DOI:
10.1039/C6RA03343A
(Paper)
RSC Adv., 2016,
6, 40399-40407
Novel hydrothermal synthesis of MoS2 nanocluster structure for sensitive electrochemical detection of human and environmental hazardous pollutant 4-aminophenol
Received
4th February 2016
, Accepted 12th April 2016
First published on 13th April 2016
Abstract
Herein, we have demonstrated a new electrochemical sensor for trace level detection of environmentally hazardous 4-aminophenol (4-AP) using a glassy carbon electrode (GCE) modified with MoS2 nanoclusters. The MoS2 nanoclusters were fabricated by a simple hydrothermal treatment without using any other organic templates or surfactants. The formation of MoS2 nanoclusters was confirmed by X-ray diffraction, FT-infrared, Raman and energy dispersive X-ray spectroscopies, scanning electron and transmission electron microscopies and selected area electron diffraction studies. The MoS2 modified GCE (MoS2/GCE) shows good electrocatalytic activity towards the redox reaction of 4-AP by means of cyclic voltammetry and differential pulse voltammetry. The DPV detection of 4-AP using MoS2/GCE delivers excellent sensitivity with a low detection limit of 0.03 (±0.008) μM and good linearity in the range of 0.04–17 μM. The sensitivity of the developed electrode is 4.278 (±0.058) μA mM−1 cm−2. The developed sensor displayed good repeatability, reproducibility and selectivity. Moreover, the practical applicability of the MoS2/GCE is demonstrated in water samples which delivered satisfactory recoveries.
1. Introduction
Aminophenols are popularly known organic compounds present in three isomeric forms especially 4-aminophenol (p-aminophenol; 4-AP) which has been widely used as a starting material and also as an intermediate product in various chemical syntheses. In pharmaceutical preparations of paracetamol, 4-AP was obtained as the primary hydrolytic degradation product or synthetic intermediate and it can be dangerous to living things causing teratogenic effects and nephrotoxicity.1 The United States of Pharmacopeia listed the high concentration limit of 4-AP in pharmaceuticals to be 50 ppm (0.005%, w/w).2 4-AP is a moderately toxic substance to the human body which causes irritation to the eyes, skin, and respiratory system. It is an environmental pollutant as well because of its high release in petroleum additives, chemical inhibitors, dye stuff and developer manufacturing industries.3–5 It has a great ability to cause methemoglobinemia due to its nephrotoxicity upon animal studies.6 Therefore, trace level detection of 4-AP in the surrounding substances is essential. Numbers of methods were used for the determination of 4-AP such as HPLC method,7,8 capillary electrophoresis with amphoteric detection method,9 micellar electro kinetic chromatography,1 1H nuclear magnetic resonance spectroscopy analysis10 and flow injection analysis with spectrophotometric detection.11 Instead of the above mentioned methods, electrochemical methods are more preferable because their low-cost, simplicity, rapid detection, high sensitivity and selectivity.
Recently, two-dimensional layered inorganic materials such as molybdenum disulfide (MoS2) has received remarkable attention due to their unique physical, chemical, electronic properties, inexpensive and easily available, low-cost, abundant availability and excellent electrocatalytic properties.12–14 MoS2 sheets have been applied in various applications such as optoelectronic devices,15 lithium ion batteries,16–18 sodium ion batteries,19 dye-sensitized solar cells,20,21 as interlayer in organic photovoltaics22,23 and hydrogen evolution reactions.24,25 Besides, MoS2 have outstanding electronic features of high on/off ratio, high charge-carrier mobility and tunable charge-carrier types.26,27 MoS2 nanosheets supported semiconductor devices, such as digital circuits28 and field effect transistors29,30 have been fabricated. In addition, MoS2 based materials have particularly well-defined spin-spitting property and these properties suitable for the application of spintronics.6,31 Also, MoS2 and their composite materials have been used for the detection of toxic and biologically active compounds.32–36 Amongst these applications, the convenience of MoS2 in the electrochemical field has been focused on two aspects: electrocatalysts and supercapacitors.37,38 In effect, it may possibly apply in the field of electrochemical sensors, owing to its large surface area and potential for surface modifications.39,40 Remarkably, a mixture of MoS2-supported composites, such as MoS2/graphene and MoS2/polyaniline hybrid materials were successfully developed which manifesting their good conductivity, high surface area and improved electrochemical stability.41,42 These interesting properties of MoS2 and MoS2 based composites make them attractive materials for electrochemical applications.
Hence, we made an attempt to synthesize MoS2 nanoclusters by simple hydrothermal route without using any other organic surfactant or template. The MoS2 nanoclusters were characterized using various analytical tools. The electrochemical behavior of MoS2 film modified electrode towards determination of 4-AP was investigated by cyclic voltammetry and differential pulse voltammetry. The experimental parameters that affect the analysis of 4-AP, such as pH of the medium, scan rate and interfering compounds were investigated and optimized. The fabricated 4-AP sensor based on MoS2 shows good electrocatalytic activity towards electrochemical detection of 4-AP. The sensor proves its good selectivity in the presence of various common metal ions and biologically co-active interfering species. To the best of our knowledge, this is the first report in which MoS2 modified GCE to detection of 4-AP.
2. Experimental
2.1 Chemicals and apparatus
Sodium molybdate dihydrate (MoNa2O4·2H2O), thiourea, 4-AP and all the chemicals were purchased from Sigma-Aldrich. The chemical used in the selectivity studies such as, glucose, fructose, dopamine, ascorbic acid and common metal ions were prepared from Sigma-Aldrich. All the chemicals were of analytical grade and used without purification. Double distilled (DD) water was used for all the experiments. The crystal structure information was determined by Bruker D8 Advance X-ray diffractometer (XRD) with monochromatized Cu-Kα radiation (λ = 1.5418 Å). FTIR analysis was performed by using a Thermo Nicolet Nexus 670 spectrometer in the range of 4000–400 cm−1. Raman spectra were collected in an NT-MDT confocal Raman microscopic system with an exciting laser wavelength of 473 nm and the laser spot-size is around 0.5 μm. For the Raman characterization, the Si peak at 520 cm−1 was used as a reference for wavenumber calibration. The morphology and quality of the sample were analyzed by scanning electron microscopy (SEM) using Zeiss SUPRA 55 SEM apparatus with an applied voltage of 20 kV and transmission electron microscopy (TEM) using JEOL JEM-3010 microscope. The chemical composition was analyzed by a JEOL JED-2300 energy dispersive X-ray spectrometer (EDX). The electrochemical impedance spectroscopy (EIS) was recorded by XPOT (ZAHNER elektrik instrument).
All electrochemical measurements were carried out using CHI 405a and CHI 900 work station at ambient temperature. Electrochemical experiments were carried out in a conventional three electrode cell using BAS GCE as a working electrode (area 0.071 cm2), saturated Ag|AgCl (saturated KCl) as a reference electrode and Pt wire as a counter electrode. The supporting electrolyte used for all the electrochemical experiments (except different pH studies) is 0.05 M phosphate buffer PBS (pH 7) which is prepared from disodium hydrogen phosphate and sodium dihydrogen phosphate. 0.05 M citric acid–sodium citrate buffer was used to prepare pH 3 and 5. 0.05 M and sodium carbonate–sodium bicarbonate buffer was used to prepare pH 9 and 11.
2.2 Synthesis of MoS2 nanoclusters
In a typical synthesis, 0.5 mM of MoNa2O4·2H2O and 0.25 mM of thiourea were dissolved in 60 mL of deionized water and stirred for 30 min. The as-obtained solutions were transferred into Teflon-lined stainless steeled autoclave of 100 mL capacity and heated at 140 °C for 12 h and then the autoclave was cooled down the room temperature. The resulting black precipitates were collected by centrifugation and washed with water and ethanol for several times, then dried at 80 °C for 4 h.
2.3 Fabrication of MoS2 modified electrode for 4-AP detection
To fabricate 4-AP sensor, GCE was polished with 0.05 μM alumina powder and then ultrasonicated about 2 min in ethanol and DD water successively to remove the adsorbed alumina slurry on the GCE surface. MoS2 modified GCE was fabricated by simple drop casting method. The as-prepared nanoclusters were re-dispersed in DD water about 5 mg mL−1 and then ultrasonicated for 30 min to get the uniform suspension. 8 μL suspension of MoS2 was drop coated on GCE and dried at ambient conditions. All the electrochemical measurements were carried out at the room temperature.
3. Results and discussion
3.1 Characterization of MoS2
X-ray diffraction analysis was used to determine the crystalline phase, orientation and grain size of the as-synthesized MoS2. As documented in Fig. 1, the pronounced diffraction peaks at 2θ degree were observed at 13.72°, 32.30°, 35.62° and 57.8° which is well agreed exactly to the (002), (100), (102) and (110) reflection planes of hexagonal phase MoS2 nanoclusters respectively.43 Aforementioned results revealing that no other discernible peaks observed corresponding to any other significant impurities, indicating the as-synthesized MoS2 nanoclusters had high crystalline nature. The average crystalline size of the MoS2 nanoclusters was examined by full-width half maximum value of prominent diffraction peak of the XRD pattern using Scherer's equation as follows,44 |
Xs = (kλ/β cos θ)
| (1) |
where Xs is the size of crystalline, λ is X-ray wavelength, β is FWHM (full width at half maximum) of the diffraction peak, θ is diffraction angle and k is Scherer's constant. The crystalline size was calculated for the diffraction plane (002) and the average size is to be 13 nm. The chemical compositions of the as-synthesized nanoparticles were identified using FTIR analysis and shown in Fig. 2A. The absorption bands at 421 and 455 cm−1 were assigned to the presence of Mo–S vibration of MoS2 and the small peak at 671 cm−1 indicates the presence of Mo–O stretching vibrations. It denotes the oxides slightly participated into the as-synthesized product.45 The bands at 1068, 1744 and 1647 cm−1 shows the C–O, C
O stretching vibrations and O–H bending vibration respectively. The peak at 1469 to 1517 cm−1 indicates the C–N stretching vibrations of thiourea. Fig. 2B presents the Raman spectrum of MoS2 nanoclusters which showed two major characteristic peaks at 372 cm−1 and 396 cm−1 agreed well with E12g and A1g Raman active modes of the hexagonal MoS2.46 The obtained E12g and A1g modes clearly demonstrate that in-plane vibrations of Mo and S atoms and out-of-plane vibrations of S atoms in different directions, respectively.
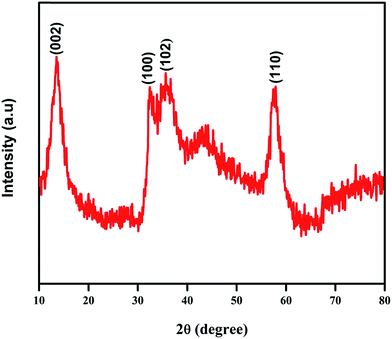 |
| Fig. 1 XRD pattern of MoS2 nanoclusters. | |
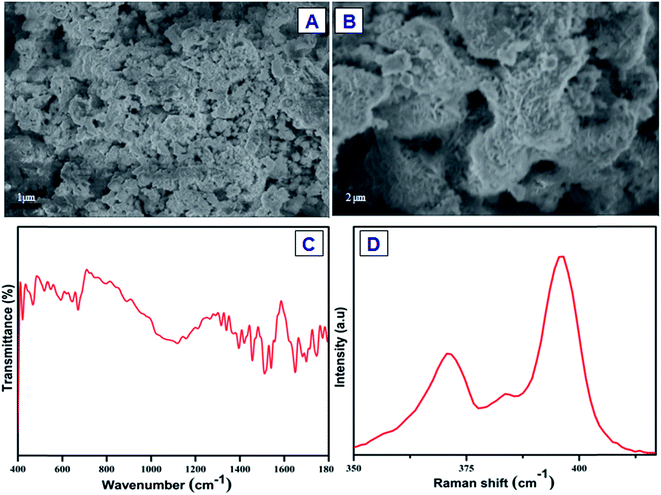 |
| Fig. 2 (A & B) scanning electron microscopy images (C) FT-IR and (D) Raman spectra for MoS2 nanoclusters. | |
The surface morphology of the as-synthesized nanoparticles was analyzed using scanning electron microscopy (SEM) as shown in Fig. 2A and B. It reveals that the as-synthesized MoS2 nanoparticles have cluster like morphology and the detailed morphology were investigated by TEM analysis. Fig. 3A and B revealed that TEM images of the as-synthesized MoS2 nanostructure having a cluster like morphology within the nano range. In addition, we can obviously observe some brighter areas which indicate that there are some pits on the cluster like surfaces. The average diameter of the pits is to be 10–20 nm. The selective area electron diffraction (SAED) pattern indicates the diffraction planes of (002), (100), (102) and (110) corresponding to the hexagonal phase of MoS2 nanoclusters (Fig. 3C). Thus, morphology studies and XRD patterns are in good consistency. The elemental compositions were analyzed using energy-dispersive X-ray (EDX) spectra which confirmed the presence of molybdenum and sulphur in the as-synthesized nanoclusters and no other impurities were detected (Fig. 3D). The inset to Fig. 3D confirms that the stoichiometric ratio of Mo and S was 2
:
1 which is in good agreement with the experimental protocols.
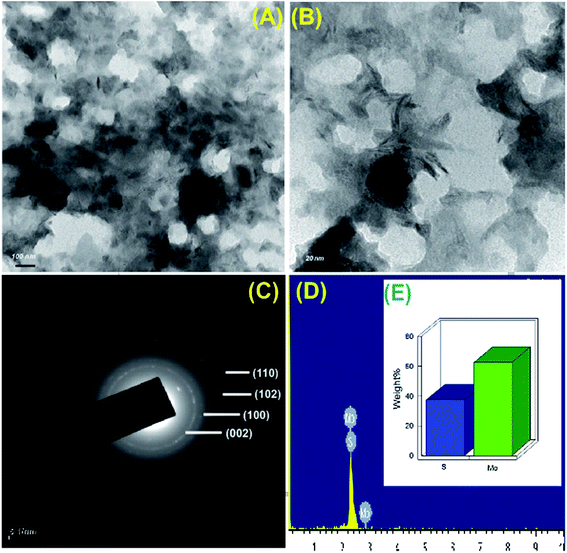 |
| Fig. 3 HR-TEM images of MoS2 nanoclusters at different magnification (A) 100 nm (B) 20 nm (C) SAED pattern (D) EDX spectrum (E) inset: quantitative result of MoS2. | |
3.2 Electron impedance spectroscopy (EIS)
Fig. 4 represents the Nyquist plot at bare GCE (a) and MoS2/GCE (b) in 0.1 M KCl aqueous solution containing 5 mM [Fe(CN)6]3−/4−. It can be seen the electron transfer resistance of the MoS2/GCE (curve b) was much larger than that of bare GCE (Fig. 4a), it proposing that a MoS2 nanoclusters was formed on the GCE surface. The upper inset; shows the enlarged view of bare GCE. Furthermore, a small fine defined semicircle at higher frequencies was observed at the bare GCE, suggested small interface impedance. After that, the MoS2 was drop casted on the GCE surface, the impedance value very larger than that at bare GCE, which could be characterized to the negative charges on MoS2, introducing a electrostatic repulsion into the solution/electrode system, leading to a lower rate of the electron transfer of [Fe(CN)6]3−/4−. This method also confirmed that MoS2 was successfully immobilized on the GCE.
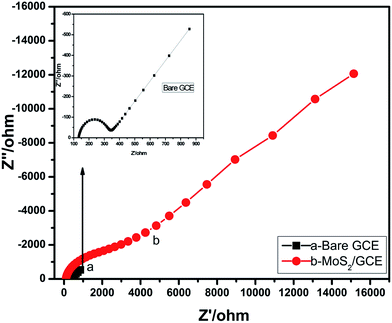 |
| Fig. 4 The electrochemical impedance spectroscopy (EIS) of bare GCE (a), MoS2/GCE (b) in 0.1 M KCl aqueous solution containing 5 mM [Fe(CN)6]3−/4−. The inset: the enlarged view of curve (a). | |
3.3 Electrocatalytic activity of 4-AP on MoS2/GCE
The electrochemical behavior of 4-AP (200 μM) on MoS2/GCE and bare GCE was investigated in 0.05 M PBS (pH 7.0) by cyclic voltammetry (Fig. 5). The applied scan rate was 50 mV s−1. No significant peaks were appeared in the absence of 200 μM of 4-AP (Fig. 5, curves a and c) in the selected scanning region. However, the background of the composite was larger than that of bare GCE which is confirmed to the large unambiguous area. At GCE, quasi-reversible redox peaks were observed in the presence of 4-AP with anodic peak potential (Epa) of 0.167 V and cathodic peak potential (Epc) of 0.065 V (curve c). At MoS2/GCE (curve d), a pair of well defined and quasi-reversible redox peaks corresponding to the electrochemical reaction of 4-AP were observed with Epa = 0.132 V and Epc = 0.077 V. Compared with bare GCE, the MoS2/GCE has shown significantly improved electrocatalysis for 4-AP which is revealed from the observed low ΔEp (54 mV). In addition, the ΔEp reported in this present work is very smaller than that reported by previous works47 which could be attributed the excellent conductivity and good electrocatalytic activity of MoS2. All these evidence suggested that MoS2/GCE is a very promising novel electrode material with enhanced performance towards 4-AP.
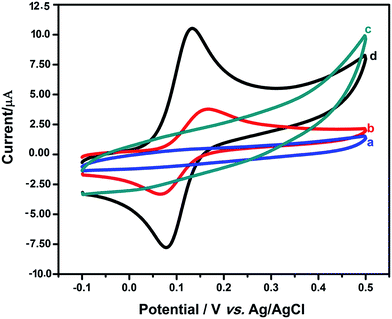 |
| Fig. 5 CVs of the bare GCE (a) and MoS2 nanoclusters modified GCE (c) in the absence (a and c) and presence (b and d) of 200 μM 4-AP in 0.05 M PBS (pH 7) solution at the scan rate 50 mV s−1. | |
3.4 Effect of pH
The phenol compounds are always involved proton transfer to form quinine in the electrochemical oxidation process and hence the electrochemical response of phenol compounds can be affected by the pH solution.48,49 The effect of the pH solution on the redox reaction of 4-AP at MoS2/GCE was investigated in the range of pH 3.0 to 11.0 as shown in Fig. 6. Both Epa and Epc were negatively shifted as the pH value is increased. The redox peak currents of 4-AP gradually increased with increasing the pH from 3.0 to 5.0. Further increase in the pH from 9.0 to 11.0, the redox peak currents started to decrease. The maximum catalytic response currents were achieved at pH 7.0 and hence the pH 7.0 was chosen as optimal pH condition for electrochemical experiments. According to the Nernst equation, formal potential value (E0′) changes linearly as a function of solution pH. The linear regression equation can be expressed as E0′/V = 0.520 − 0.055pH (R2 = 0.995) (Fig. 6). The slope value of −55.0 mV pH−1 is nearest to the theoretical value of −58.9 mV pH−1 for a reversible reaction involving equal numbers of electrons and protons. Hence, the redox reaction of 4-AP is a two-protons and two-electrons involved process at MoS2/GCE modified electrode as shown in Scheme 1.
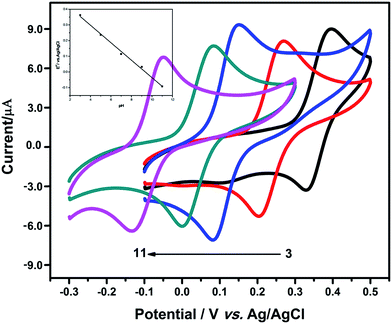 |
| Fig. 6 CVs of 200 μM 4-AP at MoS2/GCE in 0.05 M PBS with different pH values of 3.0, 5.0, 7.0, 9.0 and 11.0. Insert: the plot of formal potential vs. pH values at the scan rate 50 mV s−1. | |
 |
| Scheme 1 The redox mechanism of 4-AP at MoS2/GCE. | |
3.5 Effect of scan rate
In order to investigate reaction kinetics, the influence of scan rate on redox peaks currents of 4-AP at the MoS2/GCE was investigated by taking cyclic voltammograms (CVs). As shown in Fig. 7. The cathodic and anodic peak currents intensity increased continuously with increased the scan rate (Fig. 7A). A good linear relationship between the peak current and the square root of the scan rate (ν1/2) from 50 to 1000 mV s−1 was obtained (Fig. 7B) which revealed that the redox reaction of 4-AP at MoS2/GCE is a diffusion controlled process. The linear regression equations are, Ipa/A = −25.81(scan rate)1/2 − 2.742 (R2 = 0.999) and Ipc/A = 20.79(scan rate)1/2 + 3.234 (R2 = 0.996). The relationship between Ep and log
ν is represented as Tafel plot (Fig. 7C) which shows good linear relationship between peak potentials (anodic and cathodic) and logarithm of the scan rate. As scan rate, increases, the anodic peak potential (Epa) was shifted positively, while the cathodic peak potential (Epc) was shifted negatively. The linear regression equations can be expressed as Epa = 0.190
log
ν − 0.061 (R = 0.991) and Epc = 0.0.037
log
ν − 0.039 (R = 0.993). Besides, the plots of Ep (anodic and cathodic) vs. log
ν exhibits two straight lines with slopes of −2.3RT/(1 − α)nF and −2.3RT/αnF for the Epc and Epa, respectively.50 Here, α and na (=1) are electron transfer coefficient and number of electrons involved in the rate-determining step, while the constants R, T and F represents their usual meanings (R = 8.314 J K−1 mol−1, T = 298 K, F = 96
485 C mol−1). The charge transfer coefficient can be calculated from Tafel slopes. The value of α is 0.60 (±0.013) which is close to 0.5 means that the activated complex is around halfway between reactants and products on the reaction coordinate. In the other words, structure of the activated complex reflecting reactants and products equally.
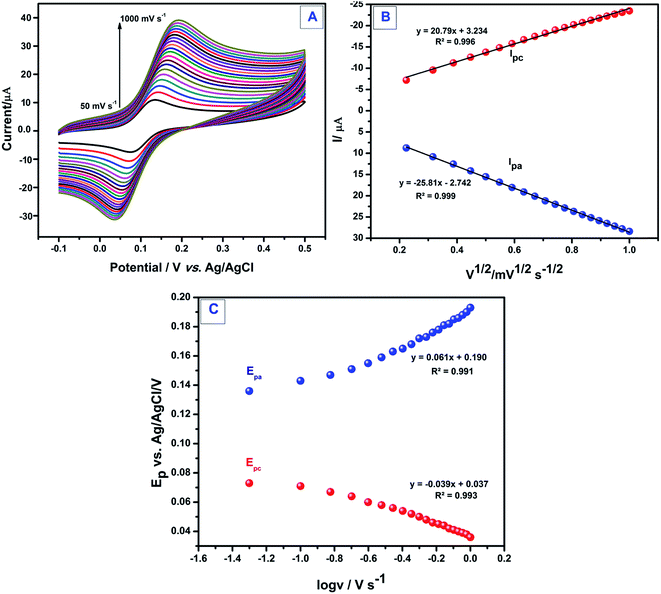 |
| Fig. 7 (A) CVs of MoS2/GCE in 0.05 M PBS (pH 7) containing 200 μM 4-AP at different scan rates (50 to 1000 mV s−1). (B) The plot for the dependence of peak current on the scan rate. (C) The relationships between Ep and log ν. | |
3.6 Determination of 4-AP
The voltammetry determination of 4-AP was carried out in PBS (pH 7.0) using Differential Pulse Voltammetry (DPV) at MoS2/GCE (Fig. 8). The optimized instrumental parameters for Differential Pulse Voltammetry (DPV) experiments are, amplitude = 0.05 V, pulse width = 0.05 s and pulse period = 0.02 s. Fig. 8A depicts the anodic peak current responses observed for oxidation of various concentrations of 4-AP in the presence of MoS2/GCE. Significantly, the anodic peak current response increased linearly with the addition of 4-AP in the range of 0.04–17 μM (Fig. 8B). The linear regression equation was expressed as Ip (μA) = 0.338 + 0.751[4-AP]/μM; R2 = 0.989. The detection limit was estimated to be 0.03 (±0.008) μM and the sensitivity of the electrode is 4.278 (±0.058) μA mM−1 cm−2. A detailed comparison of the sensor performances of different electrochemical sensors for the determination of 4-AP is shown in Table 1. Compared with previously reported modified electrodes, the MoS2 modified GCE offered a wide linear range for 4-AP detection and a low detection limit. This all results suggest that the MoS2/GCE possesses the capability for the quantitative determination of 4-AP.
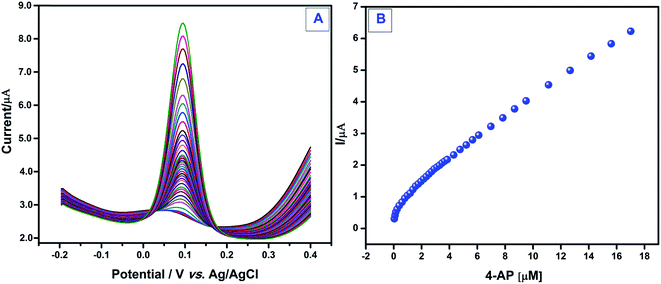 |
| Fig. 8 (A) DPV response of MoS2/GCE modified electrode with increasing the concentration of 4-AP (from 0 to17 μM). (B) The calibration plot observed in the concentration range of 0–17 μM of 4-AP. | |
Table 1 Comparison of analytical parameters for the detection of 4-AP over previously reported modified electrodes
Modified electrode |
Methods |
Sensitivity/μA mM−1 cm−2 |
Linear ranges/μM |
LOD/μM |
Ref. |
Single-wall carbon nanotubes/poly(4-aminopyridine) |
CV |
— |
0.2–100 |
0.06 |
51 |
Hemin-modified molecularly imprinted polymer |
Amperometry |
— |
10–90 |
3.00 |
52 |
Graphene–chitosan/GCE |
DPV |
— |
0.2–550 |
0.057 |
53 |
2,2-(1,4-Phenylenedivinylene) bis-8-hydroxyquinoline/XAD-7 |
Fiber-optic reflectance |
— |
0.92–20 |
0.18 |
54 |
Screen-printed carbon electrodes |
NPV |
— |
0.2–200 |
0.05 |
55 |
Mesoporous silica-modified CPE |
DPV |
— |
0.23–28 |
0.092 |
56 |
Carbon ionic liquid electrode |
DPV |
— |
0.3–1000 |
0.1 |
57 |
Poly(3,4-ethylenedioxythiophene)/GCE |
DPV |
— |
4–320 |
1.2 |
58 |
MoS2/GCE |
DPV |
4.278 |
0.04–17 |
0.03 |
This work |
3.7 Interference studies
Selectivity of the MoS2/GCE towards determination of 4-AP was investigated in the presence of likely interferences, such as metal ions, biological interferents, and drugs. The tested interferents are Ca2+, Fe3+, Ba2+, Cu2+, Zn2+, Mg2+, K+, Na+, NO3−, Cl−, SO42−, Br−, I−, glucose, fructose, dopamine, ascorbic acid and paracetamol (0.3 mM). The results indicated that the aforementioned interferents had not produced any measurable signals (Fig. 9). However, the modified electrode delivered good response to 4-AP (1 μM) which indicating good anti-interference ability of the MoS2/GCE.
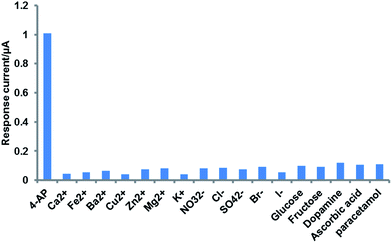 |
| Fig. 9 Selectivity study; plot between response current vs. 4-NP and interference species. DPVs were carried out in PBS (pH 7) containing 1 μM 4-NP and 0.2 mM of interfering analytes (Ca2+, Fe3+, Ba2+, Cu2+, Zn2+, Mg2+, K+, Na+, NO3−, Cl−, SO42−, Br−, I−, glucose, fructose, dopamine, ascorbic acid and paracetamol). | |
3.8 Repeatability, reproducibility and practicality
In order to calculate repeatability of the MoS2/GCE, 6 repeated DPV experiments were carried out towards determination of 4-AP (0.1 μM). The relative standard deviation (RSD) between 6 repeatable measurements at MoS2/GCE was 3.84%. To calculate reproducibility of the electrode, four different MoS2/GCE were fabricated and their DPV responses towards 4-AP (0.1 μM) were monitored. The RSD between four DPV measurements was calculated to be 3.72%. The RSD values obtained for repeatability and reproducibility experiments are in acceptable range and hence the developed modified electrode exhibited good repeatability and reproducibility towards determination of 4-AP.
Real-time application of the developed modified electrode is demonstrated in water samples. The water samples such as tap water, drinking water, and rain water were collected, filtered and spiked with known concentrations of 4-AP. The 4-AP spiked water samples were analysed using MoS2/GCE under optimized lab samples experimental condition. The added, found and recovery results were presented as Table 2. The spiked 4-AP concentrations were of 100 nM and 300 nM. The obtained satisfactory recovery values from 96.20% to 98.54% revealed good practical applicability of the modified electrode.
Table 2 Determination of 4-AP at MoS2/GCE in human serum sample
Samples |
Added/nM |
Found/nM |
Recovery/% |
RSDa/% |
Relative standard deviation of three individual measurements. |
Tap water |
100 |
98.54 |
98.54 |
3.52 |
300 |
293.78 |
97.93 |
3.82 |
Drinking water |
100 |
96.43 |
96.43 |
3.64 |
300 |
294.35 |
98.12 |
3.19 |
Rain water |
100 |
96.20 |
96.20 |
3.94 |
300 |
294.16 |
98.05 |
3.88 |
4. Conclusions
In summary, we have described a facile hydrothermal method to prepare MoS2 nanoclusters. The MoS2 nanoclusters have shown excellent electrochemical detection ability to detect 4-AP. The MoS2 nanoclusters were characterized using XRD, Raman spectra, FTIR, SEM, TEM, SAED and EDX methods. These results demonstrated that MoS2 possess nanostructured surface with high surface area and exhibited high electrocatalytic activity towards 4-AP. The MoS2/GCE showed good sensitivity, wide linear range (0.04–17 μM), low limit of detection (0.03 ± 0.008 μM) and good selectivity. The as-prepared MoS2 nanoclusters electrode is a promising electrode for the practical determination of 4-AP in environments samples.
Acknowledgements
We are grateful to thank the University Grant Commission (UGC – F. No. 42-348/2013 (SR) & 01.04.2013), New Delhi, India for financial support. We also express our gratitude to the College Managing Board, Principal and Head of the Department of Chemistry, VHNSN College, Virudhunagar for providing research facilities.
References
- T. Nemeth, P. Jankovics, J. Nemeth-Palotas and H. Koszegi-Szalai, J. Pharm. Biomed. Anal., 2008, 47, 746–749 CrossRef CAS PubMed.
- The United States Pharmacopeial Convention, The United States Pharmacopoeia 27-NF (The National Formulary), 2004, p. 2494 Search PubMed.
- S. Mitchell and K. Othmer, Encyclopedia of chemical technology, Wiely, Newyork, USA, 4th edn, 1992 Search PubMed.
- S. A. Khan, M. Hamayun and S. Ahmed, Enzyme Microb. Technol., 2006, 38, 10–13 CrossRef CAS.
- H. Xu, C. F. Duan, Z. F. Zhang, J. Y. Chen, C. Z. Lai, M. Lian, L. J. Liu and H. Cui, Water Res., 2005, 39, 396–402 CrossRef CAS PubMed.
- E. W. Kaszuba, M. W. Grzeskiewicz and Z. Fijalek, J. Pharm. Biomed. Anal., 2003, 32, 1081–1086 CrossRef.
- H. Song and T. S. Chen, J. Biochem. Mol. Toxicol., 2001, 15, 34–40 CrossRef CAS PubMed.
- M. S. Ali, S. Rafiuddin, M. Ghori and A. R. Kahtri, J. AOAC Int., 2007, 90, 82–93 CAS.
- Q. chu, L. Jiang, X. Tian and J. Ye, Anal. Chim. Acta, 2008, 606, 246–251 CrossRef CAS PubMed.
- J. Forshed, F. O. Andersson and S. P. Jacobsson, J. Pharm. Biomed. Anal., 2002, 29, 495–505 CrossRef CAS PubMed.
- M. S. Bloomfield, Talanta, 2002, 580, 1301–1310 CrossRef.
- J. Kibsgaard, Z. Chen, B. N. Reinecke and T. F. Jaramillo, Nat. Mater., 2012, 11, 963–969 CrossRef CAS PubMed.
- D. Kong, H. Wang, J. J. Cha, M. Pasta, K. J. Koski, J. Yao and Y. Cui, Nano Lett., 2013, 13, 1341–1347 CrossRef CAS PubMed.
- Z. Wu, B. Fang, Z. Wang, C. Wang, C. Wang, Z. Liu, F. Liu, W. Wang, A. Alfantazi, D. Wang and D. P. Wilkinson, ACS Catal., 2013, 3, 2101–2107 CrossRef CAS.
- O. L. Sanchez, D. Lembke, M. Kayci, A. Radenovic and A. Kis, Nat. Nanotechnol., 2013, 8, 497–501 CrossRef PubMed.
- Y. Lu, X. Yao, J. Yin, G. Peng, P. Cui and X. Xu, RSC Adv., 2015, 5, 7938–7943 RSC.
- T. Stephenson, Z. Li, B. Olsen and D. Mitlin, Energy Environ. Sci., 2014, 7, 209–231 CAS.
- X. Wang, Z. Zhang, Y. Chen, Y. Qu, Y. Lai and J. Li, J. Alloys Compd., 2014, 600, 84–90 CrossRef CAS.
- G. S. Bang, K. W. Nam, J. Y. Kim, J. Shin, J. W. Choi and S. Y. Choi, ACS Appl. Mater. Interfaces, 2014, 6, 7084–7089 CAS.
- B. Lei, G. R. Li and X. P. Gao, J. Mater. Chem. A, 2014, 2, 3919–3925 CAS.
- S. S. Kim, J. W. Lee, J. M. Yun and S. I. Na, J. Ind. Eng. Chem., 2015, 29, 71–77 CrossRef CAS.
- J. M. Yun, Y. J. Noh, J. S. Yeo, Y. J. Go, S. I. Na, H. G. Jeong, J. Kim, S. Lee, S. S. Kim, H. Y. Koo, T. W. Kim and D. Y. Kim, J. Mater. Chem. C, 2013, 1, 3777–3783 RSC.
- X. Gu, W. Cui, H. Li, Z. Wu, Z. Zeng, S. T. Lee, H. Zhang and B. Sun, Adv. Energy Mater., 2013, 3, 1262–1268 CrossRef CAS.
- D. Voiry, M. Salehi, R. Silva, T. Fujita, M. Chen, T. Asefa, V. B. Shenoy, G. Eda and M. Chhowalla, Nano Lett., 2013, 13, 6222–6227 CrossRef CAS PubMed.
- D. Wang, Z. Pan, Z. Wu, Z. Wang and Z. Liu, J. Power Sources, 2014, 264, 229–234 CrossRef CAS.
- Q. H. Wang, K. Kalantar-Zadeh, A. Kis, J. N. Coleman and M. S. Strano, Nat. Nanotechnol., 2012, 7, 699–712 CrossRef CAS PubMed.
- M. Chhowalla, H. S. Shin, G. Eda, L. J. Li, K. P. Loh and H. Zhang, Nat. Chem., 2013, 5, 263–275 CrossRef PubMed.
- B. Radisavljevic, M. B. Whitwick and A. Kis, ACS Nano, 2011, 5, 9934–9938 CrossRef CAS PubMed.
- V. K. Sangwan, H. N Arnold, D. Jariwala, T. J. Marks, L. J. Lauhon and M. C. Hersam, Nano Lett., 2013, 13, 4351–4355 CrossRef CAS PubMed.
- B. Radisavljevic, A. Radenovic, J. Brivio, V. Giacometti and A. Kis, Nat. Nanotechnol., 2011, 6, 147–150 CrossRef CAS PubMed.
- Z. Y. Zhu, Y. C. Cheng and U. Schwingenschlogl, Phys. Rev. B: Condens. Matter Mater. Phys., 2011, 84, 153402–153411 CrossRef.
- H. Wang, P. Chen, F. Wen, Y. Zhu and Y. Zhang, Sens. Actuators, B, 2015, 220, 749–754 CrossRef CAS.
- X. Wanga, C. Chua, L. Shena, W. Denga, M. Yana, S. Geb, J. Yua and X. Song, Sens. Actuators, B, 2015, 206, 30–36 CrossRef.
- J. Huang, Y. He, J. Jin, Y. Li, Z. Dong and R. Li, Electrochim. Acta, 2014, 136, 41–46 CrossRef CAS.
- G. F. Shi, J. T. Cao, J. J. Zhang, Y. M. Liu, Y. H. Chen and S. W. Ren, Sens. Actuators, B, 2015, 220, 340–346 CrossRef CAS.
- H. U Kim, H. Ki, C. Ahn, A. Kulkarni, M. Jeon, G. Y. Yeom, M. H. Lee and T. Kim, RSC Adv., 2015, 5, 10134–10138 RSC.
- M. A. Lukowski, A. S. Daniel, F. Meng, A. Forticaux, L. S. Li and S. Jin, J. Am. Chem. Soc., 2013, 135, 10274–10277 CrossRef CAS PubMed.
- H. T. Wang, Q. F. Zhang, H. B. Yao, Z. Liang, H. W. Lee, P. C. Hsu, G. Y. Zheng and Y. Cui, Nano Lett., 2014, 14, 7138–7144 CrossRef CAS PubMed.
- T. Y. Wang, R. Z. Zhu, J. Q. Zhuo, Z. W. Zhu, Y. H. Shao and M. X. Li, Anal. Chem., 2014, 86, 12064–12069 CrossRef CAS PubMed.
- M. Pumera and A. H. Loo, Trends Anal. Chem., 2014, 61, 49–53 CrossRef CAS.
- K. Chang and W. X. Chen, ACS Nano, 2011, 5, 4720–4728 CrossRef CAS PubMed.
- J. X. Zhu, W. P. Sun, D. Yang, Y. Zhang, H. H. Hoon, H. Zhang and Q. Y. Yan, Small, 2015, 11, 4123–4129 CrossRef CAS PubMed.
- B. Pourabbas and B. Jamshidi, Chem. Eng. J., 2008, 138, 55–62 CrossRef CAS.
- P. Senthil kumar, M. Selvakumar, S. G. Babu, S. Karuthapandian and S. Chattopadhyay, Mater. Lett., 2015, 151, 45–48 CrossRef CAS.
- G. Nagaraju, C. N. Tharamani, G. T. Chandrappa and J. Livage, Nanoscale Res. Lett., 2007, 2, 461–468 CrossRef CAS PubMed.
- H. T. Wang, Z. Y. Lu, S. C. Xu, D. S. Kong, J. J. Cha, G. Y. Zheng, P. C. Hsu, K. Yan, D. Bradshaw and F. B. Prinz, Proc. Natl. Acad. Sci. U. S. A., 2013, 110, 19701–19706 CrossRef CAS PubMed.
- M. Jamal, A. S. arac and E. Manger, Sens. Actuators, B, 2004, 97, 59–66 CrossRef CAS.
- L. Fernandez, C. Borras and H. Carrero, Electrochim. Acta, 2006, 52, 872–884 CrossRef CAS.
- H. Yin, L. Cui, S. Ai, H. Fan and L. Zhu, Electrochim. Acta, 2010, 55, 603–610 CrossRef CAS.
- E. Laviron, J. Electroanal. Chem., 1979, 101, 19–28 CrossRef CAS.
- Z. Wang, H. Zhu, H. Zhang, G. Gao, Z. Sun, H. Liu and X. Zhao, Electrochim. Acta, 2009, 54, 7531–7535 CrossRef CAS.
- J. Neto, W. Santos, P. Lima, S. Tanaka, A. Tanaka and L. Kubota, Sens. Actuators, B, 2011, 152, 220–225 CrossRef.
- H. Yin, Q. Ma, Y. Zhou, S. Ai and L. Zhu, Electrochim. Acta, 2010, 55, 7102–7108 CrossRef CAS.
- H. Filik, M. Hayvalı, E. Kilic, R. Apaka, D. Aksua, Z. Yanaza and T. Cengel, Talanta, 2008, 77, 103–109 CrossRef CAS PubMed.
- W. Su, S. Wang and S. Cheng, J. Electroanal. Chem., 2011, 651, 166–172 CrossRef CAS.
- D. Sun, X. Li, H. Zhang and X. Xie, Int. J. Environ. Anal. Chem., 2012, 92, 324–333 CrossRef CAS.
- A. Safavi, N. Maleki and O. Moradlou, Electroanalysis, 2008, 20, 2158–2162 CrossRef CAS.
- S. Mehretie, S. Admassie, T. Hunde, M. Tessema and T. Solomon, Talanta, 2011, 85, 1376–1382 CrossRef CAS PubMed.
|
This journal is © The Royal Society of Chemistry 2016 |