DOI:
10.1039/D0MH01293A
(Review Article)
Mater. Horiz., 2021,
8, 124-144
Bio-inspired wettability patterns for biomedical applications
Received
10th August 2020
, Accepted 10th September 2020
First published on 10th September 2020
Abstract
Benefiting from the remarkable wettability heterogeneity, bio-inspired wettability patterns present a progressive and versatile platform for manipulating and patterning liquids, which provides an emerging strategy for operating liquid samples with crucial values in biomedical applications. In this review, we present a general summary of bio-inspired wettability patterns. After a compendious introduction of natural wettability phenomena and their underlying mechanisms, we summarize the general design principles and fabrication methods for preparing artificial wettability materials. Next, we shift to patterned surface wettability with an emphasis on the fabrication approaches. Then, we discuss in detail the various practical applications of wettability patterns in the biomedical field, including cell culture, drug screening and biosensors. Critical thinking about the current challenges and future outlook is also provided. We believe that this review would propel the prosperous development of bio-inspired wettability patterns to flourish in the field of biomedical engineering.
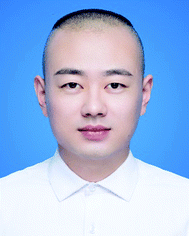
Junjie Chi
| Junjie Chi received his PhD degree in 2018 from the Southeast University. His research interest lies in the fabrication of bio-inspired surfaces and biomaterials. |
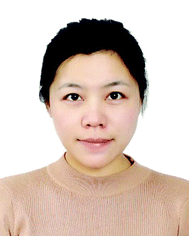
Changmin Shao
| Changmin Shao is currently an assistant professor at Wenzhou Institute, University of Chinese Academy of Sciences. She received her PhD degree in 2017 from the Northeast Forestry University. Her research interest lies in the fabrication of porous materials based on microfluidics for cell culture and tissue regeneration. |
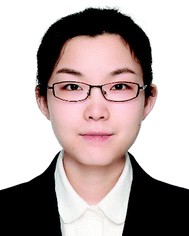
Luoran Shang
| Luoran Shang is currently an assistant professor at Fudan University, Institutes of Biomedical Sciences. She received her PhD degree from Southeast University under the supervision of Prof. Yuanjin Zhao. She worked as a postdoctoral fellow at Harvard University with Prof. David Weitz from 2017 to 2019. Her current scientific interests are focused on microfluidics and their biomedical applications. |
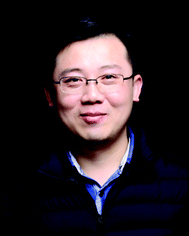
Yuanjin Zhao
| Yuanjin Zhao received his PhD degree in 2011 from Southeast University. In 2009–2010, he worked as a research scholar at Prof. David A. Weitz's group at Harvard University. In 2015, he was promoted to be a full professor at Southeast University. His current scientific interests include microfluidics, ferrofluids, biomaterials, and organs-on-chips. |
1. Introduction
Wettability is defined as the ability of a liquid to maintain contact with a solid surface, which is determined by the intermolecular forces at the interface of the interacting phases. In nature, wettability is a universal phenomenon that can be easily found from the microscale to macroscale, such as the ion channels in cell membranes and the flood tides on the beach. In nature, creatures have survived by transforming to their optimum form of existence during the long period of evolution against the complicated and changeable environment, and have developed tremendous functions, including various specific wettability features. This has long been an inspiration for human beings to investigate the underlying mechanism for the creation of novel functional materials. Research studies on wettability can be traced back to more than two hundred years ago, since the proposition of Young's equation in 1805.1 Since then, several fundamental research studies have been initiated towards the development of different principles and models, which are still widely accepted and regarded as classics nowadays. The first report on a super-antiwetting surface was made in 1907 by Ollivier et al., who coated a substrate with soot to achieve a particularly large contact angle (CA) of nearly 180°.2,3 In 1953, Bartell et al. found that paraffin with a microscale pyramid shape on its surface emerged as an excellent water repellent.4 Thereafter in 1997, Barthlott and Neinhuis came up with the famous theory of the “lotus effect” that originated from nature, which changed the continuous but relatively circumscribed attention of the so-called superhydrophobicity and raised a new climax.5 They found that both the epicuticular wax and the microscale composite structures on the surface contributed to the self-cleaning effect based on the superhydrophobic property. Except for superhydrophobicity, another crucial superwetting phenomenon is superhydrophilicity (with a CA close to 0°), which was first proposed in 1959 regarding silicon wafer pretreatment. Based on these fundamental principles, a series of bio-inspired artificial materials possessing (super)hydrophilic/(super)hydrophobic properties have been fabricated for various applications including self-cleaning,6–8 anti-fouling,9–12 anti-fogging,13,14 anti-icing,15–18 anti-corrosion,19,20 anti-adhesion,21,22 water harvesting23–25 and oil–water separation26–28 (Fig. 1).
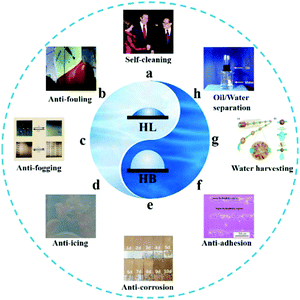 |
| Fig. 1 Bio-inspired artificial materials. (a) Superamphiphobic self-cleaning textiles. Reproduced with permission.29 Copyright 2005, Springer Nature. (b) Anti-fouling coating on ship hull to minimize manual cleaning. Reproduced with permission.30 Copyright 2013, Elsevier Inc. (c) The superhydrophilic coating exhibits an anti-fogging property by spreading the condensed water droplets quickly to form a water film. Reproduced with permission.31 Copyright 1997, Springer Nature. (d) Anti-icing, the superhydrophobic surfaces can suppress the formation of ice. Reproduced with permission.32 Copyright 2015, Wiley-VCH. (e) Anti-corrosion, the superhydrophobic surfaces can effectively inhibit the infiltration of corrosive ions. Reproduced with permission.33 Copyright 2019, Springer Nature. (f) The superhydrophobic surfaces also show anti-adhesion behavior for controllable cell culture. Reproduced with permission.22 Copyright 2010, American Chemical Society. (g) Water harvesting behavior inspired from the spindle-knotted structures of spider silks. Reproduced with permission.34 Copyright 2017, Royal Society of Chemistry. (h) A membrane with the integration of superhydrophobicity and superoleophilicity can be utilized for oil/water separation. Reproduced with permission.35 Copyright 2011, Wiley-VCH. | |
Artificial substrates with a desired single-type wettability feature can realize the manipulation of liquids, which is especially essential in scientific research, industrial manufacturing, and even daily life scenarios. These materials offer researchers a large body of inspiration for addressing the hotspot problems involving resources, environment, energy, and health.36 However, the manipulation of liquids with high selectivity and accuracy is usually difficult to achieve on a primary substrate with simple wettability. Fortunately, nature has endowed us with a solution by applying a patterned surface wettability. For example, the Stenocara beetle, mainly distributed in the Namib Desert, possesses a complex structure on its back elytra, in which hydrophilic bumps are randomly arranged on the hydrophobic elytra. Benefiting from the patterned wettability, the Stenocara beetle can consciously collect water on the hydrophilic bumps from mist, which helps it survive in the extreme desert environment.37 Similarly, spider silk is another wonderful example for water harvesting from mist. Spindle knots composed of random nanofibers and joints composed of parallelly aligned nanofibers are periodically arranged along the spider silk. Owing to the different arrangements of the nanofibers, the surface topography of the spindle knots tends to be more hydrophilic, giving rise to a gradient wettability between the adjacent spindle knots and joints, which acts as one of the driving forces for the directional transportation of the droplets originating from the joints to the spindle knots. Inspired by these wettability patterns, e.g., hydrophilic patterns on a superhydrophobic substrate and differentiated wettability distribution, precise control of liquids (e.g. converging and separating) on a patterned surface, with opposite or gradient wettability in the adjacent regions, can be achieved without external driving forces. In particular, micropatterns on a micro or even nano scale promote the development of brand new miniaturized platforms or devices, which are likely to simulate the hydromechanics and other liquid behaviors in cells, tissues and organs of living organisms.38–44 These micro and nano-fabricated devices have been broadly introduced into various fields of biomedical engineering, including drug screening, biosensing, tissue engineering and single-cell assays.
Because of the excellent performance in liquid manipulation and the applications of wettability materials, several excellent review papers on bio-inspired wettability surfaces have emerged. However, a cutting-edge review on patterned wettability with emphasis on its biomedical applications in recent years is still lacking. We believe that such a comprehensive and substantial review focusing on the various aspects of wettability patterns in the biomedical field would draw tremendous attention from researchers and arouse their inspiration. Herein, we present an overall review on recent progress in wettability patterns and their applications in biomedical engineering. Certainly, readers interested in general aspects of surface wettability can refer to some other reviews. In this review, we will first introduce the evolution of the study on surface wettability and wettability patterns, mainly concentrating on the wettability found in nature including simple surface wettability and patterned wettability. We will only briefly discuss the theoretical basis and common design principles, fabrication methods, and surface modifications for special artificial wettability, while guiding the readers to specialized reviews for more details. Subsequently, we will shift to the bio-inspired wettability patterns, with several frequently-used preparation methods as well as the features of the patterned wettability materials being emphasized. Then, we will focus on recent progress of the biomedical applications of bio-inspired wettability patterns including cell culture, drug screening, and biosensors. Finally, we will propose critical comments on the present situation and challenges, as well as perspectives on the development tendency of wettability patterns in the future.
2. General surface wettability
Inspired by the wettability phenomenon found in natural organisms, researchers have established biomimetic design for the fabrication of bio-inspired materials with (super)wettability. The principle lies in the combination of micro- and nanoscale hierarchical structures and chemical modification on materials.45–49 A substrate with a smooth surface normally shows either a hydrophilic or hydrophobic feature, while surface roughness amplifies the wettability, i.e., makes an initial hydrophilic surface more hydrophilic and a hydrophobic surface more hydrophobic, as demonstrated by the Wenzel model. On the other hand, surface energy can be modified by chemical modification, serving as another essential factor to decide the wettability of the solid surface. Thus, special (super)wettability could be achieved through the combined design of surface microstructures and chemical moieties. In this section, we mainly explore the natural wettability surface, the biomimetic design principle, and the general fabrication methods.
2.1 Organisms with special wettability
2.1.1 Superhydrophobic surface.
The lotus leaf is a representative material found in nature for superhydrophobicity, which is known as the “lotus effect” (Fig. 2a). In 2002, Jiang et al. reported that the water contact angle (WCA) on a lotus leaf is 162°, which endows it with the ability of self-cleaning.50 By investigating the microstructure of the lotus leaf, researchers found that the micro- and nanoscale hierarchical structure (microscale papillae consisting of nano-sized nipples) is the key to the superhydrophobic feature. In addition, peanut leaves51 and canna leaves52 also exhibit the feature of superhydrophobicity due to their micro- and nanoscale hierarchical structures. Except for the plants, the superhydrophobic phenomenon also exists in the animal kingdom to help the animals avoid getting wet on rainy and foggy days, and maintain self-cleaning ability. The water strider can not only float on the water surface, but also walk quickly without getting wet, which is mainly owing to the microstructure of its legs (Fig. 2b).53 The cicada with transparent, well-veined, membranous wings is another typical example of an insect that possesses natural superhydrophobic structures, benefiting from the periodic nanopillars on the wings.54
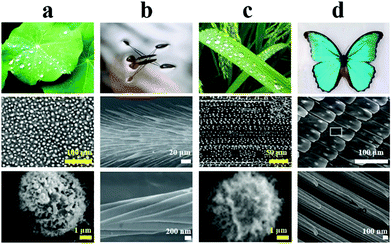 |
| Fig. 2 Organisms with superhydrophobic structures. (a) Lotus leaf. Reproduced with permission.58 Copyright 2002, Wiley-VCH. (b) Water strider legs. Reproduced with permission.53 Copyright 2004, Springer Nature. (c) Rice leaf. Reproduced with permission.58 Copyright 2002, Wiley-VCH. (d) Butterfly wings. Reproduced with permission.57 Copyright 2007, Royal Society of Chemistry. The scanning electronic microscopy (SEM) images in each column correspond to the specific microstructural feature of the respective superhydrophobic surface. | |
Additionally, rice leaf shows a particular “lotus effect”, in which water droplets can easily roll off along the direction of the leaf vein rather than in the perpendicular direction, owing to the anisotropic wettability (Fig. 2c).55 Similarly, butterfly wings not only exhibit superhydrophobicity but also possess directional adhesion properties (Fig. 2d). It is found that the directionally arranged scales on the wing surface prompt droplets to roll off the surface in the radial direction when the wings are slightly tilted down, which protects the butterfly wings from getting damp during a flight in a wet environment.56,57
2.1.2 Superhydrophilic surface.
Superhydrophilicity is another remarkable characteristic found in certain creatures in nature, which is significant for the uptake of water and nutrition as well as predation. Nepenthes alata is a typical species of prey-trapping pitcher plant, which has a highly modified, prey-trapping pitcher filled with digestive fluid. It has an arch-shaped peristome at the upper rim of the pitcher. The two-order hierarchy of parallel microgrooves shows a regular radial arrangement, which promotes water spreading from the inner side to the outer side on the peristome surface to form a film of water (Fig. 3a).59 In addition, the hydrophilic hygroscopic nectar secreted from extrafloral nectaries covers the microgrooves, which further facilitates the superhydrophilicity of the surface. Owing to the superhydrophilic surface, insects aquaplane on this slippery surface, fall into the pitcher, and then are digested by the inner digestive fluid. Interestingly, the superhydrophilic structures can also present the capability of self-cleaning. The sector-like fish scales composed of calcium phosphate, protein and a secretory mucus are overlapped into an array with rough micropapillae arranged in the radial direction (Fig. 3b).14,60 These hierarchical micro-/nanostructures structures induce water to form a film on the surface of the fish displaying an excellent superoleophobicity under-water to protect fish from being contaminated. Additionally, precorneal tear film, clam shell (Fig. 3c) and lizard skin (Fig. 3d) are also typical examples of superhydrophilic structures. Thus, benefiting from superhydrophilicity, creatures have evolved various functions, to help them survive in natural selection, such as precorneal tear film, clam shell,61 lizard skin,62etc.
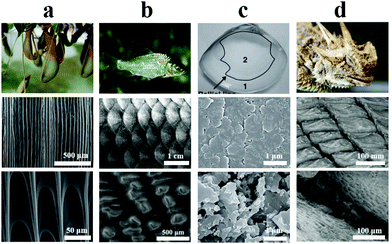 |
| Fig. 3 Organisms with superhydrophilic structures. (a) Pitcher plant. Reproduced with permission.59 Copyright 2016, Springer Nature. (b) Fish scale. Reproduced with permission.63 Copyright 2009, Wiley-VCH. (c) Clam shell. Reproduced with permission.61 Copyright 2012, Wiley-VCH. (d) Lizard skin. Reproduced with permission.62 Copyright 2015, Royal Society. The SEM images in each column correspond to the specific microstructural feature of the respective superhydrophilic surface. | |
2.2 Fabrication of an artificial wettability surface
2.2.1 Artificial microstructures.
The fantastic hierarchical micro-/nanostructures with special wettability in nature not only present us with various wonderful life forms, but also underlie a biomimetic, cost-efficient strategy for the fabrication of artificial wettability materials. This has been achieved with the joint advance of microfabrication techniques. Moulding replication is widely applied for the generation of complicated hierarchical micro-/nanostructures and allows for massive production.64,65 Usually, by casting curable materials (e.g. polydimethylsiloxane, PDMS) over a mould and removing the mould off after solidification, the negative template with the inverse structure can be obtained (Fig. 4a).66 With another round of casting, the desired material with the identical structure to that of the original copy can be fabricated flexibly.67 For example, Jiang et al. fabricated artificial peristomes of Nepenthes alata using the replica moulding method using PDMS. After adjusting their surface energy via oxygen plasma treatment to achieve superhydrophilicity, the PDMS peristome surface displayed directional water transport similar to that of the natural peristome surface (Fig. 4b and c).59
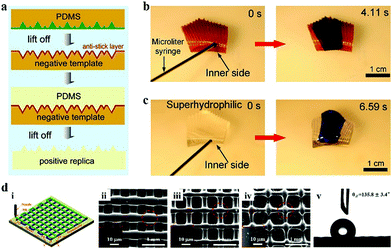 |
| Fig. 4 Moulding replication and 3D printing for wettability surfaces. (a) Scheme of moulding replication for fabricating superhydrophobic surface. Reproduced with permission.64 Copyright 2005, American Chemical Society. (b) Water transporting on the inner side of Nepenthes alata. (c) Water transporting on the artificial PDMS peristome surface. Reproduced with permission.59 Copyright 2016, Springer Nature. (d) Films with special surface wettability by 3D printing. (i) Scheme of 3D printing. (ii–iv) SEM images of samples printed at different printing speeds. (v) Water contact angle image on the fabricated sample. Reproduced with permission.68 Copyright 2017, Elsevier Ltd. | |
Compared to moulding replication, 3D printing is a unique approach that enables the generation of elaborate, large-area, and complicatedly-patterned 3D structures without the requirement of expensive moulds or multi-step processing.69–76 It has thus been employed in the fabrication of a wettability surface with desired structural features (Fig. 4d).68 Liu et al. reported a series of 3D printed PDMS surfaces with special wettability by patterning PDMS ink on wafers. On modulating the micron scale pore structures, the prepared PDMS porous structures presented differently special surface wettability including superhydrophobicity and anisotropic wettability. Two-photon polymerization is a newly developed printing technology that can construct structures with nanometer resolution at a scan speed of 10
000 μm s−1 in three dimensions.77 Using this technology, Gu et al. successfully fabricated triply reentrant arrays on both rigid and flexible substrates, and the resultant materials exhibited super-repellence to water and various organic liquids.78
Besides, lithography,79–81 laser processing,82–84 oxygen plasma treatment,85–87etc., which eliminate the restriction of natural moulds, have been put forward as versatile, flexible, scalable and economical strategies for the fabrication of artificial wettability microstructures. Except for physical methods, various approaches based on chemical reactions have also provided efficient and effective strategies for the fabrication of artificial biomimetic structures with special wettability, including electrodeposition,88–91 sol–gel process,92–95 hydrothermal method,96–98 chemical etching,99etc. By utilizing these above-mentioned methods, a series of superhydrophobic and superhydrophilic materials have been designed.
2.2.2 Surface modification.
Surface modification is another strategy for the fabrication of wettability materials since surface chemistry also strongly affects wettability. In the case of superhydrophobicity, the surface energy of the solid surface should be lower than that of the given liquid to avoid getting wet.100,101 To lower the surface energy, low-surface-tension reagents such as fluroroalkanes, fluoropolymers and fluorosurfactants are widely utilized to decrease the surface tension benefiting from properties of the fluorine atom. In the same way, a lot of reagents like silane coupling agent ending with the hydrophilic groups of –COOH, –OH, –NH2etc. can be utilized to enhance the hydrophilicity of the surfaces.102,103
Wet-chemistry refers to a chemical reaction between the substrate surfaces and compounds in solution. The most widely employed wet-chemistry modification method is silanization, in which the hydroxyl groups on a substrate surface react with silane coupling agents to form covalent Si–O–Si bonds. Wang and co-authors modified the electrospun silica nanofibrous mats using (fluoroalkyl) silane (FAS) to realize the amphiphobic property (Fig. 5a). The fabricated superhydrophilic mats, composed of different fractions of silica, showed an obvious difference in hydrophobicity, which was attributed to the difference in the amount of silicon hydroxyl groups.104 Commonly, except for those surfaces that are rich in hydroxyl groups, some effective strategies are also adopted to increase the amount of hydroxyl groups, such as O2 plasma, UV/O3 and Tesla coil treatment.105,106 In addition, hydrolysis and aminolysis of polyesters are also typical methods to generate hydrophilic or hydrophobic groups at the surface.
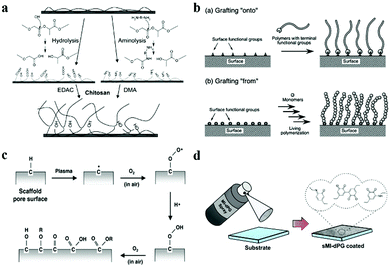 |
| Fig. 5 Surface modification. (a) Scheme of wet chemistry modification. Reproduced with permission.107 Copyright 2004, American Chemical Society. (b) Scheme of polymer grafting. Reproduced with permission.108 Copyright 2013, Royal Society of Chemistry. (c) Scheme of plasma treatment modification. Reproduced with permission.109 Copyright 2013, IOP Publishing Ltd. (d) Schematic diagram of spray coating.110 Copyright 2018, Wiley-VCH. | |
Similar to wet-chemistry surface modification, polymer grafting is another frequently used method, in which polymers with specific functional groups are introduced onto the substrate surfaces to alter the surface wettability. In general, there are two main strategies for polymer grafting: grafting “onto” and grafting “from”.108,111–113 As shown in Fig. 5b, grafting “onto” is a process of bonding between a prepared polymer and functionalized end groups on a solid surface, while grafting “from” refers to the polymerization of monomers initiated by functionalized groups on a solid surface.114
Plasma treatment is another effective strategy that can alter the surface chemical constitution. Plasma is a partially ionised gas commonly produced by extremely high-temperature heating or strong electromagnetic fields.115–120 Usually, pure reactive gases such as O2 are used to create functional groups on the surface of substrates (Fig. 5c). Sometimes, air is directly utilized to introduce hydroxyl groups onto the surface instead of O2 for the case of rough hydrophilic treatment. The introduction of hydrophilic polar groups, such as –OH, –COOH, and –NH2, onto the hydrophobic polymer surfaces by glow discharge plasma is the most generally adopted plasma surface modification method for hydrophilic treatment.121–126 In addition, prolonged plasma treatment with high power is always accompanied by surface etching, which causes melting and merging of polymer materials.127 Thus, the plasma treatment can not only change the chemical constitution but also enhance the surface roughness, which further enhances the surface wettability.
Surface coating is another method that essentially distinguishes from the aforementioned methods. Instead of undergoing chemical reactions, surface coating can be achieved simply by deposition, and the resultant surface wettability depends on the chemical property and the homogeneity of the deposition species.128 For example, Haag and co-workers developed a mussel-inspired spray coating strategy for versatile surface modification, such as anti-bacterial property and water repellency (Fig. 5d).110
Among the surface modification methods mentioned above, the methods of wet-chemistry and polymer grafting are relatively complex compared to plasma and coating. Accordingly, they can provide abundant choices for researchers to modify the surface with desired wettability using controllable chemical reactions. In contrast, the methods based on plasma treatment and coating are plain but easy to operate. The modification effect of plasma can be easily optimized by adjusting parameters like power, time and type of gas. The modification achieved by coating is mainly dependent on the physical adsorption, which lacks durability. Thus, an appropriate modification method should be adopted for fabricating surface wettability.
3. Patterned surface wettability
3.1 Natural wettability patterns
Except for the creatures with single-type wettability, structures possessing differentiated wettability in nature also present some wonderful functions and even dramatically excellent performance in certain special fields. The Stenocara beetle, a kind of beetle mainly distributed in the Namib Desert can collect water for drinking from fog on its back. Analysis of the structure of the beetle's elytra has revealed that a micropatterned surface with differentiated wettability is present on the elytra. Besides, a hydrophilic bump array with a diameter of 0.5 mm and interval of 0.5–1.5 mm is randomly arranged on the hydrophobic elytra. More specifically, the peaks of the bumps are hydrophilic attributed to the wax-free structure, while the substrate covered with wax shows hydrophobicity. Benefited from the obvious contrast in wettability between the bumps and the substrate, the beetle can collect water from the foggy air for drinking by tilting its back against the wind in extremely droughty desert areas. The water is captured in the hydrophilic peaks, forming droplets that grow fast by not only the continuous capture upon the peaks but also the converging from the hydrophobic regions. The droplets detach from the bumps on reaching a size threshold and roll down along the sloping surface of the back to the beetle's mouthparts.
Except for the patterns with two completely different states of wettability (i.e. hydrophilic and hydrophobic), a differentiated or gradient wettability pattern also exists in natural organisms, particularly for dynamic droplet behaviors such as directional transport. For example, spider silk is composed of hydrophilic flagelliform proteins and periodical puff-joint arrangement in its structure under the dry state. The puffs shrink and form spindle-knots after adsorbing water from the fog. Environmental SEM analysis shows that the spindle knot is composed of random nanofibers which render a high surface roughness, while the joint is composed of parallelly aligned nanofibers which present a relatively smooth surface topography. According to the Wenzel model, the roughness of the spindle knot surface makes the inherently hydrophilic spider silk more hydrophilic, which gives rise to a gradient of wettability between the adjacent spindle knots and joints. The surface energy gradient induced by the wettability gradient can act as a driving force, which, along with the Laplace pressure gradient created by the geometric feature, contributes to the directional transportation of droplets from the joints to the spindle knots.
3.2 Artificial wettability patterns
Benefiting from the design principles inspired by nature and the effective fabrication and modification technologies, a lot of materials with special wettability have been proposed on a large scale and have shown increasing application prospects. However, surfaces with monotonous wettability restrict their development to some extent. Interested in the distinct contrasting wettability, a vast number of research studies have been focusing on the fabrication and applications of patterned wettability surfaces. There are several emerging methods for the preparation of various patterns with different constructions such as arrays and channels. In this section, we will introduce some of the most widely used methods for the fabrication of patterned wettability materials, including mask-based fabrication, printing, particle assembly and microcontact printing.
3.2.1 Mask-based fabrication.
A mask used for pattern fabrication is a thin film with hollowed-out or transparent/non-transparent patterns on it, which can be made of stainless steel, resin or other materials.129 After being covered by a mask, active reactions like surface modification occur at the position corresponding to the patterns on the substrate surface.130 Subsequently, the wettability of the patterned areas is obviously different in contrast to the regions out of the patterns. Photolithography, which is originally derived from micro-electro-mechanical systems (MEMS), is typically associated with masks to fabricate specific patterns. Photolithography involves spin-coating of a photoresist, soft baking, exposure, post exposure baking, development and hard baking, and other procedures (Fig. 6a). A light source radiates through the mask containing designed patterns to degrade or polymerize/crosslink the photoresist at exposed areas. Commonly, photolithography is divided into UV lithography and X-ray lithography on the basis of the light source (Fig. 6b).131–135 The line width of the pattern ranges from thousands of microns down to 5 nm based on the type of mask and the wavelength of light.136 Zhang et al. first reported a facile, low-cost and high-throughput method based on photolithography for the fabrication of cloth microfluidic analytical devices. They chose cotton cloth as the substrate material and employed a hydrophobic photoresist to prepare reproducible hydrophilic–hydrophobic patterns on the cloth with well-defined and uniform boundaries. The resolution of the hydrophilic–hydrophobic patterns could be down to 100 μm, which was appropriate for achieving cloth-based high-throughput bioassays with small volumes of samples and reagents in each reservoir.137
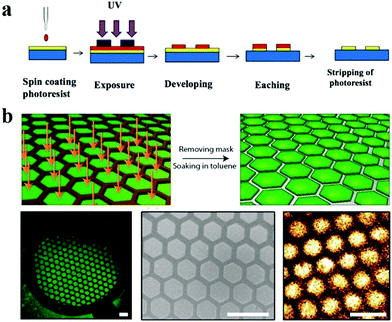 |
| Fig. 6 Lithography for preparing patterns. (a) Scheme of photolithography. (b) Patterned perovskite nanocrystal film fabricated using X-ray lithography. Scale bars are 300 μm. Reproduced with permission.133 Copyright 2015, American Chemical Society. | |
In addition, Levkin's group developed a series of droplet microarray (DMA) platforms for miniaturized high-throughput cell screening in volumes down to several nanoliters using the method of mask-based hydrophilic-superhydrophobic array fabrication. Typically, a microscope glass slide was firstly coated with a layer of nanoporous 2-hydroxyethyl methacrylate-co-ethylene dimethacrylate (HEMA-co-EDMA) followed by Steglich esterification of the polymer using 4-pentynoic acid. Next, the surface was wetted with a solution of 1H,1H,2H,2H-perfluorodecanethiole and covered with a quartz photomask of the desired pattern. After UV irradiation at 254 nm (2.0 mW cm−2), the slide was washed and wetted again with ethanol–water (1
:
1) solution containing 10 wt% of cysteamine hydrochloride. Finally, the slide was covered with a clear quartz glass slide and irradiated again with UV light. The DMA slides were prepared using the site-selective immobilization of 1H,1H,2H,2H-perfluorodecanethiole and click chemistry.138 This developed method is of great similarity to photolithography aforementioned and is believed to find a wide range of applications.
Except for the UV irradiation-induced patterns, Zhao et al. proposed a patterned plasma treatment and sol–gel combined method for preparing superhydrophilic/superhydrophobic patterns. The natural superhydrophobic butterfly wing was chosen as the substrate for the fabrication of this wettability pattern. After being covered by a stainless steel mask with the desired dotted patterns, the butterfly wing was treated using air plasma. Owing to the O2 in the air activated by the glow discharge, the exposed dotted areas on the butterfly wing were grafted with hydroxyl groups and thus became superhydrophilic by the patterned modification. To preserve the patterned wettability permanently, a UV curable sol was applied onto the patterned butterfly wing to form droplet arrays and solidified using UV irradiation. Benefiting from the difference in wettability between the superhydrophobic substrate and the superhydrophilic gel arrays, droplet arrays could form on the prepared substrate for cell culture.139
3.2.2 Printing.
A typical example of printing is the green printing technology, which was developed by Song's group, to print images based on superhydrophilic/superoleophilic patterns. Briefly, a superhydrophilic plate was firstly deposited on a substrate, then a specific transfer material was printed precisely onto the superhydrophilic plate. By the controllable printing of transfer materials and the interface properties between transfer materials and the plate, the patterns with the opposite wettability (superhydrophobic/superhydrophilic) were prepared on the plate, which could be utilized as a plate for printing directly. The oleophilic ink was selectively adsorbed on the patterns and well controlled within the patterns without spreading. Owing to the precise control of the printed patterns, the printing plate was promising to satisfy the routine print with fine resolution. Moreover, the prepared plates exhibited superior durability which was robust for printing for thousands of times.140
Actually, the conventional laser printing can also achieve wettability patterns. Liu et al. proposed an easy-to-operate method for the fabrication of hydrophilic/superhydrophobic patterns. Commonly, the layer printed by toner used in a laser printer is hydrophobic and its WCA can reach 119°. Different from the common method, the authors mechanically doped superhydrophobic silica nanoparticles into the toner, which made the print layer exhibit enhanced hydrophobicity with the increase of superhydrophobic silica nanoparticles and the contact angle could increase up to 151.1°, showing superhydrophobicity. Through the laser printing process, well-designed patterns with superhydrophobicity could be printed on papers or transparent PET films (Fig. 7a). To find practical applications, the authors further printed superhydrophobic patterns on a prepared hydrophilic photonic crystal membrane to generate droplet arrays for digital PCR.141 Owing to the significant wettability difference of the patterns, microdroplets could be easily generated through a simple operation of droplet sliding (Fig. 7c). The generated microdroplets were uniform in size, benefiting from the precise patterns generated by high print resolution (Fig. 7d and e).
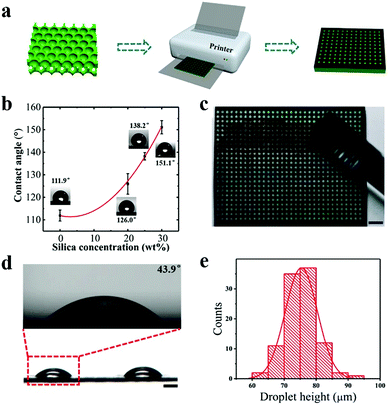 |
| Fig. 7 Printing for preparing wettability patterns. (a) Schematics of the fabrication of micropatterns. (b) Water contact angles on printing layers doped with different amounts of superhydrophobic silica. (c) Image of the droplet formation on wettability patterns. Scale bar is 2 mm. (d) Side view of the formed droplets on the wettability patterns. Scale bar is 100 μm. (e) Distribution of the droplet height. Reproduced with permission.141 Copyright 2018, American Chemical Society. | |
3.2.3 Particle assembly.
Another simple strategy for the preparation of wettability patterns is to “install” patterns on substrates with opposite wettability using physical methods, which is also called particle assembly.142 For instance, Jiang and co-workers proposed a bio-inspired photonic crystal microchip by assembling hydrophilic nanoparticles on a hydrophobic PDMS substrate.143 Briefly, the monodispersed colloidal spheres (poly(styrene-methylmethacrylate-acrylic acid), poly(St-MMA-AA) were inkjet printed on a PDMS film. Owing to the difference in wettability, the colloidal particle solution formed droplets on the film and assembled into crystal photonic dots on complete drying, which resulted in a bio-inspired wettability pattern array. It is worth noting that the assembled particle patterns were easy to detach from the hydrophobic surface. Thus, the authors first printed the colloidal spheres on a pre-curing PDMS film and further cured the PDMS after particle assembly to enhance the robustness of the patterns. Similarly, Zhao's group presented another strategy for the immobilization of the assembled colloidal crystal. They first treated the superhydrophobic butterfly wings using plasma with a dotted mask to prepare hydrophilic arrays on the wings. Then silica colloidal particle solution was dropped on the hydrophilic areas for self-assembly (Fig. 8). The specific hierarchical structure of the butterfly wing, which was covered by parallel-ridge-composed scales, allowed the permeation of the colloidal particles into the structure and promoted the immobilization of the assembled colloidal crystal. In this way, they fabricated superhydrophilic colloidal crystal patterns on superhydrophobic butterfly wings.144
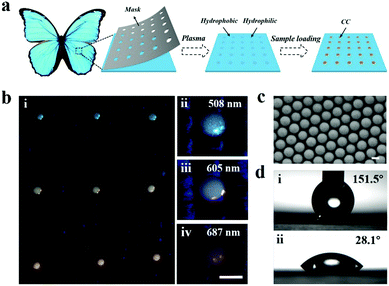 |
| Fig. 8 Wettability patterns prepared by particle assembly. (a) Scheme for the fabrication of wettability patterns on superhydrophobic butterfly wings using particle assembly. (b) Images of wettability patterns prepared by nanoparticles with different stopbands. Scale bar is 100 μm. (c) SEM image of the assembled nanoparticles. Scale bar is 200 nm. (d) Water contact angles measured on the butterfly wing and micropattern. Reproduced with permission.144 Copyright 2019, Elsevier Inc. | |
3.2.4 Micro-contact printing.
Micro-contact printing (μCP), a versatile printing technique, also provides a dramatical strategy for the fabrication of wettability patterns.145–147 Usually, a stamp with well-designed patterns is used to remove or attach a wettability material from or onto a completely contrary substrate in wettability. In this way, Song and co-workers utilized a commercial optical disk as the micro-contact printing template to construct hydrophilic–hydrophobic patterns in the mode of rolling. Benefited from the surface tension restraint between the hydrophilic and hydrophobic patterns, most of the perovskite precursor could be effectively enriched on the hydrophilic areas, which gave rise to a competitive growth during the nucleation and growth of perovskite between the opposite wettability areas. Moreover, the wettability of the substrates could be precisely tuned by the micro-contact printing template with different modification parameters (Fig. 9).145
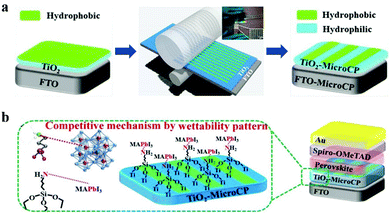 |
| Fig. 9 Micro-contact printing for wettability pattern fabrication. (a) Scheme for the fabrication of wettability patterns through micro-contact printing. (b) Schematic diagram of competitive growth of perovskite on the wettability pattern. Reproduced with permission.145 Copyright 2019, Wiley-VCH. | |
In general, the aforementioned methods are just the widely adopted ones which have acquired dramatic achievements. There are still some other advanced techniques that can realize the fabrication of wettability patterns. Kota and co-workers fabricated a metamorphic superomniphobic surface with a thermoresponsive shape memory polymer. Using a mask based reactive ion etching (RIE), elaborate microstructures of shape memory polymer could be obtained, which exhibited superomniphobicity. Furthermore, the microstructures could be transformed into a collapsed shape by stamp-based heating, resulting in the patterned wettability transformation. And, the wettability could also be recovered and remodeled, which endowed the surface with a capability of rewritable wettability patterns.148 It's worth noting that, neither the mask-based fabrication nor any other methods like printing can accomplish the wettability pattern fabrication independently. It is necessary to integrate the lager-scale wettability structure fabrication methods and surface modifications we summarized above with the patterning strategies, by which a variety of bio-inspired wettability patterns are achieved and applied to various fields, especially biomedical applications.
4. Biomedical applications of wettability patterns
The bio-inspired wettability patterns have attracted increasing interest benefiting from their excellent performance and broad applicability. Up to now, the bio-inspired wettability patterns have been employed in cell culture, drug screening, biosensors, microreactors, digital microfluidics, anti-bacterials and many other biomedical fields. It has been found that wettability patterns provide a versatile high-throughput platform of droplet arrays, making them ideal candidates for ex vivo medical research studies. Besides, wettability patterns can manipulate liquid samples spontaneously owing to the dramatical wettability difference, which makes them ideal for clinical applications. In addition, the various developed methods and integrated strategies make it convenient for fabricating wettability patterns with desired features, which is promising in mass production and production translation.
4.1 Cell culture
Cell culture provides a powerful tool for drug discovery and development, tissue engineering, exploring biomechanics and stem cell therapy. Compared to traditional cell culture using microtiter plates or flasks, some emerging strategies such as surface modification of substrates create more critical opportunities for the development of cell-based research studies, especially for high-throughput assays. For example, patterned modification of flat surfaces with antifouling polyethylene glycol (PEG) brushes could promote the formation of high-density cell microarrays.149 In addition, 2D droplet microarray technology generated by superhydrophobic/superhydrophilic micropatterning provides an ideal miniaturized and high-throughput platform for 2D cell culture and 3D cell culture, as well as the parallel addition processes during the cell culture, such as the addition of medium and cell implantation.150
4.1.1 2D cell culture.
Cells cultured in a flask can result in high-yield proliferation, while it is difficult to achieve high-throughput operation in a single flask. Although microtiter plates with hundreds of reservoirs offer an effective platform to resolve this problem, pipetting of each drop into the individual reservoir individually is not convenient and stuffy in a routine laboratory without mechanical arms. In this context, Levkin's group presented a single-step generation of discrete pico- to microliter-sized aqueous droplet arrays with defined geometry and volume on a superhydrophilic/superhydrophobic patterned slide surface (Fig. 10a). The formation of the microdroplet arrays was based on the phenomenon of discontinuous dewetting. To be specific, a bulk droplet was broken into thousands of microdroplets at each superhydrophilic spot by the extreme wettability contrast between the superhydrophilic and superhydrophobic patterns instead of forming a liquid film when rolling across the superhydrophilic/superhydrophobic micropatterned substrate (Fig. 10b).151 This discontinuous dewetting eliminated the complicated pipetting steps and thus saved much time. In the same way, cells could also be seeded onto the superhydrophilic spots by rolling cell suspension on a slide or pulling up the slides from the cell suspension (Fig. 10c).152 Cells could be cultured on the slide for proliferation and further high-throughput screening (Fig. 10d). Moreover, medium exchange was also explored on this platform, which was usually hard to achieve in miniaturized cell screening systems like droplet microfluidics (Fig. 10e).153 Owing to the openness of the droplet-microarray platform, the medium could be readily renewed by the “rolling droplet” method. Briefly, a definite volume of fresh medium was applied to the surface and rolled by tilting the slide slightly to allow the medium to roll through the whole slide, thereby forming individual droplets of fresh medium at the superhydrophilic spots.
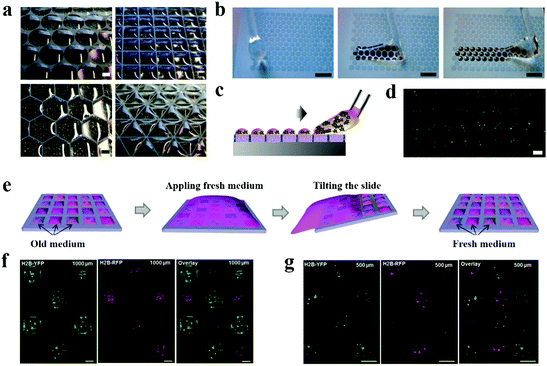 |
| Fig. 10 2D cell culture on wettability patterns. (a) Microdroplet arrays formed on the superhydrophilic/superhydrophobic patterns of different geometries. Scale bars are 1 mm. (b) Snapshots of droplets formed on a superhydrophilic/superhydrophobic patterned surface by rolling water. Scale bars are 3 mm. (c) Schematic showing the formation of droplets containing cell suspension. (d) HeLa-GFP cells cultured in microdroplet arrays. Scale bar is 500 μm. Reproduced with permission.151 Copyright 2012, Royal Society of Chemistry. (e) Scheme of medium exchange on microdroplet arrays. (f and g) HEK293 cells transfected with pCS2-H2B-YFP (green) and pCS2-H2B-RFP (red) on microdroplet arrays with 1000 μm (f) and 500 μm (g) spot size. Scale bars are 500 μm. Reproduced with permission.153 Copyright 2016, Royal Society of Chemistry. | |
High-throughput cell transfection is an essential strategy for the identification of gene and protein function. Instead of standard microplates and Sabatini's reverse cell transfection arrays, Levkin et al. proposed a reverse transfection method based on a droplet-microarray on as-prepared superhydrophilic/superhydrophobic micropatterns.153 Firstly, the transfection compounds including transfection reagents, plasmid DNA containing H2B-YFP or H2B-RFP gene and gelatin were printed onto the superhydrophilic spots on the slide and pre-immobilized by drying. Then, HEK293 cells were seeded on the slide for culture and transfection. By fluorescence microscopy assessment after seeding, HEK293 cells were successfully transfected with plasmids and expressed H2B-YFP and H2B-RFP with a transfection efficiency as high as 80% (Fig. 10f and g). In addition, cross-contamination was effectively eliminated between the individual droplets during the seeding procedure, even though the superhydrophobic gap between the adjacent superhydrophilic spots was only 175 mm.
4.1.2 3D cell culture.
2D cell culture has been regarded as the standard method for the study of cell functions. However, the growth mode of 2D culture is extraordinarily different from the stereoscopic environment in the body, which leads to obvious differences in cell morphology, cell differentiation, the interaction between the cell and matrix, cell to cell connection and cell behavior compared to physiological conditions in vivo. More and more evidence has shown that the 3D cell culture is closer to the in vivo environment. Thus, various methods and strategies have been explored for 3D cell culture. Recently, the generation of 3D cell spheroids by hanging droplets based on superhydrophilic/superhydrophobic arrays has received great attention (Fig. 11).154–156 Cells seeded on the superhydrophilic areas will converge together at the bottom of the droplets under gravity after the substrates are turned over to form a hanging drop. Cells proliferate and form cell spheroids in the droplets after several days of incubation. Benefiting from the high-throughput droplet arrays, a vast number of spheroids with controllable morphology and size as well as specific protein secretion are obtained. However, most of the methods for preparing superhydrophilic/superhydrophobic patterns require complex micromachining or surface chemical modification, which increases the costs and limits their further applications. To overcome these disadvantages, Zhao's group proposed a new strategy by choosing the superhydrophobic butterfly wing as the bio-templated substrate, which was then integrated with wettable hydrogel patterns for preparing cell spheroids using the hanging drop method (Fig. 11c).139 The superhydrophilic/superhydrophobic patterns could be prepared using a mask in one step without complicated processing and chemical modification. The as-prepared wettability patterns could be used directly to culture spheroids in hanging drops. This strategy offers an easy manufacturing and resourceful platform for the preparation of cell spheroids in large quantities.
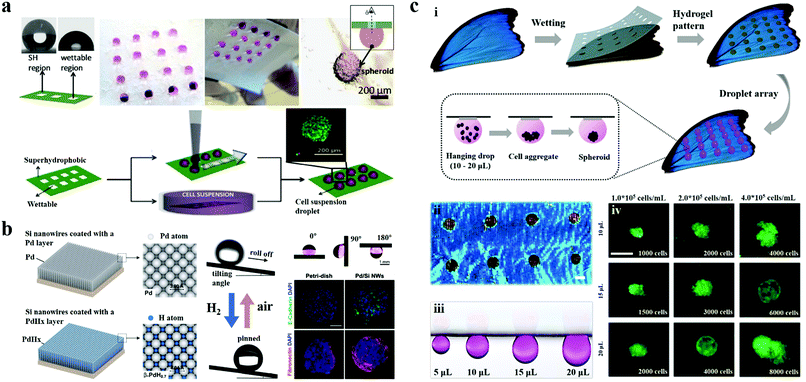 |
| Fig. 11 3D cell culture on wettability patterns. (a) Biomimetic superhydrophobic patterned chips for producing cell spheroids/microtissues using the hanging drop methodology. Reproduced with permission.155 Copyright 2014, American Chemical Society. (b) Bio-inspired superhydrophobic silicon nanowires with switchable water adhesion properties for 3D stem cell spheroid culture. Scale bar is 100 μm. Reproduced with permission.156 Copyright 2014, Wiley-VCH. (c) Hydrogel patterned butterfly wings for 3D cell spheroid preparation. Scale bars are 100 μm and 500 μm, respectively. Reproduced with permission.139 Copyright 2019, American Chemical Society. | |
4.1.3 Parallel addition.
Although high-throughput cell culture and screening are conducive to the development of biological and medical research, they are still facing the limitation of one by one and time-consuming manual operation or dependence on costly dedicated liquid-handling robots.157,158 The microdroplet arrays based on the bio-inspired wettability patterns not only provide an effective platform for high-throughput cell culture but also present an emerging strategy for the parallel addition of compound libraries (Fig. 12). Levkin et al. reported a droplet microarray “sandwiching” technology to achieve the addition of different (bio)chemicals into individual microdroplets on planar substrates with only one operation step.159–161 Typically, the to-be-added substances including mediums, transfection reagents, stains, and cells were firstly applied onto an as-prepared superhydrophilic/superhydrophobic patterned slide to form microdroplet arrays. Then, in a precise face-to-face alignment with another slide, which also contains microdroplet arrays, the “sandwiching” of the microdroplet arrays is performed, resulting in the fusion of the compounds in every individual microdroplet without any cross-contamination between adjacent droplets. Notably, this approach could be accomplished by just using a simple handheld device (Fig. 12c). This strategy offered an emerging and straightforward method for chemical addition in high-throughput assays. However, there still remained a nonnegligible challenge that approximately 50% of the added solutions would stay on the slide after the sandwiching.
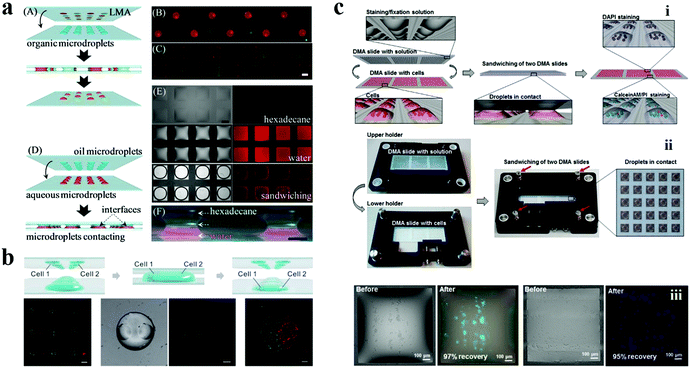 |
| Fig. 12 Convenient parallel addition by wettability patterns. (a) Simultaneous addition of chemicals. Scale bars are 500 μm. Reproduced with permission.160 Copyright 2016, Wiley-VCH. (b) Side-by-side assembly of two cell populations in individual microdroplets. Scale bars are 300 μm, 100 μm and 100 μm, respectively. Reproduced with permission.162 Copyright 2016, Wiley-VCH. (c) A handheld device for high-throughput manipulations. Reproduced with permission.163 Copyright 2016, Society for Laboratory Automation and Screening. | |
4.1.4 Single cell analysis.
By optimizing the design, the scale and the size of the wettability patterns can be controlled to meet different requirements. In practice, the volume of the produced droplets can be decided by a number of factors such as solution surface tension shape of the hydrophilic areas, ambient humidity, surface defects, and the size of the hydrophobic gap between the hydrophilic spots. By tuning these parameters, the produced droplet could be made as small as 3 nL in volume with low variability, which requires about 15
000 times less culture medium and reagents compared to the 384-well microplates. The small volume of the obtained droplets offers the possibility for single cell analysis, especially high-throughput single cell analysis by combining the microdroplet arrays. Levkin's group obtained a probability of 20% for the distribution of single cells by optimizing the microdroplet array on their wettability patterned substrate (Fig. 13a and b).164 Additionally, Song et al. utilized patterned superhydrophilic/superhydrophobic substrates to split a water droplet into uniform tiny microdroplet arrays with the volume low to femtoliter to obtain single cell arrays (Fig. 13c).165 Except for optimizing the volume of produced microdroplets, the cell suspension concentration is another key factor that should be considered for obtaining the highest single cell probability in microdroplet arrays.
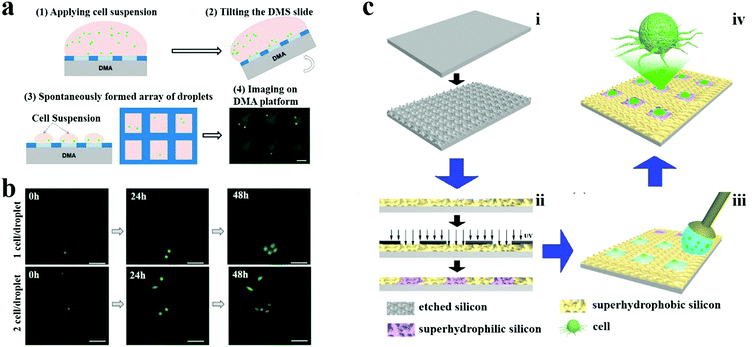 |
| Fig. 13 Single cell analysis on wettability patterns. (a) Formation of microdroplets containing single cells. Scale bar is 300 μm. Reproduced with permission. (b) Monitoring of cell proliferation in individual droplets containing 1 (upper row) and 2 (lower row) cells, initially. Scale bars are 100 μm. Reproduced with permission.164 Copyright 2016, MDPI AG. (c) Single cell array produced by splitting a droplet on a patterned superhydrophilic/superhydrophobic substrate. Reproduced with permission.165 Copyright 2015, American Chemical Society. | |
4.2 Drug screening
The cell culture on 2D droplet arrays offers a high-throughput and miniaturized platform for high-throughput drug screening, which is obviously different from the microplate form. Levkin et al. presented a “reverse approach” to verify the half maximal inhibitory concentration (IC50) of doxorubicin on the microdroplet arrays.153 They pre-printed doxorubicin on the hydrophilic spots of the prepared wettability patterned slide. HeLa cells were then seeded onto this slide using the “rolling droplet” method to perform drug concentration screening rather than adding drugs to the cultured cells as in the traditional method. The IC50 of doxorubicin obtained using this method was 63.9 μM, which is significantly different from their previous work using 96-well microplates (3 μM) performed with the same experimental setup. The increase of the IC50 in the microdroplet arrays was attributed to a higher “medium volume” to “cell number” ratio in microplates compared to that of the microdroplet. That is, although the concentration of doxorubicin was the same in both microdroplets and microwells, the actual amount of the drug for a single cell was significantly lower in the droplet microarrays. In fact, the variation in the IC50 value of a drug in certain cell types has been proven to be related to the experimental setup.166–168
The microdroplet arrays can be utilized in drug screening not only for adherent cells but also for cell suspensions. Usually, the screening of cell suspensions faces more challenges than that of adherent cells. The most crucial issue is the risk of losing cells during the procedures of washing, staining, and fixation. Levkin's group utilized their proposed sandwiching approach and patterned a superhydrophilic/superhydrophobic substrate to perform screening of antineoplastic drugs for Jurkat human T-cell lymphocyte cell suspensions.163 To avoid the loss of cells, different amounts of antineoplastic drugs (doxorubicin, daunorubicin, cytarabine, dexamethasone, vincristine, and etoposide) diluted in pure RPMI-1640 medium or DMSO were printed and dried on pre-fluorinated slides in a defined pattern shape corresponding to the geometry of the microdroplet arrays containing suspension cells. During face-to-face sandwiching, the dried antineoplastic drugs on the pre-fluorinated slides were redissolved in the medium. The hydrophobic surface of the slides would not adsorb the medium, thereby avoiding the loss of cells after removing the slides. After being incubated for 72 h, the drug effect estimated through the viability of cells demonstrated the applicability of this platform for miniaturized high-throughput screening of cell suspensions.
In addition, taking advantage of the small size of the designed superhydrophilic/superhydrophobic patterns, the microdroplet arrays can be utilized for screening rare cells with low cell consumption, such as the limited tumor cell material available from a patient. Thus, the microdroplet arrays based on wettability patterns have been demonstrated as a versatile platform for drug screening.
4.3 Biosensors
Due to their advantages including high-throughput, high sensitivity, low cost, easy accessibility, and controllable liquid manipulation, the bio-inspired wettability patterns have also attracted substantial attention as progressive strategies for biosensors. In particular, the microdroplet-based microchips present considerable capability in lowering the consumption of samples and reagents. In addition, the bio-inspired wettability patterns offer an open system for integrating different detection methods, such as fluorescence spectroscopy, surface enhanced Raman scattering (SERS), colorimetry, and electrochemical mass spectrometry to achieve versatile and robust highly-sensitive detection of nucleic acids, proteins and small molecules.169–172
4.3.1 Detection in droplets.
Droplet arrays formed on the wettability patterns provide hundreds of individual droplets for high-throughput analysis which is usually involved in quantitative PCR assay and enzyme linked immunosorbent assay (ELISA). Yao et al. fabricated a hydrophilic-spot-patterned silicon chip with the spot diameter of 200 μm and pitch of 800 μm using photolithography-assisted wet etching. The authors printed droplet arrays containing highly diluted cell suspension, cell lysis buffer, reverse transcription mix and PCR mix onto an oil-covered microchip using a microfluidic robot step by step to perform reverse transcription quantitative PCR assay for single cells (Fig. 14a). The droplets were confined to the hydrophilic areas and covered by oil to maintain droplet morphology and avoid evaporation during thermal cycles.173
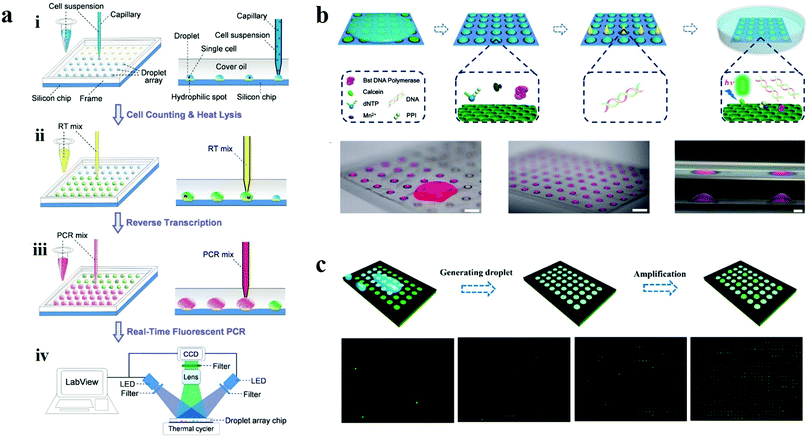 |
| Fig. 14 Droplet arrays based on wettability patterns for nucleic acid detection. (a) Scheme of the operational procedures of a droplet array system for single cell gene expression analysis. Reproduced with permission.173 Copyright 2015, Springer Nature. (b) Nucleic acid detection on a bio-inspired photonic nitrocellulose array. Scale bars are 1 cm, 1 cm and 1 mm, respectively. Reproduced with permission.174 Copyright 2018, Royal Society of Chemistry. (c) Microdroplet array for digital PCR. Reproduced with permission.141 Copyright 2018, American Chemical Society. | |
Actually, the ideal design of wettability patterns can realize automatic formation of droplets without the assistance of robots because of the different wettability. Liu et al. carried out a bio-inspired nitrocellulose array for ultrasensitive assays of nucleic acids (Fig. 14b). Inspired by the Stenocara beetle in the desert, a hydrophilic/hydrophobic patterned nitrocellulose chip with inverse opal structured spots was fabricated using a photonic crystal template and the following fluorination. The droplets of amplification reagents were formed by tilting the chip after applying reagents onto the wettability patterned chip for nucleic acid isothermal amplification. Benefiting from the fast reaction kinetics in the droplets and the fluorescence enhancement effect of the photonic crystal, the detection limit of nuclei acids of 0.60 copy per μL was obtained.174 The same group further proposed a novel strategy for digital PCR based on the wettability patterns (Fig. 14c).141 In this work, they prepared the wettability patterned chip by printing superhydrophobic silica nanoparticle doped toner onto a hydrophilic photonic nitrocellulose film with a designed array pattern. The patterned superhydrophobic print layer that covered on the hydrophilic film displayed 768 (32 × 24) hydrophilic spots for the formation of droplet arrays, during which the amplification reagents form hundreds of individual amplification systems and the nuclei acid samples were randomly divided into every droplet. After amplification, absolute quantification of nucleic acids could be obtained by calculation according to the positive spots and Poisson statistics.
The droplets formed on the wettability patterns provide microreactors or individual systems for bioassays, which can obviously reduce the consumption of costly reagents and samples, especially for some rare samples from clinical patients. Moreover, the smaller volume of the reaction system in a droplet compared to a tube leads to faster heating response and smaller diffusion distance, resulting in faster reaction kinetics than conventional detection methods in microplates.175 In addition, the mass transfer in a droplet is faster than that in a bulk solution due to the facilitated radial mixing by Marangoni convection inside the droplet, which can further accelerate the reaction rate.176 However, there are still some technological difficulties to overcome, such as the evaporation of the droplets during the detection, especially the detection involving heating with high temperature. Some effective measures have been devoted to maintaining the integrity of the droplets, like immersing into oil or encapsulating into a chip.
4.3.2 Detection with enrichment on wettability patterns.
The difference of wettability between the (super)hydrophilic and (super)hydrophobic patterns not only facilitates the formation of droplets or other liquid patterns but also confines the liquid to spread out from the patterns. Thus, the contents of the droplets will be concentrated on the hydrophilic spots along with the evaporation of droplets, which can effectively enrich analytes from a highly diluted sample. Taking advantage of the enrichment on wettability patterns, a lot of ultratrace detection methods have been proposed. Zhang and co-workers developed a superwettable microchip with superhydrophilic/superhydrophobic micropatterns for microRNA-141 (miR-141) detection.177 The superhydrophilic/superhydrophobic micropatterns were fabricated by the deposition of candle soot and UV-irradiation of the n-octadecyltrichlorosilane modified superhydrophobic nanodendritic silica coating via a mask. On evaporation, the target microRNA in the solution was concentrated and captured in the superhydrophilic spots. In addition, by integrating the aggregation-induced emission (AIE) molecules onto the probe, an ultra-low detection limit of 1 pM can be obtained with a higher signal-to-noise ratio, more excellent reproducibility, and higher sensitivity and specificity than the traditional fluorescent probes (Fig. 15a).
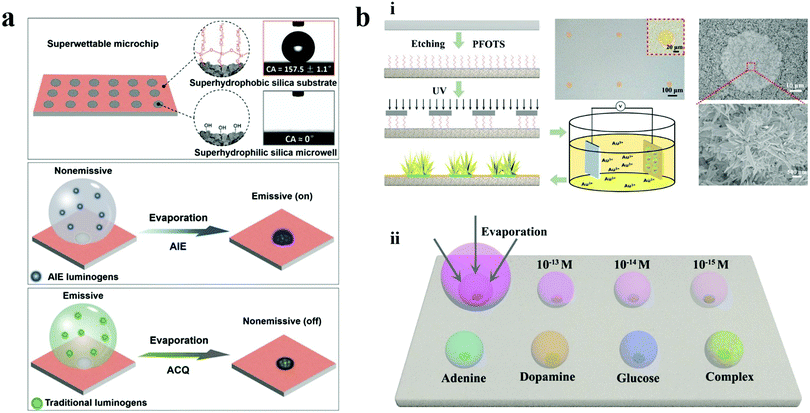 |
| Fig. 15 Enrichment strategy on wettability patterns for ultra-trace detections. (a) Superwettable microchip integrated with AIE for ultra-trace fluorescence detection of microRNA. Reproduced with permission.177 Copyright 2018, Elsevier B.V. (b) Bio-inspired micropatterned superhydrophilic Au-areoles for SERS detection. Reproduced with permission.178 Copyright 2018, Wiley-VCH. | |
In addition to the detection through probes, the wettability patterns can also achieve label-free ultratrace detection. Song et al. reported a bio-inspired micropatterned superhydrophilic Au-areoles for SERS detection (Fig. 15b).178 The Au-areoles were facilely and controllably fabricated through selective electrochemical deposition on the superhydrophilic areas of the patterned superhydrophilic/superhydrophobic substrate. The bio-wettability pattern facilitated the analytes to be concentrated on the superhydrophilic sites. Meanwhile, the Au-areoles mimicked the areole on the cactus and provided a lot of SERS hot spots benefiting from the large amounts of sharp edges, tips and coupled branches. The combination of these two effective strategies endowed the micropatterned chips with the capability of ultrasensitivity, Raman detection activity and multiple detection. Trace detection of adenine, dopamine, glucose, and rhodamine was achieved at the concentration of 10−12, 10−12 and 10−8 M, respectively. Moreover, the detection of analytes as low as pico-M or femto-M has also been demonstrated by detecting Rhodamine 6G at a concentration of 10−15 M.
By integrating the enrichment effect of the wettability patterns with other signal amplification strategies, rapid, multiplexed, high-throughput and highly-sensitive detections that possess great potential in the development of bioassays, clinic diagnosis, national security and other related fields can be achieved.144,179
4.3.3 Patterned paper chip.
The appearance of microfluidic paper-based analytical devices (μPADs) promoted the development and application of portable analytical devices especially for point-of-care testing (POCT). The paper chip was firstly proposed by Whitesides’ group in 2007 (Fig. 16a).179 A piece of chromatography paper with a diameter of 7.5 cm was soaked in SU-8 2010 photoresist and then exposed to 405 nm UV light through a mask to prepare patterns. The hydrophobic photoresist constituted defined areas of hydrophilic paper with the patterns of channels and reservoirs. These patterns provided spatial control of fluids by capillary action produced from paper which could be utilized for the transport of liquid samples and reagents during bioassays. They demonstrated this concept by detecting glucose and protein using this colorimetric method, which showed comparable sensitivity compared to commercial dipstick assays. Since then, a number of research studies focusing on the paper chip have been presented for portable, low-cost and on-site tests.180–187 For example, Crooks and Liu creatively fabricated 3D-μPADs assembled using the principles of origami (Fig. 16b).188 The patterns were fabricated on a single sheet of flat paper using photolithography. After folding, the patterned paper could form several-layered 3D-μPADs with the ability to direct the flow of fluids in three dimensions to perform parallel detections.
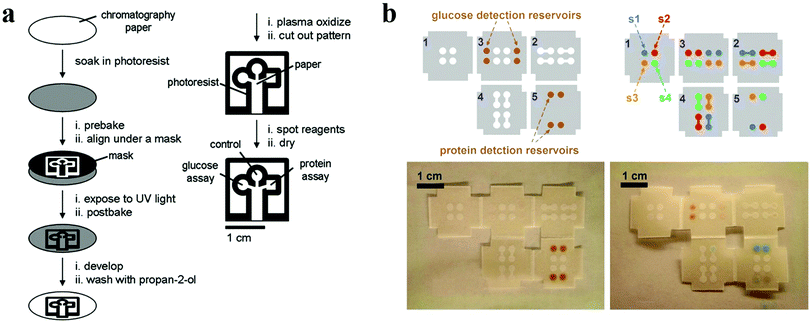 |
| Fig. 16 Paper chips with wettability patterns for bioassays. (a) Schematic diagrams showing the procedures for fabricating patterned paper chips for bioassays. Reproduced with permission.179 Copyright 2007, Wiley-VCH. (b) 3D origami paper chip. Reproduced with permission.188 Copyright 2011, American Chemical Society. | |
Derived from the paper chip, other emerging devices with wettability patterns fabricated using cloth, nitrocellulose and thread have also been developed for low-cost detections.189–193 Benefiting from the capillary action of these materials, the as-prepared devices eliminate the need of a pumping system, thus simplifying the operation procedure as well as reducing the cost. With the help of hydrophobic barriers formed by photoresist or wax, liquids including samples and reagents can be confined within the designed patterns without spread. Furthermore, the μPADs integrated with various output approaches can also offer considerable sensitivity to satisfy the clinical diagnosis. Thus, the μPADs with wettability patterns possess great potential for biosensing.
4.4 Other applications of gradient wettability surface
In addition to wettability patterns with well-defined hydrophilic and hydrophobic regions, surfaces possessing anisotropic wettability also provide excellent platforms for biomedical applications. Surface wettability gradient can mediate cell adhesion. In order to systematically investigate this response, Wang and co-workers demonstrated an adhesive spectrum of cells to a substrate surface with gradient wettability (from superhydrophilic to superhydrophobic state). The substrate was obtained by oblique deposition of candle soot and subsequent chemical modification. After co-cultivation with NIH/3T3 cells, the state of cell adhesion was characterized using immunofluorescent staining. It was found that cells were more prone to adhere on the superhydrophilic and superhydrophobic areas wetted by medium. Low adhesion was observed on the hydrophilic, hydrophobic and nonwetted superhydrophobic areas. In particular, the nonwetted superhydrophobic surface exhibited cell repellence, which was probably caused by the trapped air in the gaps of the nanodendrite.194 The results presented valuable information for the study of cell behaviours, which were instructive for applications in regenerative medicine by promoting tissue regeneration and wound healing. For example, newly generated cells adhering on a patch tend to migrate along the surface of the patch, which contributes to wound closure. Combined with ingenious design and elaborate fabrication, the wettability gradient materials would be promising in complex wound repair.
Anisotropic wettability existing in creatures in nature can fulfil directional liquid transport to realize diverse functions, such as self-cleaning in rice leaves and dewetting in butterfly wings. Owing to the one-dimensional order arrangement of nano/microstructures, the water droplets can roll off along a certain direction more easily. Inspired by the natural anisotropic wettability, surfaces with the capability of directional manipulation of liquids are proposed. For example, a wearable polymer nanofiber mat was proposed by Pisignano et al., which exhibited the properties of optical gain and anisotropic wettability. The fabricated mat composed of parallelly aligned nanofibers favored the directional movement of the liquid droplet, which was attributed to the pinning effects of the anisotropic microstructures. Benefiting from the optical gain effect of the materials, such mats showed great potential for the construction of wearable lab-on-chip platforms and smart wearable devices (Fig. 17).195
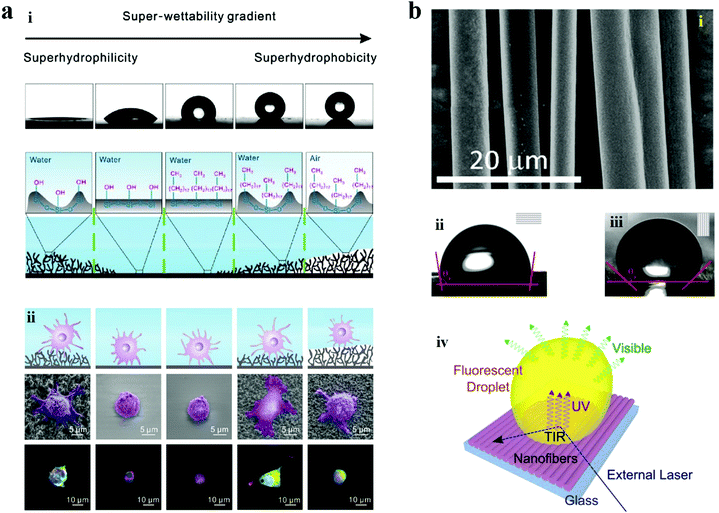 |
| Fig. 17 Application of anisotropic wettability surfaces. (a) Characterization of the gradient wettability surface: (i) contact angles and the corresponding surface structures at different areas of the gradient wettability surface. From left to right: superhydrophilic, hydrophilic, hydrophobic, wetted superhydrophobic, and nonwetted superhydrophobic.(ii) The state of cell adhesion on the different areas of the gradient wettability surface. Reproduced with permission.194 Copyright 2017, Science China Press and Springer-Verlag Berlin Heidelberg. (b) (i) SEM image of the parallelly aligned fibers. (ii and iii) Water contact angle measured along the direction parallel to the aligned fibers (ii) and perpendicular to the aligned fibers (iii). (iv) Schematics of a fluorescent droplet stimulated using laser light on a substrate with anisotropic wettability. Reproduced with permission.195 Copyright 2015, American Chemical Society. | |
Diversiform combination of different wettability in nature forms wettability patterns and thus creates novel features compared to the single-type wettability. Inspired by these wettability patterns, researchers fabricated an abundance of versatile surfaces for manipulating liquids. The liquid environment provides a relatively independent system which is essential for most biomedical research studies. The bio-inspired wettability patterns offer a strategy to guide the liquid through the well-designed channels towards the reservoirs or generate a mass of individual (micro)droplets completely dependent on the wettability difference, eliminating external complicated driving forces and repetitive liquid transfer, which are inevitable for traditional platforms in the field of biomedicine. Benefiting from the excellent performance in liquid manipulation of bio-inspired wettability patterns, cell culture with great convenience, drug screening with high throughput and analyte detection with ultra-high sensitivity have been achieved. Moreover, the wettability patterns with micro scale can effectively cut down the cost by removing costly facilities or reduce the consumption of reagents in microdroplets. Thus, the bio-inspired wettability patterns offer a versatile platform for biomedical applications.
5. Summary and perspective
In this report, we have summarized recent progress of bio-inspired wettability patterns, including the surface wettability in natural organisms, the general design principles and fabrication methods for special wettability surfaces, patterned wettability phenomena and fabrication approaches, and their biomedical applications. Benefiting from the in-depth fundamental study and continuous technical innovations, fabrication of artificial wettability materials is no longer restricted to single-type wettability (i.e. hydrophilic or hydrophobic) features. Efforts have been made on creating bio-inspired patterned wettability surfaces, where different regions show opposite or gradient wettability features. These materials possess a heterogeneous environment and allow for flexible liquid manipulation including droplet wetting/dewetting, confinement, patterning, and dynamic movement. Thus, this article sheds new light on various biomedical applications including cell culture, in situ reaction, highly-sensitive analysis, etc.
Except for the achievements discussed above, some other aspects in the biomedicine field would also bring novel techniques in combination with patterned wettability surfaces. Substrates with gradient wettability or patterned wettability are conducive to mediating cell migration and stem cell differentiation, which would shed new light on regenerative medicine. Oil–water separation is one of the most important applications of wettability materials, which can be probably utilized for lipid absorption and blood purification. Benefiting from the patterned wettability or anisotropic wettability, the separation can be easily realized without external driving force, which eliminates bulk facilities and decreases medical costs. In addition, the active transport of liquids based on anisotropic wettability can, with great expectation, be integrated with medical devices for body fluid drainage.
Despite the inspiring and remarkable achievements, several crucial challenges still remain, which construct a gap between the proof-of-concept laboratory research studies and actual applications.196–198 Here, we propose the following issues and possible improvements for future endeavors. The first issue is the long-term stability of wettability patterns, which mainly depends on the fabrication of hierarchical structures and surface modification. On the one hand, the materials for fabricating hierarchical structures should be stable and durable so that the structures can be preserved for a long time even when they suffer from severe external force or harsh environmental conditions. On the other hand, appropriate and passivated surface modification methods should be chosen. For example, the O2 plasma treatment can graft hydroxyl groups onto the surface of a given material, while the efficient hydrophilicity in the surface can only be maintained for several days after treatment. Some other methods can also be followed to improve the permanency of modified surface wettability, such as hydrogel solidification, particle assembly, and silanization.139,144,199 Thus, wettability patterns with long-term stability under excessive and complicated biomedical conditions can be expected in the future study.
Secondly, close attention should be paid to the uniformity of the patterns, which is vital for their applications since it directly impacts the geometry and size of droplets as well as the precise controllability of liquids. This usually depends on the manufacturing technique of patterning, such as resolution of the lithography machine, exposure mode, wavelength of light source, intensity homogeneity of light and quality of mask in the lithography method. Droplets with uniform sizes are especially significant in the quantitative detection, which may distinctly affect the clinical diagnosis.
The third concern is the fabrication methods for the wettability pattern. Nowadays, the current wettability patterns are relatively simple. It is of great desire to fabricate gradient wettability patterns with spatiotemporal variation. In addition, an increasing number of advanced fabrication techniques have been proposed for intelligent manufacturing, such as 4D printing through which the component is prepared by 3D printing but possesses the capability of transformation in shape and property. By integrating with the 4D printing technique, the wettability patterns would be controllably changed by external stimulations, which can realize intelligent, accurate and programmed control of the liquid as well as the associated applications.
In addition to these issues mentioned above, there still remain some defects that need to be resolved, especially for commercial-scale manufacturing. First, the elaborate microstructures can be realized only in the lab or on a small scale. Applicable industrial methods should be developed for large scale fabrication of highly uniform hierarchical structures. Besides, surface modification methods with the capability of quantity production and good repeatability are necessary for preparing wettability patterns. Moreover, natural substrates with special wettability (e.g., butterfly wings) or cheap materials need exploring to reduce the costs of commercial products. Furthermore, evaporation of liquid, which is a common problem for handling small volume liquids,200 should also be taken into account. Predictably, with further efforts, bio-inspired wettability patterns will be reliable in commercial-scale manufacturing for practical applications.
Conflicts of interest
There are no conflicts to declare.
Acknowledgements
This work was supported by the National Key Research and Development Program of China (2020YFA090035), the National Natural Science Foundation of China (grants 61927805), the Natural Science Foundation of Jiangsu (Grant no. BE2018707), and the China Postdoctoral Science Foundation funded project (2019M663090).
References
- T. Young, Philos. Trans. R. Soc. London, 1805, 95, 65 CrossRef
.
- H. Ollivier, Ann. Chim. Phys., 1907, 10, 289 Search PubMed
.
- H. Ollivier, Ann. Chim. Phys., 1907, 10, 229 Search PubMed
.
- F. E. Bartell and J. W. Shepard, J. Phys. Chem., 1953, 57, 211 CrossRef CAS
.
- W. Barthlott and C. Neinhuis, Planta, 1997, 202, 1 CrossRef CAS
.
- T.-S. Dinh Le, J. An, Y. Huang, Q. Vo, J. Boonruangkan, T. Tran, S.-W. Kim, G. Sun and Y.-J. Kim, ACS Nano, 2019, 13, 13293 CrossRef CAS
.
- Z. Han, Z. Wang, B. Li, X. Feng, Z. Jiao, J. Zhang, J. Zhao, S. Niu and L. Ren, ACS Appl. Mater. Interfaces, 2019, 11, 17019 CrossRef CAS
.
- Y. Liu, Z. Liu, Y. Liu, H. Hu, Y. Li, P. Yan, B. Yu and F. Zhou, Small, 2015, 11, 426 CrossRef CAS
.
- Q. Wu, G.-E. Chen, W.-G. Sun, Z.-L. Xu, Y.-F. Kong, X.-P. Zheng and S.-J. Xu, Chem. Eng. J., 2017, 313, 450 CrossRef CAS
.
- R.-X. Zhang, L. Braeken, T.-Y. Liu, P. Luis, X.-L. Wang and B. Van der Bruggen, Appl. Sci., 2017, 7, 81 CrossRef
.
- Y.-L. Ji, Q.-F. An, Y.-S. Guo, W.-S. Hung, K.-R. Lee and C.-J. Gao, J. Mater. Chem. A, 2016, 4, 4224 RSC
.
- S. Sunny, G. Cheng, D. Daniel, P. Lo, S. Ochoa, C. Howell, N. Vogel, A. Majid and J. Aizenberg, Proc. Natl. Acad. Sci. U. S. A., 2016, 113, 11676 CrossRef CAS
.
- Z. Sun, T. Liao, K. Liu, L. Jiang, J. H. Kim and S. X. Dou, Small, 2014, 10, 3001 CrossRef CAS
.
- Z. Sun, T. Liao, W. Li, Y. Dou, K. Liu, L. Jiang, S.-W. Kim, J. H. Kim and S. X. Dou, NPG Asia Mater., 2015, 7, e232 CrossRef CAS
.
- S. Zhang, J. Huang, Y. Cheng, H. Yang, Z. Chen and Y. Lai, Small, 2017, 13, 1701867 CrossRef
.
- J. Lv, Y. Song, L. Jiang and J. Wang, ACS Nano, 2014, 8, 3152 CrossRef CAS
.
- X. Li, G. Wang, A. S. Moita, C. Zhang, S. Wang and Y. Liu, Appl. Surf. Sci., 2020, 505, 144386 CrossRef CAS
.
- M. J. Kreder, J. Alvarenga, P. Kim and J. Aizenberg, Nat. Rev. Mater., 2016, 1, 15003 CrossRef CAS
.
- M. Cui, P.-Y. Wang, Z. Wang and B. Wang, Coatings, 2019, 9, 725 CrossRef CAS
.
- Y. Zhan, J. Zhang, X. Wan, Z. Long, S. He and Y. He, Appl. Surf. Sci., 2018, 436, 756 CrossRef CAS
.
- H. Zhang, L. Yin, X. Liu, R. Weng, Y. Wang and Z. Wu, Appl. Surf. Sci., 2016, 380, 178 CrossRef CAS
.
- T. Ishizaki, N. Saito and O. Takai, Langmuir, 2010, 26, 8147 CrossRef CAS
.
- N. Suvindran, F. Li, Y. Pan and X. Zhao, Adv. Mater. Interfaces, 2018, 5, 1800723 CrossRef
.
- H. Chen, T. Ran, Y. Gan, J. Zhou, Y. Zhang, L. Zhang, D. Zhang and L. Jiang, Nat. Mater., 2018, 17, 935 CrossRef CAS
.
- S. Zhang, J. Huang, Z. Chen and Y. Lai, Small, 2017, 13, 1602992 CrossRef
.
- C. Yang, X. Huang, Q. Jin, C. Yang, D. Liu, X. Li, L. Zhou, J. Li, T. Zhang, D. Xia, M. Li, X. Xie and H.-J. Chen, Nanotechnology, 2020, 31, 095712 CrossRef CAS
.
- S. Zhang, F. Lu, L. Tao, N. Liu, C. Gao, L. Feng and Y. Wei, ACS Appl. Mater. Interfaces, 2013, 5, 11971 CrossRef CAS
.
- X. Zhang, Z. Li, K. Liu and L. Jiang, Adv. Funct. Mater., 2013, 23, 2881 CrossRef CAS
.
- A. R. Rocha, V. M. García-suárez, S. W. Bailey, C. J. Lambert, J. Ferrer and S. Sanvito, Nat. Mater., 2005, 4, 355 CrossRef
.
- G. D. Bixler, A. Theiss, B. Bhushan and S. C. Lee, J. Colloid Interface Sci., 2014, 419, 114 CrossRef CAS
.
- R. Wang, K. Hashimoto, A. Fujishima, M. Chikuni, E. Kojima, A. Kitamura, M. Shimohigoshi and T. Watanabe, Nature, 1997, 388, 431 CrossRef CAS
.
- X. Sun, V. G. Damle, S. Liu and K. Rykaczewski, Adv. Mater. Interfaces, 2015, 2, 1400479 CrossRef
.
- H. Zhu, W. Hu, S. Zhao, X. Zhang, L. Pei, G. Zhao and Z. Wang, J. Mater. Sci., 2020, 55, 2215 CrossRef CAS
.
- L. Shang, Y. Wang, Y. Yu, J. Wang, Z. Zhao, H. Xu and Y. Zhao, J. Mater. Chem. A, 2017, 5, 15026 RSC
.
- Z. Xue, S. Wang, L. Lin, L. Chen, M. Liu, L. Feng and L. Jiang, Adv. Mater., 2011, 23, 4270 CrossRef CAS
.
- L. Sun, F. Bian, Y. Wang, Y. Wang, X. Zhang and Y. Zhao, Proc. Natl. Acad. Sci. U. S. A., 2020, 117, 4527 CrossRef CAS
.
- A. R. Parker and C. R. Lawrence, Nature, 2001, 414, 33 CrossRef CAS
.
- T. G. Fernandes, M. M. Diogo, D. S. Clark, J. S. Dordick and J. M. S. Cabral, Trends Biotechnol., 2009, 27, 342 CrossRef CAS
.
- C. M. Dumont, P. Karande and D. M. Thompson, Tissue Eng., Part C, 2014, 20, 620 CrossRef CAS
.
- B. A. Justice, N. A. Badr and R. A. Felder, Drug Discovery Today, 2009, 14, 102 CrossRef CAS
.
- A. Ranga, S. Gobaa, Y. Okawa, K. Mosiewicz, A. Negro and M. P. Lutolf, Nat. Commun., 2014, 5, 4324 CrossRef CAS
.
- F. Xu, J. Wu, S. Wang, N. G. Durmus, U. A. Gurkan and U. Demirci, Biofabrication, 2011, 3, 034101 CrossRef
.
- S. Zhao, H. Zhao, X. Zhang, Y. Li and Y. Du, Lab Chip, 2013, 13, 2350 RSC
.
- A. K. Gaharwar, A. Arpanaei, T. L. Andresen and A. Dolatshahi-Pirouz, Adv. Mater., 2016, 28, 771 CrossRef CAS
.
- R. Fang, M. Liu, H. Liu and L. Jiang, Adv. Mater. Interfaces, 2018, 5, 1701176 CrossRef
.
- F. Zhang, Q. Zhang, X. Li, N. Huang, X. Zhao and Z. Yang, Biomaterials, 2019, 194, 117 CrossRef CAS
.
- L. Liu, X. Tian, Y. Ma, Y. Duan, X. Zhao and G. Pan, Angew. Chem. Int. Ed., 2018, 57, 7878 CrossRef CAS
.
- Y. Hu, J.-O. You and J. Aizenberg, ACS Appl. Mater. Interfaces, 2016, 8, 21939 CrossRef CAS
.
- B. D. Hatton and J. Aizenberg, Nano Lett., 2012, 12, 4551 CrossRef CAS
.
- B. Su, Y. Tian and L. Jiang, J. Am. Chem. Soc., 2016, 138, 1727 CrossRef CAS
.
- S. Yang, J. Ju, Y. Qiu, Y. He, X. Wang, S. Dou, K. Liu and L. Jiang, Small, 2014, 10, 294 CrossRef CAS
.
- S. Mattaparthi and C. S. Sharma, Bioinspired, Biomimetic Nanobiomater., 2014, 3, 4 CrossRef CAS
.
- X. F. Gao and L. Jiang, Nature, 2004, 432, 36 CrossRef CAS
.
- S.-H. Hong, J. Hwang and H. Lee, Nanotechnology, 2009, 20, 385303 CrossRef
.
- Z. Guo and W. Liu, Plant Sci., 2007, 172, 1103 CrossRef CAS
.
- G. D. Bixler and B. Bhushan, Soft Matter, 2012, 8, 11271 RSC
.
- Y. Zheng, X. Gao and L. Jiang, Soft Matter, 2007, 3, 178 RSC
.
- L. Feng, S. H. Li, Y. S. Li, H. J. Li, L. J. Zhang, J. Zhai, Y. L. Song, B. Q. Liu, L. Jiang and D. B. Zhu, Adv. Mater., 2002, 14, 1857 CrossRef CAS
.
- H. Chen, P. Zhang, L. Zhang, H. L. Iu, Y. Jiang, D. Zhang, Z. Han and L. Jiang, Nature, 2016, 532, 85 CrossRef CAS
.
- X. Zheng, Z. Guo, D. Tian, X. Zhang, W. Li and L. Jiang, ACS Appl. Mater. Interfaces, 2015, 7, 4336 CrossRef CAS
.
- X. L. Liu, J. Zhou, Z. X. Xue, J. Gao, J. X. Meng, S. T. Wang and L. Jiang, Adv. Mater., 2012, 24, 3401 CrossRef CAS
.
- P. Comanns, G. Buchberger, A. Buchsbaum, R. Baumgartner, A. Kogler, S. Bauer and W. Baumgartner, J. R. Soc., Interface, 2015, 12 Search PubMed
.
- M. Liu, S. Wang, Z. Wei, Y. Song and L. Jiang, Adv. Mater., 2009, 21, 665 CrossRef CAS
.
- M. H. Sun, C. X. Luo, L. P. Xu, H. Ji, O. Y. Qi, D. P. Yu and Y. Chen, Langmuir, 2005, 21, 8978 CrossRef CAS
.
- X. Zhang, L. Sun, Y. Wang, F. Bian, Y. Wang and Y. Zhao, Proc. Natl. Acad. Sci. U. S. A., 2019, 116, 20863 CrossRef CAS
.
- T. Kong, G. Luo, Y. Zhao and Z. Liu, Adv. Funct. Mater., 2019, 29, 1808012 CrossRef
.
- L. Shang, Y. Yu, W. Gao, Y. Wang, L. Qu, Z. Zhao, R. Chai and Y. Zhao, Adv. Funct. Mater., 2018, 28, 1705802 CrossRef
.
- Z. He, Y. Chen, J. Yang, C. Tang, J. Lv, Y. Liu, J. Mei, W.-M. Lau and D. Hui, Composites, Part B, 2017, 129, 58 CrossRef CAS
.
- J. Lv, Z. Gong, Z. He, J. Yang, Y. Chen, C. Tang, Y. Liu, M. Fan and W.-M. Lau, J. Mater. Chem. A, 2017, 5, 12435 RSC
.
- S. Yuan, J. Zhu, Y. Li, Y. Zhao, J. Li, P. Van Puyvelde and B. Van der Bruggen, J. Mater. Chem. A, 2019, 7, 2723 RSC
.
- G. Li, X. Mo, Y. Wang, C.-Y. Chan and K. C. Chan, Adv. Mater. Interfaces, 2019, 6, 1900874 CrossRef CAS
.
- R. Jafari, C. Cloutier, A. Allahdini and G. Momen, Int. J. Adv. Manuf. Technol., 2019, 103, 1225 CrossRef
.
- G.-L. Ying, N. Jiang, S. Mahar, Y.-X. Yin, R.-R. Chai, X. Cao, J.-Z. Yang, A. K. Miri, S. Hassan and Y. S. Zhang, Adv. Mater., 2018, 30, 1805460 CrossRef
.
- W. Wei, Y. Li, H. Yang, R. Nassab, F. Shahriyari, A. Akpek, X. Guan, Y. Liu, S. Taranejoo, A. Tamayol, Y. S. Zhang, A. Khademhosseini and H. L. Jang, Macromol. Biosci., 2017, 17, 1700304 CrossRef
.
- Y. S. Zhang, K. Yue, J. Aleman, K. Mollazadeh-Moghaddam, S. M. Bakht, J. Yang, W. Jia, V. Dell'Erba, P. Assawes, S. R. Shin, M. R. Dokmeci, R. Oklu and A. Khademhosseini, Ann. Biomed. Eng., 2017, 45, 148 CrossRef
.
- Y. Yan, H. Chen, H. Zhang, C. Guo, K. Yang, K. Chen, R. Cheng, N. Qian, N. Sandler, Y. S. Zhang, H. Shen, J. Qi, W. Cui and L. Deng, Biomaterials, 2019, 190, 97 CrossRef
.
- N. M. Oliveira, A. I. Neto, W. Song and J. F. Mano, Appl. Phys. Express, 2010, 3, 085205 CrossRef
.
- X. Liu, H. Gu, M. Wang, X. Du, B. Gao, A. Elbaz, L. Sun, J. Liao, P. Xiao and Z. Gu, Adv. Mater., 2018, 30, 1800103 CrossRef
.
- L. Dong, Z. Zhang, R. Ding, L. Wang, M. Liu, Z. Weng, Z. Wang and D. Li, Surf. Coat. Technol., 2019, 372, 434 CrossRef CAS
.
- Y. Yang, H. He, Y. Li and J. Qiu, Sci. Rep., 2019, 9, 9961 CrossRef
.
- F. Wang, L. Wang, H. Wu, J. Pang, D. Gu and S. Li, Colloids Surf., A, 2017, 520, 834 CrossRef CAS
.
- Y. Li, D. X. Luong, J. Zhang, Y. R. Tarkunde, C. Kittrell, F. Sargunaraj, Y. Ji, C. J. Arnusch and J. M. Tour, Adv. Mater., 2017, 29, 1700496 CrossRef
.
- H. Li, Y. Lai, J. Huang, Y. Tang, L. Yang, Z. Chen, K. Zhang, X. Wang and L. P. Tan, J. Mater. Chem. B, 2015, 3, 342 RSC
.
- L. Liang, L. Lu, D. Xing, Z. Wan and Y. Tang, Surf. Coat. Technol., 2019, 357, 57 CrossRef CAS
.
- A. Chaudhary and H. C. Barshilia, J. Phys. Chem. C, 2011, 115, 18213 CrossRef CAS
.
- H. S. Salapare, III, F. Guittard, X. Noblin, E. T. de Givenchy, F. Celestini and H. J. Ramos, J. Colloid Interface Sci., 2013, 396, 287 CrossRef
.
- Y. P. Li, S. Y. Li, W. Shi and M. K. Lei, Surf. Coat. Technol., 2012, 206, 4952 CrossRef CAS
.
- R. Jain and R. Pitchumani, Langmuir, 2018, 34, 3159 CrossRef CAS
.
- G. Zhao, Y. Xue, Y. Huang, Y. Ye, F. C. Walsh, J. Chen and S. Wang, RSC Adv., 2016, 6, 59104 RSC
.
- B. Zhang, X. Zhao, Y. Li and B. Hou, RSC Adv., 2016, 6, 35455 RSC
.
- S. Wang, K. Liu, X. Yao and L. Jiang, Chem. Rev., 2015, 115, 8230 CrossRef CAS
.
- X. Su, H. Li, X. Lai, L. Zhang, J. Wang, X. Liao and X. Zeng, ACS Appl. Mater. Interfaces, 2017, 9, 28089 CrossRef CAS
.
- X.-F. Zhang, R.-J. Chen, Y.-H. Liu and J.-M. Hu, J. Mater. Chem. A, 2016, 4, 649 RSC
.
- D. Lin, X. Zeng, H. Li, X. Lai and T. Wu, J. Colloid Interface Sci., 2019, 533, 198 CrossRef CAS
.
- J. Ju, X. Yao, X. Hou, Q. Liu, Y. S. Zhang and A. Khademhosseini, J. Mater. Chem. A, 2017, 5, 16273 RSC
.
- L. Feng, Y. Zhu, J. Wang and X. Shi, Appl. Surf. Sci., 2017, 422, 566 CrossRef CAS
.
- H. Wang, Q. Yao, C. Wang, B. Fan, Q. Sun, C. Jin, Y. Xiong and Y. Chen, Sci. Rep., 2016, 6, 35549 CrossRef
.
- C. Yang, Y. Huang, F. Li and T. Li, J. Mater. Sci., 2016, 51, 1032 CrossRef CAS
.
- Z. Huang, N. Geyer, P. Werner, J. de Boor and U. Goesele, Adv. Mater., 2011, 23, 285 CrossRef CAS
.
- X. Gou and Z. Guo, Adv. Colloid Interface Sci., 2019, 269, 87 CrossRef CAS
.
- S. Li, J. Huang, Z. Chen, G. Chen and Y. Lai, J. Mater. Chem. A, 2017, 5, 31 RSC
.
- J. D. Cox, M. S. Curry, S. K. Skirboll, P. L. Gourley and D. Y. Sasaki, Biomaterials, 2002, 23, 929 CrossRef CAS
.
- Q. Zhou, S. Xu, C. Zhu, B. Cao, F. Kausar, A. Xu, W. Z. Yuan and Y. Zhang, J. Mater. Sci., 2017, 52, 11737 CrossRef CAS
.
- M. Guo, B. Ding, X. Li, X. Wang, J. Yu and M. Wang, J. Phys. Chem. C, 2010, 114, 916 CrossRef CAS
.
- J. Zhou, A. V. Ellis and N. H. Voelcker, Electrophoresis, 2010, 31, 2 CrossRef CAS
.
- H. Wu, Q. Liu, W. Du, C. Li and G. Shi, ACS Appl. Mater. Interfaces, 2018, 10, 3895 CrossRef CAS
.
- T. I. Croll, A. J. O'Connor, G. W. Stevens and J. J. Cooper-White, Biomacromolecules, 2004, 5, 463 CrossRef CAS
.
- J. Araki, Soft Matter, 2013, 9, 4125 RSC
.
- S. H. Oh and J. H. Lee, Biomed. Mater., 2013, 8, 014101 CrossRef
.
- C. Schlaich, M. Li, C. Cheng, I. S. Donskyi, L. Yu, G. Song, E. Osorio, Q. Wei and R. Haag, Adv. Mater. Interfaces, 2018, 5, 1701254 CrossRef
.
-
Y. Tsujii, K. Ohno, S. Yamamoto, A. Goto and T. Fukuda, in Surface-Initiated Polymerization I, ed. R. Jordan, 2006, vol. 197, p. 1 Search PubMed
.
- S. G. Gaynor, J. S. Wang and K. Matyjaszewski, Macromolecules, 1995, 28, 8051 CrossRef CAS
.
- T. Yano, W. O. Yah, H. Yamaguchi, Y. Terayama, M. Nishihara, M. Kobayashi and A. Takahara, Polym. J., 2011, 43, 838 CrossRef CAS
.
- K. Park, Y. M. Ju, J. S. Son, K.-D. Ahn and D. K. Han, J. Biomater. Sci., Polym. Ed., 2007, 18, 369 CrossRef CAS
.
- R. S. Kurusu and N. R. Demarquette, Int. Mater. Rev., 2019, 64, 249 CrossRef CAS
.
- S. Duan, X. Xu, X. Liu, Y. Wang, T. Hayat, A. Alsaedi, Y. Meng and J. Li, J. Colloid Interface Sci., 2018, 513, 92 CrossRef CAS
.
- T. Wang, J. Liu, Y. Zhang, H. Zhang, W.-Y. Chen, P. Norris and W.-P. Pan, Chem. Eng. J., 2018, 331, 536 CrossRef CAS
.
- S. Balasubramanyam, M. Shirazi, M. A. Bloodgood, L. Wu, M. A. Verheijen, V. Vandalon, W. M. M. Kessels, J. P. Hofmann and A. A. Bol, Chem. Mater., 2019, 31, 5104 CrossRef CAS
.
- M. Kahoush, N. Behary, A. Cayla, B. Mutel, J. Guan and V. Nierstrasz, Appl. Surf. Sci., 2019, 476, 1016 CrossRef CAS
.
- J. J. Shao, W. Tang, T. Jiang, X. Y. Chen, L. Xu, B. D. Chen, T. Zhou, C. R. Deng and Z. L. Wang, Nanoscale, 2017, 9, 9668 RSC
.
- E. O. Kudasova, L. F. Vlasova, D. E. Semenov and E. L. Lushnikova, Bull. Exp. Biol. Med., 2017, 162, 687 CrossRef CAS
.
- J. Lee, S. Hwang, D.-H. Cho, J. Hong, J. H. Shin and D. Byun, Appl. Surf. Sci., 2017, 394, 543 CrossRef CAS
.
- D. M. Correia, C. Ribeiro, V. Sencadas, G. Botelho, S. A. C. Carabineiro, J. L. Gomes Ribelles and S. Lanceros-Mendez, Prog. Org. Coat., 2015, 85, 151 CrossRef CAS
.
- A. Martins, E. D. Pinho, S. Faria, I. Pashkuleva, A. P. Marques, R. L. Reis and N. M. Neves, Small, 2009, 5, 1195 CAS
.
- M. Schnabelrauch, R. Wyrwa, H. Rebl, C. Bergemann, B. Finke, M. Schlosser, U. Walschus, S. Lucke, K.-D. Weltmann and J. B. Nebe, Int. J. Polym. Sci., 2014, 2014, 439784 Search PubMed
.
- Y. H. Kang, K. Ahn, S. Y. Jeong, J. S. Bae, J. S. Jin, H. G. Kim, S. W. Hong and C. R. Cho, Thin Solid Films, 2011, 519, 7090 CrossRef CAS
.
- L. Tian, M. P. Prabhakaran, J. Hu, M. Chen, F. Besenbacher and S. Ramakrishna, RSC Adv., 2015, 5, 49838 RSC
.
- S. J. Cho, H. Nam, H. Ryu and G. Lim, Adv. Funct. Mater., 2013, 23, 5577 CrossRef CAS
.
- W. Gao, J. Wang, X. Zhang, L. Sun, Y. Chen and Y. Zhao, Chem. Eng. J., 2020, 381, 122612 CrossRef CAS
.
- J. Wang, W. Gao, H. Zhang, M. Zou, Y. Chen and Y. Zhao, Sci. Adv., 2018, 4, eaat7392 CrossRef
.
- X.-M. Li, D. Reinhoudt and M. Crego-Calama, Chem. Soc. Rev., 2007, 36, 1350 RSC
.
- W. Mi, S. Karlsson, A. Holmberg, M. Danielsson and P. Nillius, J. Micromech. Microeng., 2016, 26, 035001 CrossRef
.
- F. Palazon, Q. A. Akkerman, M. Prato and L. Manna, ACS Nano, 2016, 10, 1224 CrossRef CAS
.
- J. R. Maldonado and M. Peckerar, Microelectron. Eng., 2016, 161, 87 CrossRef CAS
.
- D.-L. Li, Z.-Y. Wen, Z.-G. Shang and Y. She, Optoelectron. Lett., 2016, 12, 182 CrossRef
.
- V. C. Pinto, P. J. Sousa, V. F. Cardoso and G. Minas, Micromachines, 2014, 5, 738 CrossRef
.
- P. Wu and C. Zhang, Lab Chip, 2015, 15, 1598 RSC
.
- M. Brehm, S. Heissler, S. Afonin and P. A. Levkin, Small, 2020, 16, 1905971 CrossRef CAS
.
- C. Shao, Y. Liu, J. Chi, Z. Chen, J. Wang and Y. Zhao, Langmuir, 2019, 35, 3832 CrossRef CAS
.
- D. Tian, Y. Song and L. Jiang, Chem. Soc. Rev., 2013, 42, 5184 RSC
.
- J. Chi, C. Shao, X. Du, H. Liu and Z. Gu, ACS Appl. Mater. Interfaces, 2018, 10, 39144 CrossRef CAS
.
- J. Hou, M. Z. Li and Y. L. Song, Angew. Chem. Int. Ed., 2018, 57, 2544 CrossRef CAS
.
- J. Hou, H. Zhang, Q. Yang, M. Li, Y. Song and L. Jiang, Angew. Chem. Int. Ed., 2014, 53, 5791 CrossRef CAS
.
- C. Shao, J. Chi, Z. Chen, L. Cai and Y. Zhao, J. Colloid Interface Sci., 2019, 546, 122 CrossRef CAS
.
- Y. Wang, M. Li, H. Li, Y. Lan, X. Zhou, C. Li, X. Hu and Y. Song, Adv. Energy Mater., 2019, 9, 1900838 CrossRef
.
- D. T. Nguyen, M. Freitag, C. Gutheil, K. Sotthewes, B. J. Tyler, M. Bockmann, M. Das, F. Schluter, N. L. Doltsinis, H. F. Arlinghaus, B. J. Ravoo and F. Glorius, Angew. Chem. Int. Ed., 2020, 59, 13651 CrossRef CAS
.
- M. Schmidt, M. Philippi, M. Muenzner, J. M. Stangl, R. Wieczorek, W. Harneit, K. Mueller-Buschbaum, D. Enke and M. Steinhart, Adv. Funct. Mater., 2018, 28, 1800700 CrossRef
.
- W. Wang, J. Salazar, H. Vahabi, A. Joshi-Imre, W. E. Voit and A. K. Kota, Adv. Mater., 2017, 29, 1700295 CrossRef
.
- T. Tischer, C. Rodriguez-Emmenegger, V. Trouillet, A. Welle, V. Schueler, J. O. Mueller, A. S. Goldmann, E. Brynda and C. Barner-Kowollik, Adv. Mater., 2014, 26, 4087 CrossRef CAS
.
- W. Feng, E. Ueda and P. A. Levkin, Adv. Mater., 2018, 30, 1706111 CrossRef
.
- E. Ueda, F. L. Geyer, V. Nedashkivska and P. A. Levkin, Lab Chip, 2012, 12, 5218 RSC
.
- A. A. Popova, S. M. Schillo, K. Demir, E. Ueda, A. Nesterov-Mueller and P. A. Levkin, Adv. Mater., 2015, 27, 5217 CrossRef CAS
.
- A. A. Popova, K. Demir, T. G. Hartanto, E. Schmitt and P. A. Levkin, RSC Adv., 2016, 6, 38263 RSC
.
- M. Lee, K. Yang, Y. H. Hwang, Y. Byun, D. Y. Lee, S.-W. Cho and H. Lee, Adv. Healthcare Mater., 2015, 4, 511 CrossRef CAS
.
- M. B. Oliveira, A. I. Neto, C. R. Correia, M. Isabel Rial-Hermida, C. Alvarez-Lorenzo and J. F. Mano, ACS Appl. Mater. Interfaces, 2014, 6, 9488 CrossRef CAS
.
- J. Seo, J. S. Lee, K. Lee, D. Kim, K. Yang, S. Shin, C. Mahata, H. B. Jung, W. Lee, S.-W. Cho and T. Lee, Adv. Mater., 2014, 26, 7043 CrossRef CAS
.
- O. N. Burchak, L. Mugherli, M. Ostuni, J. J. Lacapere and M. Y. Balakirev, J. Am. Chem. Soc., 2011, 133, 10058 CrossRef CAS
.
- F. Kong, L. Yuan, Y. F. Zheng and W. Chen, JALA, 2012, 17, 169 CAS
.
- A. I. Neto, K. Demir, A. A. Popova, M. B. Oliveira, J. F. Mano and P. A. Levkin, Adv. Mater., 2016, 28, 7613 CrossRef CAS
.
- W. Feng, L. Li, X. Du, A. Welle and P. A. Levkin, Adv. Mater., 2016, 28, 3202 CrossRef CAS
.
- K. Xu, X. Wang, R. M. Ford and J. P. Landers, Anal. Chem., 2016, 88, 2652 CrossRef CAS
.
- Y. Li, P. Chen, Y. Wang, S. Yan, X. Feng, W. Du, S. A. Koehler, U. Demirci and B.-F. Liu, Adv. Mater., 2016, 28, 3543 CrossRef CAS
.
- A. A. Popova, C. Depew, K. M. Permana, A. Trubitsyn, R. Peravali, J. A. G. Ordiano, M. Reischl and P. A. Levkin, SLAS Technol., 2017, 22, 163 Search PubMed
.
- G. E. Jogia, T. Tronser, A. A. Popova and P. A. Levkin, Microarrays, 2016, 5, 28 CrossRef
.
- H. Li, Q. Yang, G. Li, M. Li, S. Wang and Y. Song, ACS Appl. Mater. Interfaces, 2015, 7, 9060 CrossRef CAS
.
- N. Tong, J. Zhang, Y. Chen, Z. Li, Y. Luo, H. Zuo and X. Zhao, Oncol. Lett., 2012, 3, 1263 CrossRef CAS
.
- S. K. Wattanapitayakul, L. Chularojmontri, A. Herunsalee, S. Charuchongkolwongse, S. Niumsakul and J. A. Bauer, Basic Clin. Pharmacol. Toxicol., 2005, 96, 80 CrossRef CAS
.
- H. Sadeghi-Aliabadi, M. Minaiyan and A. Dabestan, Results Pharma Sci., 2010, 5, 127 CAS
.
- Y. Chen, L.-P. Xu, J. Meng, S. Deng, L. Ma, S. Zhang, X. Zhang and S. Wang, Biosens. Bioelectron., 2018, 102, 418 CrossRef CAS
.
- T. Xu, Y. Song, W. Gao, T. Wu, L.-P. Xu, X. Zhang and S. Wang, ACS Sens., 2018, 3, 72 CrossRef CAS
.
- T. Xu, W. Shi, J. Huang, Y. Song, F. Zhang, L.-P. Xu, X. Zhang and S. Wang, ACS Nano, 2017, 11, 621 CrossRef CAS
.
- L.-P. Xu, Y. Chen, G. Yang, W. Shi, B. Dai, G. Li, Y. Cao, Y. Wen, X. Zhang and S. Wang, Adv. Mater., 2015, 27, 6878 CrossRef CAS
.
- Y. Zhu, Y.-X. Zhang, W.-W. Liu, Y. Ma, Q. Fang and B. Yao, Sci. Rep., 2015, 5, 9551 CrossRef
.
- J. Chi, B. Ma, X. Dong, B. Gao, A. Elbaz, H. Liu and Z. Gu, Analyst, 2018, 143, 4559 RSC
.
- K. M. Koo, E. J. H. Wee, Y. Wang and M. Trau, Lab Chip, 2017, 17, 3200 RSC
.
- M. Wegener and A. R. Paschedag, Int. J. Multiphase Flow, 2011, 37, 76 CrossRef CAS
.
- Y. Chen, X. Min, X. Zhang, F. Zhang, S. Lu, L.-P. Xu, X. Lou, F. Xia, X. Zhang and S. Wang, Biosens. Bioelectron., 2018, 111, 124 CrossRef CAS
.
- H. Li, Q. Yang, J. Hou, Y. Li, M. Li and Y. Song, Adv. Funct. Mater., 2018, 28, 1800448 CrossRef
.
- A. W. Martinez, S. T. Phillips, M. J. Butte and G. M. Whitesides, Angew. Chem. Int. Ed., 2007, 46, 1318 CrossRef CAS
.
- J. Qi, B. Li, X. Wang, Z. Zhang, Z. Wang, J. Han and L. Chen, Sens. Actuators, B, 2017, 251, 224 CrossRef CAS
.
- B. Li, Z. Zhang, J. Qi, N. Zhou, S. Qin, J. Choo and L. Chen, ACS Sens., 2017, 2, 243 CrossRef CAS
.
- K. N. Han, J.-S. Choi and J. Kwon, Sci. Rep., 2017, 7, 2806 CrossRef
.
- S.-W. Hu, S. Qiao, B.-Y. Xu, X. Peng, J.-J. Xu and H.-Y. Chen, Anal. Chem., 2017, 89, 2131 CrossRef CAS
.
- M. S. Khan, S. K. Misra, Z. Wang, E. Daza, A. S. Schwartz-Duval, J. M. Kus, D. Pan and D. Pan, Anal. Chem., 2017, 89, 2107 CrossRef CAS
.
- N. Ruecha, J. Lee, H. Chae, H. Cheong, V. Soum, P. Preechakasedkit, O. Chailapakul, G. Tanev, J. Madsen, N. Rodthongkum, O.-S. Kwon and K. Shin, Adv. Mater. Technol., 2017, 2, 1600267 CrossRef
.
- B. Li, X. Zhou, H. Liu, H. Deng, R. Huang and D. Xing, ACS Appl. Mater. Interfaces, 2018, 10, 4494 CrossRef CAS
.
- A. K. Yetisen, N. Jiang, A. Tamayol, G. U. Ruiz-Esparza, Y. S. Zhang, S. Medina-Pando, A. Gupta, J. S. Wolffsohn, H. Butt, A. Khademhosseini and S.-H. Yun, Lab Chip, 2017, 17, 1137 RSC
.
- H. Liu and R. M. Crooks, J. Am. Chem. Soc., 2011, 133, 17564 CrossRef CAS
.
- T. Wu, T. Xu, L.-P. Xu, Y. Huang, W. Shi, Y. Wen and X. Zhang, Biosens. Bioelectron., 2016, 86, 951 CrossRef CAS
.
- W. Guan, M. Liu and C. Zhang, Biosens. Bioelectron., 2016, 75, 247 CrossRef CAS
.
- J. Chi, B. Gao, M. Sun, F. Zhang, E. Su, H. Liu and Z. Gu, Anal. Chem., 2017, 89, 7727 CrossRef CAS
.
- B. Gao, H. Liu and Z. Gu, Anal. Chem., 2016, 88, 5424 CrossRef CAS
.
- B. Gao, H. Liu and Z. Gu, Langmuir, 2014, 30, 15041 CrossRef CAS
.
- J. Meng, Y. Gao, L. Liu, Y. Song, L. Jiang and S. Wang, Sci. China: Chem., 2017, 60, 614 CrossRef CAS
.
- G. Morello, R. Manco, M. Moffa, L. Persano, A. Camposeo and D. Pisignano, ACS Appl. Mater. Interfaces, 2015, 7, 21907 CrossRef CAS
.
- H. Chen, T. Sun, Y. Yan, X. Ji, Y. Sun, X. Zhao, J. Qi, W. Cui, L. Deng and H. Zhang, Biomaterials, 2020, 242, 119931 CrossRef CAS
.
- M. Mao, H. P. Bei, C. H. Lam, P. Chen, S. Wang, Y. Chen, J. He and X. Zhao, Small, 2020, 16, 2000546 CrossRef CAS
.
- F. Wu, S. Chen, B. Chen, M. Wang, L. Min, J. Alvarenga, J. Ju, A. Khademhosseini, Y. Yao, Y. S. Zhang, J. Aizenberg and X. Hou, Small, 2018, 14, 1702170 CrossRef
.
- A. C. Glavan, R. V. Martinez, A. B. Subramaniam, H. J. Yoon, R. M. D. Nunes, H. Lange, M. M. Thuo and G. M. Whitesides, Adv. Funct. Mater., 2014, 24, 60 CrossRef CAS
.
- E. Berthier, J. Warrick, H. Yu and D. J. Beebe, Lab Chip, 2008, 8, 852 RSC
.
|
This journal is © The Royal Society of Chemistry 2021 |