DOI:
10.1039/D4TA01040J
(Review Article)
J. Mater. Chem. A, 2024,
12, 18674-18704
A comprehensive review on advanced supercapacitors based on transition metal tellurides: from material engineering to device fabrication
Received
15th February 2024
, Accepted 6th June 2024
First published on 7th June 2024
Abstract
Transition Metal tellurides (TMTes) have garnered significant interest in recent years due to their unique properties, including high electrical conductivity, abundant redox-active sites and excellent chemical stability. These attributes make them attractive candidates for energy storage applications, particularly in supercapacitors, where high power density and long-term cycling stability are essential. Even though TMTes have demonstrated excellent energy storage capabilities, further optimization of electrode materials and devices is essential to attain competitive electrochemical performance. In this review, we provided a detailed overview of the synthesis methods employed to fabricate TMTes-based electrodes, highlighting key parameters that influence material morphology, composition and electrochemical performance. We systematically compared the electrochemical properties of various TMTes, including their specific capacitance, rate capability, and cycling stability. Furthermore, we have discussed the mechanisms governing the charge storage behavior of TMTes, elucidating their redox processes and ion transport kinetics within the electrode–electrolyte interface. We also provided a valuable insight into the design and optimization of TMTes-based supercapacitors for practical applications. The review embarks on the scrupulous elaboration of ways to enhance the electrochemical properties of TMTes through various innovative strategies followed by critical challenges and future perspectives. TMTes as an eminent electrode material holds great potential to revolutionize the energy landscape and support the growing energy demands of the future.
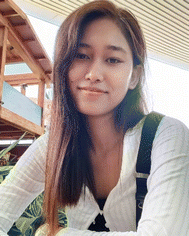 Chumuiria Debbarma | Chumuiria Debbarma received her BSc degree in Chemistry from Union Christian College (Meghalaya, India) in 2020 and M.Sc. degree in Chemistry from Jain (Deemed-to-be) University (Bangalore, India) in 2022. From 2022 to 2023, she worked as a research intern under the guidance of Prof. Chandra Sekhar Rout. |
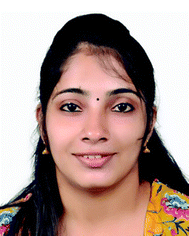 Sithara Radhakrishnan | Sithara Radhakrishnan received her B.Tech. in Polymer Engineering from Mahatma Gandhi University (Kerala, India) in 2016 and her M.Tech. in Polymer Technology from Cochin University of Science and Technology (Kerala, India) in 2018. She is a Nanoscience doctoral student at the Centre for Nano & Material Sciences (CNMS), Jain (Deemed-to-be) University, working under Prof. Chandra Sekhar Rout. Her current research focuses on designing and fabricating 2D materials and their nanocomposites for energy storage and conversion applications. |
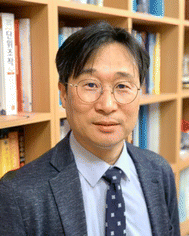 Sang Mun Jeong | Sang Mun Jeong is a Professor in the Department of Chemical Engineering at Chungbuk National University, South Korea, a leader of the Regional Leading Research Center (RLRC) for developing next-generation battery materials funded by National Research Foundation of Korea, and a director of the Korea Institute of Chemical Engineers. Also, he was a dean of the research affairs of Chungbuk National University (2021–2023). His research focuses on energy-related materials and processes based on chemical and electrochemical engineering to develop efficient, clean and renewable future energy. |
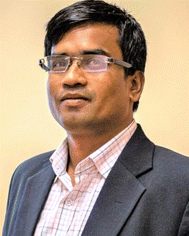 Chandra Sekhar Rout | Chandra Sekhar Rout is a full Professor at the Centre for Nano & Material Sciences (CNMS), Jain University. Before joining CNMS, he was a DST – Ramanujan Fellow at IIT Bhubaneswar, India (2013–2017). He obtained his PhD from JNCASR, Bangalore (2008) under the supervision of Prof. C.N.R. Rao followed by postdoctoral research at NUS, Purdue University, and UNIST. His research is focused on applications of 2D layered materials for different devices. He has authored more than 200 research papers and 06 books. His h-index is 58 with total citations >14,500. He was ranked top 2% of scientists by the Stanford study in 2020–2023. |
1 Introduction
The pursuit of the development and modernization of our societies has been accompanied by a steep growth in global energy consumption over the last few years. This, in turn, has imposed a strain on the fossil fuel reserves, raising concerns about their slow depletion.1 One approach to overcome this obstacle is to focus on developing technologies for harnessing intermittent renewable energy resources.2 To use the electricity generated by these sources for high power generation in a short period of time, it must also be stored in energy storage systems. Currently, the energy storage device market is predominantly occupied by batteries and supercapacitors. The development of electrode materials is critical for the practical implementation of these technologies as it directly influences the performances of energy storage devices. Transition metal dichalcogenides (TMDs) are a promising electrode material in the realm of supercapacitor applications due to their unique properties. They demonstrate rapid surface redox reactions, possess interlayer spaces for accommodating guest species and feature layered architectures facilitating ion diffusion. These features distinguish TMDs from other electrode materials being studied for electrochemical energy storage systems.3
Tellurium (Te), founded in the 16th period and 5th group of the periodic table, exhibit higher electrical conductivity compared to sulphur (S) and selenium (Se) due to its lower electronegativity and large radius. Consequently, TMTes exhibit excellent physical, chemical, and electrochemical properties due to its higher degree of covalency. TMTes offer superior ion diffusion rate capability and electrochemical performances due to their remarkable electronic conductivity (10 × 103 S m−1) compared to S (5 × 10−28 S m−1) and Se (1 × 10−3 S m−1). Thus, TMTes have emerged as a significant contender for electrode materials in supercapacitors (SCs), leading to a surge in research activities over the past five years. While a great deal of research has been done on the structural properties, synthetic routes, and various properties of TMTes, including magnetism, sensing, and superconductivity, the main focus of attention when it comes to their electrochemical storage performance has been on particular factors like the electrode materials' capacitance, conductivity, and cycling stability. However, the significance of device configuration in determining overall capacitance has been somewhat overlooked. A thorough study of the important parameters controlling capacitance from the standpoint of the overall device and ways to further improve electrochemical performance is still prominently missing from the literature. With the rapid advancement in this field, it is timely to consolidate current advancements, outline methodologies for material design and device optimization, and clarify the underlying mechanisms governing their performance. In summary (Fig. 1), there is a pressing need to bridge this gap by providing a comprehensive overview of the interplay between material properties, device configuration, and electrochemical performance of TMTes-based SCs, ultimately fostering a deeper understanding and facilitating future advancements in this field.
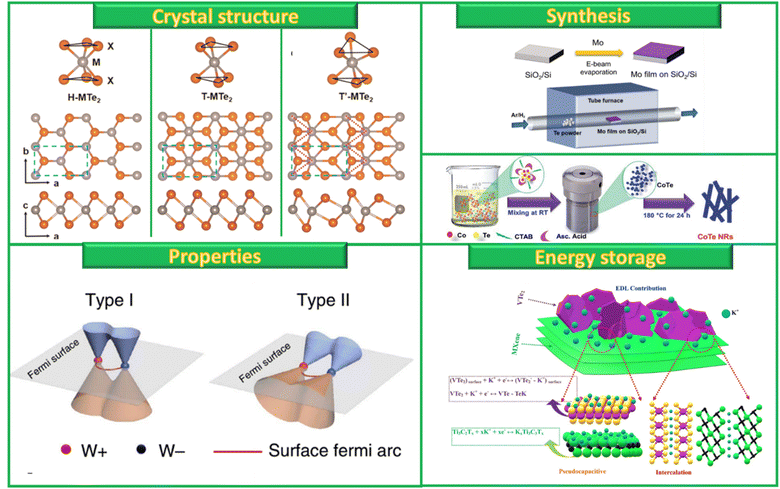 |
| Fig. 1 Schematic representation summarizing the content of the review based on TMTes. | |
2 Composition, crystal structure, and physical properties of TMTes
2.1 Composition and crystal structure
TMDs are a diverse class of materials known for their unique structural chemistry. The chalcogenides of early transition metals (groups IV, V, and VI) exhibit low-dimensional structures. The lower electronegativity of chalcogens S, Se, and Te, when compared to oxygen (O), reduces the ionicity of metal-chalcogen bonds, stabilizing anion–anion repulsions that separate layers or chains. The attraction between layers or chains is primarily due to van der Waals interactions. The compound's characteristics reflect their anisotropic bonding. Guest molecules can be intercalated into their van der Waal's gap to modify their chemical properties. While binary chalcogenides have been widely explored, introducing a third component opens up new possibilities. Some notable ternary chalcogenides include the Chevrel phases.1–3 Chevrel phase compounds, denoted by the formula MnMo6X8, are a class of inorganic materials known for their unique properties. Notably, they exhibit a remarkable ability to swiftly and reversibly incorporate various cations, including those of monovalent, divalent, and even trivalent nature. This versatility makes them highly suitable in the applications such as energy storage and electrocatalysis.4 Getting telluride Chevrel phases, such as MnMo6Te8, is more challenging compared to obtaining selenide Chevrel phases like MnMo6Se8. This difference seems to be linked to an abundance of electrons.5
Tellurium compounds have unique structures and properties compared to sulfides and selenides due to their higher covalent character. Tellurides in binary chalcogenides have different stoichiometries than sulfides and selenides. NbQ3 is known for Q = Se and S but not for Q= Te. NbQ4 exists for Q = Te but needs stabilization as (NbQ4)I0.33 for Q = Se. Even compounds with the same stoichiometry might have different coordination geometries. In NbQ2, Nb has trigonal prismatic coordination for Q = S and Se, while Q = Te has distorted octahedral coordination. Tellurium's higher covalency opens up more chalcogen–chalcogen bonding possibilities inside tellurides. Under similar chemical conditions, sulfur and selenium tend to form local (Q–Q)2− pairs (typical bond lengths are 2.05 Å for (S–S)2− and 2.35 Å for (Se–Se)2−) and adopt –I oxidation states, whereas tellurium exhibits oxidation states ranging between –I and –II, resulting in a broader spectrum of Te–Te distances. For example, in HfTe5, the Te–Te distance is 2.763 Å, whereas it is 4.0 Å (the van der Waals' separation) in HfTe2.6–9
2.1.1 Layered TMTes.
TMTes are made of a combination of two elements: a telluride (Te) and a transition metal (M) from groups 4 through 10 of the transition metal series. TMTes containing group IV–VII transition metals predominantly exhibit a layered structure, while VIII–X TMTes show a non-layered structure.10 Only certain TMTes in group VIII exhibit a layered structure, such as nickel (Ni), palladium (Pd) and platinum (Pt).
TMTes include hexagonally packed metal atoms sandwiched between two layers of tellurium atoms, which feature intralayer covalent interactions and an interlayer van der Waals interaction.11 The atoms in each layer are arranged in a trigonal prism or octahedron configuration, resulting in different lattice symmetries. The van der Waals gap between structures also makes them easier to exfoliate. The thickness of each layer in layered structures ranges from 6–7 Å. This makes physical or chemical exfoliation of bulk TMTes into single- or few-layered structures possible. Depending on the properties of the metal and chalcogen atoms, the stacked Te-M-Te sheets and the sequential stacking of the sheets that comprise the material can produce variety of polymorphs. Polymorphism is a unique feature of TMTes as it is part of TMDs. The crystal structures of the TMTes in binary compounds are mostly trigonal prismatic (2H) and octahedral (1T) phases, which belong to D3h and D3d point groups, respectively.12 It can exist in either the 2H thermodynamically stable phase or the metastable 1T phase, depending on the specific transition metal and Te combination. Furthermore, certain situations might cause the same material to exhibit both structural phases. For example, MoTe2 was found to stable in both the 2H and 1T′ phases, but WTe2 found to stable only in the orthorhombic 1Td phase.5 It should be noted that monolayer TMTes have trigonal prismatic and octahedral phases as polymorphs. Interestingly, a phase transition between the 2H and 1T phases was seen under specific conditions (temperature, charge transfer, strain, etc.). This could be explained by a change in the fraction of electrons in the transition metal's d-orbital and a relative shift in the free energies of the two phases. In other words, the type of the metallic atom determines material's electronic structure and thermodynamically stability. Wang et al.13 demonstrated that the properties of MoTe2 can be altered utilizing doping. They observed a phase transition as given in Fig. 2, highlights the influence of doping on materials behavior. These findings have major significance, since they show that controlled doping could be used to engineer local phase transitions inside monolayer MoTe2. This offers the possibility of developing tailored metal–semiconductor hybrid structures with fine control over their properties.
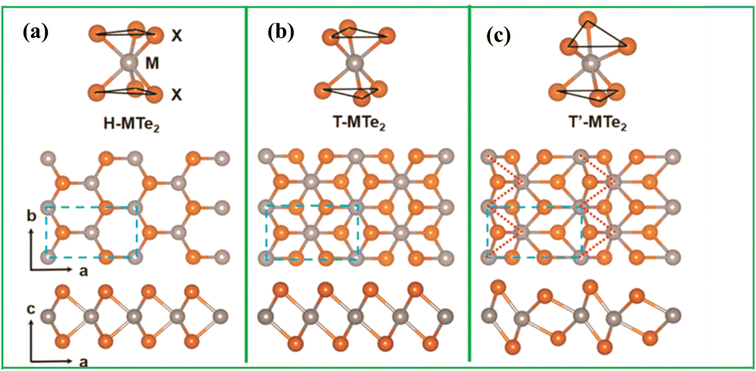 |
| Fig. 2 Atomic structure of TMTes in their (a) H phase, (b) T phase, and (c) distorted phase (T′) phase, Reproduced from ref. 14 with permission from [American chemical society], copyright [2024]. | |
2.2 Electronic properties
TMTes exhibit diverse electronic and topological properties influenced by their chemical composition and structural phases. Variations in layer stacking arrangements and potential distortions can alter the crystal structure, sometimes leading to periodic distortions caused by Jahn–Teller instability. These differences result in a range of electronic properties and correlated topological phases. In both the 2H and 1T phases of TMTes, the d bands are situated between the bonding and anti-bonding bands of the M–Te bonds. The electronic properties are significantly influenced by factors like the non-bonding d electron count and the coordination environment of the metal atoms. For example, the fully filled orbitals exhibit semiconducting behavior, while partially filled orbitals show metallic properties.15 The electrical structure of TMTes, particularly in 2D layered structures, is influenced by local bonding symmetry within layers rather than layer arrangement. Metal atom coordination with telluride atoms, as well as trigonal distortion of metal atom coordination, are important properties.16 2H-TMTes lack inversion symmetry, resulting in spin-splitting of electronic bands caused by spin–orbit interactions. Short interlayer Te⋯Te contacts play a significant role in p → d electron transport, therefore even minor arrangement changes affect electrical and physical properties.17,18 The electronic properties of TMTes can be fine-tuned by increasing the filling of transition metal d bands. TMTes, particularly MoTe2 and WTe2, are classified as type II Weyl semimetals and probable 2D topological insulators. Monolayer 2H–MoTe2 has been found to have the narrowest direct bandgap of ≈1.1 eV and smallest energy differential (≈35 meV per unit) between 2H and 1T′ structures, making them appropriate for various applications.19–23 While TMTes are well investigated for its semiconducting capabilities, they also have intriguing magnetic, optical, and thermoelectric features.24–28 Their large variety of oxidation states, due to tellurium's diffusive character and covalency, is critical for faradaic reactions-based. One of the most important properties of TMTes for faradaic reaction-dependent applications is their ability to exist in a wide range of oxidation states. Tellurium has a higher atomic number, which gives the material a diffusive character and increased covalency.29 Transition metals and telluride atoms form covalent bonds by hybridizing their respective d- and p-orbitals, resulting in a σ-bond that affects the charge distribution of both species. Increased overlap between cationic d levels and anionic sp bands causes a rise in paramagnetic shift in 125Te Nuclear Magnetic Resonance (NMR), suggesting electron transfer from Te to metal and reflecting Te's oxidation state (usually −2 to −1). In TMTes, weak interlayer van der Waals and strong intralayer interactions induce anisotropy, ideal for facile single-layer exfoliation in electronic devices. Nanosheets produced via exfoliation of TMTes exhibit HER activity primarily at edge sites, while the basal planes remain inert.25
2.3 Physical properties
TMTes have gained a lot of attention due to their ability to display a variety of unique properties, such as semiconductivity, charge density wave order (CDW), unconventional superconductivity, magnetism, and even topological phases. The instability of the linked electron lattice system, visible from bulk forms to single-layer limits, causes CDW, an interesting scientific phenomenon. CDW and other properties are regulated by electron–electron and electron–phonon interactions, which are critical in 2D materials and affect their stability under ambient and external conditions. Understanding CDW and superconductivity is critical for thin film applications such as ultrafast memory, oscillators, and integrated circuits.30–35 It was also proved that CDW transition phases are correlated to film thickness and can affect the electronic and structural phases.36 Dai et al. used Raman spectrum to identify distinct transport behaviors in VTe2 films of varying thicknesses and to detect numerous charge density wave (CDW) orders in a few-layered 1T′-VTe2.37 Similarly, the Bardeen–Cooper–Schrieffer (BCS) hypothesis states that superconductivity can be intrinsic or generated by chemical, electrostatic, or mechanical doping.38 Fatemi et al. found that superconductivity in monolayer WTe2 can be tuned by altering gate voltage. At a critical gate voltage (VMITc ∼ −0.75 V), the transition from metal to insulator occurs, resulting in a superconducting state at low temperatures.39 Li et al. also demonstrated superconductivity in type-II Weyl semimetal WTe2 by proximity effect on a WTe2/NbSe2 heterostructure, with a superconducting state was observed in WTe2 at 6.4 K which was close to the critical temperature (Tc) of NbSe2.40 Zhu et al. induced superconductivity at around 2.6 K in WTe2 by intercalating potassium (K) into the van der Waals band gap, solely through electron doping, with minimal lattice structure alteration.41 TMTes exhibit topological insulating features, characterized by metallic conducting gapless surfaces and non-trivial insulating bulk gaps at the margins of 2D materials, also known as Quantum Spin Hall (QSH) insulators. Sufyan et al. predicted band topologies in monolayer ternary systems such as NbCoTe4, NbIrTe4, NbRhTe4, TaCoTe4 and TaRhTe4, showing strain-tunable magnetism, applicable in nanodevices.42 Monolayer WTe2 and MoTe2 were also reported to display topological insulator properties as given in Fig. 3.43–46
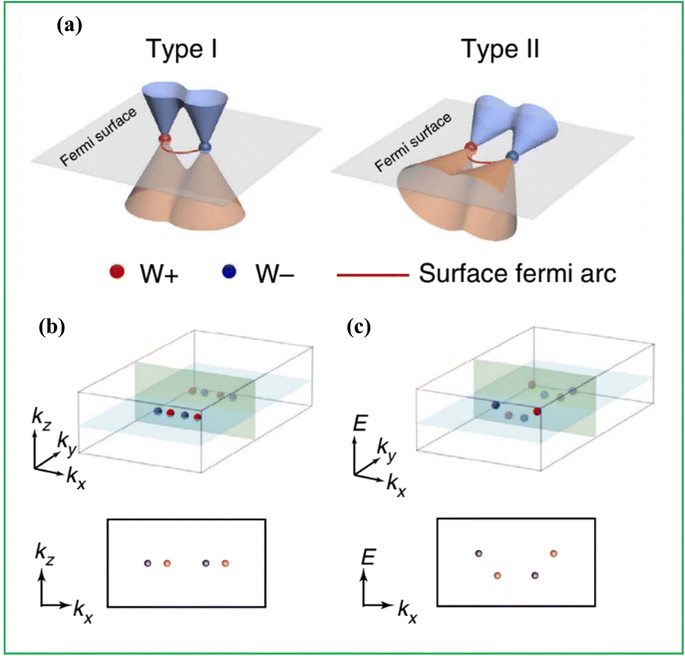 |
| Fig. 3 Schematic representation of (a) type-I and type-II Weyl fermions and Weyl points in the momentum space, (b) Weyl points of MoTe2 in a BZ and Kx–Kz plane, and (c) Weyl points in the energy-momentum space and their projection to the Kx–E plane reproduced with permission from ref. 47. | |
Additionally, TMTes have an intriguing semi metallic property and have an odd metal–semiconductor crossover. TMTes like WTe2 and MoTe2 have drawn a lot of interest because of their fascinating physical characteristics like the Type-II Weyl semimetal nature and the incredibly high magnetoresistance.48–50 WTe2 was the first identified type-II Weyl semimetal. Its unique atomic arrangement, with smaller spacing between consecutive W atoms along the x-axis compared to the y and z axes, leads to high anisotropy, resulting in novel quantum phenomena such as chiral-induced anomaly, magnetoresistance, and supersymmetry. Bulk WTe2 exhibits high and non-saturating magnetoresistance, which is especially noticeable when current flows through the Te–W–Te chains along the x-axis and a field is applied along the z-axis perpendicular to the layer stacking. Angle-Resolved Photoemission Spectroscopy (ARPES) studies of WTe2 reveal a unidirectional Fermi surface along the T–W–Te chain, as well as electron and hole pockets at the Fermi energy level, indicating outstanding topological features similar to MoTe2.51–56
3 Synthesis of TMTes
In order to produce TMTes for electrochemical storage applications, it is essential to develop scalable, affordable synthesis processes. The structural orientation, bond mechanisms, stoichiometry tuning and surface modification are determined by the synthesis procedures. Recently, a variety of methods for creating nanostructured TMTes have been devised by scientists.
3.1 Chemical vapor deposition (CVD) method
Chemical Vapor Deposition (CVD) is one of the most effective methods which combines several scientific domains like thermodynamics, chemical kinetics and fluid dynamics to produce high quality wafer-scale 2D atomic layers with large-scale uniformity, controllable thickness along with excellent physical and chemical properties. The events occurring in CVD reaction generally involves excitation of the required precursor materials to their vapor phases, the reactant gases are then transported to the substrate material where they come in contact with each other and the reactants are then adsorbed by the surface of the substrate which onsets the start of heterogeneous reaction catalyzed by the substrate surface producing the desired solid-phase materials. After that the gaseous by-products are desorbed and diffused away from the surface of the substrate by the flow of the carrier gas. A significant advantage of CVD films lies in their exceptional conformality, ensuring that the film thickness on the sidewalls of features closely matches that on the top surface. This property allows for the application of films on complex-shaped objects, including the interior and underside of features, and facilitates the complete filling of high aspect ratio holes and other features.6–9 Although CVD is effective, and has shown great promise in the synthesis of high-quality transition metal chalcogenides like sulfides and selenides, the conventional CVD still faced challenges in controllable synthesis of metal tellurides. The synthesis of stoichiometric WTe2 or MoTe2 using traditional chemical vapor deposition (CVD) methods is challenging, particularly when it comes to obtaining the 1T′ stable phase of WTe2 and MoTe2. This difficulty arises due to the low reactivity of W or Mo with Te and the small electronegativity difference (approximately 0.4 or 0.3 eV) between these elements. As a result, achieving the desired semiconducting and thermodynamically stable trigonal prismatic structure (2H phase) or the semi-metallic distorted octahedral structure (1T′ phase) is not straightforward, specifically in the case of WTe2 and MoTe2.57 To overcome this challenge, various approaches have been employed, including the utilization of rare precursors or promoters and specialized instruments. One such approach involves employing salts like sodium chloride (NaCl), potassium iodide (KI) and potassium bromide (KBr) during the CVD process to facilitate the production of monolayer metal tellurides. Zhang et al. conducted a study where they achieved the successful synthesis of few-layered, uniformly structured metastable 1T′-MoTe2 flakes by incorporating iodine as a medium in the process. The incorporation of iodine played a vital role in modifying the Mo–Te bond length, leading to the transformation of volatile intermediates required for the formation of highly crystalline 1T′-MoTe2 flakes. Additionally, the volatile nature of iodine at elevated temperatures enabled its easy removal. The resulting crystalline flakes were characterized using X-ray Diffraction (XRD), X-ray photoelectron spectroscopy (XPS), and density functional theory calculations, which confirmed the formation of uniform layers of ribbon-shaped 1T′-MoTe2 flakes measuring 46.90 μm in length and having an area of 288.54 μm as given in Fig. 4.58 Also, recently, Ma et al. reported the synthesis of CoTe2 CVD of CoTe2 onto a piece of Si as the growth substrate producing nanosheets of CoTe2 with triangular or hexagonal geometries with sizes of about 200 μm.59 Although CVD is great to accurately control the growth process, there are also many disadvantages pertaining to it. One key limitation of CVD relates to the nature of the precursors. Ideally, these substances should exhibit volatility near room temperature. Additionally, the deposition of films typically occurs at elevated temperatures, which restricts the range of substrates suitable for coating.9
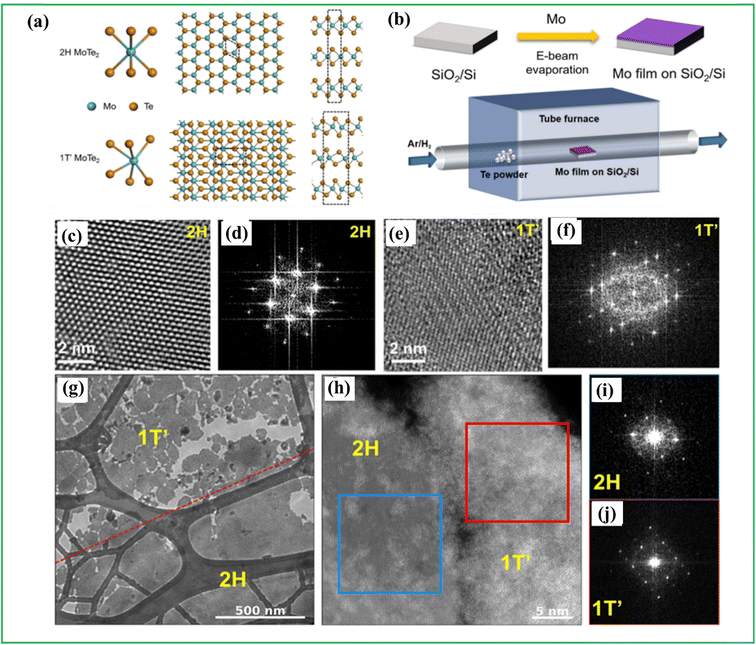 |
| Fig. 4 (a) Schematic diagram of crystal structures of 2H and 1T′ MoTe2. (b) Schematic illustration of CVD synthesis process. Transmission Electron Microscopy (TEM) images and corresponding FETs for (c and d) 2H phase and (e and f) 1T′ phase. (g) High defocus scanning TEM (STEM) Ronchigram image of the interface between 2H and 1T′ phase of MoTe2 heterostructure. (h) High-magnification STEM image of the interface (i) FFT of the region marked by a blue outline in the STEM image in (h), demonstrating that the region is single crystal and in the 2H phase. (j) FFT of the region marked by a red outline in the STEM image in (h), demonstrating that the region is a single crystalline and 1T′ phase reproduced with permission from ref. 60 with permission from [American chemical society], copyright [2024]. | |
3.2 Successive ionic layer adsorption and reaction (SILAR)
The Successive Ionic Layer Adsorption and Reaction (SILAR) method is a thin film deposition technique used in materials science and nanotechnology. It involves the sequential immersion of a substrate into solutions containing different precursor ions, allowing for the controlled buildup of thin films through ionic layer-by-layer adsorption and subsequent chemical reaction. This method is commonly used to fabricate thin films with precise control over thickness, composition, and structure, making it valuable for various applications such as solar cells, sensors, and catalysis. Researchers explain that ion adsorption onto the surface of the substrate occurs due to the presence of an attraction force between the ions in the solution and the surface of the substrate. Basically, the immersion–reaction cycle might be used to adjust film thickness, shape, and composition. An inherent advantage of SILAR lies in its ability to integrate the unique benefits of both Atomic Layer Deposition (ALD) and Chemical Bath Deposition (CBD). Like ALD, SILAR enables the deposition of a single atomic layer in a single reaction cycle. Additionally, this process can be conveniently carried out at ambient pressure, utilizing simpler setup and precursor solutions compared to other costly and complex deposition methods.10 Considering the benefits of this approach Pandit et al. for the first time anchored (VxTey) nanoparticles over multiwall carbon nanotube (MWCNT) using SILAR. A complex of triethanolomine (TEA) and vanadyl sulfate (VOSO4) used as cationic sources which will liberate VO2+ ions. Similarly, sodium tellurite (Na2TeO3) in hydrazine hydrate used as an anionic source which will liberate Te2− as given by the equations follow. | Na2TeO3 → 2Na+ + TeO32− | (1) |
| TeO32− + N2H4 → Te + N2 + 2OH− + H2O | (2) |
| 2Te + N2H4 + 4OH− → 2Te2− + N2 + 4H2O | (3) |
Here flexible steel substrate coated with MWCNT was dipped in cationic solution for an interval of 20 s. During this process VO2+ ions may be adsorbed over MWCNT due to the chemical, cohesive or van der Waal forces. Following this the same substrate was immersed in anionic precursors containing Te2−. This reaction continued to yield vanadium telluride thin film as shown in Fig. 5.60–62 SILAR has found extensive application in depositing chalcogenide films, as well as multilayer and epitaxial films, since its discovery.63 Nevertheless, despite significant efforts in this domain, such as the use of complexing agents for precursors and minimizing rinsing times, the persistently slow growth rate across various film types remains a significant drawback of SILAR. This limitation hampers its widespread adoption in the semiconductor industry.11
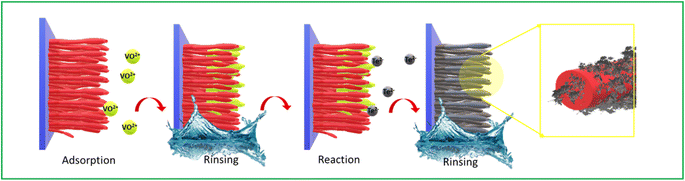 |
| Fig. 5 Schematic representation of the formation of VxTey nanoparticles over CNT using SILAR methods. | |
3.3 Hydrothermal method
One of the most adopted methods for synthesis of nanocrystalline inorganic materials is the hydrothermal method. As the name suggests, the reaction medium for this method is water, which is considered to be the most environmentally friendly solvents. Although, its physical and chemical properties like its low boiling and high polarity make it difficult for it to be used in such high temperature conditions. To overcome these limitations the high temperature (usually over 100 °C) reaction is carried out under high pressure in Teflon lined steel pressure vessels. On approaching its critical point of 374 °C and 22.4 MPa, water exhibits many unusual properties such as a change of its structure.64 The mechanism of hydrothermal synthesis is a liquid nucleation model and the parameters involved can be adjusted during hydrothermal processing to sustain a high synchronized nucleation rate and good size distribution. Surface active agents and coordinating molecules like (polyvinylpyrrolidone (PVP), ethylenediaminetetraacetic acid (EDTA), cetyltrimethylammonium bromide (CTAB), ethylene glycol, ethylenediamine, oleic acid (OA), oleyl amine (OlA)) etc. may be added to prevent aggregation and agglomeration and to adjust the size of the final nanocrystals. Reducing agents such as sodium borohydride and hydrazine may also be used to prevent the formation of impurities like oxides of oxygen due to the electropositive nature of the metal ions and the electronegativity of the tellurium ions involved.65,66 The hydrothermal synthesis method is highly advantageous compared to other growth methods for synthesizing nanomaterials due to its fast reaction kinetics, short processing times, ability to achieve phase purity, high crystallinity, high yield, low cost, environmental friendliness and reduced hazardous nature. These desirable characteristics make hydrothermal synthesis an ideal choice for producing nanomaterials.67 The preparation of nanomaterials like metal tellurides and their hybrids by hydrothermal processing has achieved great success and has provided satisfactory results. One of the earliest syntheses of TMTes by hydrothermal synthesis was done by Zhang and group. They successfully synthesized NiTe nanocrystallites and the XRD along with the Inductively Coupled Plasma (ICP) analysis of the material indicated the formation of single-phase hexagonal NiTe.68 Similarly, NiTe2 nanosheets with a vertically aligned open network-like structure were also successfully synthesized by Wang et al. using a facile two-step hydrothermal method.69 Hydrothermal method has also been proven to be effective for the synthesis of TMTe's composites. Recently, Ding et al. constructed MoTe2/rGO heterostructures with suitable Schottky barriers by adopting a simple one-pot hydrothermal synthesis. The characterization of the nanoparticles showed a uniform formation of the hexagonal monocrystalline MoTe2 nanoparticles on the surface of the rGO, showcasing the material's dispersion and homogeneity.70 Karupppusamy and group of researchers also utilized this method to prepare CdTe with boron-sulphur co-doped (CdTe/BS-rGO) nanocomposite. Morphological studies demonstrated BS-rGO nanosheet wrapped around the CdTe nanoparticles with sheet-like morphology slightly wrinkled at the edges. Structural characterization pointed towards the formation of cubic structure of CdTe and the successful formation of the CdTe/BS-rGO composite.71 While hydrothermal synthesis stands out as an environmentally friendly and cost-effective method for producing nanomaterials, a notable drawback of the conventional approach is its reliance on time-consuming empirical trial-and-error methods for process development.72 Presently, efforts are directed toward establishing a comprehensive rational engineering-based approach aimed at expediting process development.12
3.4 Electrodeposition method
Electrodeposition is a long-standing method for producing semiconducting nanomaterials, including metal tellurides with diverse structures such as nanorods and nanowires. It involves the growth of an electrodeposit from an electrolyte or the coating of the cathode surface with a metallic layer through reduction reactions in aqueous or organic solvents. This method relies on the solid-phase transformation of ionic species present in the solution and operates through a two-step process. Firstly, nucleation occurs as molecular clusters rapidly decompose and engage in heterogeneous reactions on the substrate surface. Subsequently, particle growth takes place as the particles combine and expand to form a film of a specific thickness, following an ion-by-ion mechanism.73,74 While there are alternative organic routes for synthesizing nanomaterials like metal tellurides, electrodeposition stands out as the most cost-effective approach. It operates at low temperatures without requiring a vacuum, offers high deposition rates and crystallinity, enables film fabrication on large and irregular surfaces, and allows for easy scalability. Moreover, electrodeposition occurs closer to equilibrium compared to many high-temperature methods, minimizing complications related to inter-element diffusion.75 She et al. reported a template-free approach for the synthesis of Copper Telluride (CuTe) nanoribbons. The synthesis was achieved through a typical electrodeposition process using an aqueous solution containing TeO2, copper(II) sulfate (CuSO4) and NH3·H2O. The experimental setup included Pt as the counter electrode, a standard saturated calomel electrode (SCE) as the reference electrode, and Indium-tin oxide (ITO) coated glass as the working electrode. The synthesis was carried out under basic conditions at a temperature of 85 °C for a duration of 30 minutes. The synthesized CuTe nanoribbons exhibited high purity, as confirmed by Energy-Dispersive X-ray Spectrometry (EDXS) and XPS analyses.76 Park et al. also reported the synthesis of Ag2Te nanotubes by electrodepositing tellurium on the surface of Ag nanofibers inducing in a chemical reaction which results in the formation of the desired nanotubes (Fig. 6).77 Khalafallah et al.78 reported the synthesis of nickel cobalt selenide@nickel telluride core shell architecture (NixCo12−xSe@NiTe) by a two-step electrodeposition method. Firstly, a precleaned Ni foam (NF) as the working electrode was immersed in an electrolytic bath containing the precursors to grow the NiTe dendritic frameworks onto the NF. The electrodeposition was done in a three-electrode system with SCE as the reference electrode and graphite rod as the counter electrode while applying a constant potential of −0.6 V vs. SCE for 300 s. Secondly, the NixCo12−xSe shell onto the prepared NiTe film-modified NF by electrochemical deposition with a fixed potential of −0.8 V vs. SCE for 200 s. After electrodeposition, the samples were washed several times and dried at 60 °C overnight. The growth of the freestanding NixCo12−xSe@NiTe heterostructures on the Ni foam was confirmed by XRD, Field Emission Scanning Electron Microscopy (FESEM), and TEM analysis of the prepared samples.
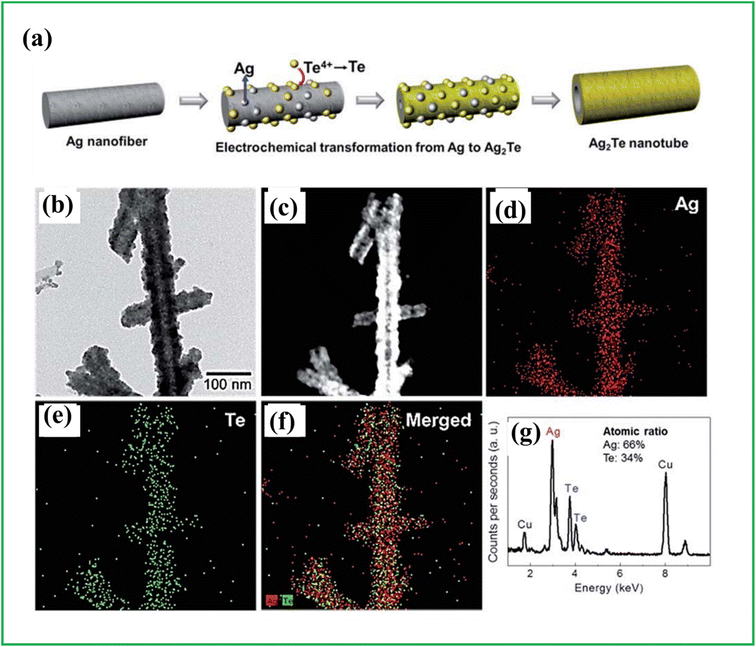 |
| Fig. 6 (a) Schematic representing fabrication of Ag2Te nanotubes by electrochemical deposition method. (b) TEM images, (c) high-angle annular dark-field imaging (HAADF)-STEM image, (d–f) energy-dispersive X-ray spectroscopy (EDS) mapping and (g) EDS pattern, reproduced from ref. 79 with permission from [Royal society of chemistry], copyright [2024]. | |
3.5 Molecular beam epitaxy (MBE)
MBE, which stands for Molecular Beam Epitaxy, is a meticulous and regulated technique employed to cultivate epitaxial crystalline thin films. It involves the reaction of one or more molecular beams on a crystalline surface within an environment of extremely high vacuum.80,81 This technique is commonly employed for the growth of epitaxial layers in a wide range of semiconducting materials. One of the advantages of this method is that the slow reaction rate of MBE enables exceptional dimensional control, allowing for precise composition variation and accurate incorporation of impurities.82 One of the disadvantages though is with the increased generation of defects during growth, as deposited atoms have reduced mobility and often need to traverse the substrate to find the optimal ‘epitaxial’ site.13 Recently, Lasek et al.83 demonstrated the growth of monolayered VTe2, TiTe2 and also CrTe2, a compound that is only metastable in its bulk form, with octahedral 1T growth structures by MBE. He also reported the multilayer growth of intercalated transition metals between the transition metal dichalcogenides for all the three TMTes mentioned above. Table 1 shows the summary of synthesis approach used for the synthesis of TMTes.
Table 1 Various reported synthesis techniques for TMTes
Material |
Precursors |
Additives |
Method |
Temp (°C), time (h) |
Template |
Ref. |
HgTe |
Mercury chloride, sodium telluride |
Hydrazine hydrate, ammonia |
Hydrothermal |
170 °C, 12 h |
Nil |
84
|
Ag2Te |
Silver nitrate, sodium telluride |
Hydrazine hydrate, ammonia |
Hydrothermal |
120 °C, 12 h |
Nil |
85
|
CoTe, CoTe2 |
Cobalt nitrate, H2TeO3 |
Hydrazine hydrate |
Hydrothermal |
140 °C, 24–48 h |
Nil |
86
|
Ag2Te |
Silver nitrate, tellurium |
Polyvinylpyrrolidone |
Electrospinning, thermal reduction, electrodeposition |
|
Ag nanofibers |
77
|
CdTe |
Ag2Te, cadmium nitrate |
Tributylphosphine |
Electrospinning, electrodeposition, cation exchange |
50–90 °C, 3.5 h |
Ag nanofibers |
87
|
CuTe |
TeO2, CuSO4 |
NH3·H2O |
Electrodeposition |
85 °C, 30 min |
Indium–tin oxide (ITO) coated glass |
76
|
MoTe2 |
MoO3, Te powder |
NaCl |
CVD |
670 °C, 690 °C, 710 °C |
Nil |
88
|
CoTe2 |
Cobalt chloride, tellurium powder, SiO2 |
|
CVD |
420 °C, 615 °C |
Nil |
59
|
CrTe2/graphene |
CrTe2, graphene/SiC substrate |
Nil |
MBE |
375 °C |
|
89
|
4 Factors influencing charge storage mechanism in TMTes
The evolution of TMTes can be summarized as given in Fig. 7(a). TMTes have a large surface area that facilitate ion adsorption and energy storage. When voltage is applied to electrode materials like TMTes, the charge is stored by transferring electrons from the current collector to the electrode. This electric charge is often stored on the electrode surface by either an electric double layer or surface redox processes. Understanding the role of active sites and ion insertion is critical for understanding how energy is stored and released in various electrochemical energy storage systems. It is critical to understand that active sites and surface area are distinct concepts. While it is commonly assumed that a greater surface area always leads to better performance, this is not always the case, especially in the case of electrochemical applications. Surface area refers to the total outer area of the electrode material exposed to the electrolyte. However, superior performance is not only solely determined by the surface area, but also other factors such as active sites, porosity, surface chemistry and conductivity etc. also play crucial roles.90 Therefore, materials with lower surface area might still exhibit excellent performance if they possess favorable properties. Active sites denote specific locations within the electrode material where ion adsorption and redox reactions take place. A larger surface area does offer more contact points for ion adsorption, potentially enhancing the overall electrochemical performance by increasing the availability of active sites for ion storage and reaction. Active sites act as binding sites for ions from the electrolyte, facilitating their storage within the electrode material or their participation in electrochemical reactions.91,92 Various charging mechanisms, such as ion exchange, ion desorption, and ion adsorption, occur within these active sites, influenced by the polarization of the electrodes and the selected ions. These mechanisms have the potential to alter ion diffusion by modifying the density of ions within the pores and the kinetics of ion diffusion.14
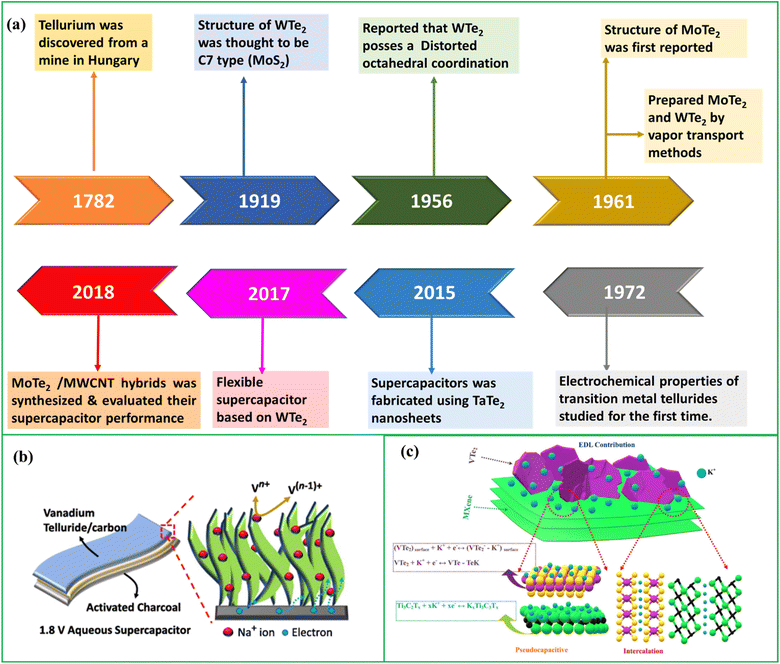 |
| Fig. 7 (a) History of TMTes, (b) charge storage mechanism in VxTey/CNB reproduced ref. 110 with permission from [Elsevier], copyright [2024] and (c) charge storage mechanism in VTe2/MXene hybrid system, reproduced with permission from ref. 94. | |
Ion insertion on the other hand refers to the processes by which the ion from the electrolyte is inserted or intercalated into the structure of the electrode material while charging. Insertion mechanism of ions usually follows several steps, where depending on the nature of the ions and surface properties of TMTes, the ions may undergo adsorption into the surface or penetrate into the interlayer spaces between the layers. Typically, ions penetrate into the pristine TMTes primarily from the edges, thereafter diffusing along the interlayers.15 The interlayer insertion mechanism is particularly relevant with layered structures, as the weak van der Waals forces allows ions to intercalate between the layers. The insertion mechanism can be influenced by various factors, including the size and charge of the ions, the structure and composition of the TMTes and the external conditions such as the temperature and applied potential. Furthermore, the impact of potential value on ion selectivity is a critical aspect to consider. The applied potential can affect the energetics of the ion insertion, influencing the rate and extent of the ion uptake into the material. By varying the applied potential, researchers can modulate the ion selectivity of the material, enabling the preferential insertion of specific ions over others.16 Aside from this, TMTes have a high specific capacitance, which allows for higher charge storage in a smaller volume of electrodes. Pandit et al. in detail explained the charge storage mechanism in a composite composed of VTe2 and MWCNT. In this hybrid system the charge is stored by utilizing both electric double layer (EDL) capacitance and surface–redox reactions. Here, the CNT helps in the formation of an EDL layer in between the electrode and the electrolyte, whereas VTe2 acts as an active electrode and undergoes redox reactions during charging/discharging cycles. During charging electrolyte ions will intercalate into the porous of VTe2 and results in the reduction of V5+ to V4+ and the oxidation of Te2− to Te. This process results in charge storage via faradaic redox reactions. During discharging the reverse process happens where vanadium undergoes oxidation from V4+ to V5+ where Te undergoes reduction from Te to Te2−. This reaction releases the stored charge via faradaic reactions.93 Similarly, in the case of VxTey/carbon nanobelts (CNBs) the EDL layer formed at the electrode–electrolyte interfaces. The double layer is essential for storing charges as it establishes a voltage disparity between the electrode and the electrolyte, allowing for charge separation. Throughout the charge–discharge process, VxTey/CNBs experience faradaic redox reactions with electrolyte ions, leading to ion intercalation and charge accumulation within the electrode structure. The impressive capacitive behavior of VxTey/CNBs is attributed to the presence of surface functional groups, such as hydroxyl and carboxyl groups, which foster the formation of an electrical double layer at the interface between the electrode and the electrolyte through electrostatic interactions.
The charge storage can be summarized as given below the eqn (4) and given in Fig. 7(b)
| VxTey + zNa + ze−1 ↔ Vx−zTeyNazz+ | (4) |
Zaw et al. investigated the hierarchical synthesis of metal-telluride nanomaterials over nanoclays as anodes for supercapacitors. The charging mechanism of this setup involves a fusion of pseudocapacitance and intercalation. Nanostructured metal tellurides combined with clay exhibit a hierarchical arrangement, boasting a sizable surface area that facilitates the smooth intercalation of electrolyte ions into the electrode material. These nanostructures display pseudocapacitive traits due to their inherent redox activity. As the charge–discharge cycle progresses, the metal telluride engages in reactions with the electrolyte, enabling charge storage through pseudocapacitance. Incorporating metal telluride with clay enhances the intercalation dynamics.
Sreeraj et al. fabricated a VTe2/(Ti3C2Tx) MXene-based asymmetric supercapacitor utilizing VTe2/MXene as positive electrode and MoS2/MXene as negative electrode. The transition metal promoted greater interfacial contact between MXene flakes, facilitating the creation of shorter ion diffusion channels. The charge storage mechanism involves intercalation and pseudocapacitance is as follows eqn (5)–(7) as shown in Fig. 7(c).
| (VTe2)surface + K+ + e− ↔ (VTe2–K+)surface | (5) |
| VTe2 + K+ + e− ↔ VTe − TeK | (6) |
| Ti3C2Tx + xK+ + xe− ↔ KxTi3C2Tx | (7) |
4.1 Morphological effects on electrochemical performance
4.1.1 Surface area and porosity considerations.
Nano/microstructure and morphology are intimately related to SC performance, which influences charge/mass transport during electrochemical processes. Achieving optimal energy storage device performance relies on the structure and morphology of electrode materials should have a high specific surface area, ample active sites, and structural durability. Effective structural design is key to preventing active material aggregation, thereby enhancing electrode material cycling performance. Consequently, researchers focus on synthesizing numerous metal tellurides with varied morphologies and nano/microstructures, adjusting synthesis parameters like reaction temperature, duration, and reactant ratio to control the outcomes. For example, Mao et al. synthesized NiTe2 using a simple hydrothermal approach at 160 °C. They observed a nanoflower morphology and this may provide lot off reaction sites for the electrochemical reactions to happen and also these thin flakes helps to increase the contact area for the electrolyte and also helps to enhance the ion transfer rates during the time of electrochemical reactions.95 Porous nanomaterials also gained lot of attention in the field of supercapacitors due to their interesting functionalities and the usage of the nanochannels. The remarkable electrochemical performance of the cobalt telluride-carbon porous structure formed from a metal–organic framework highlights the promise of porous metal tellurides in improving electrochemical capabilities. This porous nature stands out as an intriguing path for improving and changing electrochemical capabilities, demonstrating significant potential for advancement in this field.
While the outer surface characteristics of these porous structures may be less remarkable, their interior and wall properties hold greater interest. By harnessing both the inner and outer surfaces, along with the tubular wall, 1D tubular nanomaterials featuring discrete and single nanochannels with high aspect ratios can exhibit cooperative and synergistic behaviors. Thus, 1D hollow NiTe2 nanotubes synthesized using simple hydrothermal approach also shows exceptional electrochemical performances. This could be owing to their high electrical conductivity and structural benefits of 1D structures, which ensure sufficient ion diffusion channels during electrochemical charging/discharging phenomena. Similarly, Ni0.33Co0.67Te nanotubes are found to be conductive to transport the ions/electrons and were able to deliver capacitance of nearly 131.2 mA h g−1 at 1 A g−1.96 Heterogeneous core–shell nanostructures have emerged as a sought-after subject in the realm of high-performance supercapacitors. Thanks to their interconnected design, these nanostructures not only facilitate the creation of hierarchical porous pathways for efficient charge transfer but also boast enhanced electrical conductivity and structural integrity. Based on these properties of core–shell structures Ye et al. constructed a NiTe@NiCoSe2 core/shell nanostructure using an electrodeposition method. Here NiCoSe2 nanoparticles were grown over NiTe nanorods where NiTe act as building network that will provide enough active area surfaces and enhances the electrochemical activity. This core/shell electrode is stable, as evidenced by its exceptional durability (96.6% capacitance retention after 10
000 cycles).97 The findings suggest that TMTes hold promise as electrode materials for practical applications in supercapacitors as given in Fig. 8.
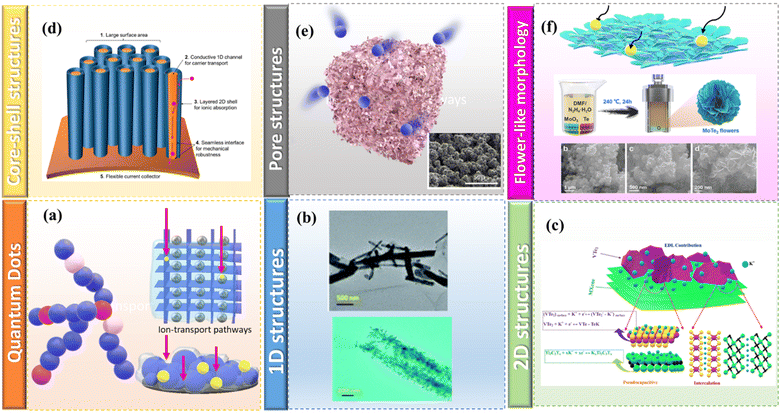 |
| Fig. 8 Various types of morphology of TMTes (a) quantum dot, (b) 1D structures reproduced with permission from ref. 139, (c) 2D structures reproduced with permission from ref. 94, (d) core–shell structures reproduced with permission from ref. 103 with permission from [American chemical society], copyright [2024], (e) porous structures reproduced from ref. 104 with permission from [American chemical society], copyright [2024], (f) flower-like morphology reproduced ref. 105 with permission from [John Wiley and Sons], copyright [2024]. | |
4.1.2 Influence of crystal structure and phase composition.
The capacitive behavior can be greatly influenced by the crystal structure and phase composition. Deshagani et al. showed that altering the crystal structure of a material can have a huge impact on its morphology and simultaneously its electrochemical behavior. In their work, NiTe was doped with Se to obtain a morphological transformation from fibrillar NiTe to a flaky NiTe:Se. The introduction of Se into the crystal structure of NiTe as a dopant altered the morphology, resulting in a different particle structure compared to the pristine NiTe material. This Se doping decreased magnetic character, as proven by Magnetic Force Microscopy-Atomic Force Microscopy (MFM-AFM). Uniform Se distribution over NiTe increased surface roughness 2 times, improved electrical conductivity 8-fold, and facilitated rapid charge transport and ion/electron movement in NiTe: Se. This was reflected in a capacity of 565 C g−1 (or a specific capacitance of 943 F g−1) for the NiTe:Se electrode, which is 1.5 times higher than that of the NiTe electrode.98 The crystal phase in which the material is present in can bear a significant change in the supercapacitive behavior of the material. Take, for instance, Ni and Manganese (Mn), which comprise numerous phases can exhibit varying electrochemical performances depending on the phase its present in ref. 99. One of the works by Bommineedi and coworkers revealed the formation of higher electroactive surface area and surface roughness through the formation of a mixed phase FeTe:Fe2TeO5. The assembly of embedded quantum dots, forming a surface structure resembling nanopebbles, enhances the material's hydrophilic properties. This improvement is evidenced by reduced contact angles and increased surface roughness observed in atomic force micrographs. This morphology facilitates deeper penetration of electrolyte ions into the electrode surface, leading to an increased inner charge contribution. As a result of this structural modification, the material offers a larger electroactive surface area for interaction with electrolyte ions, leading to a significant specific capacitance of 591 F g−1 (166 mF cm−2) at a potential scan rate of 3 mV s−1 and 107 F g−1 (30 mF cm−2) at 0.4 mA cm−2 in NaCl electrolyte.100 Nevertheless, it has been observed that numerous TMTes, including monolayer Cr2Ge2Te6, exhibit instability when exposed to atmospheric conditions. For instance, the aforementioned material tends to degrade, while exfoliated VTe2 nanosheets react with moisture and oxygen, resulting in the formation of surface bubbles.101 To augment the charge storage capacity, one viable approach is to enhance the specific surface area and interlayer spacing of the material. This can be achieved by fabricating heterostructures that possess enhanced functionality.102In lieu of this, recent developments have been focused on different strategies for the designing of these heterostructures, which are discussed below.
4.2 Device engineering
Materials engineering and several modification techniques have been developed for fabricating electrodes for supercapacitors with high power density, enhanced charge storage capacity and long-term stability. Supercapacitors typically utilize a symmetric configuration where two similar capacitive electrodes (negative and positive electrodes) are separated by a separator and immersed in an electrolyte. However, this setup limits the working voltage of the devices to the potential of the symmetric cell. The energy density of such devices is directly related to the integrated area under the charge–discharge curve, underscoring the need to expand the working voltage. Asymmetric designs have garnered significant attention due to their potential to address this limitation. These designs incorporate two different charge storage electrodes, each active in distinct potential windows. When combined, these electrodes offer a broader working voltage range. Additionally, the disparate water decomposition potentials of these electrodes, with varying overpotentials for hydrogen and oxygen evolution, can further extend the working voltage beyond the theoretical water splitting potential range of 1.23 V. Consequently, asymmetric assemblies are poised to significantly enhance the energy density of aqueous supercapacitors. It's important to emphasize that supercapacitors primarily serve as power devices. Advancements in achieving high energy density electrodes involve not only the fabrication of capacitive and pseudocapacitive charge storage materials but also the development of battery-like and hybrid materials. These hybrid materials combine aspects of both batteries and capacitors to optimize power output. In asymmetric designs, maintaining maximum power response is crucial. To achieve this, capacitive TMTes are often incorporated into one of the electrodes, despite their relatively modest energy density resulting from limited pseudocapacitive behaviour.
Carbon materials can store charge in negative regions of potential window. Thus, it is possible to combine carbon materials as negative electrodes and TMTes as positive electrode to deliver high power density, high capacitance and long-term stability. Asymmetric combinations have shown tremendous promise in increasing the energy density of supercapacitor cells while maintaining sufficient power responsiveness, even when using aqueous electrolytes. Notably, designs with battery-like responsive electrodes are commonly referred to as hybrid supercapacitors.
Supercapacitors with high energy density have become a key focus of current research and development efforts. For example, the development of miniaturised devices, as well as the appearance of flexible and wearable electronics, necessitates the use of dependable micro-supercapacitors and/or flexible supercapacitors. These specialised designs are required to fulfil the applications' unique form factors and performance requirements, resulting in smooth integration and excellent functionality.
4.2.1 Electrolyte modification.
Electrolytes serve as vital components in supercapacitors, affecting various aspects of their functionality. Firstly, the type of electrolyte greatly impacts ion transport processes within the cell and at electrode interfaces, as well as the charge storage mechanisms in supercapacitors. Furthermore, the size and charge of electrolyte ions play a critical role in determining the electrochemical double-layer capacitance (EDLC) of the supercapacitor. Smaller ions can access more surface area on the electrode, as the pore structure and ion's size can strongly impact the capacitance and energy storage capacity. Additionally, the redox potentials of different electrolyte ions dictate the maximum voltage window of the supercapacitor, which directly influences its energy density. The choice of electrolyte also determines the electrochemical stability window of the supercapacitor, ensuring safe and reliable operation within specified voltage ranges. The electrolyte & potential window plays a critical role in determining the voltage (V) and, consequently, achieving higher energy density, which follows a quadratic dependence on V. Additionally, the selection of electrolyte, along with electrodes optimized for pore size, significantly influences the equivalent series resistance (ESR). Finally, it is also possible to enhance the selectivity and sensitivity of electrochemical sensors integrated into supercapacitors, by adjusting the composition of the electrolyte, thereby improving their detection accuracy. Thus, proper selection and management of electrolytes are essential for optimizing the performance, longevity, and safety of supercapacitors, making them key considerations in the design and operation of these energy storage devices.106,107
4.2.1.1 Types of electrolytes.
Electrolytes are classified as aqueous (protic electrolytes), aprotic electrolytes, ionic liquid, organic and quasi-/all-solid-state electrolytes based on their ion-conducting medium. Because of their simplicity, low cost, and excellent ion conductivity, aqueous electrolytes are the most often utilized. Neutral, acid and alkaline electrolytes are represented by K2SO4, H2SO4, and KOH, respectively. However, due to the water decomposition phenomenon, aqueous electrolyte was able to provide only a small potential window (1–1.2 V), which limits the energy density. Thus, to prevent unwanted oxygen and hydrogen evolution reactions it has been suggested to increase the salt concentration or alkalinity of aqueous electrolytes. On the other hand, overconcentrated electrolytes reduce ionic conductivity and cause electrode surface corrosion, resulting in poor cycle stability. Aprotic electrolytes have a larger operating voltage window than water soluble electrolytes, resulting in a higher energy density. Among the most commonly used aprotic electrolytes are lithium salts such as lithium perchlorate (LiClO4), lithium hexafluorophosphate (LiPF6), and lithium tetrafluoroborate (LiBF4) mixed in a variety of solvents such as ethylene carbonate (EC), diethyl carbonate (DEC) and dimethyl carbonate (DMC). For example, Kumbhar et al. synthesized a thin film of Sm2Te3 using SILAR approach and this Sm2Te3 thin film supercapacitive performance was evaluated using lithium perchlorate–propylene carbonate (LiClO4–PC). The electrolyte selection is critical due to its critical function in promoting charge transfer and ion mobility. They also investigated the electrolyte concentration effect and observed that beyond 1.5 M concentration, capacitance decreases, possibly due to a decrease in the number of solvation cells around electrolyte ions. Considering the benefits of LiClO4 Karade et al. constructed a flexible symmetric supercapacitors using PVA-LiClO4 gel, resulting in enhanced capacitance and energy density due to enhanced potential window.108 Despite being another possible alternative, there have been no reports on using organic electrolytes to expand the potential window of supercapacitors based on VTe2 metal tellurides.
Solid-state electrolytes have several advantages over liquid electrolytes: (1) they eliminate the need for additional separators, simplifying manufacturing and lowering costs; (2) they prevent liquid leakage, making devices safer; and (3) they are suitable for flexible devices like portable electronics and wearables. A gel polymer electrolyte is a quasi-solid-state electrolyte consisting of a liquid solvent and polymer. Poly (vinyl alcohol) (PVA) is a popular polymer matrix with LiCl, Na2SO4, KOH, and other aqueous solutions. Pandit et al. gave one of the typical examples wherein he used a PVA-LiClO4 gel to construct a flexible VxTey/MWCNTs-based symmetric supercapacitor. This device was able to operate in a potential window of 2 V and able to deliver energy density of nearly 34.5 W h kg−1 energy density at a power density of 0.7 kW kg−1.93 Despite challenges such as high cost, difficult manufacturing, poor conductivity, high interface impedance, and compatibility issues, electrolyte advancements remain promising.
4.2.1.2 Redox additive.
Faradaic redox reactions at the electrode–electrolyte interface have the potential to generate substantially higher pseudocapacitance than electrochemical double-layer capacitance caused by electrostatic ion adsorption. By incorporating redox additives into the electrolyte, supercapacitors' specific capacitance can be increased by supplementing the pseudocapacitance above the intrinsic capacitance of the electrode materials. Prangya et al. studied that the performance of NiLaTe microfibers (NiLaTe MFs) over the Ni foam in bare KOH solution and after the addition of potassium ferrocyanide (KFC). Their studies reveal that the performance of NiLaTe MFs can be doubled by the addition of aqueous KFC solution which allows faster transport of ions inside the active electrode surfaces and thereby enhancing the electrochemical performances.109 Similarly, Ye et al. demonstrated that the addition of redox additive K4Fe(CN)6 into alkaline electrolyte (KOH) can increase the energy density of asymmetric supercapacitor composed of CoTe//AC. When 0.03 M K4Fe(CN)6 is added, the CV curve expands, indicating that the addition of a redox additive like K4Fe(CN)6 can increase specific capacitance. The CV curve containing a redox additive exhibits a redox peak, potentially arising from a redox reaction involving the Fe(CN)63−/Fe(CN)64− redox pair. The possible redox is as follows eqn (8). | Fe(CN)64− ↔ Fe(CN)63− + e− | (8) |
5 Electrochemical behavior of specific TMTes
5.1 Vanadium-based tellurides: structural insights and capacitive behavior
Vanadium(V)-based materials are gaining more attention in the field of high-performance SCs. These materials have a number of favorable properties, including a distinct layered structure, the ability to achieve numerous oxidation states, and unusual physical and chemical properties. Furthermore, their intrinsic non-toxicity adds to their attraction, making them a critical class of electrode materials in the search of better supercapacitor technology. The 2D layered structure of TMTes, like VTe2, offers an expanded surface area, enhancing charge accumulation and ion diffusion within the layers, thus improving their redox capabilities. However, challenges such as low inherent conductivity, susceptibility to degradation due to volume fluctuations, and the tendency for layered interfaces to reassemble hinder the efficiency of TMTes in energy conversion and storage applications. To address these limitations, the creation of composites offers a promising solution. By blending TMTes with carbon materials like graphene, MWCNT, and conducting polymers, among others, these composites can overcome the constraints of TMTes, enhancing their performance and stability for various applications.
Thus, Panda et al. synthesized VxTey/MWCNT hybrid using SILAR method. Here, the hybrid formation with CNT clearly demonstrate that hybrid take advantage of both insertion/extraction mechanisms and EDLC behavior. Even at 2 mV s−1 this hybrid was able to deliver capacitance of nearly 470 F g−1. The Trassati studies demonstrate that the hybrid exhibits 68.2% participation from inner charge contribution, highlighting MWCNTs' distinctive contribution of its EDLC characteristics within the hybrid system. The device fabricated using the same hybrid shows an operating potential window of 0–2 V with excellent stability and energy/power density of around 34.5 W h kg−1/0.7 kW kg−1. DFT simulations demonstrate the synergistic interactions between VxTey and CNT, as well as an increase in electron density at the Fermi level of the VxTey/CNT hybrid.93 Similarly, Rathore et al. synthesized another hybrid of VxTey and MWCNT using a tubular furnace. The precursors used include ammonium metavanadate and tellurium as VxTey and melamine as carbon source. The steps involved in the formation of Vanadium telluride include eqn (9) and (10).
(i) Decomposition reaction temperature at 390 °C
| 2NH4VO3 → V2O5 + 2NH3 + H2O | (9) |
(ii) Formation of vanadium telluride
| 2V2O5 + 13Te → 4VTe2 + 5TeO2 | (10) |
This hybrid electrode composed of VTe2/CNT delivered capacitance of nearly 73.5 F g−1 with an areal capacitance of around 166.5 mF cm−2 at 10 mV s−1. The inclusion of micro and meso-pores within the hybrid structure enhances the transport of electrolytic ions during electrochemical reactions. The primary mechanism of charge storage involves reversible faradaic redox reactions, which entail the insertion and extraction of Na+ ions, along with shifts between different oxidation states of V. The give eqn (11) summarizes the mechanism
| VxTey + zNa+ + ze− → Vx−zTeyNazz+ | (11) |
The GCD curves demonstrated a non-linear curve and able to deliver capacitance of nearly 103.0 F g−1 with areal capacitance of nearly 233 mF cm−2. The same electrode exhibits coulombic efficiency of nearly 70% after 5000 cycles. These studies demonstrate that the presence of carbon and Te in this hybrid helps to increases the electrical conductivity, resulting in good rate capability. Furthermore, carbon aids in the prevention of dissolution or oxidation of V-based compounds during electrochemical processes in aqueous electrolytes.110
Recently, from our group Sree Raj et al.94 for the first time synthesized a hybrid composed of VTe2/Ti3C2Tx MXene heterostructures by a simple hydrothermal method. In this composite, MXene served as a highly conductive backbone for the VTe2 nanosheets while also introducing structural defects in the material's composition. The VTe2 as well as VTe2/MXene shows a quasi-rectangular CV curve as shown in Fig. 9(a) which may be due to possible insertion and exertion of K+ ions. The capacitive and diffusive contribution was shown in Fig. 9(b). Similar to the CV curves, GCD curves given in Fig. 9(c) and (d) shows similar responses with maximum capacitance around 250 F g−1 at 0.2 A g−1 which could be attributed to the enhanced interlayer spacing of VTe2/MXene (Fig. 9(e)). The asymmetric cell constructed demonstrated maximum energy density/power density around 46.3 W h kg−1/6400 W kg−1 (Fig. 9(f–h)) and able to retain 87% of its initial capacitance even after 7000 GCD cycles. The summary of the energy storage performance of supercapacitors based on vanadium tellurides is given in Table 2.
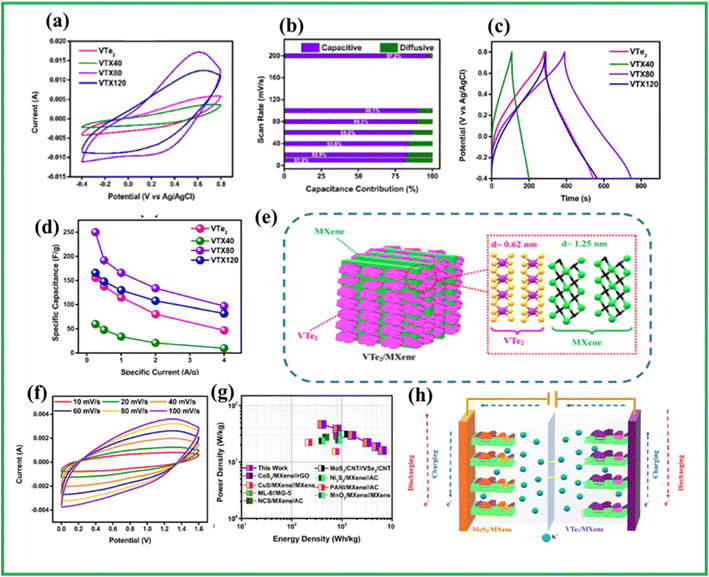 |
| Fig. 9 (a) CV curves of VTe2/MXene (b) capacitive and diffusive contribution of VTe2/MXene (c) GCD curves of VTe2/MXene with varying concentration of MXene (d) specific capacitance vs. specific current graph (e) interlayer spacing of VTe2/MXene (f) CV curve of asymmetric VTe2/MXene//MoS2/MXene ASC (g) Ragone plot of VTe2/MXene//MoS2/MXene (h) schematic representation of the during the charging and discharging process in VTe2/MXene//MoS2/MXene, reproduced with permission from ref. 94. | |
Table 2 Summary of the energy storage performance of supercapacitors based on vanadium tellurides
Electrodes |
Electrolytes |
Capacitance (F g−1) |
Energy density (W h kg−1) and power density (kW kg−1) |
% Of capacity retention |
Ref. |
VxTey/MWCNT |
PVA-LiClO4 |
62.2 |
ED = 34.5 and PD = 0.7 |
82.5% after 10 000 cycles |
93
|
VxTey/MWCNT |
103.0 |
— |
70.2% after 5000 cycles |
VxTey/MWCNT//AC |
1.0 M Na2SO4 |
42.8 |
ED = 19.3 |
70.4% after 3000 cycles |
110
|
PD = 89.9 |
VTe2/MXene |
0.5 M K2SO4 |
250 |
ED = 46.3 |
87% after 7000 cycles |
94
|
VTe2/MXene//MoS2/MXene |
130 |
PD = 400 |
5.2 Molybdenum-based tellurides: structural insights and capacitive behavior
Liu et al. for the first time synthesized ultrathin 1T′-MoTe2 nanosheets and evaluated its electrochemical performances. Fig. 10(a) illustrates the growth mechanism of 1T′-MoTe2 nanosheets. The anodic peak at Fig. 10(b) corresponds to the extraction of K+ ions between the MoTe2 layers. Remarkably, they achieved a high specific capacitance of 1393 F g−1 at a current density of 1 A g−1 (Fig. 10(c)), and 714 F g−1 at 100 A g−1. To further assess their performance, an asymmetric supercapacitor was fabricated using 1T′-MoTe2 as the positive electrode and activated carbon (AC) as the negative electrode. This configuration yielded a specific capacitance of 158.9 F g−1 at a current density of 1 A g−1. Moreover, the MoTe2//AC system demonstrated a high energy density of 56.4 W h kg−1 at a power density of 800 W kg−1.111 Hu et al. exfoliated these MoTe2 using a liquid-phase exfoliation method with N-methyl pyrrolidone (NMP) as the solvent, as shown in Fig. 10(d). The CV curve in Fig. 10(e) clearly reveals that the exfoliated MoTe2 has higher capacitance. The cathodic peaks around 0.26 V most likely correspond to the insertion of K+ ions and the anodic peaks at 0.4 V are most likely caused by the electrochemical extraction of K+ ions from MoTe2, whilst the evident into the MoTe2 layered structure. The GCD curve exhibits Fig. 10(f) comparable results, with the specific capacitance of bulk MoTe2 being 271 F g−1 and exfoliated MoTe2 having a capacitance approximately four times that of bulk MoTe2, which was roughly 859 F g−1. Furthermore, the solution resistance (Rs) in the high-frequency region is 0.72 Ω (Fig. 10(g), which is much lower than the bulk material's value of 1.96 Ω. This shows that MoTe2 nanosheets have considerably higher charge and discharge rates, which is consistent with the GCD results.112
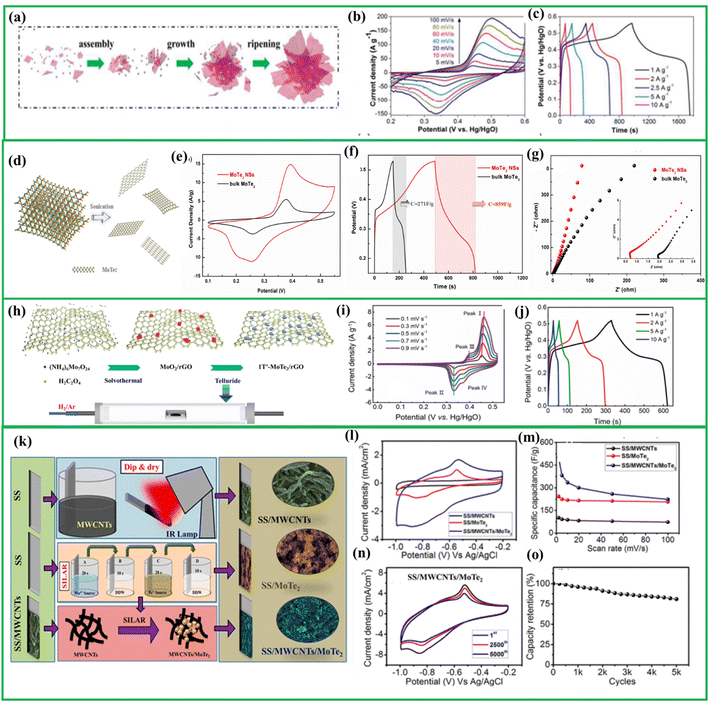 |
| Fig. 10 (a) Schematic illustration of growth process of 1T′ MoTe2, (b) CV curves of 1T′ MoTe2 (c) GCD curves of 1T′ MoTe2 reproduced from ref. 111 with permission from [Royal Society of Chemistry], copyright [2024], (d) schematic diagram showing exfoliation of MoTe2 (e) CV curves of exfoliated MoTe2 and bulk MoTe2 (f) GCD curves of exfoliated MoTe2 and bulk MoTe2 (g) Nyquist plot of exfoliated MoTe2 and bulk MoTe2 reproduced with permission from ref. 112. (h) Schematic diagram showing synthesis of 1T′ MoTe2 over rGO using solvothermal followed by tellurization (i) CV curves of 1T′ MoTe2/rGO hybrid (j) GCD curves of 1T′ MoTe2/rGO hybrid reproduced from ref. 115 with permission from [American chemical society], copyright [2024], (k) schematic illustration of development of thin films based on SS/MWCNT/MoTe2, (l) comparative CV curves of SS/MWCNT,SS/MoTe2 and SS/MWCNT/MoTe2, (m) specific capacitance vs. scan rate graph of SS/MWCNT,SS/MoTe2 and SS/MWCNT/MoTe2, (n) CV curves of SS/MWCNT/MoTe2 at 100 mV s−1 after different cycles (o) capacity retention of SS/MWCNT/MoTe2 after 5000 cycles, reproduced from ref. 108 with permission from [American chemical society], copyright [2024]. | |
The graphene-MoTe2 and composites with TMTes is widely studied. Sarwar and group developed MoTe2/graphene hybrid utilizing a microwave-assisted synthesis route. The composite material, combining the pseudocapacitive behavior of MoTe2 with the electric double-layer capacitance (EDLC) behavior of graphene, resulting in enhanced performance. This hybrid was able to deliver specific capacitance of around 434 F g−1 with high capacitive retention of 98% even after undergoing 10
000 cycles.113 In another case, Liu et al. reported the fabrication of lamellar 1T′ MoTe2@rGO hybrid by in situ colloidal synthesis. The capacitance of the composite material was drastically enhanced, reaching 1791 F g−1. Similarly, Abdullah and group MoTe2/rGO using a simple hydrothermal approach.114 In contrast to these synthesis approaches Li and colleagues utilized a different method to construct the 1T′ MoTe2/rGO heterostructure. Initially they synthesized MoO2/rGO utilizing a simple hydrothermal approach followed by tellurization to yield MoTe2/rGO heterostructure as shown in Fig. 10(h). The CV graph Fig. 10(i) display analogous patterns characterized by distinct redox peaks, signifying the electrochemical insertion of K+ ions and a reversible reaction between Mon+ ions in varied valence states of +4 and +6. This suggests that the capacitance contribution is primarily driven by the faradaic redox reaction. Increasing the sweep rate results in approximately linear variations in peak currents, confirming the electrode's strong ion diffusion and electron transport capabilities. The GCD curves of heterostructure as given in Fig. 10(j) also demonstrate a non-linear curve which demonstrate its pseudocapacitance behavior.115 These studies clearly show that the combination of controlled morphology, higher purity, lower defect density, optimized surface area, and improved crystallinity achieved through colloidal synthesis contributes to the enhanced electrochemical performance of 1T MoTe2 compared to other synthesis methods such as hydrothermal and microwave synthesis.
Similar to graphene utilizing the material mutualism Karade et al. constructed a nanocomposite containing MoTe2 and MWCNT utilizing a SILAR approach as given in Fig. 10(k). Here SS/MWCNTs as given in Fig. 10(l) displays a rectangular CV curve, suggesting the EDL behavior with a lower current distribution. Conversely, the SS/MoTe2 electrode demonstrates faradaic (pseudocapacitive) charge storage, as evidenced by distinct reduction and oxidation peaks observed in NaOH electrolyte. The plausible electrochemical reaction kinetics are outlined as follows:
| MoTe2 + OH− ↔ MoTe2OH + e− | (12) |
But the composite containing MoTe2 and MWCNT shows a nearly rectangular CV curve with well-distinguished oxidation and reduction peaks that demonstrate the synergistic effect of both MWCNT and MoTe2. Thus, the composite was able to deliver capacitance of nearly 502 F g−1 as shown in Fig. 10(m). The composite also demonstrates excellent cyclic stability (81% after 5000 cycles) that can be clearly visible from the Fig. 10(n) and (o).108 These investigations clearly demonstrate that integrating nanomaterials such as rGO and CNT, known for their exceptional mechanical properties in terms of stress and strain resistance, enhances the suitability of heterostructures for applications as flexible electrodes with enhanced stability.116
Similar to heterostructure formation Janus structure formation also helps to enhance the performance of supercapacitors. Thus Vikraman et al. utilized a one-pot reaction for constructing a Janus structure composed SeMoTe. Faradaic processes occurring at the edges where Se is replaced with Te alter the valence states of Mo atoms, contributing to the attainment of a substantial capacitance. The possible intercalation reaction is as follows (eqn (13) and (14))
| SeMoTe + xK+ + xe− ↔ KxSeMoTe (Faradaic reaction) | (13) |
| SeMoTe + xK+ + xe− ↔ KTe(Se)–MoSe(Te) (Non-faradaic reaction) | (14) |
This Janus structure of SMoTe was able to deliver capacitance of nearly 1057 F g−1 at 1 A g−1. This enhancement in capacitance can be attributed to the mutual EDLC-pseudo behavior and complete association of Te and Se active-edge sharing. The symmetric device constructed utilizing the same shows energy density around 41.3 W h kg−1 at power density of 4 kW kg−1.117
The integration of nanohybrid conducting polymers (NHCPs) with diverse nanomaterials offers a versatile platform for developing advanced materials utilized across a spectrum of applications, including batteries, supercapacitors, electronics, and sensors. Polyaniline (PANI) is notable for its exceptional theoretical capacitance, derived from its multiple redox states. Its unique electrical properties, make it a promising candidate for various applications. Additionally, PANI exhibits active pseudocapacitive behavior, driven by rapid and reversible faradaic reactions, further enhancing its potential for energy storage. However, PANI faces limitations in its application for energy storage. One significant constraint is its cycle life, as PANI-based devices may experience degradation over repeated charge–discharge cycles, impacting long-term performance. Additionally, restricted electrolyte access within PANI structures can impede ion diffusion and hinder overall energy storage efficiency. Overcoming these challenges is crucial for unlocking the full potential of PANI in energy storage applications. Moghaddam et al. systematically studied and compared the performance of exfoliated group 6 transition metal dichalcogenide materials such as MX2; M = Mo, W; X= S, Se, Te and find out that MoTe2 shows better performance among all the other chalcogenides. This material was further hybridized with PANI and studied their performances in 1 M H2SO4 aqueous electrolyte. The 2D structure of MoTe2 act as a substrate for the growth of PANI nanofibers and this creates a larger electrochemically active region for ions to involve in charge/discharge reactions. The order of capacitance of this hybrid consisting of PANI with several TMDs is as follows: MoTe2/PANI (499 F g−1) > MoS2/PANI (474 F g−1) > MoSe2/PANI (465 F g−1) > WS2/PANI (456 F g−1) > WSe2/PANI (450 F g−1) > WTe2/PANI (431 F g−1) at 0.4 A g−1.112 The summary of energy storage performance of molybdenum-based TMTes supercapacitor electrodes is given in Table 3.
Table 3 The summary of energy storage performance of molybdenum-based TMTes supercapacitor electrodes
Electrodes |
Electrolytes |
Capacitance (F g−1) |
Energy density (W h kg−1) and power density (kW kg−1) |
% Of capacity retention |
Ref. |
1T′ MoTe2 |
2 M KOH |
1393 |
ED = 56.4 |
— |
111
|
1T′ MoTe2//AC |
158.7 |
PD = 800 |
MoTe2/graphene electrode |
1 M Na2SO4 |
434 |
ED = 43.2 |
125% after 5000 cycles |
118
|
PD = 3000 |
1T′ MoTe2/rGO |
2 M KOH |
1791 |
— |
98.6% retention after 2000 cycles |
119
|
MoTe2/rGO |
2 M KOH |
1196 |
ED = 83.06 |
94.23% after 5000 cycles |
114
|
PD = 353.5 |
1T′-MoTe2 NPs/rGO |
6 M KOH |
563 |
ED = 35.2 |
— |
115
|
1T′-MoTe2 NPs/rGO//AC |
98.8 |
PD = 0.8 |
SS/MWCNTs/MoTe2 |
1 M NaOH |
502 |
— |
— |
120
|
SeMoTe |
6 M KOH |
367 |
ED = 41.3 |
97% after 5000 cycles |
117
|
PD = 13.6 |
Bulk MoTe2 |
1 M KOH |
271 |
— |
92.7% after 1000 cycles |
112
|
Exfoliated MoTe2 in NMP |
859 |
Exfoliated MoTe2 using tBuLi solvent |
1 M H2SO4 |
45 |
— |
— |
121
|
PANI-MoTe2 |
499 |
— |
54% after 200 cycles |
5.3 Tungsten-based tellurides: structural insights and capacitive behavior
Yu et al. utilized the liquid exfoliation method to synthesize WTe2 as given in Fig. 11(a). Fig. 11(b) illustrates the configuration of 1Td WTe2, consisting of deformed WTe6 octahedra with interconnected Te–Te edges, bounded together by weak van der Waals forces, thus forming a 2D 1Td structure. A solid-state flexible supercapacitor was constructed using this 1Td WTe2 which was able to show capacitance of nearly 221 F g−1, as indicated in Fig. 11(c) and (d). Impressively, the device maintained 95% efficiency even after undergoing 5500 cycles, demonstrating its excellent cycling stability.122 These results emphasize the potential of TMTes, including WTe2, in advancing the performance and durability of supercapacitors. Other TMTes such as the first-row TMTes were also reported to have good supercapacitor performance and cycling stability.
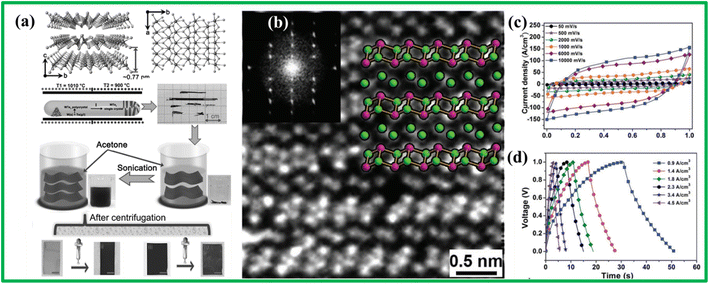 |
| Fig. 11 (a) Schematic diagram showing synthesis of WTe2 and crystal structure (b) STEM image of WTe2 and corresponding FFT pattern (c and d) CV and GCD curves of WTe2, reprinted from ref. 122 with permission from [John Wiley and Sons], copyright [2024]. | |
5.4 Ni-based tellurides: material design and capacitive behavior
NiTe is an intriguing material due to the influence of its different valence states on achieving high capacitance. Manikandan et al., were successfully synthesized NiTe nanorods using a simple hydrothermal method. These nanorods exhibited a high specific capacitance of 618 F g−1 at a current density of 1 A g−1 in a 3.5 M KOH electrolyte.123 Zhang et al. also fabricated hollow NiTe2 nanotubes with 566 F g−1 and 90% of the capacitance was preserved after 6000 cycles. The unique hollow structures with abundant defects of the material provided large surface area along with faster transfer channels and pathways contributing to better electrochemical performance.124 Recently, Jayababu et al.125 reported the fabrication of Ag-decorated NiFe mixed metal telluride hierarchical nanorods Ni0.75Fe0.25Te (AMMT HNRs) by a binder-free oven based wet chemical method. This Ag decoartion led to an increase in both electroactive sites and the overall surface conductivity of the material which can be clearly visible in the CV curve of Fig. 12(a) and GCD curve of Fig. 12(b). The specific capacity for this electrode was around 699.58 mA h g−1 at 3 mA cm−2 (Fig. 12(c)) with battery-type faradaic behavior, as shown in Fig. 12(d). A hybrid asymmetric supercapacitor device was fabricated by employing AMMT HNRs/NF as the positive electrode and activated carbon-coated Ni foam (AC/NF) as the negative electrode, as given in Fig. 12(e). This device exhibited a high areal capacitance of 1176.5 mF cm−2 and high areal energy density of 0.669 mW h cm−2. It also delivered a high-power density of 64 mW cm−2 at 50 mA cm−2 with 86% capacitance retention even after 5000 cycles. It was found out that the proportion of Ni and Fe played a crucial role in the electrochemical performance, as an increase in Ni content improved the performance of MMT HNRs/NF. Furthermore, the incorporation of highly conductive Ag nanoparticles onto the MMT HNRs/NF promoted electron transport to the current collector by creating numerous conductive pathways across the electrodes. The fabricated hybrid supercapacitors (HSC) device was also utilized successfully to power LEDs and digital multi-sensors as given in Fig. 12(f) and (g), which suggested that mixed metal telluride decorated with Ag has potential for practical use in high-energy storage devices.
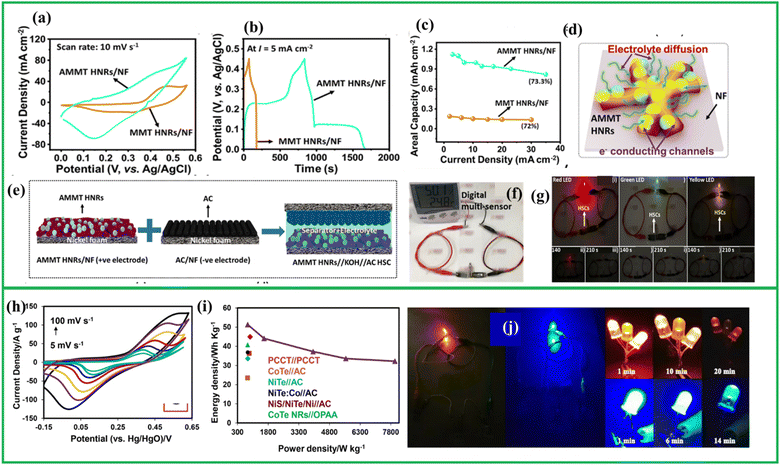 |
| Fig. 12 (a) CV and (b) GCD curves of AMMT HNRs/NF electrodes (c) areal capacities with current density of MMT HNRs/NF and AMMT HNRs/NF electrodes (d) schematic representation of the working mechanism of AMMT HNRs/NF electrode (e) schematic representation of the ASC device based on AMMT HNRs/NF//AC/NF (f and g) real-time applicability of AMMT HNRs/NF//AC/NF device, reproduced from ref. 126 with permission from [American chemical society], copyright [2024], (h) CV curves of NiTe2–Co2Te2@rGO(I) Ragone plot of NiTe2–Co2Te2@rGO//AC device (j) real-time application of NiTe2–Co2Te2@rGO//AC device after connecting in parallel and in series, reproduced with permission from ref. 127. | |
In a recent study by Khalafallah et al., bifunctional core–shell heterostructures of nickel cobalt selenide@nickel telluride (NixCo12−xSe@NiTe) were successfully synthesized using a two-step electrodeposition method. The prepared NixCo12−xSe@NiTe structures have cross-linked NixCo12−xSe nanosheets that are vertically anchored on epitaxially grown NiTe dendritic arrays, forming a hierarchical core–shell architecture. They form a 3D array of dendrites, which provided a favorable environment for the nucleation of 2D porous nanosheets. This led to the formation of additional defects and heterointerfaces within the structure, thereby expanding the contact area between the active material and the electrolyte. The presence of these defects and heterointerfaces is beneficial as they create more electroactive sites and facilitate easier pathways for electron and ion transport. Consequently, this results in an enhancement of the overall electrical conductivity of the material. A hybrid asymmetric supercapacitor device was fabricated NixCo12−xSe@NiTe//AC. The fabricated device delivered a specific energy density/power density of around 67.7 W h kg−1/724.8 W kg−1 with 92.4% capacity retention after 12
000 cycles. The heterointerface engineering of the NixCo12−xSe@NiTe core–shell heterostructure provided a favorable electrochemical activity and stability for its application in fabrication of high-performance energy storage devices.78
Similarly, Farshadnia et al. designed a bimetallic telluride over rGO such as NiTe2–Co2Te2@rGO using a simple hydrothermal approach where CoNi2@rGO is subjected to tellurization. This tellurization increases active sites, hence providing contact sites with the electrolyte during faradaic processes. As a result, the composite could offer a capacitance of almost 223.6 mA h g−1 at 1 A g−1. The CV curve shown in Fig. 12(h) exhibits two distinct anodic peaks which could be attributed to the Co2+/Co3+ and Ni2+/Ni3+ oxidation. Thus, the reversible reaction in this case can be as follows (eqn (15) and (16))
| Ni/CoTe + OH− → Ni/CoTeOH + e− | (15) |
| Ni/CoTeOH + OH− → Ni/CoTe + H2O + e− | (16) |
They also constructed an asymmetric supercapacitor utilizing AC as the negative electrode. This device was able to extend the potential window up to 1.6 V and able to deliver capacitance of nearly 64 mA h g−1 with energy density and power density around 51 W h kg−1 and 800 W kg−1 as given in Fig. 12(i) and able to light yellow and red light by connecting the device in series as shown in Fig. 12(j).
Molaei et al. synthesized a hierarchical structure composed of NiTe2/CoTe2. The NiCo-layered double hydroxide (NiCo-LDH) nanoarrays were synthesized on Ni foam through a hydrothermal method, followed by tellurization, leading to the formation of NiTe2/CoTe2 nanostructures. This removes the requirement for inert, slow-conducting binders, streamlining the redox chemistry process. The NF/FNCT utilizes conductive tellurium and a hierarchical flower-like nanomorphology to achieve improved efficiency, swifter electron/ion transport and superior electrochemical performance. The NF/FNCT electrode shows high capacity of 1388.9 C g−1 with good capacitance retention of around 83.45% and a cycle durability of 96.67%. The asymmetric supercapacitor fabricated utilizing AC shows energy density around 58.85 W h kg−1 at a power density of around 806.85 W kg−1.128
The studies conducted by Meghanathan et al. explore the application of metal–organic framework-derived Nickel Telluride porous structured composites as electrode materials for asymmetric supercapacitors. Their approach involved employing a hydrothermal method to incorporate ZIF-67 into a core/shell architecture of NiTe, resulting in a 3D hierarchical design.129 Zaw et al. constructed an advanced-battery type electrode utilizing montmorillonite as a template for the growth of NiTe with considerable porosity.130
Several notable studies have demonstrated the effectiveness of alloying transition metals or doping in TMTes to enhance their overall conductivity and improve their electrochemical activity. These strategies have been widely explored and have shown promising results. Deshagani et al. reported the fabrication of Se doped NiTe flakes by a simple hydrothermal method which showed remarkable performance as compared to the pristine NiTe. The specific capacitance of the NiTe:Se was found to be 943 F g−1 which was 1.5 times higher than the pristine NiTe. The performance of the AC//NiTe:Se supercapacitor, with a capacity of 300 C g−1, Emax of 66 W h kg−1, and Pmax of 400 W kg−1, also underwent a significant enhancement with the incorporation of electrically and ionically conducting PMP layers on the surfaces of both AC and NiTe:Se. This resulted in a capacity of 404 C g−1, along with Emax and Pmax values of 90 W h kg−1 and 400 W kg−1, respectively, marking a notable improvement compared to AC//NiTe:Se, AC//NiTe and AC//AC cells. This was attributed to the substantial enhancement in the surface roughness, electrical conductivity, and free charge-carrier concentration, after the introduction of Se into NiTe crystal structure resulting in fast ion transport and electron movement.91 Ye et al.131 also synthesized Co ions doped NiTe by hydrothermal method with different doping ratios of Co to Ni. FESEM images showed the undoped NiTe to be flaky nanosheet structure and upon doping with Co the structure was transformed to particulate nanostructures, which increased with increase in the loading mass of the Co and have a coralloid structure on the particle's surface. The summary of energy storage performance of nickel-based TMTes based supercapacitor electrodes is shown in Table 4.
Table 4 The summary of energy storage performance of Ni-based TMTes supercapacitor electrodes
Electrodes |
Electrolytes |
Capacitance (F g−1) |
Energy density (W h kg−1) and power density (kW kg−1) |
% Of capacity retention |
Ref. |
NiTe nanorod |
3.5 M KOH |
618 |
— |
75% after 5000 cycles |
123
|
NiTe2 nanorod |
0.5 M H2SO4 |
566 |
ED = 101.8 |
90% after 6000 cycles |
124
|
PD = 2.02 |
Ni0.75Fe0.25Te (AMMT HNRs) |
1 M KOH |
Areal capacitance- 1.1 mA h cm−2 |
Areal energy density of 0.669 mWh cm−2 and a power density of 64 mW cm−2 |
86 |
125
|
AMMT HNRs/NF (positive electrode) and EDLC-type AC/NF |
1176.5 mF cm−2 |
% After 5000 cycles |
Ni6Co6Se@NiTe |
2 M KOH |
223 mA h g−1 |
ED = 67.7 |
92.4% after 12 000 cycles |
78
|
Ni6Co6Se@NiTe//AC |
72.6 mA h g−1 |
PD = 724.8 |
NiTe2–Co2Te2@rGO |
2 M KOH |
223.6 mA h g−1 |
ED = 51 |
89.3% stability after 3000 cycles |
127
|
NiTe2–Co2Te2@rGO//AC |
64 mA h g−1 |
PD = 800 |
NiTe2/CoTe2 from NiCo-LDH) nanoarrays |
6 M KOH |
1388.9 C g−1 |
ED = 58.85 |
83.45% after 5000 cycles |
128
|
NiTe2/CoTe2//AC |
264.8 |
PD = 806.85 |
Metal–organic framework-derived nickel telluride porous structures |
3 M KOH |
1521 |
ED = 45.3 |
93.5% after 5000 cycles |
129
|
NiTe//AC |
— |
PD = 5689.1 |
NiTe grown over montmorillonite//AC |
1 M KOH |
— |
ED = 15.32 |
93.4% after 1000 cycles |
130
|
PD = 320 |
5.5 Co-based tellurides: synthetic and performance enhancement strategies
Bhat et.al. reported the synthesis of CoTe2 by an anion exchange method where CoTe2 was obtained under hydrothermal conditions from pre-synthesized Co(OH)2. The CoTe2 electrode delivered a specific capacitance of 360 F g−1 at a scan rate of 5 mV s−1 and also retained 52% of capacitance at 2500 cycles.132 In another study by Manikandan et al. CoTe nanorods were fabricated as the positive electrode, while orange-peel derived activated carbon (OPPA) served as the negative electrode in a supercapacitor. The CoTe nanorods demonstrated a high specific capacity of 170 C g−1, indicating their ability to store a significant amount of charge. Additionally, the supercapacitor exhibited excellent life cycle performance, with a retention rate of 99% over multiple cycles. In a study conducted by Mao et al., CoTe2 was synthesized using a simple solvothermal route, resulting in the formation of an amorphous nanoflower structure. The fabricated CoTe2 nanoflower electrodes exhibited a high specific capacitance of up to 460 F g−1 at a current density of 1.5 A g−1. Remarkably, even after 5000 cycles, the electrode maintained a cycling stability of 91%, indicating its suitability for long-term use in supercapacitor applications.133 The amorphous structure of the synthesized CoTe2 material was found to contribute to an increased number of active sites and improved ion diffusion, leading to enhanced electrochemical performance. Kshetri and group134 fabricated a supercapacitor electrodes composed of porous cobalt telluride-carbon structures derived from metal–organic frameworks (MOFs) on Ni foam. An intriguing characteristic of this material is its ability to function as a working electrode in both positive and negative potential windows. Electrochemical characterization studies have revealed that the material exhibits a combination of EDLC and pseudocapacitive behavior contributed by the O and N functional group, demonstrating the mixed-charge storage nature of the hybrid. The charge storage mechanism can be deconvoluted into three regions at region of low potential (ranging from −0.8 to −0.6 V) where a rapid migration of hydrated K+ ions happens due to the outer Helmholtz layer formation. Between the potential range of −0.6 to −0.2 V, hydrated K+ ions disperse due to elevated interfacial tension, leading to significant diffusion throughout the bulk electrolyte solution. In the higher potential range from 0.2 to 0.0 V, hydrated K+ ions are constrained within electrode structure holes through a quasi-reversible process involving insertion and extraction. The overall process can be summarized as eqn (17). | S-CxO + [(K(H2O)n]+ + e− ↔ S − CxOK(H2O)n−y | (17) |
Here, the CV curves in positive potential region (0–0.5) much wider area than negative potential region demonstrate the faradaic reactions happening due to the redox reactions as follows (eqn (18) and (19)) | CoTe + OH− ⇌ CoTeOH + e− | (18) |
| CoTeOH + OH− ⇌ CoTeO + H2O + e− | (19) |
Presence of 3D conducting highly porous structures of N-doped carbon synergistically complimenting the pseudocapacitive CoTe nanostructures (CoTe@C–NiF) significantly improved the electrochemical performance. The fabricated CoTe@C–NiF symmetric device also delivered an energy density of 43.84 W h kg−1 at a power density of 738.88 W kg−1 in 2 M KOH electrolyte. Similarly, Shi et al. derived a bimetal telluride i.e., CoTe2–NiTe2 from a Co-MOF over carbon fibers using Ni ion etching process and tellurization. This hierarchical structure preserves the layered structure of NiCoLDH while additionally utilizing cooperative oxidation energy storage between CoTe2 and NiTe2 nanoparticles. Thus, these nanosheets arrays have a stable array structure and contain a large number of interconnected nanoparticles. When compared to pure CoTe2 nanoparticles based on Co-MOF the CoTe2–NiTe2 nanoparticles are smaller in size. This allows for more active sites with different oxidation states and a larger contact surface with the electrolyte. The CV curves here shown Fig. 13(a) exhibits two-distinct redox peaks which could be due to the vast redox reaction of CF@CoTe2–NiTe2 during the electrochemical process (eqn (20) and (21)).
| Ni/CoTe + OH− ↔ Ni/CoTeOH + e− | (20) |
| Ni/CoTeOH + OH− ↔ Ni/CoTeO + H2O + e− | (21) |
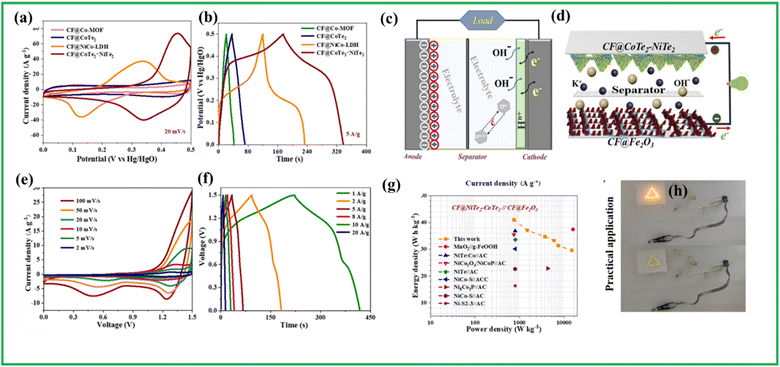 |
| Fig. 13 (a) Comparison CV curves of the CF@Co-MOF, CF@CoTe2, CF@NiCo-LDH, and CF@CoTe2–NiTe2 electrodes, (b) comparison GCD curves of the CF@Co-MOF, CF@CoTe2, CF@NiCo-LDH, and CF@CoTe2–NiTe2 electrodes (c) storage mechanism of CF@CoTe2–NiTe2 based supercapacitor (d) schematic illustration of charge storage mechanism in CF@CoTe2–NiTe2//CF@Fe2O3 ASCs (e) CV curves of CF@CoTe2–NiTe2//CF@Fe2O3 ASCs (f) GCD curves of CF@CoTe2–NiTe2//CF@Fe2O3 ASCs (g) Ragone plot of CF@CoTe2–NiTe2//CF@Fe2O3 ASCs (h) real-time application of CF@CoTe2–NiTe2//CF@Fe2O3 ASCs reproduced from ref. 135 with permission from [American chemical society], copyright [2024]. | |
The specific capacity calculated from GCD given in Fig. 13(b) was around 261 F g−1. They also constructed an asymmetric supercapacitor utilizing CF@Fe2O3 as negative electrode as shown in Fig. 13(c) and (d). The device was able to extend the potential window of around 1.5 V as shown in Fig. 13(e) with capacitance around 131 F g−1 at 1 A g−1 (Fig. 13(f)). The device exhibited (Fig. 13(g)) maximal energy density of 41 W h kg−1 at 750 W kg−1 with good output characteristics as shown in Fig. 13(h).135
This article delves into the use of mixed metal telluride NiLaTe microfibers (MFs) on Ni foam (NF) for the construction of a flexible solid-state hybrid supercapacitor (FSSHSC). Three-electrode tests reveal that NiLaTe microfibers offer double the electrochemical performance compared to standard aqueous KOH electrolytes. The [Fe(CN)6]3−/[Fe(CN)6]4− redox pair boosts ion transport in the active electrode, enhancing performance. The fabricated asymmetric solid-state devices using PVA-KOH-KFC gel electrolyte and NiLaTe//AC shows areal capacity of 65.6 μA h cm−2 (60.0 mA h g−1) at 2 mA cm−2, with a power density of 5488.7 W kg−1 (6312.0 μW cm−2) and a max energy density of 45.5 W h kg−1 (52.36 μW h cm−2).94
A CoTe@rGO was synthesized, demonstrating significant improvements in comparison to pristine CoTe. The constructed asymmetric device utilized CoTe@rGO as the positive electrode and AC as the negative electrode in a 3 M KOH electrolyte. Remarkably, this device exhibited a high-power density of 4004.93 W kg−1 at an energy density of 33.43 W h kg−1. Furthermore, it demonstrated exceptional durability, with 87.1% capacitance retention even after undergoing 5000 cycles.136 In another work, reported by Bhol et al. tellurium nanotubes were used as the sacrificial template, wherein cobalt and iron were decorated over it via a wet chemical method. Here, the incorporation of Co2+ and Fe3+ results in a rougher surface. This alteration led to an increase in the surface area and the presence of more active sites on the nanotubes. This resulted in an increase in the number of electroactive spots, moreover. The intact Te NTs could be seen serving as the backbone for Co and Fe nanoparticles to grow on it creating a synergistic effect between the Co, Fe and Te. The asymmetric device Co–Fe decorated Te NTs//AC was able to specific capacitance of 179.2 F g−1 and also delivered an energy density 62.1 W h kg−1 at a power density of 1138.2 W kg−1. It also retained 73% of its initial capacitance after being subjected 4000 cycles. The tubular conductive template of the Te NTs and Co–Fe decoration over the surface seemed to contribute to its increased electrochemical performance.137 The summary of energy storage performance of cobalt-based TMTes supercapacitor electrodes in Table 5.
Table 5 Summary of energy storage performance of cobalt-based TMTes supercapacitor electrodes
Electrodes |
Electrolytes |
Capacitance (F g−1) |
Energy density (W h kg−1) and power density (kW kg−1) |
% Of capacity retention |
Ref. |
CoTe2 |
1 M KOH |
360 |
— |
52% after 2500 cycles |
138
|
CoTe nanorods |
3.5 M KOH |
170 C g−1 |
40.7 |
85% after 10 000 cycles |
139
|
CoTe nanorods//AC |
183 C g−1 |
800 |
CoTe2 nanoflowers |
1 M KOH |
460 |
|
91% after 5000 cycles |
95
|
CoTe@C–NiF |
2 M KOH |
Areal capacitance-307.5 mF cm− 2 |
ED = 43.84 |
83.3% after 10 000 cycles |
134
|
PD = 738.88 |
CoTe2–NiTe2 |
6 M KOH |
223.2 |
ED = 41 |
87.6% after 6000 cycles |
135
|
CF@ CoTe2–NiTe2//CF@Fe2O3 |
131.1 |
PD = 750 |
CoTe@rGO |
3 M KOH |
810.6 |
ED = 40.04 |
87.1% after 5000 cycles |
136
|
CoTe@rGO//AC |
112.6 |
PD = 799.91 |
Cobalt–iron decorated tellurium nanotubes |
4 M KOH |
179.2 |
ED = 62.1 |
73% after 4000 cycles |
140
|
PD = 1138.2 |
5.6 Other metal tellurides: performance and potential
Chakravarty and a group of researchers reported one of the earliest works for TMTes in supercapacitor applications.141 TaTe2 was synthesized by a simple and rapid microwave-assisted synthesis method. The observed coulombic efficiency of the fabricated electrode was more than 95% and the maximum charge–discharge capacity was less than 2.4 mV s−1. This indicated a preferable characteristic for attaining supercapacitor behavior. The use of samarium, a rare earth ion with multiple oxidation states (Sm2+ and Sm3+), holds promise as a potential candidate for supercapacitor applications. Khumbar and colleagues reported the successful synthesis of Sm2Te3 thin films using a one-step chemical bath deposition (CBD) method.142 The prepared Sm2Te3 electrode exhibited a specific capacitance of 207 F g−1 and after 1000 cycles the electrochemical stability was maintained up to 78%. The group also reported the synthesis of Sm2Te3 thin films by employing a different route, i.e., by SILAR method. The structural and morphological characterization displayed a cloud-like morphology with an orthorhombic crystal structure. The maximum specific capacitance was observed to be 144 F g−1 and the energy density to be 10 W h kg−1 with an electrochemical cycling stability of 69.3%.143 CdTe, a II-Vi semiconductor was prepared using a simple hydrothermal reported by Manikandan and group showing a nanorod morphology with a length and diameter of 200 and 46 nm respectively. At 2 mA cm−2 it exhibited specific capacitance of 438 F g−1 and 95% of the capacitance was retained at a current density of 30 mA cm−2 even after 5000 cycles. Further evaluation of the material was done by fabricating a CdTe symmetric supercapacitor which achieved specific capacitance of 103 F g−1 at 2.5 mA cm−2 current density and high energy and power density of 20.54 W h kg−1 and 746 W kg−1 respectively.144 Abdullah et al. examined the performance of Ag2Te with varied Ag concentrations. Bomineedi et al. also utilized MWCNTs by incorporating it with mixed phase iron telluride (FeTe:Fe2TeO5). The functionalized MWCNTs solution was applied to a conducting stainless steel (SS) substrate by a simple “dip and dry” coating method, subsequently FeTe:Fe2TeO5 nanodots was then grown onto the MWCNTs by SILAR. The deposition of FeTe: Fe2TeO5 nanodots onto the MWCNTs could also be observed, creating a nanodot-nanotube system, which is especially helpful in facilitating the transfer of electrons while maintaining a higher ionic conductivity. The electrochemical analysis for supercapacitor application of the prepared MWCNT/FeTe:Fe2TeO5 nanocomposite was performed in 0.5 M NaCl electrolyte in a three-electrode setup, which exhibited a specific capacitance of 773.69 F g−1 at 3 mV s−1 and capacitive stability of 95% after 5000 cycles.145 Farshah et al. developed an effective strategy to create a hollow FeNiCoTe nanocubes from Prussian blue analog (PBAs) a sub-class of metal organic frameworks. FeNiCoPBA which was synthesized using a co-precipitation method was subjected to tellurization to form FeNiCoTe nanocubes. The FeNiCoTe-based electrode performs exceptionally well due to the strong electroactivity of the mixed metal FeNiCo and due to the superior conductivity of tellurium and also because of the hollow shape of the FeNiCoTe nanocubes. Thus this FeNiCoTe nanocubes-based electrode was able to deliver a high capacity (1402 C g−1 at 1 A g−1).146 The summary of other TMTes performance is given in Table 6.
Table 6 Summary of energy storage performance of other TMTes supercapacitor electrodes
Electrodes |
Electrolytes |
Capacitance (F g−1) |
Energy density (W h kg−1) and power density (kW kg−1) |
% Of capacity retention |
Ref. |
TaTe2 |
0.5 M KOH |
4.09 |
— |
— |
141
|
Sm2Te3 |
LiClO4 |
207 |
ED = 25.60 |
78% after 1000 cycles |
147
|
Propylene carbonate |
PD = 14.18 |
CdTe |
2 M KOH |
103 |
ED = 20.54 |
95% after 5000 cycles |
144
|
PD = 87.6 |
FeTe: Fe2TeO5/MWCNT |
0.5 M NaCl |
773.69 |
ED = 8.19 |
95% after 5000 cycles |
145
|
PD = 108 |
FeNiCoPBA |
6 M KOH |
1402 C g−1 |
ED = 62.9 |
89.6% after 75 000 cycles |
146
|
FeNiCoPBA//AC |
283.2 C g−1 |
PD = 806.4 |
5.7 Wearable and flexible supercapacitors
Karade et al. synthesized an SS/MWCNT/MoTe2 nanostructure composite via a two-step chemical synthetic route followed by SILAR. The fabricated composite electrode showed significantly improved specific capacitance of 502 F g−1 at 2 mV s−1 scan rate as compared to the pure MoTe2 with capacitive retention of 81% at 5000 cycles as given in Fig. 14(a) and (b). A flexible solid-state symmetric supercapacitor device was fabricated utilizing a PVA-LiClO4 gel electrolyte. This device exhibited improved specific capacitance, energy density (18.51 W h kg−1) as given in Fig. 14(c), and electrochemical stability compared to conventional counterparts. Modern technology necessitates lightweight and adaptable energy storage systems. To assess the impact of bending on device performance, GCD analysis was performed using a 45° bending step and a constant current density of 0.5 mA cm−2 (Fig. 14(d) inset). The GCD shape remained unchanged even at 175°, indicating high mechanical stability and material adhesion. Fig. 14(e) displays a retention curve based on bending angle, indicating a consistent increase in retention because of the large mechanical strength of the substrate and MWCNTs, as well as the nanostructured MWCNTs/MoTe2 hybrid thin film.108 The device constructed using the hybrid was charged with a 3 V dc source for 30 seconds (Fig. 14(f)), then discharged through the red LED. The LED shines for up to 60 seconds, indicating that the symmetric FCSS-SC gadget can store a substantial amount of charge and power. Yu et al. demonstrated that 1Td WTe2 nanosheets developed utilizing CVD approach can be used for the fabrication of flexible devices as given in Fig. 14(g).
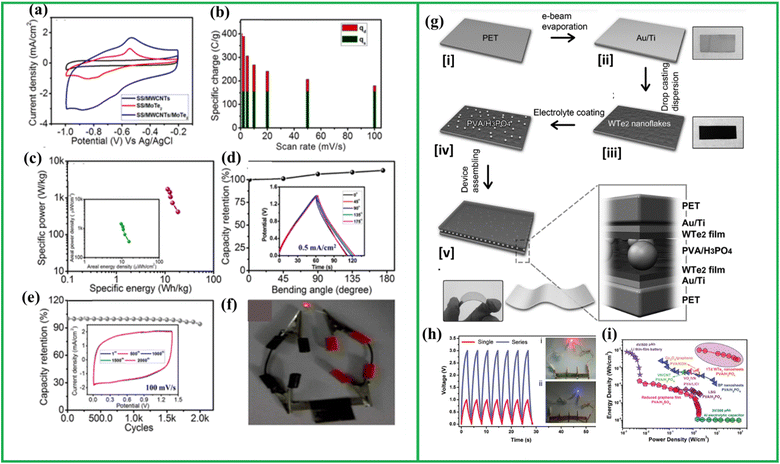 |
| Fig. 14 (a) Comparative CV curves of SS/MWCNTs, SS/MoTe2 and SS/MWCNTs/MoTe2 based electrodes (b) Demonstration of the contributions from the surface capacitive (qs) and diffusion controlled (qd) charge to the total charge stored, at different scan rates (c) Ragone plot of flexible device based on SS/MWCNTs/MoTe2 (d) plot of capacity retention with bending angle (e) no: of cycles of flexible device based on SS/MWCNTs/MoTe2 (f) practical application of SS/MWCNTs/MoTe2 flexible devices, reproduced from ref. 120 with permission from [American chemical society], copyright [2024], (g) schematic illustration of flexible supercapacitor based on 1Td WTe2, (h) practical application of flexible device based on 1Td WTe2 by connecting in series, (i) Ragone plot of flexible device based on 1Td WTe2, reprinted from ref. 122 with permission from [John Wiley and Sons], copyright [2024]. | |
Prangya et al. also constructed a flexible supercapacitor using NiLaTe as the positive, activated carbon (AC) as the negative electrode, and PVA-KOH-KFC gel. The constructed device was able to deliver maximum areal capacity of around 65.6 μA h cm−2 with energy density and power density of around 52.36 μW h cm−2/6312.0 μW cm−2. The device shows excellent stability of around 80% after 10
000 cycles.109 Similarly, Cui et al. developed a core–shell structure composed of CoTe nanowires and NixSy nanosheets using electrochemical depositions. CoTe@ NixSy core–shell nanowires based flexible hybrid supercapacitors and AC exhibits remarkable volume energy density of 3.95 mW h cm−3 and areal energy density of 0.77 mW h cm−2. These results show outstanding rate capacity, improved cycle stability, and strong commercial viability of the metal tellurides in the development of flexible and wearable supercapacitors.148
The summary of performance of flexible/wearable supercapacitors based on TMTes is given in Table 7.
Table 7 Performance of flexible/wearable supercapacitors based on TMTes
Electrodes |
Electrolytes |
Capacitance (F g−1) |
Energy density (W h kg−1) and power density (kW kg−1) |
% Of capacity retention |
Ref. |
SS/MWCNTSs/MoTe2 |
PVA-LiClO4 |
|
ED = 18.51 |
— |
108
|
NiLaTe |
PVA-KOH-KFC |
65.6 μA h cm−2 |
ED = 52.36 μW h cm−2 |
80% after 10 000 cycles |
109
|
PD = 6312.0 μW cm−2 |
CoTe nanowires and NixSy nanosheets |
PVA/KOH |
1.926 mA cm−2 |
ED = 3.95 mW h cm−3 |
82.1% after 5000 cycles |
148
|
PD = 22.64 mW cm−3 |
6. Future perspectives and conclusion
The emergence of new age electronic devices and energy harvesters has created a desire for more efficient electrochemical supercapacitors. TMTes are proposed to have an important role as active electrode materials in supercapacitors due to their enormous surface area, nontoxicity and mechanical stability. These features allow them to store considerable amounts of energy via electrical double layers and pseudocapacitive charge storage mechanisms. However, its low conductivity remains a significant obstacle, leading to poor capacitive performance and limiting its potential applications in the realm of supercapacitors. Additionally, several other limitations have been identified, such as degradation and volume expansion occurring during extended charging/discharging cycles.149 These constraints hinder their cost-effective utilization for widespread industrial manufacturing. This review addresses the advantages of TMTes over other chalcogenides in the field of supercapacitors and the strategies used to improve the performance of TMT's based supercapacitors from the material perspectives and optimization of devices.
From the materials perspective:
(1) Designing the morphology and nano/microstructure helps in facilitating mass transportation and accommodating large volume expansion during cycling.
(2) The majority of the research concentrated on designing heterostructures, doping and phase engineering by combining various tactics to improve performance by producing synergistic effects. However, more research has to be done to fully understand how each particular element contributes. In order to achieve that generating various controlled design strategies for heterostructures to investigate the nature of the competing effects. It may be possible to move forward objectively and beyond the available options by investigating alternative strategies with the aid of theoretical viewpoints.
(3) The interlayer spacing in the case of layered 2D TMTes creates the ideal environment for the storage of a range of guest species in energy storage devices. The interlayer charge carrier hopping, which is essential for the material's electrical conductivity and electrochemical characteristics, has an inverse relationship with the interlayer spacing. Further investigations in regard to both theoretical and experimental points is required to enquire the dominant contribution out of the two factors to its overall performance.
(4) To optimize the utilization of TMTes material properties, minimizing restacking is crucial, as it can detrimentally impact device performance in various applications. Restacking diminishes access to electrolytes and ions within TMTes, thereby compromising their performance to levels akin to bulk materials. Additionally, prevalent restacking hinders the exploitation of the diverse surface chemistries accessible by well-dispersed TMTes.
(5) TMTes are often synthesized through complex and expensive methods, limiting their large-scale production. Overcoming this challenge requires the development of scalable and cost-effective synthesis techniques for TMTes. This includes exploring solution-based methods, scalable deposition techniques and efficient precursor materials to enable the mass production of TMTes for supercapacitors. One of the important points to note is that tellurium is a relatively rare and expensive element, which can pose a challenge for large-scale production of TMTes.
From the device aspect: hybrid supercapacitors integrating battery electrodes can effectively balance the conflict between energy density and power density by storing charge at the anode and cathode through different mechanisms. The selection of an appropriate electrolyte is crucial as it can expand the working potential and consequently achieve higher energy density. The composite materials made of TMTes with carbon materials and other metal-based compounds have emerged as viable options for advanced asymmetric supercapacitor. By combining additional materials with complimentary inherent qualities, these composite structures not only improve energy storage capacity compared to single-material structure, but also display increased stability and flexibility. Presently, significant attention has been directed towards materials design for TMTes-based supercapacitors, with valuable insights drawn from experiences with other materials or in diverse application fields. However, the optimization of electrolytes remains an ongoing challenge. With a comprehensive understanding of charge storage mechanisms and the interaction between electrolytes and electrode materials, key parameters such as ionic conductivity, chemical stabilities, operating potential windows, and compatibility can be efficiently optimized. The breakthroughs in electrolyte development hold the potential to greatly accelerate the advancement and industrial adoption of SCs, and may also pave the way for advancements in other energy storage technologies. The comparative data of different TMTes, showcasing the best-performing materials from each category is given in Table 8.
Table 8 Best performing TMTes electrode materials for supercapacitors
Electrodes |
Electrolytes |
Capacitance (F g−1) |
Energy density (W h kg−1) and power density (kW kg−1) |
% Of capacity retention |
Ref. |
VTe2/MXene |
0.5 M K2SO4 |
250 |
ED = 46.3 |
87% after 7000 cycles |
107
|
VTe2/MXene//MoS2/MXene |
130 |
PD = 400 |
MoTe2/rGO |
2 M KOH |
1196 |
ED = 83.06 |
94.23% after 5000 cycles |
116
|
PD = 353.5 |
WTe2 film |
PVA/H3PO4 |
221 |
ED = 0.0029 W h cm−3 |
91% after 5500 cycles |
122
|
PD = 83.6 W cm−3 |
CoTe@rGO |
3 M KOH |
810.6 |
ED = 40.04 |
87.1% after 5000 cycles |
138
|
PD = 799.91 |
FeNiCoPBA |
6 M KOH |
1402 C g−1 |
ED = 62.9 |
89.6% after 75 000 cycles |
148
|
FeNiCoPBA//AC |
283.2 C g−1 |
PD = 806.4 |
Future research can explore on developing TMTes compositions that reduces the reliance on tellurium while maintaining the desired properties. This can help mitigate the cost implications associated with the use of tellurium-based TMTes in supercapacitors. Last but not least, as attention is still mostly focused on other chalcogenides-based materials like sulphur and selenides, not much research has been done on TMTes for supercapacitor applications. The creation of heterostructures using different materials would likely result in noticeable changes in their physicochemical properties and lead to the development of materials more suitable for these electrochemical systems. Exploring these less studied TMTes components may also offer new insights into basic mechanisms. Theoretical DFT computations would be of great help in providing prompt and thorough screening of material conjugations. Before creating effective electrochemical energy-storage and conversion systems, more work still has to be done given the aforementioned difficulties and the significant opportunity for improvement. Though the prospects for the effective development of these technologies are undoubtedly bright with advances in knowledge. TMTes are currently in an early stage of development to meet various practical demands. High performance, safety, nontoxicity, and flexibility are the primary considerations and significant challenges for unconventional SCs in emerging devices such as wearable equipment and bioimplant chips. So far, no reports are available on the fabrication of microsupercapacitors and wearable supercapacitors based on TMTes. Furthermore, leveraging the structural properties and characteristics of 2D TMDs, innovative development of other unconventional supercapacitors (SCs) is possible. This includes the creation of optically transparent SCs, fiber-based SCs, and self-healing SCs, expanding the scope of potential applications in energy storage technology.
Thus, TMTes-based electrode materials present numerous potential and challenges for researchers to produce high-performance SCs. Researchers can improve the charge storage performance of TMTes-based electrodes, resulting in efficient and scalable supercapacitors with higher energy and power densities, longer lifespan, and more environmentally friendly features. The Fig. 15 shows the overall summary of the TMTes future perspectives.
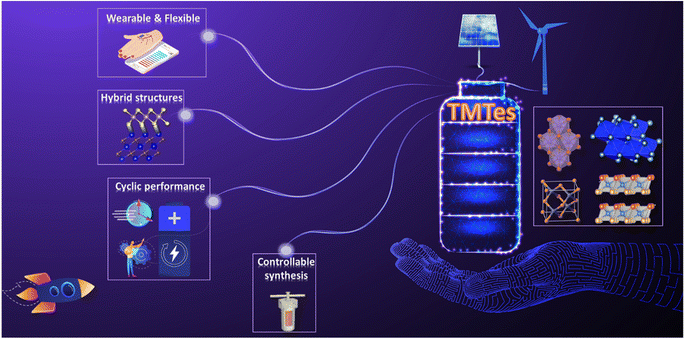 |
| Fig. 15 Summary and future perspectives of TMTes-based supercapacitors. | |
Data availability
No primary research results, software or code have been included and no new data were generated or analysed as part of this review.
Conflicts of interest
Authors declare no conflict of interest.
Acknowledgements
The authors extend their appreciation for the financial assistance provided by the SERB Core Research Grant (Grant No. CRG/2022/000897) and the Department of Science and Technology (DST/NM/NT/2019/205(G)). They also express gratitude for the support received through the Minor Research Project Grant from Jain University (JU/MRP/CNMS/29/2023). CSR acknowledges backing from the National Research Foundation of Korea under the Brain Pool program, funded by the Ministry of Science and ICT, South Korea (Grant No. RS-2023-00222186). The work is further supported by the National Research Foundation of Korea (NRF) and the Commercialization Promotion Agency for R&D Outcomes (COMPA), funded by the Ministry of Science and ICT (Grant No. RS-2023-00217581, RS-2023-00304768).
References
- S. Deshagani, P. Ghosal and M. Deepa, Electrochim. Acta, 2020, 345, 136200 CrossRef CAS.
- J. Chow, R. J. Kopp and P. R. Portney, Science, 2003, 302, 1528–1531 CrossRef PubMed.
- M. A. Bissett, S. D. Worrall, I. A. Kinloch and R. A. W. Dryfe, Electrochim. Acta, 2016, 201, 30–37 CrossRef CAS.
- J. Jiang, M. Gao, W. Sheng and Y. Yan, Angew. Chem., 2016, 128, 15466–15471 CrossRef.
- M. L. Agiorgousis, Y.-Y. Sun, D. West and S. Zhang, ACS Appl. Energy Mater., 2018, 1, 440–446 CrossRef CAS.
- K. M. Naik and S. Sampath, Electrochim. Acta, 2017, 252, 408–415 CrossRef CAS.
- O. Peña, Phys. C, 2015, 514, 95–112 CrossRef.
- X. Shi, L. Wang, L. Chen and X. Chen, Trans. Nonferrous Met. Soc. China, 2009, 19, 642–645 CrossRef CAS.
- L. Jing, E. B. Michael and F. J. Di Salvo, Inorg. Chem., 1992, 31(6), 1050–1054 CrossRef.
-
W. Y. Liang and M. S. Dresselhaus, Electronic Properties of Transition Metal Dichalcogenides and Their Intercalation Complexes, Springer US, Boston, MA, 1986, pp. 31–73 Search PubMed.
- M. Chhowalla, H. S. Shin, G. Eda, L.-J. Li, K. P. Loh and H. Zhang, Nat. Chem., 2013, 5, 263–275 CrossRef PubMed.
- J. Su, K. Liu, F. Wang, B. Jin, Y. Guo, G. Liu, H. Li and T. Zhai, Adv. Mater. Interfaces, 2019, 6, 1900741 CrossRef CAS.
- Y. Wang, J. Xiao, H. Zhu, Y. Li, Y. Alsaid, K. Y. Fong, Y. Zhou, S. Wang, W. Shi, Y. Wang, A. Zettl, E. J. Reed and X. Zhang, Nature, 2017, 550, 487–491 CrossRef CAS PubMed.
- C. Zhang, S. Kc, Y. Nie, C. Liang, W. G. Vandenberghe, R. C. Longo, Y. Zheng, F. Kong, S. Hong, R. M. Wallace and K. Cho, ACS Nano, 2016, 10, 7370–7375 CrossRef CAS PubMed.
- J. A. Wilson and A. D. Yoffe, Adv. Phys., 1969, 18, 193–335 CrossRef CAS.
-
W. Y. Liang, in Intercalation in Layered Materials, ed. M. S. Dresselhaus, Springer US, Boston, MA, 1986, pp. 31–73 Search PubMed.
- E. Canadell, S. Jobic, R. Brec, J. Rouxel and M.-H. Whangbo, J. Solid State Chem., 1992, 99, 189–199 CrossRef CAS.
- K. F. Mak and J. Shan, Nat. Photonics, 2016, 10, 216–226 CrossRef CAS.
- S. Song, D. H. Keum, S. Cho, D. Perello, Y. Kim and Y. H. Lee, Nano Lett., 2016, 16, 188–193 CrossRef CAS PubMed.
- K.-A. N. Duerloo, Y. Li and E. J. Reed, Nat. Commun., 2014, 5, 4214 CrossRef CAS PubMed.
- S. Cho, S. Kim, J. H. Kim, J. Zhao, J. Seok, D. H. Keum, J. Baik, D.-H. Choe, K. J. Chang, K. Suenaga, S. W. Kim, Y. H. Lee and H. Yang, Science, 2015, 349, 625–628 CrossRef CAS PubMed.
- Y. Wang, J. Xiao, H. Zhu, Y. Li, Y. Alsaid, K. Y. Fong, Y. Zhou, S. Wang, W. Shi, Y. Wang, A. Zettl, E. J. Reed and X. Zhang, Nature, 2017, 550, 487–491 CrossRef CAS PubMed.
- H. Zhang, C. Bao, Z. Jiang, K. Zhang, H. Li, C. Chen, J. Avila, Y. Wu, W. Duan, M. C. Asensio and S. Zhou, Nano Lett., 2018, 18, 4664–4668 CrossRef CAS PubMed.
- E. Uchida and H. Kondoh, J. Phys. Soc. Jpn., 1956, 11, 21–27 CrossRef CAS.
- T. T. M. Palstra, M. L. Steigerwald, A. P. Ramirez, Y.-U. Kwon, S. M. Stuczynski, L. F. Schneemeyer, J. V. Waszczak and J. Zaanen, Phys. Rev. Lett., 1993, 71, 1768–1771 CrossRef CAS PubMed.
- A. Puthirath Balan, S. Radhakrishnan, R. Neupane, S. Yazdi, L. Deng, C. A. de los Reyes, A. Apte, A. B. Puthirath, B. M. Rao, M. Paulose, R. Vajtai, C.-W. Chu, A. A. Martí, O. K. Varghese, C. S. Tiwary, M. R. Anantharaman and P. M. Ajayan, ACS Appl. Nano Mater., 2018, 1, 6427–6434 CrossRef CAS.
- Y.-X. Lei, N.-X. Miao, J.-P. Zhou, Q. U. Hassan and J.-Z. Wang, Sci. Technol. Adv. Mater., 2017, 18, 325–333 CrossRef CAS PubMed.
- H. E. Lim, Y. Nakanishi, Z. Liu, J. Pu, M. Maruyama, T. Endo, C. Ando, H. Shimizu, K. Yanagi, S. Okada, T. Takenobu and Y. Miyata, Nano Lett., 2021, 21, 243–249 CrossRef CAS PubMed.
- T. Chivers and R. S. Laitinen, Chem. Soc. Rev., 2015, 44, 1725–1739 RSC.
- M. Calandra, Nat. Nanotechnol., 2015, 10, 737–738 CrossRef CAS PubMed.
- M. Calandra, I. I. Mazin and F. Mauri, Phys. Rev. B, 2009, 80, 241108 CrossRef.
- Y. Ge and A. Y. Liu, Phys. Rev. B, 2012, 86, 104101 CrossRef.
- X. Xi, L. Zhao, Z. Wang, H. Berger, L. Forró, J. Shan and K. F. Mak, Nat. Nanotechnol., 2015, 10, 765–769 CrossRef CAS PubMed.
- Y. Song, F. Meng, T. Ying, J. Deng, J. Wang, X. Han, Q. Zhang, Y. Huang, J. Guo and X. Chen, J. Phys. Chem. Lett., 2021, 12, 12180–12186 CrossRef CAS PubMed.
- P. Dreher, W. Wan, A. Chikina, M. Bianchi, H. Guo, R. Harsh, S. Mañas-Valero, E. Coronado, A. J. Martínez-Galera, P. Hofmann, J. A. Miwa and M. M. Ugeda, ACS Nano, 2021, 15, 19430–19438 CrossRef CAS PubMed.
- J.-A. Yan, M. A. D. Cruz, B. Cook and K. Varga, Sci. Rep., 2015, 5, 16646 CrossRef CAS PubMed.
- T. Dai, S. Kang, X. Ma, S. Dang, H. Li, Z. Ruan, W. Zhou, P. Hu, S. Li and S. Wu, J. Phys. Chem. C, 2019, 123, 18711–18716 CrossRef CAS.
- M. Sun, A. V. Parafilo, K. H. A. Villegas, V. M. Kovalev and I. G. Savenko, New J. Phys., 2021, 23, 023023 CrossRef CAS.
- V. Fatemi, S. Wu, Y. Cao, L. Bretheau, Q. D. Gibson, K. Watanabe, T. Taniguchi, R. J. Cava and P. Jarillo-Herrero, Science, 2018, 362, 926–929 CrossRef CAS PubMed.
- Q. Li, C. He, Y. Wang, E. Liu, M. Wang, Y. Wang, J. Zeng, Z. Ma, T. Cao, C. Yi, N. Wang, K. Watanabe, T. Taniguchi, L. Shao, Y. Shi, X. Chen, S.-J. Liang, Q.-H. Wang and F. Miao, Nano Lett., 2018, 18, 7962–7968 CrossRef CAS PubMed.
- L. Zhu, Q.-Y. Li, Y.-Y. Lv, S. Li, X.-Y. Zhu, Z.-Y. Jia, Y. B. Chen, J. Wen and S.-C. Li, Nano Lett., 2018, 18, 6585–6590 CrossRef CAS PubMed.
- A. Sufyan, G. Macam, C.-H. Hsu, Z.-Q. Huang, S.-M. Huang, H. Lin and F.-C. Chuang, Chin. J. Phys., 2021, 73, 95–102 CrossRef CAS.
- R. Sankar, G. Narsinga Rao, I. P. Muthuselvam, C. Butler, N. Kumar, G. Senthil Murugan, C. Shekhar, T.-R. Chang, C.-Y. Wen, C.-W. Chen, W.-L. Lee, M.-T. Lin, H.-T. Jeng, C. Felser and F. C. Chou, Chem. Mater., 2017, 29, 699–707 CrossRef CAS.
- C. H. Naylor, W. M. Parkin, J. Ping, Z. Gao, Y. R. Zhou, Y. Kim, F. Streller, R. W. Carpick, A. M. Rappe, M. Drndić, J. M. Kikkawa and A. T. C. Johnson, Nano Lett., 2016, 16, 4297–4304 CrossRef CAS PubMed.
- A. Kononov, G. Abulizi, K. Qu, J. Yan, D. Mandrus, K. Watanabe, T. Taniguchi and C. Schönenberger, Nano Lett., 2020, 20, 4228–4233 CrossRef CAS PubMed.
- C. Zhao, M. Hu, J. Qin, B. Xia, C. Liu, S. Wang, D. Guan, Y. Li, H. Zheng, J. Liu and J. Jia, Phys. Rev. Lett., 2020, 125, 046801 CrossRef CAS PubMed.
- J. Jiang, Z. K. Liu, Y. Sun, H. F. Yang, C. R. Rajamathi, Y. P. Qi, L. X. Yang, C. Chen, H. Peng, C.-C. Hwang, S. Z. Sun, S.-K. Mo, I. Vobornik, J. Fujii, S. S. P. Parkin, C. Felser, B. H. Yan and Y. L. Chen, Nat. Commun., 2017, 8, 13973 CrossRef CAS PubMed.
- A. A. Soluyanov, D. Gresch, Z. Wang, Q. Wu, M. Troyer, X. Dai and B. A. Bernevig, Nature, 2015, 527, 495–498 CrossRef CAS PubMed.
- K. Deng, G. Wan, P. Deng, K. Zhang, S. Ding, E. Wang, M. Yan, H. Huang, H. Zhang, Z. Xu, J. Denlinger, A. Fedorov, H. Yang, W. Duan, H. Yao, Y. Wu, S. Fan, H. Zhang, X. Chen and S. Zhou, Nat. Phys., 2016, 12, 1105–1110 Search PubMed.
- S. Lee, J. Jang, S.-I. Kim, S.-G. Jung, J. Kim, S. Cho, S. W. Kim, J. Y. Rhee, K.-S. Park and T. Park, Sci. Rep., 2018, 8, 13937 Search PubMed.
- M. N. Ali, J. Xiong, S. Flynn, J. Tao, Q. D. Gibson, L. M. Schoop, T. Liang, N. Haldolaarachchige, M. Hirschberger, N. P. Ong and R. J. Cava, Nature, 2014, 514, 205–208 CrossRef CAS PubMed.
- Y. Wu, D. Mou, N. H. Jo, K. Sun, L. Huang, S. L. Bud’ko, P. C. Canfield and A. Kaminski, Phys. Rev. B, 2016, 94, 121113 CrossRef.
- S. Li, iScience, 2021, 24, 103229 CrossRef CAS PubMed.
-
H. O. Pierson, Handbook of Chemical Vapor Deposition: Principles, Technology and Applications, William Andrew, 1999 Search PubMed.
- P.-C. Shen, Y. Lin, H. Wang, J.-H. Park, W. S. Leong, A.-Y. Lu, T. Palacios and J. Kong, IEEE Trans. Electron Devices, 2018, 65, 4040–4052 CAS.
-
J.-H. Park and T. S. Sudarshan, Chemical Vapor Deposition, ASM International, 2001 Search PubMed.
- J. Zhou, F. Liu, J. Lin, X. Huang, J. Xia, B. Zhang, Q. Zeng, H. Wang, C. Zhu, L. Niu, X. Wang, W. Fu, P. Yu, T.-R. Chang, C.-H. Hsu, D. Wu, H.-T. Jeng, Y. Huang, H. Lin, Z. Shen, C. Yang, L. Lu, K. Suenaga, W. Zhou, S. T. Pantelides, G. Liu and Z. Liu, Adv. Mater., 2017, 29, 1603471 CrossRef PubMed.
- Q. Zhang, Y. Xiao, T. Zhang, Z. Weng, M. Zeng, S. Yue, R. G. Mendes, L. Wang, S. Chen, M. H. Rümmeli, L. Peng and L. Fu, Chem. Mater., 2017, 29, 4641–4644 CrossRef CAS.
- H. Ma, W. Dang, X. Yang, B. Li, Z. Zhang, P. Chen, Y. Liu, Z. Wan, Q. Qian, J. Luo, K. Zang, X. Duan and X. Duan, Chem. Mater., 2018, 30, 8891–8896 CrossRef CAS.
- X. Zhang, Z. Jin, L. Wang, J. A. Hachtel, E. Villarreal, Z. Wang, T. Ha, Y. Nakanishi, C. S. Tiwary, J. Lai, L. Dong, J. Yang, R. Vajtai, E. Ringe, J. C. Idrobo, B. I. Yakobson, J. Lou, V. Gambin, R. Koltun and P. M. Ajayan, ACS Appl. Mater. Interfaces, 2019, 11, 12777–12785 CrossRef CAS PubMed.
- H. Soonmin, M. B. Tahir, S. N. Das and M. R. Das, Eurasian J. Anal. Chem., 2019, 14, 165–172 Search PubMed.
- B. Pandit, S. R. Rondiya, R. W. Cross, N. Y. Dzade and B. R. Sankapal, Chem. Eng. J., 2022, 429, 132505 CrossRef CAS.
- M. Sağlam and B. Güzeldir, Mater. Res. Bull., 2016, 81, 55–62 CrossRef.
-
O. Schäf, H. Ghobarkar and P. Knauth, in Nanostructured Materials: Selected Synthesis Methods Properties and Applications, ed. P. Knauth and J. Schoonman, Springer US, Boston, MA, 2002, pp. 23–41 Search PubMed.
- S. Feng and R. Xu, Acc. Chem. Res., 2001, 34, 239–247 CrossRef CAS PubMed.
- R. A. Hussain and I. Hussain, Mater. Chem. Phys., 2020, 256, 123691 CrossRef CAS.
- M. K. Devaraju and I. Honma, Adv. Energy Mater., 2012, 2, 284–297 CrossRef CAS.
- H. T. Zhang, Y. M. Xiong, X. G. Luo, C. H. Wang, S. Y. Li and X. H. Chen, J. Cryst. Growth, 2002, 242, 259–262 CrossRef CAS.
- Z. Wang and L. Zhang, ChemElectroChem, 2018, 5, 1153–1158 CrossRef CAS.
- L. Ding, J. Wei, Y. Qiu, Y. Wang, Z. Wen, J. Qian, N. Hao, C. Ding, Y. Li and K. Wang, Chem. Eng. J., 2021, 407, 127213 CrossRef CAS.
- N. Karuppusamy, V. Mariyappan, S.-M. Chen, M. Keerthi and R. Ramachandran, Colloids Surf., A, 2021, 626, 127094 CrossRef CAS.
- K. Byrappa and T. Adschiri, Prog. Cryst. Growth Charact. Mater., 2007, 53, 117–166 CrossRef CAS.
- W. Ruythooren, K. Attenborough, S. Beerten, P. Merken, J. Fransaer, E. Beyne, C. V. Hoof, J. D. Boeck and J. P. Celis, J. Micromechanics Microengineering, 2000, 10, 101–107 CrossRef CAS.
- X. Chen and S. S. Mao, Chem. Rev., 2007, 107, 2891–2959 CrossRef CAS PubMed.
- S. S. Dhasade, S. H. Han and V. J. Fulari, J. Semicond., 2012, 33, 093002 CrossRef.
- G. She, X. Zhang, W. Shi, Y. Cai, N. Wang, P. Liu and D. Chen, Cryst. Growth Des., 2008, 8, 1789–1791 CrossRef CAS.
- K.-R. Park, S. Kim, N. V. Myung, S.-O. Kang and Y.-H. Choa, RSC Adv., 2015, 5, 29782–29785 RSC.
- D. Khalafallah, H. Weibo, M. Zhi and Z. Hong, Energy Environ. Mater., 2022, e12528 Search PubMed.
- K.-R. Park, S. Kim, N. V. Myung, S.-O. Kang and Y.-H. Choa, RSC Adv., 2015, 5, 29782–29785 RSC.
-
M. A. Herman and H. Sitter, Molecular Beam Epitaxy: Fundamentals and Current Status, Springer Science & Business Media, 2012 Search PubMed.
- R. Samal and C. S. Rout, Adv. Mater. Interfaces, 2020, 7, 1901682 CrossRef CAS.
- A. Y. Cho, J. Appl. Phys., 1970, 41, 2780–2786 CrossRef CAS.
- K. Lasek, P. M. Coelho, K. Zberecki, Y. Xin, S. K. Kolekar, J. Li and M. Batzill, ACS Nano, 2020, 14, 8473–8484 CrossRef CAS PubMed.
- A.-M. Qin, Y.-P. Fang and C.-Y. Su, Mater. Lett., 2007, 61, 126–129 CrossRef CAS.
- A. Qin, Y. Fang, P. Tao, J. Zhang and C. Su, Inorg. Chem., 2007, 46, 7403–7409 CrossRef CAS PubMed.
- R. Shi, X. Liu, Y. Shi, R. Ma, B. Jia, H. Zhang and G. Qiu, J. Mater. Chem., 2010, 20, 7634–7636 RSC.
- K.-R. Park, H.-B. Cho and Y.-H. Choa, Mater. Chem. Phys., 2017, 189, 64–69 CrossRef CAS.
- L. Meng, C. Xu, H. Li, X. Wang and X. Yan, Chem. Phys. Lett., 2019, 728, 156–159 CrossRef CAS.
- X. Zhang, Q. Lu, W. Liu, W. Niu, J. Sun, J. Cook, M. Vaninger, P. F. Miceli, D. J. Singh, S.-W. Lian, T.-R. Chang, X. He, J. Du, L. He, R. Zhang, G. Bian and Y. Xu, Nat. Commun., 2021, 12, 2492 CrossRef CAS PubMed.
- X. Wang, A. Y. Mehandzhiyski, B. Arstad, K. L. Van Aken, T. S. Mathis, A. Gallegos, Z. Tian, D. Ren, E. Sheridan, B. A. Grimes, D. Jiang, J. Wu, Y. Gogotsi and D. Chen, J. Am. Chem. Soc., 2017, 139, 18681–18687 CrossRef CAS PubMed.
- A. Patra, N. K, J. R. Jose, S. Sahoo, B. Chakraborty and C. S. Rout, J. Mater. Chem. A, 2021, 9, 25852–25891 RSC.
- A. C. Forse, C. Merlet, J. M. Griffin and C. P. Grey, J. Am. Chem. Soc., 2016, 138, 5731–5744 CrossRef CAS PubMed.
- B. Pandit, S. R. Rondiya, R. W. Cross, N. Y. Dzade and B. R. Sankapal, Chem. Eng. J., 2022, 429, 132505 CrossRef CAS.
- K. A. Sree Raj, N. Barman, S. Radhakrishnan, R. Thapa and C. S. Rout, J. Mater. Chem. A, 2022, 10, 23590–23602 RSC.
- H. Mao, J. Yu, J. Li, T. Zheng, J. Cen and Y. Ye, Ceram. Int., 2020, 46, 6991–6994 CrossRef CAS.
- S. Zhang, D. Yang, M. Zhang, Y. Liu, T. Xu, J. Yang and Z.-Z. Yu, Inorg. Chem. Front., 2020, 7, 477–486 RSC.
- B. Ye, S. Xiao, X. Cao, J. Chen, A. Zhou, Q. Zhao, W. Huang and J. Wang, J. Power Sources, 2021, 506, 230056 CrossRef CAS.
- S. Deshagani, P. Ghosal and M. Deepa, Electrochim. Acta, 2020, 345, 136200 CrossRef CAS.
- S. Manzeli, D. Ovchinnikov, D. Pasquier, O. V. Yazyev and A. Kis, Nat. Rev. Mater., 2017, 2, 1–15 Search PubMed.
- L. K. Bommineedi, T. B. Deshmukh, A. Agarwal, N. Upadhyay, A. C. Mendhe, S. R. Sankapal, S. A. Pande and B. R. Sankapal, J. Taiwan Inst. Chem. Eng., 2023, 142, 104607 CrossRef CAS.
- C. Gong, L. Li, Z. Li, H. Ji, A. Stern, Y. Xia, T. Cao, W. Bao, C. Wang, Y. Wang, Z. Q. Qiu, R. J. Cava, S. G. Louie, J. Xia and X. Zhang, Nature, 2017, 546, 265–269 CrossRef CAS PubMed.
- T. Li, H. Li, J. Yuan, Y. Xia, Y. Liu and A. Sun, Materials, 2022, 15, 2654 CrossRef CAS PubMed.
- N. Choudhary, C. Li, H.-S. Chung, J. Moore, J. Thomas and Y. Jung, ACS Nano, 2016, 10, 10726–10735 CrossRef CAS PubMed.
- H. Kwon, D. Bae, D. Won, H. Kim, G. Kim, J. Cho, H. J. Park, H. Baik, A. R. Jeong, C.-H. Lin, C.-Y. Chiang, C.-S. Ku, H. Yang and S. Cho, ACS Nano, 2021, 15, 6540–6550 CrossRef CAS PubMed.
- W. Gao, B. Song, Q. Zhang, J. He and Y. Wu, Small, 2023, 2310225 Search PubMed.
- C. Zhong, Y. Deng, W. Hu, J. Qiao, L. Zhang and J. Zhang, Chem. Soc. Rev., 2015, 44, 7484–7539 RSC.
- A. Mendhe and H. S. Panda, Discov. Mater., 2023, 3, 29 CrossRef.
- S. S. Karade and B. R. Sankapal, ACS Sustain. Chem. Eng., 2018, 6, 15072–15082 CrossRef CAS.
- P. Bhol, P. B. Jagdale, N. Barman, R. Thapa, M. Saxena and A. K. Samal, J. Energy Storage, 2023, 65, 107286 CrossRef.
- H. K. Rathore, M. K. G. Singh, D. Debanjan, K. Manoj, K. Awasthi and S. Debasish, J. Colloid Interface Sci., 2022, 621, 110–118 CrossRef CAS PubMed.
- M. Liu, Z. Wang, J. Liu, G. Wei, J. Du, Y. Li, C. An and J. Zhang, J. Mater. Chem. A, 2017, 5, 1035–1042 RSC.
- R. Hu, H. Qiao, Y. Shu, J. Li, Z. Huang, J. Tao and X. Qi, J. Electron. Mater., 2021, 50, 2277–2286 CrossRef CAS.
- S. Sarwar, Z. Liu, J. Li, Y. Wang, R. Wang and X. Zhang, J. Alloys Compd., 2020, 846, 155886 CrossRef CAS.
- M. Abdullah, S. Khan, K. Jabbour, M. Imran, M. F. Ashiq, P. John, S. Manzoor, T. Munawar and M. N. Ashiq, Electrochim. Acta, 2023, 466, 143020 CrossRef CAS.
- Y. Li, X. Xiao, F. Yang and C. An, ACS Appl. Energy Mater., 2022, 5, 10680–10689 CrossRef CAS.
- M. Liu, X. Wang, Z. Huang, P. Guo and Z. Wang, Mater. Lett., 2017, 206, 229–232 CrossRef CAS.
- D. Vikraman, S. Hussain, I. Rabani, A. Feroze, M. Ali, Y.-S. Seo, S.-H. Chun, J. Jung and H.-S. Kim, Nano Energy, 2021, 87, 106161 CrossRef CAS.
- S. Sarwar, Z. Liu, J. Li, Y. Wang, R. Wang and X. Zhang, J. Alloys Compd., 2020, 846, 155886 CrossRef CAS.
- M. Liu, X. Wang, Z. Huang, P. Guo and Z. Wang, Mater. Lett., 2017, 206, 229–232 CrossRef CAS.
- S. S. Karade and B. R. Sankapal, ACS Sustain. Chem. Eng., 2018, 6, 15072–15082 CrossRef CAS.
- A. Sajedi-Moghaddam, C. C. Mayorga-Martinez, E. Saievar-Iranizad, Z. Sofer and M. Pumera, Appl. Mater. Today, 2019, 16, 280–289 CrossRef.
- P. Yu, W. Fu, Q. Zeng, J. Lin, C. Yan, Z. Lai, B. Tang, K. Suenaga, H. Zhang and Z. Liu, Adv. Mater., 2017, 29, 1701909 CrossRef PubMed.
- M. Manikandan, K. Subramani, M. Sathish and S. Dhanuskodi, ChemistrySelect, 2018, 3, 9034–9040 CrossRef CAS.
- F. Zhang, T. Eom, M. Cho, H.-J. Lee and H. Pang, J. Energy Storage, 2021, 42, 103098 CrossRef.
- N. Jayababu, S. Jo, Y. Kim and D. Kim, ACS Appl. Mater. Interfaces, 2021, 13, 19938–19949 CrossRef CAS PubMed.
- N. Jayababu, S. Jo, Y. Kim and D. Kim, ACS Appl. Mater. Interfaces, 2021, 13, 19938–19949 CrossRef CAS PubMed.
- M. Farshadnia, A. A. Ensafi, K. Z. Mousaabadi, B. Rezaei and M. Demir, Sci. Rep., 2023, 13, 1364 CrossRef CAS PubMed.
- M. Molaei, G. R. Rostami, A. M. Zardkhoshoui and S. S. H. Davarani, J. Colloid Interface Sci., 2024, 653, 1683–1693 CrossRef CAS PubMed.
- K. L. Meghanathan, M. Parthibavarman, V. Sharmila and J. R. Joshua, J. Energy Storage, 2023, 72, 108665 CrossRef.
- N. Y. W. Zaw, S. Jo, J. Park, N. Kitchamsetti, N. Jayababu and D. Kim, Appl. Clay Sci., 2022, 225, 106539 CrossRef CAS.
- B. Ye, M. Huang, L. Fan, J. Lin and J. Wu, J. Alloys Compd., 2019, 776, 993–1001 CrossRef CAS.
- K. S. Bhat, S. Shenoy, H. S. Nagaraja and K. Sridharan, Electrochim. Acta, 2017, 248, 188–196 CrossRef CAS.
- H. Mao, J. Yu, J. Li, T. Zheng, J. Cen and Y. Ye, Ceram. Int., 2020, 46, 6991–6994 CrossRef CAS.
- T. Kshetri, T. I. Singh, Y. S. Lee, D. D. Khumujam, N. H. Kim and J. H. Lee, Composites, Part B, 2021, 211, 108624 CrossRef CAS.
- C. Shi, Q. Yang, S. Chen, Y. Xue, Y. Hao and Y. Yan, ACS Appl. Energy Mater., 2022, 5, 2817–2825 CrossRef CAS.
- T. Wang, Y. Su, M. Xiao, M. Zhao, T. Zhao and J. Shen, Trans. Tianjin Univ., 2022, 28, 112–122 CrossRef CAS.
- P. Bhol, S. Swain, A. Altaee, M. Saxena and A. K. Samal, Mater. Today Chem., 2022, 24, 100871 CrossRef CAS.
- K. S. Bhat, S. Shenoy, H. S. Nagaraja and K. Sridharan, Electrochim. Acta, 2017, 248, 188–196 CrossRef CAS.
- M. Manikandan, K. Subramani, M. Sathish and S. Dhanuskodi, RSC Adv., 2020, 10, 13632–13641 RSC.
- P. Bhol, S. Swain, A. Altaee, M. Saxena and A. K. Samal, Mater. Today Chem., 2022, 24, 100871 CrossRef CAS.
- D. Chakravarty, P. Kumar, V. S. Ugale and D. J. Late, Eur. J. Inorg. Chem., 2015 DOI:10.1002/ejic.201403220.
- V. S. Kumbhar, A. C. Lokhande, N. S. Gaikwad and C. D. Lokhande, Chem. Phys. Lett., 2016, 645, 112–117 CrossRef CAS.
- V. S. Kumbhar, A. C. Lokhande, N. S. Gaikwad and C. D. Lokhande, Mater. Sci. Semicond. Process., 2016, 46, 29–34 CrossRef CAS.
- M. Manikandan, P. N. Francis, S. Dhanuskodi, N. Maheswari and G. Muralidharan, J. Mater. Sci.: Mater. Electron., 2018, 29, 17397–17404 CrossRef CAS.
- L. K. Bommineedi, T. B. Deshmukh, A. Agarwal, N. Upadhyay, A. C. Mendhe, S. R. Sankapal, S. A. Pande and B. R. Sankapal, J. Taiwan Inst. Chem. Eng., 2023, 142, 104607 CrossRef CAS.
- D. Dehghanpour Farashah, F. Beigloo, A. Mohammadi Zardkhoshoui and S. Saeed Hosseiny Davarani, Chem. Eng. J., 2023, 474, 145584 CrossRef CAS.
- V. S. Kumbhar, A. C. Lokhande, N. S. Gaikwad and C. D. Lokhande, Chem. Phys. Lett., 2016, 645, 112–117 CrossRef CAS.
- K. Cui, L. Du, W. Du, W. Chen, Y. Zhang, L. Cui and D. Wu, J. Alloys Compd., 2023, 955, 170290 CrossRef CAS.
- A. P. Nayak, Z. Yuan, B. Cao, J. Liu, J. Wu, S. T. Moran, T. Li, D. Akinwande, C. Jin and J.-F. Lin, ACS Nano, 2015, 9, 9117–9123 CrossRef CAS PubMed.
Footnote |
† CD and SR contributed equally. |
|
This journal is © The Royal Society of Chemistry 2024 |