DOI:
10.1039/D2QI00497F
(Research Article)
Inorg. Chem. Front., 2022,
9, 3771-3778
Interstitially O-doped CdxZn1−xS solid solution derived from chalcogenide molecular clusters for photocatalytic hydrogen evolution†
Received
5th March 2022
, Accepted 25th May 2022
First published on 25th May 2022
Abstract
Interstitial oxygen dopants (Oi) in sulfide nanocrystals are more conducive to charge carrier separation for improving the photocatalytic hydrogen evolution (PHE) performance than substituted oxygen dopants (OS). However, oxygen dopants exist dominantly in the form of OS, rather than Oi, in oxygen-doped sulfides prepared via traditional methods. Herein, an Oi-doped CdxZn1−xS solid solution was facilely synthesized through pyrolyzing a mixture of oxygen-containing zinc sulfide clusters and CdCl2 salts. At a controlled pyrolysis temperature, the oxygen components inside the cluster were successfully converted into Oi dopants associated with sulfur vacancies. The as-synthesized Oi-doped CdxZn1−xS solid solution exhibits good PHE performance due to its unique energy band structure caused by the two coexistent defects. The synthetic method of using the intrinsic components in molecular clusters to modulate the types of specific dopants in photocatalysts may provide a new synthetic strategy for creating doped photocatalysts with excellent PHE activities.
Introduction
Solar water splitting to generate hydrogen over photocatalysts is considered as a promising approach to address the energy crisis and environmental pollution issues.1–6 Various semiconducting materials, such as metal oxides,7–9 metal sulfides,10–16 and carbon nitrides,17,18 have been investigated as potential candidates for solar water splitting. Among them, metal sulfide solid solutions have attracted the attention of the academic community for a long time because their band gaps can be adjusted in a wide range.19,20 For example, CdxZn1−xS solid solution bears a tunable band gap in the range from 3.6 eV (ZnS) to 2.4 eV (CdS) and exhibits better photocatalytic activity than single ZnS or CdS.21 Unfortunately, the rapid electron–hole recombination, weak visible light absorption, and poor photocatalytic stability in CdxZn1−xS solid solution deteriorated its PHE activity. A simple and common method for solving the above problems is to modify CdxZn1−xS by doping with a heterogeneous element, which may narrow the band gap for more light absorption and insert impurity energy levels for better charge separation.22–25 For example, Chen's group doped ZnxCd1−xS solid solution with interstitial P element, and the S vacancies associated with the introduction of interstitial P prolonged the lifetime of charge carriers and enhanced the carrier separation efficiency.24 However, the P atoms with a larger radius relative to S atoms may cause larger lattice distortion of ZnxCd1−xS solid solution during the doping process and result in the formation of new electron–hole pair recombination centers, which in turn degrade the PHE performance to a certain extent. We believe that introducing interstitial oxygen doping elements with a small atomic radius into CdxZn1−xS solid solution is expected to reduce the carrier recombination probability caused by lattice distortion and simultaneously realize rapid photogenerated carrier separation and high PHE performance.
The common synthetic method for doping oxygen in metal sulfide nanocrystals is to add oxygen-containing raw materials during the preparation.26–28 For instance, Xie's group prepared oxygen-doped ZnIn2S4 photocatalysts by adding oxygen-containing polyvinyl pyrrolidone during the synthesis, wherein the type of oxygen dopant was substituted oxygen (Os) rather than interstitial oxygen (Oi).28 Unfortunately, such substituted oxygen dopants are inferior to interstitial oxygen dopants in promoting carrier separation since interstitial oxygen dopants are often associated with S vacancies. These two different types of defects can trap electrons and holes, respectively, which is better for improving the charge separation efficiency.24,29 Therefore, to improve the photocatalytic performance of metal sulfides, it is particularly necessary to seek synthetic methods for introducing interstitial oxygen dopants into metal sulfide nanocrystals.
Herein, we intentionally used well-defined oxygen-containing zinc sulfide molecular clusters (denoted as ZSP, Scheme 1a) as the precursor to prepare oxygen-doped metal sulfide semiconductors. The title sample of CdxZn1−xS solid solution (denoted as 500-Cd) with abundant S vacancies (VS) and interstitial oxygen (Oi) was synthesized by pyrolyzing a mixture of cluster-based crystals and CdCl2 salts at 500 °C under N2 (Schemes 1c and d). Comparative synthesis experiments showed that the temperature control and introduction of CdCl2 salts were key to the conversion of oxygen elements in metal sulfide molecular clusters into Oi (Schemes S1 and 2†). The prepared Oi-doped CdxZn1−xS photocatalyst (i.e., 500-Cd) exhibited an excellent PHE performance (1240 μmol h−1 g−1), which is 29.5 and 3.3 times higher than that of ZSP (41 μmol h−1 g−1) and CdxZn1−xS solid solution without Oi dopants (denoted as 400-Cd, 380 μmol h−1 g−1) in the presence of a sacrificial agent. This is because Oi and Vs introduce new intermediate energy levels, which position near the valence band and between the conduction band and the Fermi level, respectively. Such a band structure may effectively and separately trap holes and electrons to improve the separation efficiency of photogenerated carriers, thereby improving the PHE performance.
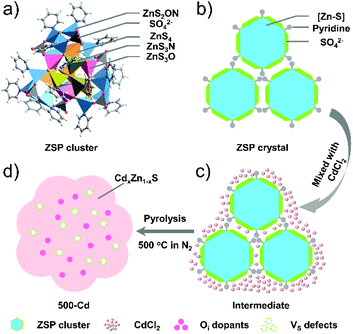 |
| Scheme 1 Illustration of the fabrication process of Oi-doped CdxZn1−xS solid solution. (a) Polyhedral model of the [Zn–S–O] molecular cluster. (b) Simplified structure of the ZSP-packed crystal. (c) Simplified model of the mixture of ZSP-packed crystals and CdCl2 salt. (d) Oi-doped CdxZn1−xS solid solution with the coexistence of Vs defects. | |
Results and discussion
Morphology and structure characterization
The ZSP cluster-based microcrystals were prepared according to a previously reported procedure,30 and their phase purity was also confirmed through powder XRD measurements (Fig. S1†). The ZSP cluster is a structurally well-defined oxygen-containing zinc sulfide molecular cluster with a formula [(Zn25S19)(SO4)6(Py)18], which is constructed by a [Zn–S] inner core terminally capped by six sulfate groups and eighteen pyridine ligands. In each ZSP cluster, zinc ions have four types of coordination modes: ZnS4, ZnS3N, ZnS3O, and ZnS2NO (Scheme 1a). For convenience, the molecular cluster may be simplified as an easily understood structure motif, i.e., the [Zn–S] inner core is wrapped around by sulfate groups and pyridine ligands in the outer layer (Scheme 1b). Such a unique structure was anticipated to be a useful template for constructing an O-doped chalcogenide semiconductor.
To synthesize the CdxZn1−xS solid solution, a mixture of ZSP cluster-based microcrystals and CdCl2 powder was pyrolyzed at different temperatures (400 °C, 500 °C, and 600 °C) in N2 for 2 hours (Scheme 1c). The obtained samples are denoted as 400-Cd, 500-Cd, and 600-Cd, respectively (Scheme 1 and S1†). Their structure, composition, and morphology were revealed by TEM and HRTEM images. It was suggested that 400-Cd exists in the form of nanoparticles (NPs) (Scheme S1a† and Fig. 1a), and is identified as CdxZn1−xS solid solution due to the clear lattice fringes of 0.33 nm corresponding to the (002) lattice plane of CdxZn1−xS (Fig. 1d).23,31 When the heating temperature was elevated to 500 °C, 500-Cd exhibited a large sheet morphology with a few grain boundaries (Fig. 1b), which is also identified as CdxZn1−xS solid solution (Fig. 1e). The elements Cd, Zn, and S were found to be evenly distributed in the solid solution of CdxZn1−xS (Fig. S3†). Also, a small amount of oxygen element was present in the CdxZn1−xS solid solution. The morphological difference between 400-Cd and 500-Cd may be ascribed to CdCl2.9,10 In the mixture of ZSP-based microcrystals and CdCl2 salts, CdCl2 wraps around ZSP microcrystals. When the pyrolysis temperature is set at 400 °C, partial CdCl2 salts may diffuse into ZSP microcrystals, and granular CdxZn1−xS NPs were gradually formed. Meanwhile, the unreacted CdCl2 salts adsorbed on the surface of NPs may block their further aggregation because CdCl2 is not molten under 400 °C. However, when the pyrolysis temperature is increased above 500 °C, the molten CdCl2 salts combine with the CdxZn1−xS NPs, thereby leading to the sheet morphology of 500-Cd and 600-Cd (Fig. 1c). Furthermore, the ZnO phase (PDF#36-1451) is observed in 600-Cd according to the clear lattice fringes of 0.28 nm corresponding to the (100) lattice planes in the HRTEM image (Fig. 1f). These results are consistent with the XRD results (Fig. S2†). Obviously, the oxygen element in ZnO stemmed from the decomposition of SO42− anions in ZSP microcrystals. Also, due to the formation of molten CdCl2 salts in 500-Cd and 600-Cd, the oxygen components inside the ZSP molecular clusters were retained during the pyrolysis of the intermediate mixture to form CdxZn1−xS solid solution.
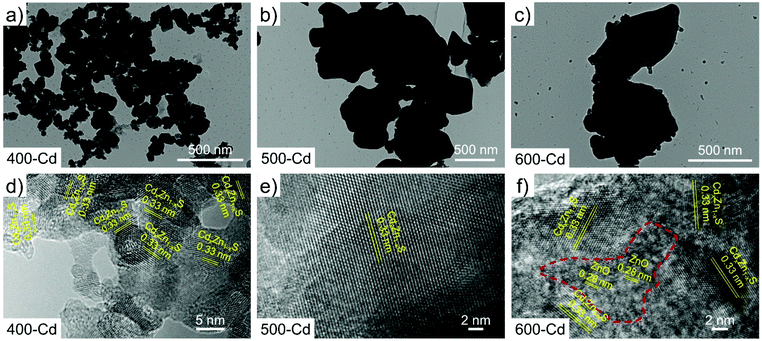 |
| Fig. 1 TEM images of (a) 400-Cd, (b) 500-Cd, and (c) 600-Cd. HRTEM images of (d) 400-Cd, (e) 500-Cd, and (f) 600-Cd. | |
For comparison, pyrolyzed products by directly annealing pure ZSP microcrystals at different temperatures (400 °C, 500 °C, and 600 °C) in N2 were also obtained (Scheme S2†), which are denoted as 400-D, 500-D, and 600-D, respectively. The morphologies of 400-D, 500-D, and 600-D are characterized using TEM and HRTEM images (Fig. S4†). It was clearly observed that 400-D and 500-D were prepared in the form of NPs, and the size of NPs in 400-D is smaller than that of NPs in 500-D (Fig. S4a and b†). The NPs in 600-D present signs of merging (Fig. S4c†). This is caused by the absence of CdCl2 salts. The NPs in 400-D and 500-D are characterized as the ZnS phase because the clear lattice fringes of 0.31 nm corresponding to the (006) lattice planes of ZnS (PDF#89-2739) are observed in the HETEM images (Fig. S4d and e†). These results were consistent with the XRD results (Fig. S5†). In addition, the sample of 600-D contains a partial ZnO phase because the lattice fringes of 0.28 nm corresponding to the (100) lattice planes of ZnO (PDF#36-1451) are observed in the HRTEM images (Fig. S4f†). This phenomenon is similar to that observed in 600-Cd. Considering that the oxygen species are not found in the samples of 400-D, 500-D, 400-Cd and 500-Cd, we can't help but wonder where the oxygen components are at low temperatures (400 °C and 500 °C).
To determine the existing form of the oxygen species in the pyrolyzed samples, X-ray photoelectron spectroscopy (XPS) was conducted on all of the above samples. The XPS scan survey spectra of 400-Cd, 500-Cd, and 600-Cd confirm the presence of Zn, O, Cd, C, and S elements in the as-synthesized samples (Fig. S6†). Fig. S7† shows the high-resolution XPS spectra of C 1s, Cd 3d, Zn 2p, and N 1s. It is clearly observed that the peaks of Cd 3d in 400-Cd, 500-Cd, and 600-Cd shift slightly to lower binding energy (Fig. S7b†). However, the Zn 2p XPS peaks shift first to a lower binding energy, then to a higher binding energy with increasing pyrolysis temperature (Fig. S7c†). This may be attributed to the formation of ZnO at high pyrolysis temperature. Meanwhile, there are no N 1s XPS peaks observed in all samples (Fig. S7d†), suggesting that pyridine molecules are not retained in CdxZn1−xS solid solution. In addition, the high-resolution XPS spectra of S 2p and O 1s are also characterized to determine the existing form of O species (Fig. 2a and b). There are two kinds of S 2p XPS peaks, corresponding to SO42− and Zn–S bonds, and the weak XPS signals of SO42− observed in all samples suggest that SO42−species are easily pyrolyzed in the presence of CdCl2. As shown in Fig. 2b, 400-Cd exhibits three O 1s XPS peaks at 532.83, 531.99, and 531.29 eV, which are assigned to water, hydroxyl groups, and lattice oxygen, respectively.26,28 This might be because SO42− anions are not pyrolyzed completely under 400 °C. However, a new XPS peak at 530.29 eV is observed in 500-Cd. We conjecture that this peak is attributed to Oi, i.e. the O anions are doped into the interstitial lattice of CdxZn1−xS solid solution. To test our guess, electron spin resonance (ESR) measurements were employed to further confirm the existence of interstitial oxygen dopants in all samples. As shown in Fig. 2c, pristine ZSP microcrystals have no ESR signal. However, 400-Cd shows a clear ESR signal at 327 mT (g-value of 2.001), and 500-Cd exhibits two ESR signals at 312 mT (g-value of 2.096) and 335 mT (g-value of 1.953), respectively. By contrast, a large number of other ESR signals are observed in 600-Cd. According to the literature,32,33 the EPR signal at g = 2.096 was ascribed to S vacancies. Similarly, we think that the EPR signal at g = 1.953 is attributed to interstitial O (Oi) dopants in 500-Cd.34,35 For further comparison, the existing type of oxygen species in 400-D, 500-D, and 600-D was also analyzed by XPS and ESR measurements (Fig. 2d–f and S8†). Clearly, the high-resolution Zn 2p XPS peaks show the same shift trend as that observed in 400-Cd, 500-Cd, and 600-Cd. According to the N 1s XPS peaks (Fig. S8d†), pyridine molecules are thought to be decomposed, and nitrogen atoms are doped into ZnS (Scheme S1†). SO42− anions in pristine ZSP microcrystals are thought to be gradually pyrolyzed into S and O species with the increasing temperature (Fig. 2d). Also, three O 1s XPS peaks in 400-D are assigned to water, hydroxy, and lattice oxygen, respectively (Fig. 2e). In combination with the ESR results (Fig. 2f and Fig. S9†), the new O 1s XPS signal appearing in 500-D and 600-D is assigned to the substituted oxygen (Os). This is because the EPR signal at g = 2.001 in 500-D was significantly different from that in 500-Cd, and, if the interstitial oxygen dopant cannot be formed in 500-D, the oxygen element located in ZnS can only replace the S element to form OS dopants. Therefore, it was concluded that oxygen species exist in the form of Oi in 500-Cd and 600-Cd, along with the formation of Vs, however, in the form of Os in 500-D and 600-D. Therefore, in the presence of CdCl2 salts, the oxygen components in ZSP are easily converted into interstitial oxygen dopants rather than substituted oxygen dopants.
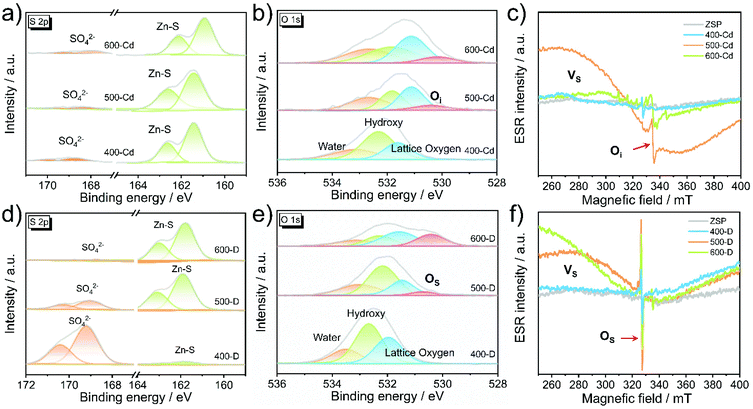 |
| Fig. 2 High-resolution XPS signals of S 2p (a) and O 1s (b) in 400-Cd, 500-Cd, and 600-Cd. (c) ESR signals of ZSP microcrystals, 400-Cd, 500-Cd, and 600-Cd. High-resolution XPS signals of S 2p (d) and O 1s (e) in 400-D, 500-D, and 600-D. (f) ESR signals of ZSP microcrystals, 400-D, 500-D, and 600-D. | |
Photocatalytic hydrogen evolution performance
PHE experiments were performed under visible-light irradiation using triethanolamine (TEOA) as a hole scavenger. As shown in Fig. S10,† the pristine ZSP microcrystals exhibit a very low PHE activity with a rate of 42 μmol g−1 h−1, indicating that ZSP is less active for hydrogen evolution. As expected, 500-Cd delivers a high PHE rate of 1.24 mmol g−1 h−1, which is 29.5 times higher than that of pristine ZSP (Fig. 3a, S10, and S14†). This is ascribed to the formation of the Oi-doped CdxZn1−xS solid solution. In addition, 500-Cd still exhibits a stable photoactivity after 10 consecutive cycles for a total of 50 h (Fig. S11†). The XRD patterns and TEM images of the cycled samples further confirm the PHE stability (Fig. S12 and S13†). To explain why 500-Cd has higher photocatalytic activity than other samples, photocurrent response experiments were explored. As shown in Fig. 3b, 500-Cd displays the highest photocurrent density, suggesting the fastest photo-generated carrier separation among all samples. Also, according to the results of photoluminescence (PL) and electrochemical impedance spectroscopy (EIS), 500-Cd exhibits the fastest carrier separation efficiency (Fig. 3c and d). For comparison, the PHE performance of 400-D, 500-D, and 600-D samples was also investigated (Fig. S14†). 500-D delivers a PHE rate of 0.71 mmol g−1 h−1, which is lower than that of 500-Cd. Also, the results of the photocurrent response, EIS, and PL reveal that the carrier separation efficiency in 500-D is higher than that in both 400-D and 600-D (Fig. S15–S17†), but lower than that in 500-Cd. This could be explained by the different types of oxygen dopants.
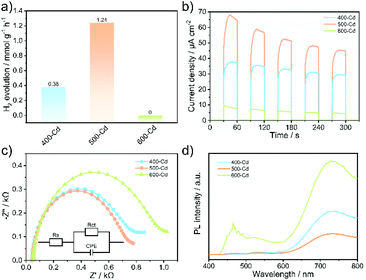 |
| Fig. 3 (a) The PHE rate, (b) photocurrent response, (c) EIS plots, and (d) PL spectra of 400-Cd, 500-Cd, and 600-Cd. | |
Band structure and photocatalytic mechanism
To understand the PHE mechanism, the energy band structures of photocatalysts are analyzed. As discussed above, the major component in 400-Cd is CdxZn1−xS solid solution without any oxygen dopants, 500-Cd consists of CdxZn1−xS with Oi dopants and Vs defects, and 600-Cd is the mixture of ZnO and CdxZn1−xS with Oi and Vs defects. The band gap (Eg) of 400-Cd (2.58 eV) was estimated using the Kubelka–Munk function:11 (ahν)2 = A(hν − Eg) (Fig. S18 and 19†). The valence band (VB) is determined based on the Mulliken electronegativity theory: EVB = χ − E0 + 0.5Eg, where EVB is the VB edge potential. Moreover, E0 is the energy of free electrons vs. NHE (4.5 eV). Finally, χ is the electronegativity of the semiconductor and is calculated using the following equation:36χ = [χ(A)aχ(B)bχ(C)c]1/(a+b+c), where a, b, and c are the number of atoms in the compounds. The value of χ is calculated to be 5.25 eV. Therefore, ECB and EVB of 400-Cd are calculated to be −0.54 and 2.04 eV (vs. NHE), respectively. Next, the XPS VB spectra of 400-Cd are analyzed to determine the Fermi level (Fig. S21†).37 Based on the value of the XPS VB plot (2.24 eV), the Fermi level of 400-Cd is determined to be −0.23 eV (vs. NHE), which is close to −0.25 eV using the M–S profile (Fig. S20†). The band energy structure diagram of 400-Cd is shown in Fig. 4a. Similarly, the band gap of 500-Cd is determined to be 2.43 eV. Also, the ECB and EVB of 500-Cd are calculated to be −0.47 and 1.96 eV (vs. NHE), respectively. Using the M–S plot in Fig. S20,† the Fermi level is determined to be −0.13 eV (vs. NHE).11,38 It is worth noting that the difference between EVB and Ef is 2.06 eV, while the value of the XPS VB plot of 500-Cd is 1.65 eV (Fig. S22†). This suggests that there is an intermediate level at 1.52 eV between Ef and EVB, which may be ascribed to the energy level of Oi. Meanwhile, on the basis of previous references, the shallow trap state of Vs locates between the conduction band and the Fermi level at −0.25 eV.23,29,39 Thus, the band energy structure diagram of 500-Cd is shown in Fig. 4b. This unique energy structure can not only improve the light absorption (Fig. S18†), but also accelerate the photoinduced carrier separation (Fig. 3b–d). This is because the coexistence of Oi and Vs may narrow the band gap to a certain extent. In addition, Oi and Vs are able to separately trap holes and electrons, resulting in fast carrier separation and preventing the recombination of electron–hole pairs. The band structure of 600-Cd is shown in Fig. 4c. The photoexcited electrons in CdxZn1−xS solid solution could be transferred to ZnO because ZnO has a more positive conduction band position and more negative Fermi level than CdxZn1−xS solid solution, but ZnO locates inside the whole sample (Fig. 1c and f); because H2O molecules cannot reach the surface of ZnO for the reduction reaction, there is no PHE rate in 600-Cd (Fig. 3a).
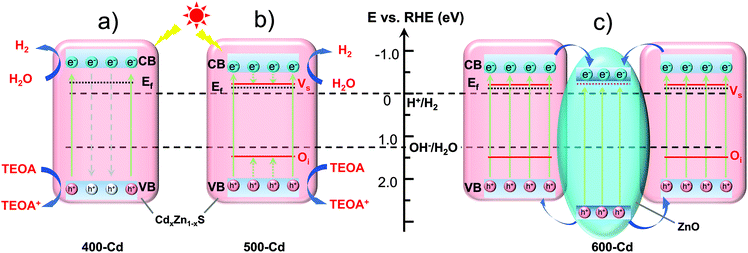 |
| Fig. 4 The diagrams of the energy band structure of 400-Cd (a), 500-Cd (b), and 600-Cd (c). | |
Conclusions
In summary, the Oi-doped CdxZn1−xS solid solution (500-Cd) with rich S vacancies was fabricated by pyrolyzing a mixture of oxygen-containing chalcogenide microcrystals and CdCl2 salt. The oxygen components in the ZSP cluster were proved to be successfully converted into interstitial oxygen dopants in CdxZn1−xS solid solution by controlling the temperature at 500 °C and adding CdCl2 salts. Notably, 500-Cd gives a PHE rate of 1.24 mmol h−1 g−1, which is 3.3 times higher than that (0.38 mmol h−1 g−1) of 400-Cd without Oi dopants. Also, the PHE rate of 500-Cd is 1.75 times higher than that (0.71 mmol h−1 g−1) of 500-D without Oi dopants. The enhanced photocatalytic activity is attributed to the rapid separation of photogenerated electron–hole pairs because the coexistence of Oi dopants and Vs defects allows for trapping holes and electrons separately and simultaneously. Meanwhile, the interstitially O-doped CdxZn1−xS solid solution has greater stability than the undoped one during the PHE process. The adopted synthetic method for preparing the CdxZn1−xS solid solution with Oi dopants may be a useful synthetic strategy for the creation of specific dopants into photocatalysts for boosting the PHE performance.
Experimental
Materials and chemicals
Zinc acetate dihydrate (Zn, 99%, powder), sulfur (S, 99.9%, powder), cadmium chloride (CdCl2, 99%, powder), pyridine (Py, >99%, liquid), 3,5-dimethylpyridine (3,5-DMPy, >99%, liquid), N,N-dimethylformamide (DMF >99%, liquid), isopropyl alcohol (IPA, >99%, liquid), ethanol (>99%, liquid), and deionized water (H2O, 18.5 MΩ cm) were used as received without further purification.
Synthesis of ZSP30
Zinc acetate dihydrate (60 mg), sulfur (30 mg), pyridine (1.5 mL), and DMF (0.5 mL) were added into a 5 mL glass tube. The vessel was sealed, shaken at room temperature, and then heated at 120 °C for 24 h. The tube was subsequently cooled to room temperature, and then colorless regular rhombic prism crystals were obtained with the yield of 35.0 mg (76% based on the Zn source).
Synthesis of 400-D, 500-D, and 600-D
A group of samples were synthesized by pyrolyzing the as-synthesized ZSP-based microcrystals in a tube furnace for 2 h under N2 at 400 °C, 500 °C, and 600 °C, respectively. The tube furnace was subsequently cooled to room temperature, and the obtained powders were labeled as 400-D, 500-D, and 600-D, respectively.
Synthesis of 400-Cd, 500-Cd, and 600-Cd
100 mg of ZSP-based microcrystals and 100 mg of CdCl2 powder were uniformly mixed together. Then, the mixture was heated in a tube furnace for 2 h under a N2 atmosphere at 400 °C, 500 °C, and 600 °C, respectively. After cooling to room temperature, the products were collected, washed several times with H2O and ethanol, and dried at 60 °C under vacuum. The obtained powders were labeled as 400-Cd, 500-Cd, and 600-Cd, respectively, based on different pyrolysis temperatures.
Author contributions
Weijie Yang: conceptualization, methodology, investigation, resources, data curation, formal analysis, visualization, and writing – original draft preparation. Xiang Wang: HRTEM characterization of materials, and resources. Zhiqiang Wang: PL characterization of materials and resources. Peipei Sun: UV-vis DRS characterization of materials and resources. Jiaqi Tang: investigation and resources. Juan Li: UV-vis DRS characterization of materials and resources. Dong-Sheng Li: resources, supervision and funding acquisition. Tao Wu: resources, writing – reviewing & editing, supervision, project administration and funding acquisition.
Conflicts of interest
There are no conflicts to declare.
Acknowledgements
We acknowledge the financial support from the National Natural Science Foundation of China (No. 21875150 and 22071165) and the 111 Project (D20015).
Notes and references
- X. Chen, S. Shen, L. Guo and S. S. Mao, Semiconductor-based photocatalytic hydrogen generation, Chem. Rev., 2010, 110, 6503–6570 CrossRef CAS PubMed.
- X. Li, J. Yu, J. Low, Y. Fang, J. Xiao and X. Chen, Engineering heterogeneous semiconductors for solar water splitting, J. Mater. Chem. A, 2015, 3, 2485–2534 RSC.
- S. Fang and Y. H. Hu, Recent progress in photocatalysts for overall water splitting, Int. J. Energy Res., 2019, 43, 1082–1098 CrossRef.
- Y. Fang, Y. Zheng, T. Fang, Y. Chen, Y. Zhu, Q. Liang, H. Sheng, Z. Li, C. Chen and X. Wang, Photocatalysis: an overview of recent developments and technological advancements, Sci. China: Chem., 2019, 63, 149–181 CrossRef.
- Z. Wang, C. Li and K. Domen, Recent developments in heterogeneous photocatalysts for solar-driven overall water splitting, Chem. Soc. Rev., 2019, 48, 2109–2125 RSC.
- Y. Zhao, S. Zhang, R. Shi, G. I. N. Waterhouse, J. Tang and T. Zhang, Two-dimensional photocatalyst design: A critical review of recent experimental and computational advances, Mater. Today, 2020, 34, 78–91 CrossRef CAS.
- L. Sun, Y. Zhuang, Y. Yuan, W. Zhan, X. J. Wang, X. Han and Y. Zhao, Nitrogen-Doped Carbon-Coated CuO-In2O3 p-n Heterojunction for Remarkable Photocatalytic Hydrogen Evolution, Adv. Energy Mater., 2019, 9, 1902839 CrossRef CAS.
- C. Zhou, Z. Sanders-Bellis, T. J. Smart, W. Zhang, L. Zhang, Y. Ping and M. Liu, Interstitial Lithium Doping in BiVO4 Thin Film Photoanode for Enhanced Solar Water Splitting Activity, Chem. Mater., 2020, 32(15), 6401–6409 CrossRef CAS.
- W. Yang, W. Wu, W. Chen, J. Zhao and X. Hu, Structural modulation of CdS/ZnO nanoheterojunction arrays for full solar water splitting and their related degradation mechanisms, Catal. Sci. Technol., 2018, 8, 5280–5287 RSC.
- W. Chen, W. Wu, W. Yang, J. Zhao, M. Xiao and W. Kong, CdCl2-assisting heat-treatment: Enhanced photoelectrocatalytic hydrogen generation and stability of CdS/ZnO nanoheterojunction arrays, Int. J. Hydrogen Energy, 2018, 43, 9969–9977 CrossRef CAS.
- W. Yang, X.-L. Wang, N. Kong, C. Liu, P. Sun, Z. Wang, Y. Ding, H. Lin, D. Li and T. Wu, Minimized external electric field on asymmetric monolayer maximizes charge separation for photocatalysis, Appl. Catal., B, 2021, 295, 120266 CrossRef CAS.
- D. Liu, Y. Liu, P. Huang, C. Zhu, Z. Kang, J. Shu, M. Chen, X. Zhu, J. Guo and L. Zhuge,
et al., Highly tunable heterojunctions from multimetallic sulfide nanoparticles and silver nanowires, Angew. Chem., Int. Ed., 2018, 57, 5374–5378 CrossRef CAS PubMed.
- Q. Li, X.-Q. Qiao, Y. Jia, D. Hou and D.-S. Li, Amorphous CoMoS4 Nanostructure for Photocatalytic H2 Generation, Nitrophenol Reduction, and Methylene Blue Adsorption, ACS Appl. Nano Mater., 2020, 3, 68–76 CAS.
- Q. Li, X.-Q. Qiao, Y. Jia, D. Hou and D.-S. Li, Noble-metal-free amorphous CoMoSx modified CdS core-shell nanowires for dramatically enhanced photocatalytic hydrogen evolution under visible light irradiation, Appl. Surf. Sci., 2019, 498, 143863 CrossRef CAS.
- L. Shang, B. Tong, H. Yu, G. I. N. Waterhouse, C. Zhou, Y. Zhao, M. Tahir, L.-Z. Wu, C.-H. Tung and T. Zhang, CdS Nanoparticle-Decorated Cd Nanosheets for Efficient Visible Light-Driven Photocatalytic Hydrogen Evolution, Adv. Energy Mater., 2016, 6, 1501241 CrossRef.
- Y. Zhu, X. Jiang, L. Lin, S. Wang and C. Chen, Fabrication of ZnS/CdS Heterojunction by Using Bimetallic MOFs Template for Photocatalytic Hydrogen Generation, Chem. Res. Chin. Univ., 2020, 36, 1032–1038 CrossRef CAS.
- H. Luo, Y. Liu, S. D. Dimitrov, L. Steier, S. Guo, X. Li, J. Feng, F. Xie, Y. Fang and A. Sapelkin,
et al., Pt single-atoms supported on nitrogen-doped carbon dots for highly efficient photocatalytic hydrogen generation, J. Mater. Chem. A, 2020, 8, 14690–14696 RSC.
- G. Li, Z. Xie, S. Chai, X. Chen and X. Wang, A facile one-step fabrication of holey carbon nitride nanosheets for visible-light-driven hydrogen evolution, Appl. Catal., B, 2021, 283, 119637 CrossRef CAS.
- D. Huang, M. Wen, C. Zhou, Z. Li, M. Cheng, S. Chen, W. Xue, L. Lei, Y. Yang and W. Xiong,
et al., ZnxCd1−xS based materials for photocatalytic hydrogen evolution, pollutants degradation and carbon dioxide reduction, Appl. Catal. B: Environ., 2020, 267, 118651 CrossRef CAS.
- C. Zeng, H. Huang, T. Zhang, F. Dong, Y. Zhang and Y. Hu, Fabrication of Heterogeneous-Phase Solid-Solution Promoting Band Structure and Charge Separation for Enhancing Photocatalytic CO2 Reduction: A Case of ZnXCa1−XIn2S4, ACS Appl. Mater. Interfaces, 2017, 9, 27773–27783 CrossRef CAS PubMed.
- Q. Li, H. Meng, P. Zhou, Y. Zheng, J. Wang, J. Yu and J. Gong, Zn1−xCdxS solid solutions with controlled bandgap and enhanced visible-light photocatalytic H2-production activity, ACS Catal., 2013, 3, 882–889 CrossRef CAS.
- Y. Wang, J. Wu, J. Zheng, R. Jiang and R. Xu, Ni2+-doped ZnxCd1−xS photocatalysts from single-source precursors for efficient solar hydrogen production under visible light irradiation, Catal. Sci. Technol., 2012, 2, 581–588 RSC.
- X. Tang, J. H. Zhao, Y. H. Li, Z. J. Zhou, K. Li, F. T. Liu and Y. Q. Lan, Co-doped Zn1−xCdxS nanocrystals from metal-organic framework precursors: porous microstructure and efficient photocatalytic hydrogen evolution, Dalton Trans., 2017, 46, 10553–10557 RSC.
- H.-F. Ye, R. Shi, X. Yang, W.-F. Fu and Y. Chen, P-doped ZnxCd1−xS solid solutions as photocatalysts for hydrogen evolution from water splitting coupled with photocatalytic oxidation of 5-hydroxymethylfurfural, Appl. Catal., B, 2018, 33, 70–79 CrossRef.
- K. Khan, X. Tao, M. Shi, B. Zeng, Z. Feng, C. Li and R. Li, Visible-light-driven photocatalytic hydrogen production on Cd0.5Zn0.5S nanorods with an apparent quantum efficiency exceeding 80%, Adv. Funct. Mater., 2020, 30, 2003731 CrossRef CAS.
- B. Pan, Y. Wu, B. Rhimi, J. Qin, Y. Huang, M. Yuan and C. Wang, Oxygen-doping of ZnIn2S4 nanosheets towards boosted photocatalytic CO2 reduction, J. Energy Chem., 2021, 57, 1–9 CrossRef.
- X. Yang, Y. Guo, Y. Lou and J. Chen, O-MoS2/Mn0.5Cd0.5S composites with enhanced activity for visible-light-driven photocatalytic hydrogen evolution, Catal. Sci. Technol., 2020, 10, 5298–5305 RSC.
- W. Yang, L. Zhang, J. Xie, X. Zhang, Q. Liu, T. Yao, S. Wei, Q. Zhang and Y. Xie, Enhanced photoexcited carrier separation in oxygen-doped ZnIn2S4 nanosheets for hydrogen evolution, Angew. Chem., Int. Ed., 2016, 55, 6716–6720 CrossRef CAS PubMed.
- R. Shi, H. F. Ye, F. Liang, Z. Wang, K. Li, Y. Weng, Z. Lin, W. F. Fu, C. M. Che and Y. Chen, Interstitial P-doped CdS with long-lived photogenerated electrons for photocatalytic water splitting without sacrificial agents, Adv. Mater., 2018, 30, 1705941 CrossRef PubMed.
- C. Xue, J. Zhang, X. Wang, M. Gu, Y. Zhu, D. S. Li, J. Guo, Y. Liu and T. Wu, Light-triggered evolution of molecular clusters toward sub-nanoscale heterojunctions with high interface density, Chem. Commun., 2019, 55, 8146–8149 RSC.
- F.-Y. Tian, D. Hou, F. Tang, M. Deng, X.-q. Qiao, Q. Zhang, T. Wu and D.-S. Li, Novel Zn0.8Cd0.2S@g-C3N4 core–shell heterojunctions with a twin structure for enhanced visible-light-driven photocatalytic hydrogen generation, J. Mater. Chem. A, 2018, 6, 17086–17094 RSC.
- S. Zhang, X. Liu, C. Liu, S. Luo, L. Wang, T. Cai, Y. Zeng, J. Yuan, W. Dong and Y. Pei,
et al., MoS2 quantum dot growth induced by S vacancies in a ZnIn2S4 monolayer: Atomic-level heterostructure for photocatalytic hydrogen production, ACS Nano, 2018, 12, 751–758 CrossRef CAS PubMed.
- C. Du, Q. Zhang, Z. Lin, B. Yan, C. Xia and G. Yang, Half-unit-cell ZnIn2S4 monolayer with sulfur vacancies for photocatalytic hydrogen evolution, Appl. Catal., B, 2019, 248, 193–201 CrossRef CAS.
- P. Gu, X. Wang, T. Li and H. Meng, Investigation of defects in N-doped ZnO powders prepared by a facile solvothermal method and their UV photocatalytic properties, Mater. Res. Bull., 2019, 48, 4699–4703 CrossRef.
- X.-H. Zhang, N. Li, J. Wu, Y.-Z. Zheng and X. Tao, Defect-rich O-incorporated 1T-MoS2 nanosheets for remarkably enhanced visible-light photocatalytic H2 evolution over CdS: The impact of enriched defects, Appl. Catal., B, 2018, 229, 227–236 CrossRef CAS.
- M. Mousavi, A. Habibi-Yangjeh and M. Abitorabi, Fabrication of novel magnetically separable nanocomposites using graphitic carbon nitride, silver phosphate and silver chloride and their applications in photocatalytic removal of different pollutants using visible-light irradiation, J. Colloid Interface Sci., 2016, 480, 218–231 CrossRef CAS PubMed.
- L. Zhang, J. Ran, S. Z. Qiao and M. Jaroniec, Characterization of semiconductor photocatalysts, Chem. Soc. Rev., 2019, 48, 5184–5206 RSC.
- J. Lin, Y. Dong, Q. Zhang, D. Hu, N. Li, L. Wang, Y. Liu and T. Wu, Interrupted chalcogenide-based zeolite-analogue semiconductor: atomically precise doping for tunable electro-/photoelectrochemical properties, Angew. Chem., Int. Ed., 2015, 54, 5103–5107 CrossRef CAS PubMed.
- X. Xu, Y. Zhao, E. J. Sie, Y. Lu, B. Liu, S. A. Ekahana, X. Ju, Q. Jiang, J. Wang and H. Sun, Dynamics of bound exciton complexes in CdS nanobelts, ACS Nano, 2011, 5, 3660–3669 CrossRef CAS PubMed.
|
This journal is © the Partner Organisations 2022 |