Inorg. Chem. Front., 2017, 4, 1149–1156 RSC
.
- W.-H. Zhu, X. Xiong, C. Gao, S. Li, Y. Zhang, J. Wang, C. Zhang, A. K. Powell and S. Gao, A family of one-dimensional lanthanide complexes bridged by two distinct carboxylate ligands with the Dy analogue displaying magnetic relaxation behaviour, Dalton Trans., 2017, 46, 14114–14121 RSC
.
- Y. Li, P. Zhao, S. Zhang, R. Li, Y.-Q. Zhang, E.-C. Yang and X.-J. Zhao, A rare water and hydroxyl-extended one-dimensional Dysprosium(III) chain and its magnetic dilution effect, Inorg. Chem., 2017, 56, 9594–9601 CrossRef CAS PubMed
.
- J.-M. Li, R. Huo, X. Li and H.-L. Sun, Lanthanide–Organic Frameworks Constructed from 2, 7-Naphthalenedisulfonate and 1 H-Imidazo [4, 5-f][1, 10]-phenanthroline: Synthesis, Structure, and Luminescence with Near-Visible Light Excitation and Magnetic Properties, Inorg. Chem., 2019, 58, 9855–9865 CrossRef CAS PubMed
.
- D. Shao, J. Yang, X. Yang and Z. Tian, An Azido-Bridged Dysprosium Chain Complex Showing Zero-field Slow Magnetic Relaxation, Chem. – Asian J., 2021, 16, 3331–3335 CrossRef CAS PubMed
.
- T. Han, W. Shi, Z. Niu, B. Na and P. Cheng, Magnetic blocking from exchange interactions: Slow relaxation of the magnetization and hysteresis loop observed in a dysprosium–nitronyl nitroxide chain compound with an antiferromagnetic ground state, Chem. – Eur. J., 2013, 19, 994–1001 CrossRef CAS PubMed
.
- C.-M. Liu, D.-Q. Zhang, J.-B. Su, Y.-Q. Zhang and D.-B. Zhu, Single-molecule magnet behavior of 1D coordination polymers based on DyZn2(salen)2 units and Pyridin-N-Oxide-4-Carboxylate: structural divergence and magnetic regulation, Inorg. Chem., 2018, 57, 11077–11086 CrossRef CAS PubMed
.
- J. D. Rinehart, M. Fang, W. J. Evans and J. R. Long, Strong exchange and magnetic blocking in N23−-radical-bridged lanthanide complexes, Nat. Chem., 2011, 3, 538–542 CrossRef CAS PubMed
.
- E. M. Fatila, M. Rouzières, M. C. Jennings, A. J. Lough, R. Clérac and K. E. Preuss, Fine-tuning the single-molecule magnet properties of a [Dy(III)-radical]2 pair, J. Am. Chem. Soc., 2013, 135, 9596–9599 CrossRef CAS PubMed
.
- T. Lancaster, B. Huddart, R. Williams, F. Xiao, K. Franke, P. Baker, F. Pratt, S. Blundell, J. Schlueter and M. Mills, Probing magnetic order and disorder in the one-dimensional molecular spin chains CuF2(pyz) and [Ln(hfac)3(boaDTDA)]n (Ln=Sm, La) using implanted muons, J. Phys.: Condens. Matter, 2019, 31, 394002 CrossRef CAS PubMed
.
- E. M. Fatila, A. C. Maahs, M. B. Mills, M. Rouzières, D. V. Soldatov, R. Clérac and K. E. Preuss, Ferromagnetic ordering of –[Sm(III)-radical]n– coordination polymers, Chem. Commun., 2016, 52, 5414–5417 RSC
.
- J. González, P. Sevilla, G. Gabarró-Riera, J. Jover, J. Echeverría, S. Fuertes, A. Arauzo, E. Bartolomé and E. C. Sañudo, A Multifunctional Dysprosium-Carboxylato 2D Metall-Organic Framework, Angew. Chem., Int. Ed., 2021, 60, 12001–12006 CrossRef PubMed
.
- J.-W. Zhang, Y. Man, W.-H. Liu, B.-Q. Liu and Y.-P. Dong, A Dy2 dimer derived from a two-dimensional network with a high U eff value, Dalton Trans., 2019, 48, 2560–2563 RSC
.
- A. Mondal, S. Roy and S. Konar, Remarkable Energy Barrier for Magnetization Reversal in 3D and 2D Dysprosium-Chloranilate-Based Coordination Polymers, Chem. – Eur. J., 2020, 26, 8774–8783 CrossRef CAS PubMed
.
- J.-F. Chen, Y.-L. Ge, D.-H. Wu, H.-T. Cui, Z.-L. Mu, H.-P. Xiao, X. Li and J.-Y. Ge, Two-dimensional dysprosium(III) coordination polymer: Structure, single-molecule magnetic behavior, proton conduction, and luminescence, Front. Chem., 2022, 10, 1–8 Search PubMed
.
- Y.-S. Jin, K. Yu, N. Zhang, Y.-Q. Zhang, C.-M. Liu and H.-Z. Kou, From dinuclear to two-dimensional Dy (iii) complexes: single crystal–single crystal transformation and single-molecule magnetic behavior, J. Mater. Chem. C, 2023, 11, 1550–1559 RSC
.
- S. P. Bera, A. Mondal and S. Konar, Lanthanide-Based Layer-Type Two-Dimensional Coordination Polymers Featuring Slow Magnetic Relaxation, Magnetocaloric Effect and Proton Conductivity, Chem. – Asian J., 2019, 14, 3702–3711 CrossRef CAS PubMed
.
- C.-M. Liu, J. Xiong, D.-Q. Zhang, B.-W. Wang and D.-B. Zhu, Multiple thermal magnetic relaxation in a two-dimensional
ferromagnetic dysprosium(III) metal–organic framework, RSC Adv., 2015, 5, 104854–104861 RSC
.
- Q. Chen, J. Li, Y.-S. Meng, H.-L. Sun, Y.-Q. Zhang, J.-L. Sun and S. Gao, Tuning slow magnetic relaxation in a two-dimensional dysprosium layer compound through guest molecules, Inorg. Chem., 2016, 55, 7980–7987 CrossRef CAS PubMed
.
- S. Wang, R. Ma, Z. Chen, Y. Li, T. Cao, C. Zhou and J. Bai, Solvent-and metal-directed lanthanide-organic frameworks based on pamoic acid: observation of slow magnetization relaxation, magnetocaloric effect and luminescent sensing, Sci. China: Chem., 2016, 59, 948–958 CrossRef CAS
.
- H. Wu, S. Zhang, M. Li, C. Qiao, L. Sun, Q. Wei, G. Xie, S. Chen and S. Gao, Lanthanide–Organic Frameworks (LnOFs) Containing 1D Metal/Oxygen Ribbons with Cubane-like and Triangle Motifs: Synthesis, Structure, Luminescence and Slow Magnetic Relaxation, ChemistrySelect, 2016, 1, 3335–3342 CrossRef CAS
.
- K. Liu, H. Li, X. Zhang, W. Shi and P. Cheng, Constraining and tuning the coordination geometry of a lanthanide ion in metal–organic frameworks: approach toward a single-molecule magnet, Inorg. Chem., 2015, 54, 10224–10231 CrossRef CAS PubMed
.
- A. A. García-Valdivia, A. Zabala-Lekuona, A. Goñi-Cárdenas, B. Fernández, J. A. García, J. F. Q. del Moral, J. Cepeda and A. Rodríguez-Diéguez, Dilution effect on the slow relaxation of a luminescent dysprosium Metal-Organic Framework based on 2, 5-dihydroxyterephthalic acid, Inorg. Chim. Acta, 2020, 509, 119687 CrossRef
.
- X. J. Zhang, F. Z. Su, W. Y. Guo, E. C. Sañudo and C. S. Liu, An Unusual LnIII-Based Metal-Organic Framework with Dinuclear Nodes Exhibiting Single-Molecular Magnet Behavior, Eur. J. Inorg. Chem., 2018, 2018, 5007–5011 CAS
.
- S. Wang, T. Cao, H. Yan, Y. Li, J. Lu, R. Ma, D. Li, J. Dou and J. Bai, Functionalization of microporous lanthanide-based metal–organic frameworks by dicarboxylate ligands with methyl-substituted thieno [2,3-b] thiophene groups: sensing activities and magnetic properties, Inorg. Chem., 2016, 55, 5139–5151 CrossRef CAS PubMed
.
- S. Zhang, W. Shi, L. Li, E. Duan and P. Cheng, Lanthanide coordination polymers with “fsy-type” topology based on 4, 4′-azobenzoic acid: syntheses, crystal structures, and magnetic properties, Inorg. Chem., 2014, 53, 10340–10346 CrossRef CAS PubMed
.
- O. Pajuelo-Corral, J. A. García, O. Castillo, A. Luque, A. Rodríguez-Diéguez and J. Cepeda, Single-ion magnet and photoluminescence properties of lanthanide(III) coordination polymers based on pyrimidine-4, 6-dicarboxylate, Magnetochemistry, 2021, 7, 8 CrossRef CAS
.
- J.-J. Hu, Y.-G. Li, H.-R. Wen, S.-J. Liu, Y. Peng and C.-M. Liu, Stable Lanthanide Metal–Organic Frameworks with Ratiometric Fluorescence Sensing for Amino Acids and Tunable Proton Conduction and Magnetic Properties, Inorg. Chem., 2022, 61, 6819–6828 CrossRef CAS PubMed
.
- L. Zhang, S. Guan, Y. Fan, C. Du, D. Zhao and B. Liu, Towards solvent tuning of slow magnetic relaxation and ferroelectric properties in a dysprosium metal–organic framework system, Z. Kristallogr. - Cryst. Mater., 2019, 234, 33–41 CrossRef CAS
.
- C.-M. Liu, D.-Q. Zhang, Y.-S. Zhao, X. Hao and D.-B. Zhu, Two-step warming solvothermal syntheses, luminescence and slow magnetic relaxation of isostructural dense LnMOFs based on nanoscale 3-connected linkers, Inorg. Chem. Front., 2016, 3, 1076–1081 RSC
.
- F. Ma, J. Xiong, Y.-S. Meng, J. Yang, H.-L. Sun and S. Gao, Rational construction of a porous lanthanide coordination polymer featuring reversible guest-dependent magnetic relaxation behavior, Inorg. Chem. Front., 2018, 5, 2875–2884 RSC
.
- J. J. Baldoví, E. Coronado, A. Gaita-Ariño, C. Gamer, M. Giménez-Marqués and G. M. Espallargas, A SIM-MOF: Three-dimensional organisation of single-ion magnets with anion-exchange capabilities, Chem. – Eur. J., 2014, 20, 10695–10702 CrossRef PubMed
.
- J. Su, S. Yuan, J. Li, H. Y. Wang, J. Y. Ge, H. F. Drake, C. F. Leong, F. Yu, D. M. D'Alessandro and M. Kurmoo, Rare–Earth Metal Tetrathiafulvalene Carboxylate Frameworks as Redox-Switchable Single-Molecule Magnets, Chem. – Eur. J., 2021, 27, 622–627 CrossRef CAS PubMed
.
- T. Hu, Q. Zhao, L. Huo, L. Gao, J. Zhang, X. Wang and L. Fan, Two lanthanide metal–organic frameworks constructed from tris (4-carboxyphenyl) phosphane oxide with gas adsorption and magnetic properties, CrystEngComm, 2018, 20, 4291–4296 RSC
.
- Q. Zou, T. Shang, X.-D. Huang, Q.-Q. Guo, J.-G. Jia, S.-S. Bao, Y.-Q. Zhang and L.-M. Zheng, Dysprosium–dianthracene framework showing thermo-responsive magnetic and luminescence properties, J. Mater. Chem. C, 2021, 9, 10749–10758 RSC
.
- L. H. Kalinke, D. Cangussu, M. Mon, R. Bruno, E. Tiburcio, F. Lloret, D. Armentano, E. Pardo and J. Ferrando-Soria, Metal–Organic Frameworks as Playgrounds for Reticulate Single-Molecule Magnets, Inorg. Chem., 2019, 58, 14498–14506 CrossRef CAS PubMed
.
- Q. Wan, M. Arczyński, M. Wakizaka, S. Gupta, N. Funakoshi and M. Yamashita, Single-Ion Magnetism in a Three-Dimensional Thiocyanate-Bridged Dysprosium(III) Framework, Chemistry, 2023, 5, 987–995 CrossRef CAS
.
- S. S. Nagarkar, H. Kurasho, N. T. Duong, Y. Nishiyama, S. Kitagawa and S. Horike, Crystal melting and glass formation in copper thiocyanate based coordination polymers, Chem. Commun., 2019, 55, 5455–5458 RSC
.
- F. Jin, H.-P. Zhou, X.-C. Wang, Z.-J. Hu, J.-Y. Wu, Y.-P. Tian and M.-H. Jiang, Synthesis, structures and photoluminescence of thiocyanate bridged metal-organic polymers containing functional imidazole ligand, Polyhedron, 2007, 26, 1338–1346 CrossRef CAS
.
- M. Abedi, G. Mahmoudi, A. M. Kirillov and W. Kaminsky, Self-assembled 3D heterometallic Zn(II)/K(I) metal–organic framework with the fluorite topology, Polyhedron, 2018, 142, 110–114 CrossRef CAS
.
- S. E.-D. H. Etaiw, M. M. El-bendary, A. E.-A. S. Fouda and M. M. Maher, A new metal-organic framework based on cadmium thiocyanate and 6-methylequinoline as corrosion inhibitor for copper in 1M HCl solution, Prot. Met. Phys. Chem. Surf., 2017, 53, 937–949 CrossRef CAS
.
- Z.-L. Wu, A.-L. Gu, N. Gao, H.-Y. Cui, W.-M. Wang and J.-Z. Cui, Solvent-dependent assembly and magnetic relaxation behaviors of [Cu4I3] cluster-based lanthanide MOFs: Acting as efficient catalysts for carbon dioxide conversion with propargylic alcohols, Inorg. Chem., 2020, 59, 15111–15119 CrossRef CAS PubMed
.
- S. P. Bera, A. Mondal, S. Roy, B. Dey, A. Santra and S. Konar, 3D isomorphous lanthanide coordination polymers displaying magnetic refrigeration, slow magnetic relaxation and tunable proton conduction, Dalton Trans., 2018, 47, 15405–15415 RSC
.
- S. Biswas, J. Chakraborty, V. Singh Parmar, S. P. Bera, N. Ganguli and S. Konar, Channel-Assisted Proton Conduction Behavior in Hydroxyl-Rich Lanthanide-Based Magnetic Metal–Organic Frameworks, Inorg. Chem., 2017, 56, 4956–4965 CrossRef CAS PubMed
.
|
This journal is © the Partner Organisations 2023 |