DOI:
10.1039/D4SC01802H
(Edge Article)
Chem. Sci., 2024,
15, 9557-9565
Rapid solar-driven atmospheric water-harvesting with MAF-4-derived nitrogen-doped nanoporous carbon†
Received
18th March 2024
, Accepted 9th May 2024
First published on 11th May 2024
Abstract
Sorption-based atmospheric water-harvesting (AWH) could help to solve global freshwater scarcity. The search for adsorbents with high water-uptake capacity at low relative humidity, rapid adsorption–desorption kinetics and high thermal conductivity is a critical challenge in AWH. Herein, we report a MAF-4 (aka ZIF-8)-derived nanoporous carbon (NPCMAF-4-800) with multiple N-doped sites, considerable micropore characteristics and inherent photothermal properties, for efficient water production in a relatively arid climate. NPCMAF-4-800 exhibited optimal water-sorption performance of 306 mg g−1 at 40% relative humidity (RH). An excellent sunlight-absorption rate was realized (97%) attributed to its high degree of graphitization. A proof-of-concept device was designed and investigated for the practical harvesting of water from the atmosphere using natural sunlight. NPCMAF-4-800 achieved an unprecedentedly high water production rate of 380 mg g−1 h−1 at 40% RH, and could produce 1.77 L kg−1 freshwater during daylight hours in an outdoor low-humidity climate of ∼25 °C and 40% RH. These findings may shed light on the potential of MOF-derived porous carbons in the AWH field, and inspire the future development of solar-driven water-generation systems.
Introduction
The shortage of fresh potable water is a critical challenge that threatens human health and economic development.1,2 The atmosphere holds ∼13 thousand trillion liters of fresh water in the form of vapor and droplets. Atmospheric water-harvesting (AWH) is an attractive way to capture water vapor from air. Then, the adsorbed water is condensed into liquid water and concentrated in a container.3,4 Typically, several traditional AWH technologies are utilized for water collection and onsite production. For example, fog collection is achieved by a network of fibers or meshes with macroscopic pores, and water droplets are gathered into a container.5 Dew condensation can be realized by a refrigerator with the ambient temperature below the dew point, and then the water is condensed from moist air.6 However, the technologies stated above face major challenges, such as universality under an extremely arid environment.7,8 In recent years, adsorbent technology based on porous solids, with moisture capture in relatively arid conditions (relative humidity (RH) ≤40%) and exploitation of low-grade energy (e.g., sunlight, waste energy) for desorption, has become an optimal strategy to achieve water-harvesting from the atmosphere on an industrial scale.9–12 Among them, the adsorption–desorption cycle of AWH systems is dependent upon the water–absorption properties of porous solids at specific humidity. In addition, the rapid adsorption–desorption kinetics of materials have important roles for an increased water yield through more water-harvesting cycles.13 Recently, one study demonstrated that a tremendous increase in water production could be realized if multiple water-production cycles within 1 day were instigated.14 Therefore, the development of new porous adsorbents with excellent water uptake and rapid rate of adsorption–desorption could enable the scale-up of AWH systems that work with greater efficiency and productivity.
Several types of porous adsorbents, such as silica gels,15 zeolites,16 activated carbon17 and metal–organic frameworks (MOFs),18–20 have been investigated for water capture from the atmosphere. Silica gels can achieve high water uptake with large-pore properties. However, due to their hydrophobicity, water uptake is restricted to high RH.21,22 Zeolites can capture water at relatively low moisture, but the strong interaction between the absorption site and water molecule results in higher energy consumption.23 Activated carbon can rapidly adsorb water molecules onto oxygenated/hydroxylated surface sites, but its saturated water-absorption capacity is relatively low.24 Among these materials, MOFs have rich porosity, structural diversity, and numerous open sites. Researchers have developed them and their derivatives for AWH.25–27 Yaghi et al. determined the water-filling mechanism of MOF-303 by single-crystal X-ray diffraction (SCXRD). They elucidated that the N heteroatoms of organic linkers have a dominant role as adsorptive sites in a MOF.28 Yaghi et al. developed a multivariate strategy for “tuning” the hydrophilicity, regeneration temperatures and heat of the MOF by introducing active groups.29 Unfortunately, due to the extremely low photothermal conversion, the MOF usually must be mixed with a solar absorber (e.g., carbon black, CNTs, graphene) to realize passive solar-driven desorption.30–32 However, the overall energy efficiency is impaired by restrictions elicited by the poor thermal transmission between the water adsorbent and solar absorber.33 An effective strategy to overcome this shortcoming is the thermal conversion from MOFs to derived nanoporous carbon.34 Under thermal conversion, the metal species can be easily separated from the backbone, thereby leaving nitrogen heterocyclic rings to blend around metal ions or clusters. Meanwhile, the microporosity of the MOF-derived carbon should be increased further by leaching the metal species with an acid solution. Therefore, MOF-derived carbons obtained through pyrolysis can inherit the intrinsic pore structures of MOF precursors, but also increase the contents of N-doped or O-doped species to improve the affinity of water molecules. More importantly, the additives needed to absorb solar energy in the desorption process can be exempt because of the black colour of derived carbons.
As a typical metal-azolate framework (MAF), zinc(II) 2-methylimidazolate (MAF-4, also known as ZIF-8)35–37 features a sodalite zeolite-type structure composed of tetrahedral metal ions and imdazolate ligands. MAF-4 has micropores and rich N-containing functional groups.38,39 Compared with other MOFs, MAF-4 is ideal as a basic precursor for preparing N-doped nanoporous carbon due to its facile and scalable synthesis, as well as the readily available and inexpensive raw material of the 2-methylimidazole ligand. Herein, advanced MAF-4-derived nanoporous carbon (NPCMAF-4-800) with ideal micropore characteristics, rich N-doped species and inherent photothermal properties was obtained for atmospheric water-harvesting (Scheme 1).
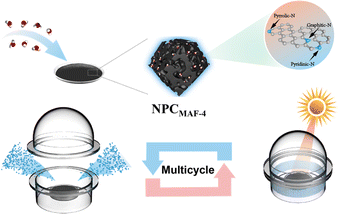 |
| Scheme 1 Water capture with MAF-4-derived N-doped nanoporous carbon (NPCMAF-4) materials and the working principle of a solar-driven atmosphere water-harvesting device (schematic). | |
Specially, NPCMAF-4-800 displayed a remarkable capacity for water uptake at relatively low humidity, rapid water adsorption/desorption kinetics and excellent photothermal performance. According to the properties stated above, we demonstrated a solar-driven AWH device based on NPCMAF-4-800 realizing the highest water-harvesting rate of 380 mg g−1 h−1 at 40% RH and achieving 1.77 L kg−1 fresh-water production during the daytime outdoors.
Materials and methods
Materials
All chemicals were obtained commercially and used directly without further purification. Zinc nitrate hexahydrate (Zn(NO3)2·6H2O; purity = 99.0%) was purchased from Xilong Science. 2-Methylimidazole (98.0%) was obtained from Damas-beta. Methanol (AR) was sourced from Macklin. Hydrochloric acid (AR) was purchased from Xilong Science.
Synthesis of MAF-4
Zn(NO3)2·6H2O (1.68 g (5.65 mmol)) was added to 80 mL of methanol and stirred for 20 min. In another vial, 3.7 g (45.06 mmol) of 2-methtlimidazole was dissolved in 80 mL of methanol with vigorous stirring. Then, the second solution was added dropwise into the first solution with stirring to form a white suspension. The resulting mixture was stirred at room temperature for 24 h to afford the white crystals of MAF-4. Finally, the crystals were collected by centrifugation and washed thrice with methanol. The resulting MAF-4 was dried at 70 °C before further carbonization.
Synthesis of MAF-4 derived N-doped nanoporous carbon
The carbonization of MAF-4 was realized by direct pyrolysis using a tubular furnace. MAF-4 powders (500 mg) were spread on a quartz vessel, and calcined in a N2 atmosphere at 700 °C, 800 °C and 900 °C for 2 h in a furnace at a heating rate of 5 °C min−1, respectively. The carbonized products were cooled down to ambient temperature and subsequently pickled in 2 M aqueous HCl solution under stirring for 24 h to remove metal species and residual solvent. After that, the derived carbon was centrifuged and rinsed with deionized water twice. The sample was dried at 70 °C overnight to yield N-doped nanoporous carbons, which were labeled NPCMAF-4-T, where T represents the carbonization temperature (700, 800 or 900 °C).
Characterization
Powder X-ray diffraction (PXRD) patterns were acquired using a an X-ray diffractometer (Ultima IV; Rigaku) with Cu-Kα (λ = 1.5418 Å) radiation operated at a generator voltage of 40 kV and generator current of 40 mA. Scanning was performed over a range 2θ = 3–80° at 2.0°·min−1. Scanning electron microscopy (SEM) images were obtained using an Apreo 2S HiVac system (Thermo Fisher Scientific) with an acceleration voltage of 20 kV. Nitrogen adsorption–desorption isotherms were used to investigate the specific surface area and pore characteristics of samples utilizing a physisorption analyzer (ASAP 2460; Micromeritics) at 77 K. The surface area was calculated using the Brunauer–Emmett–Teller (BET) method at P/P0 = 0.01–0.3. Pore-size distributions were analyzed by nonlinear density functional theory (NLDFT). Before measurement, the sample was degassed for 24 h at 150 °C under a vacuum. The elemental compositions of samples were determined on an elemental analyzer (EL; Vario). The inherent light absorption of samples was directly characterized by an UV-vis-NIR spectrophotometer (Lambda 950; PerkinElmer). Raman spectroscopy (using 532 nm as the excitation wavelength) on a DXR 2xi System (Thermo Fisher Scientific) was employed to investigate the defect density and molecular configuration of derived carbons. X-ray photoelectron (XPS) studies were conducted on a spectrometer (Nexsa; Thermo Fisher Scientific) with a monochromatic Al-Kα X-ray source, all spectra were calibrated using the C 1s neutral-carbon peak at 284.8 eV. The content of metal ions in water captured by an AWH device was tested through inductively coupled plasma-optical emission spectroscopy (ICP-OES).
Measurement of adsorption of water vapor
Water-sorption experiments were conducted on a gravimetric vapor sorption analyzer (BSD-DVS&VVS; BSD-Sorb) where the humidity was controlled by a mixture of saturated vapor and dry gas in a certain proportion. Initially, ∼30 mg of samples were pre-activated at 150 °C for 10 h by in situ degassing to ensure complete removal of residual water. Then, they were placed in the crucible of the gravimetric vapor sorption analyzer for adsorption measurement. The weight change after adsorption of water vapor during the measurement was recorded by an electronic balance (accuracy = 0.001 mg) at regular intervals. Each equilibrium value of each corresponding humidity was determined if the weight change was <0.1 mg within 60 min. Dynamic water adsorption–desorption tests were performed on a thermogravimetric module of the BSD-DVS&VVS analyzer. The testing chamber was heated to 150 °C to ensure completely dried NPCMAF-4 samples, and then cooled down to 25 °C by an external constant-temperature water-bath under 40% RH for 100 min for water sorption. After sorption, the chamber was heated from 25 °C to 80 °C within 10 min to release water from the samples. The mass changes of samples in the above process were recorded by an internal precision balance in real-time.
Fabrication of a solar-driven AWH device
As shown in Fig. 6b, the atmospheric water-harvester was assembled by an acrylic curved plate, sample vessel and condenser. Among them, the acrylic curved plate was used to condense vapor into liquid water and collect it in the water-collection area by gravity. The glass vessel served to load the adsorbent, and separate the condensate water and samples. The NPCMAF-4-800 sample was weighed and spread out uniformly on the vessel with a sample thickness of ∼2 mm. The simulated solar light source utilized a sunlight generator (PLS-SXE300; Perfect Light) with a radiation intensity of 1 kW m−2. The surface temperature of the absorbent was measured by an infrared camera (One Pro; FLIR). The weight change of NPCMAF-4-800 was recorded by an analytical balance at certain time intervals. Environmental conditions were controlled at 25 °C and 40% RH. The collected water capacity was determined by weighing the mass of water preserved in the water-collection area.
Results and discussion
Structure and morphological characterization
Primarily, the purity of the obtained MAF-4 precursor was confirmed by PXRD patterns, SEM and N2 adsorption test at 77 K (Fig. 1a and S1†).35 The MAF-4-derived N-doped nanoporous carbons were highly dispersed nanoparticles (size of 100–200 nm) with a polyhedral morphology as revealed by SEM and TEM images (Fig. 2). The prepared NPCMAF-4 inherited the original surface structures after carbonization. TEM images of NPCMAF-4-700 and NPCMAF-4-800 revealed amorphous structures with interconnected micropores, but some worm-like mesopores and locally ordered arrangement could be observed in NPCMAF-4-900.40 Evidently, the interior pore structures of NPCMAF-4 derived from MAF-4 could be tuned by regulating the pyrolysis temperature from 700 °C to 900 °C.
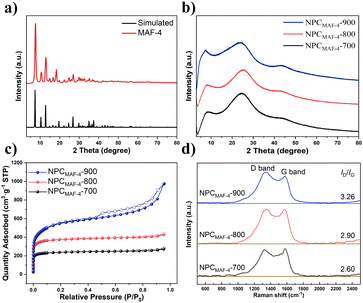 |
| Fig. 1 (a) PXRD patterns of the MAF-4 precursor. Structural characterization of NPCMAF-4. (b) PXRD patterns. (c) N2 adsorption–desorption isotherms at 77 K. (d) Raman spectra. | |
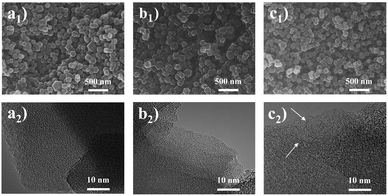 |
| Fig. 2 SEM and TEM images of (a1, a2) NPCMAF-4-700, (b1, b2) NPCMAF-4-800, and (c1, c2) NPCMAF-4-900. | |
As seen in Fig. 1b, the narrow and sharp diffraction peaks of MAF-4 microcrystals changed into broad and low-intensity peaks at ∼2θ = 25° and 44° of derived porous carbons. These two peaks were attributed to (002) and (100) crystal planes, suggesting that NPCMAF-4 products had typical amorphous structures. With an increase in the thermal treatment temperature, the intensity and degree of these peaks became virtually the same, which confirmed the complete graphitization of NPCMAF-4 when the temperature exceeded 700 °C.
The BET surface areas and porosities of MAF-4-derived N-doped nanoporous carbons were characterized by N2 adsorption–desorption at 77 K. The curves of NPCMAF-4-700 and NPCMAF-4-800 exhibited type-I isotherms with no obvious hysteresis loops (Fig. 1c), which manifested the characteristic of micropores. With an increase in the carbonization temperature from 700 °C to 900 °C, the specific surface area of NPCMAF-4 increased gradually from 968 to 2045 m2 g−1 (Table 1). The micropore ratios of NPCMAF-4-700, -800 and -900 were 44.25%, 72.41% and 26.68%, respectively. The micropore volume accounted for a greater proportion of NPCMAF-4-800 than NPCMAF-4-700 or NPCMAF-4-900. As mentioned above, NPCMAF-4-800 had the highest micropore characteristics and an ideal specific surface area.
Table 1 Structural properties of NPCMAF-4-T
Samples |
S
BET
m2 g−1 |
V
total
cm3 g−1 |
V
micro
cm3 g−1 |
N wt% |
S
BET was calculated using a Brunauer–Emmett–Teller (BET) method at P/P0 = 0.01–0.3.
V
total refers to single-point adsorption of the total pore volume of pores at P/P0 = 0.99.
V
micro denotes the t-Plot micropore volume with a pore size ≤ 2 nm. Values in parentheses represent the proportion of the micropore volume relative to the overall pore volume.
|
NPCMAF-4-700 |
968 |
0.66 |
0.29(44.25) |
19.58 |
NPCMAF-4-800 |
1481 |
0.66 |
0.48(72.41) |
16.62 |
NPCMAF-4-900 |
2045 |
1.51 |
0.40(26.68) |
3.67 |
To further explore the crystal configurations of MAF-4-derived N-doped nanoporous carbons, Raman spectroscopy was undertaken. As illustrated in Fig. 1d, NPCMAF-4 samples were confirmed by spectra showing two bands at 1580 cm−1 (G band) and 1360 cm−1 (D band), which were attributed to the symmetric bond stretching of a graphitic structure and breathing modes of a disordered structure, respectively.41,42 The area ratio of the D band to the G band (ID/IG) is used to indicate the defect degree of carbon materials. Obviously, the ID/IG of NPCMAF-4-700, -800, -900 products were at a high level, suggesting many amorphous defects arising from heteroatomic species after carbonization.43,44 Furthermore, the values of ID/IG increased with an increase in the pyrolysis temperature. This finding indicated that the degree of graphitization of NPCMAF-4 was promoted, which could be expected to improve photothermal conversion during solar-driven AWH.
From the perspective of dynamics, the N-doped polar sites from adsorbents may greatly promote low-pressure water absorption, which can induce the formation of water clusters around these sites by hydrogen-bonding interactions.45 Therefore, the N contents in NPCMAF-4 were determined through elemental analysis (Table 1). With an increase in the carbonization temperature, the N content of NPCMAF-4 decreased. Specifically, NPCMAF-4-700 and NPCMAF-4-800 exhibited high N contents of 19.58 wt% and 16.62 wt%, respectively, while NPCMAF-4-900 showed the lowest N content of 3.67 wt%. Hence, NPCMAF-4-700 and NPCMAF-4-800 had more abundant N-decorated adsorption sites and increased the affinity of guest molecules. To further clarify the doping types of N element in MAF-4-derived N-doped nanoporous carbons, the XPS spectra of N1s of NPCMAF-4-700, NPCMAF-4-800, NPCMAF-4-900 were investigated in detail (Fig. 3) and the N1s spectra were deconvolved to pyridinic N (398.4 eV), pyrrolic N (399.8 eV), graphitic N (400.9 eV), and oxidized N (402.4 eV). Pyridinic-N and pyrrolic-N species have more affinity with water molecules than other N species.46,47 Accordingly, XPS showed that the proportion of pyridinic-N and pyrrolic-N species in NPCMAF-4 samples were reduced with an increasing pyrolysis temperature. However, the contents of graphitic-N species gradually increased, suggesting that these stable graphitic-N species had been converted from the above two types of nitrogen groups. The contents of pyridinic-N/pyrrolic-N species of NPCMAF-4-700, NPCMAF-4-800, and NPCMAF-4-900 corresponded to 43.86/16.41, 41.34/15.1, and 36.35/14.97 wt%, respectively (Fig. 3d). Given the well-developed micropore structure and strong N-doped adsorbed sites, we considered that NPCMAF-4-800 was a promising N-doped nanoporous carbon for adsorbing water at relatively low humidity.
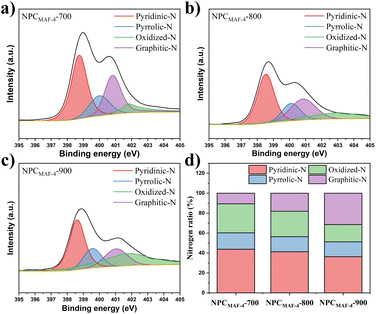 |
| Fig. 3 High-resolution N 1s XPS spectra of (a) NPCMAF-4-700, (b) NPCMAF-4-800, (c) NPCMAF-4-900 and (d) the distribution ratio of N species of NPCMAF-4-T. | |
Water–sorption properties
To verify the water-adsorption ability of MAF-4-derived N-doped nanoporous carbon, we evaluated the dynamic vapor sorption (DVS) isotherms of NPCMAF-4 samples at 298 K. As shown in Fig. 4a, the water-sorption isotherm of NPCMAF-4-900 showed a type-III curve and a two-step process with the highest saturated adsorption capacity (746 mg g−1 at 95% RH) attributed to its highest specific surface area. However, NPCMAF-4-900 displayed very little water uptake at low RH (below 40% RH). This kind of isotherm was due to a shortage of N-doped sites by the excessive pyrolysis temperature, which resulted in a rather weak interaction between the porous carbon and water molecules. Different from the NPCMAF-4-900 sample, both NPCMAF-4-700 and NPCMAF-4-800 exhibited the type-I isotherm characteristic with no apparent hysteresis. Also, water adsorption increased sharply with increasing pressure until 40% RH due to the strong interaction between water molecules and N-doped polar groups. This finding implied that the abundant micropore structure and more N-doped species were conducive to water absorption at relatively low humidity. Moreover, the subsequent slow adsorption above 40% RH indicated that the micropores and adsorption sites of NPCMAF-4-700 and NPCMAF-4-800 were fully occupied by the adsorbate, along with the weak hydrogen-bonding interactions between the absorbent and water molecules.48 NPCMAF-4-800 exhibited the highest absorptivity (306 mg g−1 at 40% RH), which could be attributed to its superior micropore volume and high N content. Compared with the MAF-4 precursor, NPCMAF-4-800 possessed superior water adsorption (Fig. S2†). To evaluate the recyclability of NPCMAF-4-800, water adsorption/desorption experiments were conducted for seven cycles at 40% RH (Fig. 4b). The water-uptake capacity consistently remained ∼306 mg g−1, which aligned with the results for dynamic vapor sorption and illustrated the excellent cyclic stability of NPCMAF-4-800.
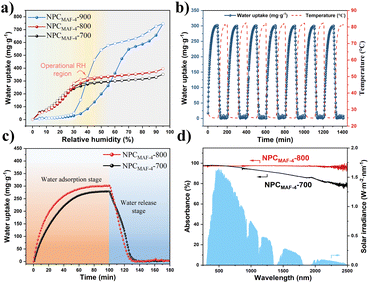 |
| Fig. 4 (a) Water-sorption isotherms at 298 K of NPCMAF-4-T. (b) Stability tests of NPCMAF-4-800 over multiple cycles at 40% RH. (c) Dynamic adsorption–desorption performance of NPCMAF-4-700 and NPCMAF-4-800. (d) UV-vis-NIR absorption spectra of NPCMAF-4-700 and NPCMAF-4-800. | |
The rapid rate of water absorption and desorption at a specific humidity is the key of realizing high-yield water-harvesting. The measurement of water adsorption–desorption dynamics was performed (Fig. 4c). A constant temperature of 25 °C and 40% RH were maintained throughout the sorption process for NPCMAF-4-700 and NPCMAF-4-800. First, the water uptake of NPCMAF-4-700 and NPCMAF-4-800 increased with the adsorption time and reached saturation capacities within 60 min. At 80 °C of the desorption condition (equal to one-solar irradiation), >99% of absorbed water could be released with 30 min for NPCMAF-4-700/800. Notably, the adsorption and desorption kinetics of NPCMAF-4-800 reached 12.50 mg g−1 min−1 and 10.26 mg g−1 min−1 within 10 min, respectively, which were 1.53- and 1.44-times faster than those of NPCMAF-4-700 (section S3†). Consequently, the synergistic effect of more accessible porosity and N-doped adsorption sites of NPCMAF-4-800 could explain the outstanding water absorption and release behavior. Prior to the AWH experiment, evaluation of optical absorption by UV-vis-NIR spectra was done (Fig. 4d). NPCMAF-4-800 exhibited a broadband solar adsorption from 300 nm to 2500 nm with an absorptivity of 97%, higher than that of NPCMAF-4-700. These results showed the higher graphitization of NPCMAF-4-800 with more abundant carbon contents, which coincided with the analysis of Raman spectra.
Water production of a solar-driven AWH device based on NPCMAF-4-800
First, the light-thermal conversion performance is the most crucial factor for the dehydration rate of samples. Therefore, the desorption capability of NPCMAF-4-800 saturated with water from 40% RH under one-solar irradiation was explored (Fig. S3†). As presented in Fig. 5a and b, the heating temperature rapidly increased and reached 75 °C within 10 min irradiation time under the solar simulator (1 kW m−2), which further verified the result of UV-vis-NIR absorption spectroscopy. In addition, the photothermal properties of NPCMAF-4-800 and MOF-303 mixed with graphene were characterized, and the results showed that the NPCMAF-4-800 exhibited higher surface temperature and heat-transfer efficiency (Fig. S4†). Meanwhile, the adsorption of saturated water in NPCMAF-4-800 could be released by >90% during this period. The rapid desorption of NPCMAF-4-800 is beneficial for inherent photothermal properties, which are superior to conventional solar-driven adsorbents requiring an external light-absorbing medium.49,50 Building upon the results stated above, the AWH performance of NPCMAF-4-800 based on a proof-of-concept device under indoor conditions powered by one-solar irradiation was tested, and the detailed experimental method is described in the ESI† (Fig. S5 and S6†). To fully realize the maximum daily water productivity of a water-harvester, it is necessary to investigate the optimal operating parameters of the device, such as adsorption time and solar exposure time.51,52 As shown in Fig. 5c, the results indicated that a time of 10 min adsorption plus 10 min solar irradiation in one cycling test could elicit the highest water production rate of 380 mg g−1 h−1 at 40% RH with one-solar illumination. This result could be attributed to the high water-uptake capacity at low RH, rapid adsorption–desorption rate and the exceptional photothermal properties of NPCMAF-4-800. These attributes enabled substantial water production throughout entire AWH periods. On the basis of this scheme, the solar-driven AWH application of NPCMAF-4-800 coordinated with a device was tested for 10 cycles to ensure stability during consecutive cycles of water adsorption and desorption. As shown in Fig. 5d, the rate of water production did not show obvious attenuation during 10 cycles, suggesting the outstanding long-term operational stability and adsorption–desorption-reversible properties of NPCMAF-4-800. We compared the water-production rate of NPCMAF-4-800 and other reported state-of-art adsorbents for solar-driven AWH (Fig. 5e and Table S2†). NPCMAF-4-800 achieved the highest water-production rate recorded in a relatively arid condition compared with other solar-driven AWH absorbents reported to date.30,53–63
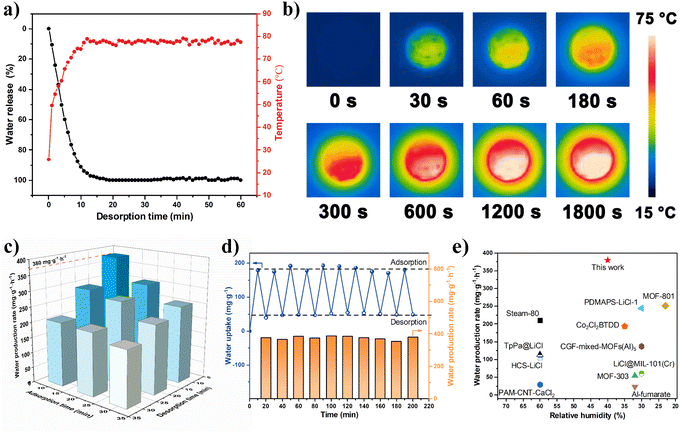 |
| Fig. 5 (a) Time-course of temperature and mass change of saturated adsorbed NPCMAF-4-800 at 40% RH under one-solar irradiation. (b) Time-dependent infrared images of saturated adsorbed NPCMAF-4-800 under one-solar irradiation. (c) Indoor atmosphere water-harvesting of NPCMAF-4-800 at different adsorption and desorption times (adsorption process: 40% RH and 25 °C; desorption process: one-sun illumination). (d) Cycling performance and water-production rate of a solar-driven AWH device based on NPCMAF-4-800. (e) Comparison of the water-production rate and applied humidity with those of representative AWH absorbents. | |
Outdoor AWH demonstration of NPCMAF-4-800
The rapid dynamic AWH performance of NPCMAF-4-800 encouraged us to perform an outdoor field test at the Zhuhai campus of Sun Yat-sen University (SYSU) (22.35° N, 113.59° E) (Section S6†). Specifically, the local ambient air during this period in Zhuhai remained at 30–40% RH and 25 °C. Therefore, a rapid-cycling, solar-driven water-production device was built to validate the practicability of NPCMAF-4-800, which consisted of a vapor condenser, NPCMAF-4-800 absorber in a container, light-transparent cover, supporting plate and water-collecting room (Fig. 6b). To ensure the sorption stage was carried out in the positive direction, we placed the absorber in a dark place for sufficient vapor absorption within 10 min. Then, we moved the AWH device to the outdoor location to perform solar-driven water release and condensation within 10 min to complete one AWH cycle (Fig. S7†). The weather data in the outdoor environment during experimental periods were recorded in real-time (Fig. 6d). The formation, growth and cumulation of droplets on the sidewall within 10 min are shown in Fig. 6a. In the first 2 min, fogging was observed on the window. Subsequently, water vapor accumulated and condensed constantly on the inner walls driven by the temperature difference. After 10 min, many water droplets adhered onto the container wall. Ultimately, the AWH device achieved a cumulative water production of 1.77 L kg−1 during daytime (Fig. 6c). To further reduce the energy cost of the device, a second-generation AWH device was powered by solar energy (Fig. S8 and S9†), which also realized satisfactory water production (Fig. S10†). The actual water production of NPCMAF-4-800 was comparable (or even superior) to the values of reported adsorbents under similar climates, such as MOF-303 (0.29 L kg−1 day−1),50 Ni2Cl2(BTDD) (0.7 L kg−1 day−1),64 CNF/LiCl aerogel (0.63 L kg−1 day−1)65 and Li-SHC (1.09 L kg−1 day−1).66 In addition, the quality of condensate water collected by the outdoor AWH device was checked by ICP-OES by measuring the concentrations of primary metal ion (Fig. S11†). We found that the content of metal ions was below WHO guidelines for drinking-water quality and, thus, suitable for direct potable consumption.67
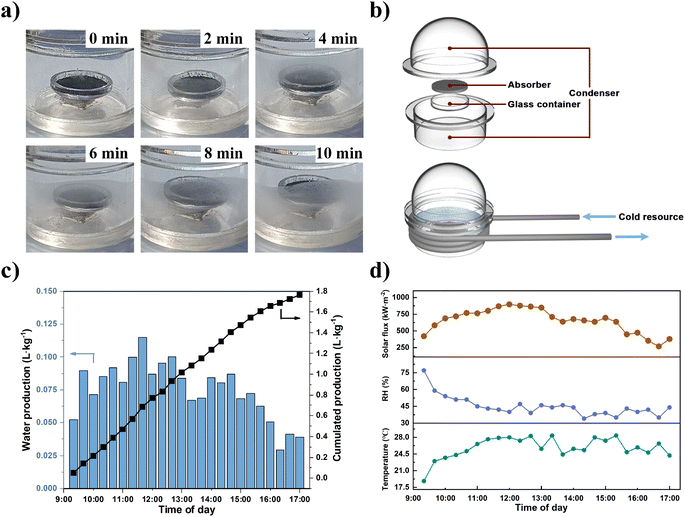 |
| Fig. 6 Performance of outdoor atmosphere water-harvesting and release. (a) Optical image of a device with condensed droplets on inclined walls during water collection. (b) Structure of the AWH device. (c) Freshwater collecting in one daytime via 24 sorption–desorption cycles within 8 h. (d) Environment temperature, humidity and solar flux for outdoor water production during daytime. | |
Conclusions
We demonstrated that MAF-4 derived N-doped nanoporous carbons exhibited high water adsorption at low RH, rapid adsorption–desorption kinetics and exceptional photothermal properties for efficient solar-driven AWH. Benefiting from rich N-doped species, ideal micropore characteristics and excellent photothermal properties, NPCMAF-4-800 achieved an outstanding water-uptake capacity of 306 mg g−1 at 40% RH and an impressive sunlight absorption rate of 97%. Featuring rapid delivery dynamics and high thermal conductivity, a solar-driven AWH device was created. It realized a superior water-production rate of 380 mg g−1 h−1 at 40% RH, which is higher than that of previous benchmarks from other solar-driven AWH absorbents reported so far. Moreover, efficient water production of 1.77 L kg−1 in the daytime under outdoor conditions was realized. This work reveals the great potential of MOF-derived functional porous carbon materials for freshwater production from the atmosphere by a solar thermal process, which can help solve global water scarcity. Considering this practical solar-driven water enrichment/production, future investigations on the improvement of solar-to-thermal conversion efficiency and sorption dynamics, as well as enhancement of the water production rate of the apparatus, are underway.
Data availability
The data that support the findings of this study are available from the corresponding author upon reasonable request.
Author contributions
Jin-Hua Feng conceptualization: equal; data analysis: equal; investigation: lead; validation: equal; writing (original draft): lead; Feng Lu data analysis: equal; investigation: equal; methodology: lead; writing (original draft): equal; Zhen Chen investigation: supporting; validation: supporting; Miao–Miao Jia data analysis: supporting; investigation: supporting; Yi-Le Chen data curation: supporting; Wei-Hai Lin methodology: supporting; Qing-Yun Wu resources: supporting; Yi Li funding acquisition: supporting; investigation: supporting; Ming Xue funding acquisition: equal; investigation: equal; resources: equal; supervision: lead, writing (review and editing): lead; Xiao-Ming Chen funding acquisition: equal; investigation: equal; resources: equal; writing (review and editing): equal.
Conflicts of interest
The authors declare no conflicts of interest.
Acknowledgements
This work was supported by National Natural Science Foundation of China (22090061, 22071076), Department of Science and Technology of Guangdong Province (2021A1515010204, 2022A1515010021), and Science and technology planning project of Zhuhai (2320004000169).
Notes and references
- M. M. Mekonnen and A. Y. Hoekstra, Sci. Adv., 2016, 2, e1500323 CrossRef PubMed
.
- J. Eliasson, Nature, 2015, 517, 6 CrossRef CAS PubMed
.
- M. Ejeian and R. Z. Wang, Joule, 2021, 5, 1678–1703 CrossRef
.
- A. LaPotin, H. Kim, S. R. Rao and E. N. Wang, Acc. Chem. Res., 2019, 52, 1588–1597 CrossRef CAS PubMed
.
- A. R. Parker and C. R. Lawrence, Nature, 2001, 414, 33–34 CrossRef CAS PubMed
.
- M. Muselli, D. Beysens, J. Marcillat, I. Milimouk, T. Nilsson and A. Louche, Atmos. Res., 2002, 64, 297–312 CrossRef CAS
.
- Y. Zheng, H. Bai, Z. Huang, X. Tian, F.-Q. Nie, Y. Zhao, J. Zhai and L. Jiang, Nature, 2010, 463, 640–643 CrossRef CAS PubMed
.
- R. V. Wahlgren, Water Res., 2001, 35, 1–22 CrossRef CAS PubMed
.
- N. Hanikel, M. S. Prévot and O. M. Yaghi, Nat. Nanotechnol., 2020, 15, 348–355 CrossRef CAS PubMed
.
- H. Lu, W. Shi, Y. Guo, W. Guan, C. Lei and G. Yu, Adv. Mater., 2022, 34, 2110079 CrossRef CAS PubMed
.
- Y. Tu, R. Wang, Y. Zhang and J. Wang, Joule, 2018, 2, 1452–1475 CrossRef CAS
.
- T. D. Bennett, F. X. Coudert, S. L. James and A. I. Cooper, Nat. Mater., 2021, 20, 1179–1187 CrossRef CAS PubMed
.
- C. Xiang, F. Deng and R. Wang, Matter, 2022, 5, 2487–2490 CrossRef CAS
.
- F. Fathieh, M. J. Kalmutzki, E. A. Kapustin, P. J. Waller, J. Yang and O. M. Yaghi, Sci. Adv., 2018, 4, eaat3198 CrossRef PubMed
.
- M. R. Manila, S. Mitra and P. Dutta, Appl. Therm. Eng., 2020, 178, 115552 CrossRef CAS
.
- S. G. Wang, R. Z. Wang and X. R. Li, Renewable Energy, 2005, 30, 1425–1441 CrossRef CAS
.
- Y. Huang, Q. Cheng, Z. Wang, S. Liu, C. Zou, J. Guo and X. Guo, Chem. Eng. J., 2020, 398, 125557 CrossRef CAS
.
- H. Furukawa, F. Gándara, Y.-B. Zhang, J. Jiang, W. L. Queen, M. R. Hudson and O. M. Yaghi, J. Am. Chem. Soc., 2014, 136, 4369–4381 CrossRef CAS PubMed
.
- T. Yang, L. Ge, T. Ge, G. Zhan and R. Wang, Adv. Funct. Mater., 2021, 32, 2105267 CrossRef
.
- M. O'Shaughnessy, P. R. Spackman, M. A. Little, L. Catalano, A. James, G. M. Day and A. I. Cooper, Chem. Commun., 2022, 58, 13254–13257 RSC
.
- S. Inagaki, Y. Fukushima, K. Kuroda and K. Kuroda, J. Colloid Interface Sci., 1996, 180, 623–624 CrossRef CAS
.
- P. L. Llewellyn, F. Schueth, Y. Grillet, F. Rouquerol, J. Rouquerol and K. K. Unger, Langmuir, 1995, 11, 574–577 CrossRef CAS
.
- S. Kayal, S. Baichuan and B. B. Saha, Int. J. Heat Mass Transfer, 2016, 92, 1120–1127 CrossRef CAS
.
- J. Canivet, A. Fateeva, Y. Guo, B. Coasne and D. Farrusseng, Chem. Soc. Rev., 2014, 43, 5594–5617 RSC
.
- S. Bagi, A. M. Wright, J. Oppenheim, M. Dincă and Y. Román-Leshkov, ACS Sustain. Chem. Eng., 2021, 9, 3996–4003 CrossRef CAS
.
- W. Xu and O. M. Yaghi, ACS Cent. Sci., 2020, 6, 1348–1354 CrossRef CAS PubMed
.
- M. Yuan, M. Gao, Q. Shi and J. Dong, Chem. Eng. J., 2020, 379, 122412 CrossRef CAS
.
- N. Hanikel, X. Pei, S. Chheda, H. Lyu, W. Jeong, J. Sauer, L. Gagliardi and M. Yaghi Omar, Science, 2021, 374, 454–459 CrossRef CAS PubMed
.
- Z. Zheng, N. Hanikel, H. Lyu and O. M. Yaghi, J. Am. Chem. Soc., 2022, 144, 22669–22675 CrossRef CAS PubMed
.
- J. Xu, T. Li, J. Chao, S. Wu, T. Yan, W. Li, B. Cao and R. Wang, Angew. Chem., Int. Ed., 2020, 59, 5202–5210 CrossRef CAS PubMed
.
- B. Wang, X. Zhou, Z. Guo and W. Liu, Nano Today, 2021, 40, 101283 CrossRef CAS
.
- M. Xia, D. Cai, J. Feng, P. Zhao, J. Li, R. Lv, G. Li, L. Yan, W. Huang, Y. Li, Z. Sui, M. Li, H. Wu, Y. Shen, J. Xiao, D. Wang and Q. Chen, Adv. Funct. Mater., 2023, 33, 2214813 CrossRef CAS
.
- Y. Hu, Z. Fang, X. Ma, X. Wan, S. Wang, S. Fan, Z. Ye and X. Peng, Appl. Mater. Today, 2021, 23, 101076 CrossRef
.
- H.-L. Jiang, B. Liu, Y.-Q. Lan, K. Kuratani, T. Akita, H. Shioyama, F. Zong and Q. Xu, J. Am. Chem. Soc., 2011, 133, 11854–11857 CrossRef CAS PubMed
.
- X.-C. Huang, Y.-Y. Lin, J.-P. Zhang and X.-M. Chen, Angew. Chem., Int. Ed., 2006, 45, 1557–1559 CrossRef CAS
.
- K. S. Park, Z. Ni, A. P. Côté, J. Y. Choi, R. Huang, F. J. Uribe-Romo, H. K. Chae, M. O'Keeffe and O. M. Yaghi, Proc. Natl. Acad. Sci. U.S.A., 2006, 103, 10186–10191 CrossRef CAS PubMed
.
- J.-P. Zhang, Y.-B. Zhang, J.-B. Lin and X.-M. Chen, Chem. Rev., 2012, 112, 1001–1033 CrossRef CAS
.
- L. L. Zhuo, P. Chen, K. Zheng, X. W. Zhang, J. X. Wu, D. Y. Lin, S. Y. Liu, Z. S. Wang, J. Y. Liu, D. D. Zhou and J. P. Zhang, Angew. Chem., Int. Ed., 2022, 61, e202204967 CrossRef CAS PubMed
.
- Y. C. Tang, Z. S. Wang, H. Yi, M. Y. Zhou, D. D. Zhou, J. P. Zhang and X. M. Chen, Angew. Chem., Int. Ed., 2023, 62, e202303374 CrossRef CAS PubMed
.
- Z. Y. Sui, Y. N. Meng, P. W. Xiao, Z. Q. Zhao, Z. X. Wei and B. H. Han, ACS Appl. Mater. Interfaces, 2015, 7, 1431–1438 CrossRef CAS PubMed
.
- X. Li, C. Hao, B. Tang, Y. Wang, M. Liu, Y. Wang, Y. Zhu, C. Lu and Z. Tang, Nanoscale, 2017, 9, 2178–2187 RSC
.
- G.-P. Hao, F. Han, D.-C. Guo, R.-J. Fan, G. Xiong, W.-C. Li and A.-H. Lu, J. Phys. Chem. C, 2012, 116, 10303–10311 CrossRef CAS
.
- A. Li, Y. Tong, H. Song and X. Chen, J. Phys. Chem. C, 2018, 122, 17278–17286 CrossRef CAS
.
- S. Liu, J. Zhou and H. Song, Small, 2018, 14, 1703548 CrossRef PubMed
.
- H.-J. Wang, A. Kleinhammes, T. P. McNicholas, J. Liu and Y. Wu, J. Phys. Chem. C, 2014, 118, 8474–8480 CrossRef CAS
.
- T. Matsuoka, H. Hatori, M. Kodama, J. Yamashita and N. Miyajima, Carbon, 2004, 42, 2346–2349 CrossRef CAS
.
- V. A. Cardozo-Mata, J. A. Pescador-Rojas, A. Hernández-Hernández, L. A. Hernández-Hernández, A. Miralrio, F. J. Martínez-Farías, E. Vallejo-Castañeda and E. Rangel, Appl. Surf. Sci., 2020, 502, 144149 CrossRef CAS
.
- T. Horikawa, N. Sakao, T. Sekida, J. i. Hayashi, D. D. Do and M. Katoh, Carbon, 2012, 50, 1833–1842 CrossRef CAS
.
- G. Yilmaz, F. L. Meng, W. Lu, J. Abed, C. K. N. Peh, M. Gao, E. H. Sargent and G. W. Ho, Sci. Adv., 2020, 6, eabc8605 CrossRef CAS PubMed
.
- W. Song, Z. Zheng, A. H. Alawadhi and O. M. Yaghi, Nat. Water., 2023, 1, 626–634 CrossRef
.
- X. Wang, X. Li, G. Liu, J. Li, X. Hu, N. Xu, W. Zhao, B. Zhu and J. Zhu, Angew. Chem., Int. Ed., 2019, 58, 12054–12058 CrossRef CAS
.
- H. Yao, P. Zhang, Y. Huang, H. Cheng, C. Li and L. Qu, Adv. Mater., 2020, 32, e1905875 CrossRef PubMed
.
- Y. Song, N. Xu, G. Liu, H. Qi, W. Zhao, B. Zhu, L. Zhou and J. Zhu, Nat. Nanotechnol., 2022, 17, 857–863 CrossRef CAS PubMed
.
- R. Li, Y. Shi, M. Wu, S. Hong and P. Wang, Nano Energy, 2020, 67, 104255 CrossRef CAS
.
- R. Li, Y. Shi, M. Alsaedi, M. Wu, L. Shi and P. Wang, Environ. Sci. Technol., 2018, 52, 11367–11377 CrossRef CAS PubMed
.
- Y. Wang, W. Chen, J. Fu and Y. Liu, eScience, 2023, 3, 100154 CrossRef
.
- C. Lei, Y. Guo, W. Guan, H. Lu, W. Shi and G. Yu, Angew. Chem., Int. Ed., 2022, 61, e202200271 CrossRef CAS PubMed
.
- H. Kim, S. Yang, S. R. Rao, S. Narayanan, E. A. Kapustin, H. Furukawa, A. S. Umans, O. M. Yaghi and E. N. Wang, Science, 2017, 356, 430–434 CrossRef CAS PubMed
.
- H. A. Almassad, R. I. Abaza, L. Siwwan, B. Al-Maythalony and K. E. Cordova, Nat. Commun., 2022, 13, 4873 CrossRef CAS PubMed
.
- H. Kim, S. R. Rao, E. A. Kapustin, L. Zhao, S. Yang, O. M. Yaghi and E. N. Wang, Nat. Commun., 2018, 9, 1191 CrossRef PubMed
.
- A. J. Rieth, S. Yang, E. N. Wang and M. Dinca, ACS Cent. Sci., 2017, 3, 668–672 CrossRef CAS PubMed
.
- F. Luo, T. Liao, X. Liang, W. Chen, S. Wang, X. Gao, Z. Zhang and Y. Fang, J. Cleaner Prod., 2022, 373, 133838 CrossRef CAS
.
- N. Hanikel, M. S. Prévot, F. Fathieh, E. A. Kapustin, H. Lyu, H. Wang, N. J. Diercks, T. G. Glover and O. M. Yaghi, ACS Cent. Sci., 2019, 5, 1699–1706 CrossRef CAS PubMed
.
- Z. Shao, Z.-S. Wang, H. Lv, Y.-C. Tang, H. Wang, S. Du, R. Sun, X. Feng, P. Poredoš, D.-D. Zhou, J.-P. Zhang and R. Wang, Appl. Phys. Rev., 2023, 10, 041409 CAS
.
- P. Zhu, Z. Yu, H. Sun, D. Zheng, Y. Zheng, Y. Qian, Y. Wei, J. Lee, S. Srebnik, W. Chen, G. Chen and F. Jiang, Adv. Mater., 2023, 36, e2306653 CrossRef PubMed
.
- H. Shan, C. Li, Z. Chen, W. Ying, P. Poredos, Z. Ye, Q. Pan, J. Wang and R. Wang, Nat. Commun., 2022, 13, 5406 CrossRef CAS PubMed
.
-
World Health Organization, Guidelines for Drinking-Water Quality, 4th edn incorporating the 1st addendum, 2017 Search PubMed
.
Footnotes |
† Electronic supplementary information (ESI) available: The SEM image and N2 adsorption–desorption isotherm, the elemental analysis, the calculation formula of the water adsorption/desorption kinetic; the optical images and operation steps of solar-driven water desorption experiment in the lab environment; the actual operation of indoor AWH device; the demonstration of outdoor atmosphere water harvesting; the water quality analysis by ICP-OES. See DOI: https://doi.org/10.1039/d4sc01802h |
‡ These authors contributed equally to this work. |
|
This journal is © The Royal Society of Chemistry 2024 |
Click here to see how this site uses Cookies. View our privacy policy here.