High-performance TiO2 catalyst composited with In–1,1,2,2-tetra(4-carboxylbiphenyl)ethylene for the efficient degradation of organic pollutants†
Received
13th November 2023
, Accepted 25th January 2024
First published on 30th January 2024
Abstract
A combination of metal–organic frameworks (MOFs) with TiO2 can improve the performance of TiO2 in the photocatalytic degradation of organic pollutants. The selection of organic ligands for MOF preparation is a key factor for designing MOF–TiO2 composites with excellent photocatalytic properties. However, the photocatalytic performance of such composites has rarely been examined. In this study, a series of TiO2 nanoflowers (NFs) modified with In-based MOFs were prepared via a hydrothermal method using 1,1,2,2-tetra(4-carboxylbiphenyl)ethylene (TCBPE) as the organic ligand. The fabricated TiO2@In–TCBPE (TIT) heterojunctions exhibited enhanced light absorption, charge separation, and electron transfer characteristics owing to the transport of ·O2− free radicals through the charge transfer pathway between heterostructures. Rhodamine B (RhB) was chosen as a typical representative of organic pollutants under simulated sunlight and is used to evaluate the photocatalytic performance of TIT composites. The optimized TIT composite exhibited a strong photocatalytic effect, and the complete degradation of RhB was achieved in 13 min. In addition, the prepared TIT composite demonstrated excellent photocatalytic activity during the degradation of organic pollutants in different aqueous environments (tap water, lake water, and river water). This study provides a reference for the selection of ligands for the subsequent construction of high-performance MOF photocatalysts.
Environmental significance
As a common water pollutant, rhodamine B (RhB) may reduce water transparency and have toxic effects on the growth and reproduction of aquatic organisms. At medium and high concentrations, it causes toxic reactions and some genotoxicity in the human body. In this work, RhB is chosen as a typical representative of organic pollutants to evaluate the photocatalytic performance of In-based MOFs compounded with TiO2 under visible light, and the results illustrate that the degradation effect of the material is efficient and stable under a variety of complex environments.
|
1. Introduction
The rapid economic growth is associated with numerous pollution problems. As a focus of environmental pollution, water pollution has become a hot research topic. Dyes (macromolecular organics), which are necessary for industrial production, are widely used in the textile, pharmaceutical, and food industries, and wastewater is discharged, polluting the environment.1,2 The textile industry accounts for more than half of the overall water pollution, which poses a serious threat to human lives. Therefore, the development of efficient and environmentally friendly degradation techniques for the removal of organic matter from water is urgently required.
In recent years, photocatalytic technology has attracted considerable attention for the treatment of pollutants owing to its environmental friendliness, low cost, and high efficiency.3–5 TiO2, the first reported semiconductor photocatalytic material, has been widely used in the field of photocatalysis because of its low cost, non-polluting nature, and easy accessibility.6–8 Meanwhile, changing the sample morphology to increase the number of active sites on the photocatalyst surface is an effective way of improving the photocatalytic performance of the material, resulting in the synthesis of various types of TiO2 with different morphologies, such as floral, columnar, spherical, and tubular ones.9–12 Flower-like TiO2 structures have attracted considerable attention from researchers owing to their large specific surface areas and excellent catalytic activities.13 However, TiO2 suffers from the low adsorption capacity and solar energy conversion efficiency. Currently, combinations of TiO2 with other materials, such as noble metals, ions, and composite semiconductors, are commonly used to enhance its photocatalytic performance by constructing heterogeneous structures.14,15 For example, Faupel et al. loaded Au onto the TiO2 surface to significantly enhance the light absorption of TiO2 in the ultraviolet (UV) region and achieve a higher photocatalytic efficiency, while Chen et al. modified the original energy band structure of S-doped TiO2 to enhance its photo absorption under visible light irradiation.16–18 Zou et al. used WO3 and TiO2 to construct Z-type semiconductor heterostructures to enhance visible light absorption and the transfer of photogenerated electron–hole pairs.19 Wei et al. designed C-doped TiO2 and metal organic framework (MOF)-derived ZnO to prepare composites with increased specific surface areas and active sites to promote the synergistic effect of the heterojunctions between the materials.20 MOFs are considered a new type of photocatalysts suitable for pollutant degradation.21 They represent crystalline porous materials composed of metal ions serving as the central nodes and organic ligands arranged according to certain rules.22 MOFs have distinct advantages: their unique framework structure endows the material with a high specific surface area and large number of active sites, while their light absorption range can be adjusted by organic molecules to regulate the gap between the highest occupied and lowest unoccupied molecular orbitals, thus creating a suitable bandgap structure.23–25 However, single-MOF photocatalysts suffer from high photogenerated carrier complexation rates and poor electrical conductivities.26,27 To overcome these limitations, researchers usually construct heterostructures by combining semiconductors and MOFs materials to accelerate electron transfer through the synergistic effect between the materials, while inter-electronic interactions lead to surface electronic modulation enhancing the photocatalytic activity of the catalysts.28,29
Compared with traditional transition and rare-earth metal MOFs, group IIIA MOFs have been studied less intensively, and In has attracted considerable attention in the field of photoelectrochemistry because of its unique network topology.30,31 In ions in In-based MOFs can be paired with other ligands to form various coordination structures that exhibit excellent gas absorption, fluorescence, light absorption, and ion exchange properties.32,33 In-based MOFs also have a large near-UV absorption region, which can enhance the light-absorption capability of materials under visible light irradiation.34
1,1,2,2-Tetra(4-carboxylbiphenyl)ethylene (TCBPE) is a tetraphenylethylene derivative. Its introduction into the framework of organometallic compounds helps limit the rotation and vibration of organic groups through hydrogen bonding, π–π stacking, and intermolecular van der Waals forces, thereby increasing the quantum yield of the material.35–37 However, the photocatalytic properties of TCBPE–MOFs and photocatalytic applications of In-based MOFs have not been examined in detail.
In this study, TiO2 with a flower-like structure via a solvothermal method were synthesized and then In-based MOFs were in situ grown on the surface of TiO2 through a hydrothermal synthesis to obtain a new Z-type hetero-structured photocatalyst TiO2@In–TCBPE (TIT) with TiO2 as substrate. Traditional photocatalytic tests were conducted to evaluate the photocatalytic performance of the prepared TIT composites, and rhodamine B (RhB) was selected as a representative organic pollutant. Multiple path analysis was used to investigate the structure, elemental composition and photoelectric properties of the composites. The composition of the radicals generated in the photocatalytic reaction was determined, and the Z-type heterostructure and charge separation transfer process were analyzed to systematically elucidate the photocatalytic reaction mechanism of the composites.
2. Experimental section
2.1 Materials
Tetrabutyl titanate (4C16H36O4Ti4 99%) was purchased from Shanghai Haohong Biomedical Technology Co. Glycerol (99.5%) and indium trichloride tetrahydrate (InCl3·4H2O, 99%) were procured from Macklin. Acetic acid (HAc, 99.5%) was acquired from Boer. TCBPE (97%) was purchased from Alpha Chemicals, Ltd. N,N-Dimethylformamide (DMF, AR) and ethanol (AR) were obtained from Aladdin and GHTECH, respectively. Rhodamine B (RhB, AR) was purchased from Aladdin. All materials were used without further purification.
2.2 Preparation of photocatalysts
2.2.1 Preparation of TiO2 nanoflowers.
Glycerol acid peptide was prepared via a solvent heat reaction. Briefly, 10 mL of glycerol and 30 mL of ethanol were stirred on a stirrer for 1 h. Next, 1 g of tetrabutyl titanate was added to the reaction mixture and stirred for 5 min; the obtained solution was transferred to a 50 mL reactor, and the reaction was conducted for 24 h at a temperature of 180 °C. The collected white powder was washed 4 times with anhydrous ethanol and placed in a vacuum oven to dry at 60 °C for 12 h. The powder was calcined in air at a heating rate of 5 °C min−1 and temperature of 480 °C for 90 min to obtain flower-like TiO2. In this reaction, tetrabutyl titanate reacted with glycerol to form glycerol acid peptide, which was calcined to remove carbon and hydrogen atoms.38
2.2.2 Preparation of In–TCBPE.
First, 117 mg of InCl3·4H2O and 32 mg of TCBPE were dispersed in a solution consisting of 20 mL DMF, 5 mL HAc, and 1 mL H2O at room temperature via ultrasonication for 5 min. Subsequently, the reaction suspension was stirred in an oil bath at 90 °C for 6 h. The resulting In–TCBPE samples were washed several times with water and ethanol, and dried in a vacuum oven at 60 °C for 10 h.
2.2.3 Preparation of TIT.
To synthesize TIT composites, a fixed amount of TiO2 (0.4 g) was sonicated in 20 mL of DMF for 20 min, and the resulting system was denoted as suspension A. A proportion of InCl3·4H2O and TCBPE was sonicated for 10 min in 20 ml DMF, then 10 ml HAc and 2 ml H2O were added dropwise and stirred well to give solution B. Suspension A and solution B were mixed and stirred for 30 min to give suspension C, which was placed in an oil bath at 90 °C for 6 h. The TiO2 content in the composites was controlled by adjusting the In–TCBPE synthesis ratio. Herein, In–TCBPE is referred to as IT and the produced composite is denoted as TIT-X, where an increase in X (X = 1, 2, 3, and 4) represents an increase in the In–TCBPE content (from 5 to 30 wt%).
2.3 Characterization techniques
The morphologies and elemental compositions of the fabricated composites were determined using a JSM-7800F scanning electron microscope (SEM). X-ray diffraction (XRD) was performed at angles between 5° and 90° using an X'Pert PRO MPD instrument. X-ray photoelectron spectroscopy (XPS) was conducted using a axis Ultra DLD Kratos AXIS SUPRA instrument. The loading of In and the content of Ti in different samples were determined by inductively coupled plasma-optical emission spectrometer (ICP-OES) on Agilent 5110. Photoluminescence (PL) spectra were recorded on a 970CRT fluorescence spectrophotometer. UV-visible absorption/diffuse reflectance spectra of the synthesized materials were recorded using a PE Lambda 750 instrument, and electrochemical impedance analyses and the Mott–Schottky experiments were carried out on a CHI760E electrochemical work station. DMPO and TEMPO were used to discover radicals using electron paramagnetic resonance ((EPR) (Bruker EMXplus-6/1)). Shimadzu UV-2700i UV-vis spectrophotometer was used to characterize organic pollutants.
2.4 Photocatalytic activity experiments
The synthesized composites were used as photocatalysts for the photodegradation of organic pollutants under simulated sunlight with RhB representing a typical organic pollutant. The utilized setup included a 300 W xenon lamp light source (CME-Xe300F), matching reaction chamber, 200 mL double-layer beaker, 6 L low-temperature thermostat (SN-DHC-0506), and magnetic stirrer (Fig. S1†). The double-layer beaker and cryostat were used to maintain a constant temperature during the photocatalytic reaction.
Photocatalysis experiments were carried out under a 20 A xenon lamp. Distance between reactor and xenon lamp is 10 cm, and the reactor temperature was maintained at 30 °C. First, 40 mg of catalyst was added to 200 mL of RhB solution at a concentration of 10 ppm. The material was stirred for 20 minutes under light avoidance conditions to reach an adsorption–desorption equilibrium. Afterwards, the xenon lamp light source was switched on, and the mixture was stirred continuously. For each sample, 2 mL of reaction solution was taken at the same time interval. The solution was filtered through 0.22 μm syringe filter, and its photocatalytic efficiency was examined at a wavelength of 554 nm.
3. Results and discussion
3.1 Composite characterization
The crystallinity and crystal surfaces of TiO2 and the TIT composites were analyzed via XRD. The XRD pattern of TiO2 contains multiple sharp diffraction peaks (Fig. 1A). Here, the diffraction peaks of the prepared TiO2 at 25.3°, 37.8°, 48.0°, 53.9°, and 55.0° correspond to the (101), (004), (200), (105), and (211) planes of anatase-type TiO2, which is in agreement with the previously reported.39 The XRD patterns of the synthesized TIT-X composites include the characteristic TiO2 peaks, indicating that the crystal structure of TiO2 was preserved after coating it with the In-based MOFs. No distinct characteristic peaks of In–TCBPE are present in the TIT-X patterns, which may be due to the absence of a distinct crystal structure and low doping degree of the In-based MOFs (Fig. S2†). The presence of the In-based MOFs in the complexes is confirmed by SEM and XPS below.
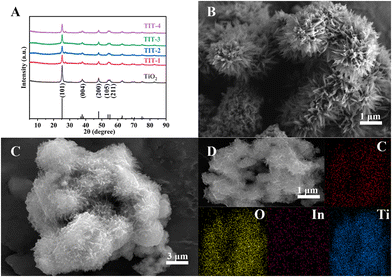 |
| Fig. 1 (A) XRD patterns of TiO2, TIT-1, TIT-2, TIT-3, and TIT-4. SEM images of (B) TiO2 and (C) TIT-3. (D) Elemental mapping images of the TIT-3 composite. | |
The structures and morphologies of the synthesized TiO2 and TIT-3 composites were characterized via SEM. According to Fig. 1B, the synthesized TiO2 exhibits a well-defined flower-like structure with a diameter of approximately 1.5 μm and thickness of 20 nm. During heat treatment, the carbon and hydrogen atoms bound to the TiO2 surface were removed, resulting in the combination of excess oxygen and titanium atoms and formation of multiple Ti vacancies and pore structures. Therefore, the synthesized MOF material was likely attached to the TiO2 pore surface. The composite morphologies are shown in Fig. 1C, where the outline of the TiO2 nanoflower becomes blurred, and a material layer is encapsulated between the petals. The four elements of the synthesized TIT-3 are uniformly distributed in the composite core (Fig. 1D) with overlapping regions of C, In, and Ti atoms, which confirm a well-dispersed growth of the In-based MOF around the TiO2 core.40
To investigate the elemental compositions and chemical states of the prepared photocatalysts, the synthesized TIT-3 composite was analyzed via XPS. As shown in Fig. 2A, the full spectrum of TIT-3 contains the C 1s, O 1s, Ti 2p, and In 3d, respectively. It can be assumed that Ti is derived from TiO2, while C and In originate from the In-based MOF. As shown in Fig. 2B, the C 1s XPS profile of TIT-3 exhibits three peaks at 284.88, 286.38, and 288.91 eV corresponding to C–C, C–O, and C
O–O species, respectively.41 The binding energies of the Ti 2p XPS peaks of the TIT-3 composite are 458.68 and 464.48 eV, indicating the presence of Ti 2p3/2 and Ti 2p1/2 species.
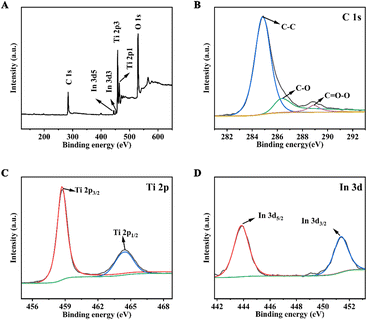 |
| Fig. 2 (A) XPS survey spectrum of TIT-3. High-resolution (B) C 1s, (C) Ti 2p, and (D) In 3d XPS profiles of the TIT-3 composite. | |
The binding energy peaks of In 3d are 443.88 and 451.38 eV, which correspond to In 3d5/2 and In 3d3/2, respectively. The presence of the Ti 2p and In 3d regions in the TIT-3 heterostructure (Fig. 2C and D) confirms the simultaneous presence of TiO2 and In-based MOF in the TIT-3 complex.42 In order to be able to understand the amount of both In and Ti in the catalyst. We analyzed the material by ICP-OES. The exact contents of In in TIT-1, TIT-2, TIT-3 and TIT-4 were characterized to be 0.164 wt%, 0.228 wt%, 0.235 wt% and 0.315 wt%, respectively. The amounts of Ti were 44.069 wt%, 44.468 wt%, 40.086 wt% and 42.843 wt%, respectively. Therefore, the In/Ti are corresponded to 0.37%, 0.51%, 0.59% and 0.73% respectively.
In order to gain a deeper understanding of the mechanism of the TIT-X composites in the degradation process of organic pollutants, the synthesized composites were examined via UV-vis diffuse reflectance absorption spectroscopy. TiO2 exhibits significant light absorption in the UV region, while In–TCBPE absorbs light from the UV to visible light region (Fig. 3A). Compared with pure TiO2, the TIT complexes exhibit the characteristic absorption peaks of TiO2 and In–TCBPE, further demonstrating their successful complexation. With increasing In-based MOF content, the light absorption intensity between 350 and 500 nm significantly increases, indicating that the introduction of In–TCBPE effectively promotes the absorption of visible light. In contrast, the UV part of the TIT-4 absorption spectrum is considerably reduced, which is due to the excessive In–TCBPE coating on the TiO2 surface masking the UV absorption sites of the original TIO2. Bandgap values were calculated using Tauc's equation: the bandgap of TiO2 was 3.21 eV (Fig. 3B), and the bandgaps of TIT-1, TIT-2, TIT-3, and TIT-4 were 3.17, 3.13, 3.09, and 2.96 eV, respectively. The bandgap of In–TCBPE was 2.75 eV. These results indicate that the composites of TiO2 and In–TCBPE were characterized by the significantly narrowed bandgaps and produced more photogenerated charges at the same energy.
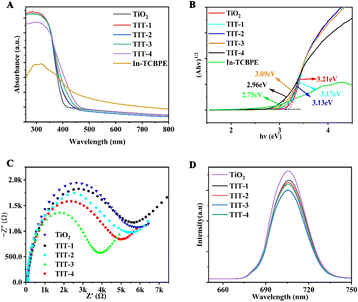 |
| Fig. 3 (A) UV-visible diffuse reflectance absorption spectra, and (B) Tauc plots of TiO2, TIT-1, TIT-2, TIT-3, TIT-4, and In–TCBPE. (C) Electrochemical impedance spectra, and (D) PL emission spectra of TiO2, TIT-1, TIT-2, TIT-3, and TIT-4. | |
Electrochemical impedance experiments were performed to further investigate the electrochemical properties of the studied composites. According to Fig. 3C, TiO2 exhibits the highest electrochemical impedance, and the TIT-3 composite demonstrates the lowest electrochemical impedance as compared with that of the single-component catalyst. After increasing the In MOF loading four times, the charge separation efficiency of the photocatalysts decreased because of the reduction in the number of active sites. This indicates that with an appropriate amount of In–TCBPE, the constructed heterojunction can improve the electron separation efficiency.43
The degradation efficiency of a catalyst is thought to be limited by the complexation of photogenerated electron–hole pairs. Therefore, PL spectroscopy was performed to determine the surface structure and charge-carrier transitions within the composites. Fig. 3D shows the PL emission spectra of the TIT heterostructures excited at a wavelength of 550 nm and room temperature. The luminescence spectrum of TiO2 contains a strong emission peak at 705 nm. After the In-based MOF addition, the TIT emission peak was similar to that of TiO2; however, the intensity of the TIT complex was weaker than that of TiO2, and the PL intensities of the studied samples decreased in the order TiO2, TIT-1, TIT-2, TIT-4, and TIT-3.
A higher PL intensity corresponds to the faster recombination of point charge carriers. The observed decrease in the PL intensity indicates the lower rate of the photogenerated electron–hole complexation and long carrier lifetime in the TIT-3 composite. This is due to the formation of a heterostructure between TiO2 and In–TCBPE in TIT-3, which enhances the effective separation of photogenerated carriers and thus improves the photocatalytic activity of the material, as confirmed by the results of electrochemical impedance measurements. This heterostructure was constructed by loading TiO2 with an appropriate amount of In–TCBPE, which increased the separation efficiency of photogenerated carriers.
In addition, the energy band boundaries of the materials are closely related to their photocatalytic performance. To investigate the detailed energy band structures of TiO2 and In–TCBPE, Mott–Schottky tests were performed (Fig. S3†). The positive slopes of the obtained curves indicate that TiO2 is a typical n-type semiconductor, and the conduction band (CB) positions calculated versus the normal hydrogen electrode (NHE) at different frequencies are −0.21 eV for TiO2 and 1.17 eV for In–TCBPE. According to the equation E (SCE) = E (NHE) −0.24, the calculated CB position of TiO2versus the saturated calomel electrode (SCE) is −0.45 eV, and the corresponding CB position of In–TCBPE is 0.93 eV.44
3.2 Photocatalytic degradation of RhB in aqueous solution
To investigate the photocatalytic degradation performance of the prepared samples, a representative organic pollutant, RhB, was selected for a series of RhB degradation experiments conducted at 10 ppm in aqueous solutions. First, the materials were stirred prior to the photocatalytic reaction, and the C/C0 ratio was used to calculate the degradation efficiency of the photocatalysts, where C0 was the initial concentration of the model pollutant, and C was the residual concentration at irradiation time t, which was determined by measuring the maximum absorption intensity. During the photocatalytic degradation process, the UV-vis absorption peak of RhB was centered at 554 nm. The different materials were subjected to adsorption experiments in the dark (Fig. S4†), and all catalysts reached the adsorption equilibrium within 20 min. The dark adsorption efficiency of the synthesized TIT-3 catalyst was relatively high, indicating that the specific surface area of the composites increased after coating with In–TCBPE.
As shown in Fig. 4A, all the samples reached adsorption equilibrium within 20 min in the dark chamber, and then photocatalytic degradation was carried out under visible light. RhB was very stable under visible light irradiation, and its self-degradation was negligible.
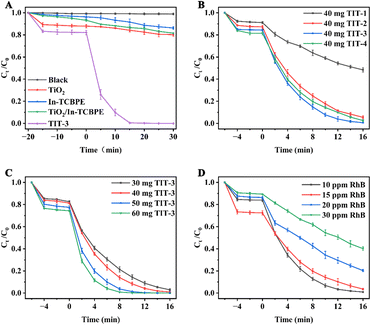 |
| Fig. 4 (A) Photocatalytic removal of 10 ppm RhB under visible light irradiation. Influences of the (B) TiO2 deposition volume, (C) photocatalyst dosage, and (D) RhB concentration on the photocatalytic process. | |
After 30 min, the degradation rate of TiO2 was 20%, while the degradation rate of In–TCBPE was 14.5%. This indicates that many reactive radicals cannot be generated in a single material to remove organic pollutants. The lower degradation rate of In–TCBPE was attributed to the insufficient adsorption of RhB by the material and rapid complexation of photogenerated carriers. The photocatalytic efficiency of TIT-3 was significantly improved with the complete degradation of RhB achieved in 13 min. The composite of In–TCBPE and TiO2 exhibited more intense absorption of visible light owing to the larger number of active sites. The TIT-3 composite catalyst can effectively separate photogenerated electron–hole pairs compared to a single catalyst. Thus, the constructed heterojunction promoted electron transport at the material interface.
3.3 Effects of different reaction conditions
Follow-up experiments were performed to establish the optimum degradation conditions (In–TCBPE loading, photocatalyst amount, and RhB concentration) for the studied materials.
The amount of In–TCBPE loaded onto the TiO2 surface significantly affected the photocatalytic performance of the composites. As shown in Fig. 4B, the RhB degradation rates of TIT-1, TIT-2, TIT-3, and TIT-4 reach 49%, 93%, 98%, and 95%, respectively, within 14 min. With increasing In–TCBPE content, the TIT composites enhanced the absorption of visible light and provided more active sites. However, the further increase in the In–TCBPE loading led to the overcoating of the TiO2 surface, which in turn decreased the photocatalytic efficiency. On the one hand, the higher In–TCBPE loading suppressed the absorption of light in the UV region, decreasing the photocatalytic energy. On the other hand, the active sites on the material surface were shielded, and the electrochemical impedance of the material increased; as a result, the photogenerated carriers were unable to undergo effective separation and complexation. Therefore, TIT-3 was selected for the subsequent experiments.
One of the factors that also has a significant effect on the degradation efficiency of organic pollutants is the amount of photocatalyst, which was chosen to be 30, 40, 50 and 60 mg for the photocatalytic experiments. Fig. 4C shows that the photocatalytic degradation rate increased significantly with increasing catalyst content. At a catalyst amount of 30 mg, the removal rate of RhB was 83% in 10 min, whereas the removal efficiency reached 100% with 60 mg of the catalyst. With an increase in the catalyst dosage, the number of active sites provided by the material continuously increased, which in turn promoted the generation of electron–hole pairs for the degradation of RhB. Based on the obtained results, 40 mg was selected as the standard catalyst dosage for the subsequent experiments in terms of the material efficiency and economic considerations.
The RhB concentration is another important factor affecting the photocatalytic efficiency (Fig. 4D). The reaction dark adsorption time remained essentially unchanged and the photodegradation reaction time increased with increasing pollutant concentration. When the RhB concentration was increased from 10 ppm to 30 ppm, the degradation efficiency of the photocatalyst decreased from 100% to 60% within 16 minutes, indicating that the TIT-3 composite still has excellent activity at high concentrations.
3.4 Effects of solution pH and aqueous matrix
In this section, optimal degradation conditions were established for the TIT-3 composite. In general, the use of photocatalysts in practical applications requires complex conditions. To determine the catalytic efficiencies of the prepared photocatalysts under practical conditions, environmental interferences at different pH values and water qualities were investigated.
The pH of RhB industrial wastewater fluctuates constantly; therefore, the degradation of materials must be studied under different pH conditions. As shown in Fig. 5A, the RhB degradation rates at different initial pH values (3.0, 5.0, 7.0, 9.0, and 11.0) are equal to 97.3%, 98.2%, 98.0%, 98.9%, and 72.4%, respectively. These results revealed that the TIT-3 complex exhibited excellent catalytic performance in a wide pH range of 3.0–9.0 and that its degradation efficiency varied insignificantly with pH. However, when the pH was further increased from 9.0 to 11.0, the degradation efficiency significantly decreased owing to the mutual repulsion between the photocatalyst and RhB dye caused by the excess of OH− ions on the catalyst surface. As a result, the dye was not effectively adsorbed onto the photocatalyst surface, which decreased the photocatalytic efficiency.45
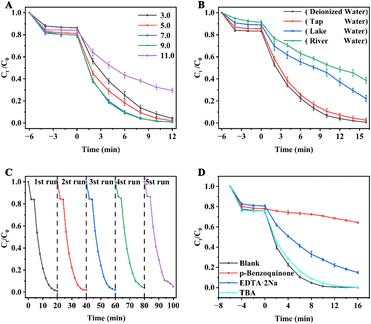 |
| Fig. 5 Influences of the (A) solution pH on the photocatalytic process and (B) aqueous matrix on the degradation of RhB in the TIT-3 system. (C) Results of the catalyst reusability studies. (D) Reactive species trapping by TIT-3 for RhB degradation. | |
The photocatalytic degradation of RhB was also investigated in four commonly used aqueous matrices. Lake water samples were collected from Fuxian Lake in Kunming, and river water samples were collected from Laoyu River in Chenggong, Kunming. According to Fig. 5B, the removal rate of BPA from tap water is 96.3%, which is close to that from deionized water. The low concentration of natural organic matter in tap water and its overall acidity partially affected the degradation of RhB. In contrast, the lower degradation efficiencies of 79.7% and 62.4% were obtained for the lake and river water, respectively. This is due to the fact that the water samples contain a high level of dissolved natural organic matter and co-existing ions, which consume a certain amount of active free radicals. In addition, the presence of tiny insoluble particles in the solution reduces the intensity of the light shone on the surface of the catalyst, hindering the activation of the photocatalysts.
3.5 Reusability and stability of the TIT-3 catalyst
Cycling stability is another important catalyst performance indicator. Therefore, experiments were conducted on the TIT-3 sample with the highest catalytic activity to investigate its cycling stability and reusability. After each cycle, residual RhB was removed from the TIT-3 material with a solution containing deionized water and ethanol and dried in a vacuum oven for reuse. Fig. 5C shows that after five catalytic cycles, the RhB removal rate from the material is approximately 95%, which is only 5% lower than the initial catalytic efficiency, indicating that the TIT-3 composite is a stable and reusable high-efficiency catalyst.
3.6 RhB degradation mechanism
To elucidate the photocatalytic mechanism of the prepared catalysts, the TIT-3 material with the highest catalytic activity was used. In the heterogeneous photocatalytic reaction process, three active species—superoxide radicals (·O2−), hydroxyl radicals (·OH), and holes (h+)—are primarily involved. EPR was used to check the nature and presence of free radicals in the reaction. In Fig. S5A,† after 5 min of exposure to light, the reaction between 2,2,6,6-tetramethylpiperidinyl-1-oxide (TEMPO) and the generated h+ and thus the characteristic peaks decrease, demonstrating the generation of h+. Similarly, 5,5-dimethyl-1-pyrroline N-oxide (DMPO) was used to detect the presence of ·OH and ·O2− in the reaction. At 0 min of light irradiation, there was no obvious characteristic peak in the graph, and when the light was irradiated for 5 min, the characteristic signals corresponding to DMPO·OH and DMOP·O2− could be clearly observed (Fig. S5B and C†). The continuous production of ·OH and ·O2− in the reaction solution under incident light irradiation was further demonstrated. Further investigation of the reaction mechanism and the role of free radicals in the photocatalytic process. Therefore, tert-butanol (TBA), p-benzoquinone (BQ) and disodium acetate dihydrate (EDTA-2NA) were used as ·OH, ·O2− and h+ capturing agents, respectively.46–48 The obtained results (Fig. 5D) indicate that the addition of TBA produced no apparent effect on the degradation rate, suggesting that ·OH plays a minor role in the degradation reaction. The addition of BQ strongly inhibited the photocatalytic degradation, indicating that ·O2− may be the main free radical in the reaction. When EDTA-2Na was used, the RhB degradation rate was 78% of the initial value, suggesting the involvement of h+ in the photocatalytic degradation reaction.49
It is well known that the energy band structure of semiconductor catalysts strongly influences their photocatalytic performance. The energy band positions of TiO2 and In–TCBPE can be calculated using the following equation:
where
EVB is the valence band (VB) potential,
ECB is the CB potential, and
Eg is the energy bandgap.
50 According to the obtained Tauc and Mott–Schottky plots, the
ECB values of TiO
2 and In–TCBPE
versus NHE are −0.21 and 1.17 V (Fig. S3
†), and the corresponding magnitudes calculated
versus SCE amount to −0.45 and 0.93 V, respectively. The bandgaps of TiO
2 and In–TCBPE are equal to 3.21 and 2.75 eV, and their VB positions are 2.76 and 3.68 V, respectively.
Based on these results, a reasonable photocatalytic degradation mechanism is proposed for the RhB degradation by the TIT-3 composite photocatalyst (Fig. 6). Because the EVB and ECB of In–TCBPE are positive for TiO2, a staggered heterojunction can be formed between TiO2 and In–TCBPE. Note that ·OH plays a minor role in the photocatalytic degradation (as indicated by the results of the radical trapping experiments); therefore, it does not correspond to the conventional type II heterojunction.51 The reaction involves ·O2− and h+ species; hence, it likely proceeds through a Z-type heterojunction conduction mechanism.52,53 First, the light excitation of the catalyst generates electrons and holes in the CB and VB of TiO2 and In–TCBPE, respectively, and the photogenerated electrons generated in the CB of In–TCBPE are transferred through the heterostructure to the VB holes of TiO2 to rapidly complex with them.
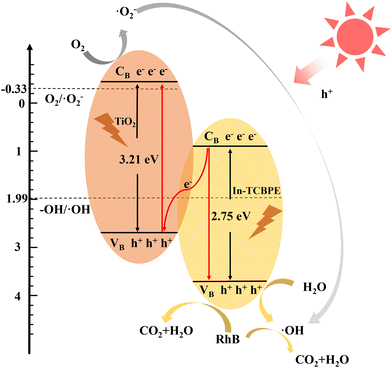 |
| Fig. 6 Schematic of the photocatalytic mechanism of the TIT-3 composite. | |
The migration of the photogenerated carriers leads to the accumulation of electrons in the CB (−0.45 V) of TiO2, which combine with the trapped O2 species to form superoxide radicals (·O2−); meanwhile, the holes in the VB of In–TCBPE exhibit a stronger oxidation ability, which can directly oxidize RhB. The photocatalytic degradation mechanism of organic dyes by the TIT-3 composite photocatalyst can be described by the following reaction equations:
TIT-3 + hv → TIT-3 + e−(CB) + h+(VB) |
RhB + h+ → CO2 + H2O + others |
RhB + ·OH + ·O2− → CO2 + H2O + others |
4. Conclusions
In summary, novel TIT composites were successfully prepared using a hydrothermal method and used to photocatalyze the degradation of organic pollutants (RhB). Both TiO2 and In–TCBPE formed a Z-type heterostructure, and the photocatalytic efficiency of the TIT-3 composite was significantly higher than those of the single materials under sunlight irradiation because TIT-3 effectively induced charge separation and the generated h+ and e− reacted with H2O and O2 to produce ·O2− and ·OH active species, respectively, which degraded RhB. Under sunlight, the catalyst effectively generated ·O2− and h+ to remove RhB and reached the 100% degradation rate in 13 min. The optimal degradation experimental conditions (In–TCBPE loading, catalyst dosage, and RhB concentration) were determined, and the catalyst performance was examined under different pH and water quality conditions. The TIT-3 catalyst also demonstrated excellent anti-interference properties and maintained excellent performance after five catalytic cycles, indicating a high potential for practical applications. The obtained results can facilitate the selection of ligands for the design of high-performance MOF photocatalysts.
Author contributions
Xinyu Liu: investigation, conceptualization, methodology, writing – original draft. Chenchen Li: investigation, methodology. Jian Nan: software. Ruike Liang: conceptualization, data curation. Huabin Wang: formal analysis, visualization. Rui Xu: supervision, project administration, funding acquisition. Yong Zhang: supervision, writing – review& editing, funding acquisition.
Conflicts of interest
The authors declare that they have no known competing financial interests or personal relationships that could have appeared to influence the work reported in this paper.
Acknowledgements
This work was supported by the National Natural Science Foundation of China (No. 22264025), the Applied Basic Research Foundation of Yunnan Province (No. 202301AS070097, 202101AS070064), the Zhang Yong Expert Grassroots Research Workstation in Yunnan Province, and the Yunnan Province Education Department Scientific Research Foundation Project (No. 2022J0136).
Notes and references
- C. Chen, W. Ma and J. Zhao, Semiconductor-mediated photodegradation of pollutants under visible-light irradiation, Chem. Soc. Rev., 2010, 39, 4206–4219 RSC.
- M. A. Al-Ghouti, M. A. M. Khraisheh, S. J. Allen and M. N. Ahmad, The removal of dyes from textile wastewater: a study of the physical characteristics and adsorption mechanisms of diatomaceous earth, J. Environ. Manage., 2003, 69, 229–238 CrossRef CAS PubMed.
- T. Zhang and W. Lin, Metal–organic frameworks for artificial photosynthesis and photocatalysis, Chem. Soc. Rev., 2014, 43, 5982–5993 RSC.
- C. Wang, J. Li, X. Lv, Y. Zhang and G. Guo, Photocatalytic organic pollutants degradation in metal–organic frameworks, Energy Environ. Sci., 2014, 7, 2831–2867 RSC.
- Y. Asakura, Y. Inaguma, K. Ueda, Y. Masubuchi and S. Yin, Synthesis of gallium oxynitride nanoparticles through hydrothermal reaction in the presence of acetylene black and their photocatalytic NOx decomposition, Nanoscale, 2018, 10, 1837–1844 RSC.
- Q. Guo, C. Zhou, Z. Ma and X. Yang, Fundamentals of TiO2 Photocatalysis: Concepts, Mechanisms, and Challenges, Adv. Mater., 2019, 31, 1901997 CrossRef CAS PubMed.
- A. Fujishima and K. Honda, Electrochemical Photolysis of Water at a Semiconductor Electrode, Nature, 1972, 238, 37–38 CrossRef CAS PubMed.
- G. Yang, H. Ding, J. Feng, Q. Hao, S. Sun, W. Ao and D. Chen, Highly Performance Core-Shell TiO2(B)/anatase Homojunction Nanobelts with Active Cobalt phosphide Cocatalyst for Hydrogen Production, Sci. Rep., 2017, 7, 14594 CrossRef PubMed.
- M. Wang, B.-J. Kim, D. Suk Han and H. Park, Electrocatalytic activity of nanoparticulate TiO2 coated onto Ta-doped IrO2/Ti substrates: Effects of the TiO2 overlayer thickness, Chem. Eng. J., 2021, 425, 131435 CrossRef CAS.
- K. He, Q. Wen, C. Wang, B. Wang, S. Yu, C. Hao and K. Chen, A facile synthesis of hierarchical flower-like TiO2 wrapped with MoS2 sheets nanostructure for enhanced electrorheological activity, Chem. Eng. J., 2018, 349, 416–427 CrossRef CAS.
- K.-I. Shimizu, H. Murayama, A. Nagai, A. Shimada, T. Hatamachi, T. Kodama and Y. Kitayama, Degradation of hydrophobic organic pollutants by titania pillared fluorine mica as a substrate specific photocatalyst, Appl. Catal., B, 2005, 55, 141–148 CrossRef CAS.
- B. Zhang, B. Chen, K. Shi, S. He, X. Liu, Z. Du and K. Yang, Preparation and characterization of nanocrystal grain TiO2 porous microspheres, Appl. Catal., B, 2003, 40, 253–258 CrossRef CAS.
- D. Liu, B. Sun, S. Bai, T. Gao and G. Zhou, Dual co-catalysts Ag/Ti3C2/TiO2 hierarchical flower-like microspheres with enhanced photocatalytic H2-production activity, Chin. J. Catal., 2023, 50, 273–283 CrossRef CAS.
- H. Safajou, H. Khojasteh, M. Salavati-Niasari and S. Mortazavi-Derazkola, Enhanced photocatalytic degradation of dyes over graphene/Pd/TiO2 nanocomposites: TiO2 nanowires versus TiO2 nanoparticles, J. Colloid Interface Sci., 2017, 498, 423–432 CrossRef CAS PubMed.
- M. Pazoki, M. Parsa and R. Farhadpour, Removal of the hormones dexamethasone (DXM) by Ag doped on TiO2 photocatalysis, J. Environ. Chem. Eng., 2016, 4, 4426–4434 CrossRef CAS.
- S. Veziroglu, A.-L. Obermann, M. Ullrich, M. Hussain, M. Kamp, L. Kienle, T. Leißner, H.-G. Rubahn, O. Polonskyi, T. Strunskus, J. Fiutowski, M. Es-Souni, J. Adam, F. Faupel and O. C. Aktas, Photodeposition of Au Nanoclusters for Enhanced Photocatalytic Dye Degradation over TiO2 Thin Film, ACS Appl. Mater. Interfaces, 2020, 12, 14983–14992 CrossRef CAS PubMed.
- S. Liu and X. Chen, A visible light response TiO2 photocatalyst realized by cationic S-doping and its application for phenol degradation, J. Hazard. Mater., 2008, 152, 48–55 CrossRef CAS PubMed.
- G. Chen, Z. Zhou, B. Li, X. Lin, C. Yang, Y. Fang, W. Lin, Y. Hou, G. Zhang and S. Wang, S-scheme heterojunction of crystalline carbon nitride nanosheets and ultrafine WO3 nanoparticles for photocatalytic CO2 reduction, J. Environ. Sci., 2023 DOI:10.1016/j.jes.2023.05.028 , in press.
- L. Pan, J. Zhang, X. Jia, Y. Ma, X. Zhang, L. Wang and J. Zou, Highly efficient Z-scheme WO3–x quantum dots/TiO2 for photocatalytic hydrogen generation, Chin. J. Catal., 2017, 38, 253–259 CrossRef CAS.
- X. Hou, S. L. Stanley, M. Zhao, J. Zhang, H. Zhou, Y. Cai, F. Huang and Q. Wei, MOF-based C-doped coupled TiO2/ZnO nanofibrous membrane with crossed network connection for enhanced photocatalytic activity, J. Alloys Compd., 2019, 777, 982–990 CrossRef CAS.
- Y. Fu, M. Tan, Z. Guo, D. Hao, Y. Xu, H. Du, C. Zhang, J. Guo, Q. Li and Q. Wang, Fabrication of wide-spectra-responsive NA/NH2-MIL-125(Ti) with boosted activity for Cr(VI) reduction and antibacterial effects, Chem. Eng. J., 2023, 452, 139417 CrossRef CAS.
- Y. Gao, S. Li, Y. Li, L. Yao and H. Zhang, Accelerated photocatalytic degradation of organic pollutant over metal-organic framework MIL-53(Fe) under visible LED light mediated by persulfate, Appl. Catal., B, 2017, 202, 165–174 CrossRef CAS.
- H. Xu, J. Hu, D. Wang, Z. Li, Q. Zhang, Y. Luo, S. Yu and H. Jiang, Visible-Light Photoreduction of CO2 in a Metal–Organic Framework: Boosting Electron–Hole Separation via Electron Trap States, J. Am. Chem. Soc., 2015, 137, 13440–13443 CrossRef CAS PubMed.
- L. Shen, R. Liang and L. Wu, Strategies for engineering metal-organic frameworks as efficient photocatalysts, Chin. J. Catal., 2015, 36, 2071–2088 CrossRef CAS.
- Y. Wang, H. Wang, F. Luo, S. Yao, T. Lu and Z. Zhang, Inter-clusters synergy in iron-organic frameworks for efficient CO2 photoreduction, Appl. Catal., B, 2022, 300, 120487 CrossRef CAS.
- T. Araya, M. Jia, J. Yang, P. Zhao, K. Cai, W. Ma and Y. Huang, Resin modified MIL-53 (Fe) MOF for improvement of photocatalytic performance, Appl. Catal., B, 2017, 203, 768–777 CrossRef CAS.
- D. Dong, C. Yan, J. Huang, N. Lu, P. Wu, J. Wang and Z. Zhang, An electron-donating strategy to guide the construction of MOF photocatalysts toward co-catalyst-free highly efficient photocatalytic H2 evolution, J. Mater. Chem. A, 2019, 7, 24180–24185 RSC.
- A. Crake, K. C. Christoforidis, A. Kafizas, S. Zafeiratos and C. Petit, CO2 capture and photocatalytic reduction using bifunctional TiO2/MOF nanocomposites under UV–vis irradiation, Appl. Catal., B, 2017, 210, 131–140 CrossRef CAS.
- Y. Chen, D. Yang, X. Xin, Z. Yang, Y. Gao, Y. Shi, Z. Zhao, K. An, W. Wang, J. Tan and Z. Jiang, Multi-stepwise charge transfer via MOF@MOF/TiO2 dual-heterojunction photocatalysts towards hydrogen evolution, J. Mater. Chem. A, 2022, 10, 9717–9725 RSC.
- R. R. Ozer and J. P. Hinestroza, One-step growth of isoreticular luminescent metal–organic frameworks on cotton fibers, RSC Adv., 2015, 5, 15198–15204 RSC.
- B. Guan, Q. Li, Y. Xu, L. Chen, Z. Wu, Z. Fan and W. Zhu, Highly selective and sensitive detection towards cationic Cu2+ and Fe3+ contaminants via an In-MOF based dual-responsive fluorescence probe, Inorg. Chem. Commun., 2020, 122, 108273 CrossRef CAS.
- J. Wang, C. Cao, J. Wang, Y. Zhang and L. Zhu, Insights into highly efficient photodegradation of poly/perfluoroalkyl substances by In-MOF/BiOF heterojunctions: Built-in electric field and strong surface adsorption, Appl. Catal., B, 2022, 304, 121013 CrossRef CAS.
- L. Hu, D. Mao, L. Yang, M. Zhu, Z. Fei, S. Sun and D. Fang, In2S3 nanoparticles coupled to In-MOF nanorods: The structural and electronic modulation for synergetic photocatalytic degradation of Rhodamine B, Environ. Res., 2022, 203, 111874 CrossRef CAS PubMed.
- D. Liu, X. Bai, J. Sun, D. Zhao, C. Hong and N. Jia, Hollow In2O3/In2S3 nanocolumn-assisted molecularly imprinted photoelectrochemical sensor for glutathione detection, Sens. Actuators, B, 2022, 359, 131542 CrossRef CAS.
- J. Annamalai, P. Murugan, D. Ganapathy, D. Nallaswamy, R. Atchudan, S. Arya, A. Khosla, S. Barathi and A. K. Sundramoorthy, Synthesis of various dimensional metal organic frameworks (MOFs) and their hybrid composites for emerging applications – A review, Chemosphere, 2022, 298, 134184 CrossRef CAS.
- Z. Wei, Z. Gu, R. K. Arvapally, Y. Chen, R. N. McDougald Jr., J. F. Ivy, A. A. Yakovenko, D. Feng, M. A. Omary and H. Zhou, Rigidifying Fluorescent Linkers by Metal–Organic Framework Formation for Fluorescence Blue Shift and Quantum Yield Enhancement, J. Am. Chem. Soc., 2014, 136, 8269–8276 CrossRef CAS PubMed.
- Z. Yang, Z. Chi, T. Yu, X. Zhang, M. Chen, B. Xu, S. Liu, Y. Zhang and J. Xu, Triphenylethylene Carbazole Derivatives as a New Class of AIE Materials with Strong Blue Light Emission and High Glass Transition Temperature, J. Mater. Chem., 2009, 19, 5541 RSC.
- J. Yang, M. Huang, L. Xu, X. Xia and C. Peng, Self-assembled titanium-deficient undoped anatase TiO2 nanoflowers for ultralong-life and high-rate Li+/Na+ storage, Chem. Eng. J., 2022, 445, 136638 CrossRef CAS.
- X. Pan, Y. Zhao, S. Liu, C. L. Korzeniewski, S. Wang and Z. Fan, Comparing Graphene-TiO2 Nanowire and Graphene-TiO2 Nanoparticle Composite Photocatalysts, ACS Appl. Mater. Interfaces, 2012, 4, 3944–3950 CrossRef CAS PubMed.
- X. Liu, X. Duan, T. Bao, D. Hao, Z. Chen, W. Wei, D. Wang, S. Wang and B.-J. Ni, High-performance photocatalytic decomposition of PFOA by BiOX/TiO2 heterojunctions: Self-induced inner electric fields and band alignment, J. Hazard. Mater., 2022, 430, 128195 CrossRef CAS PubMed.
- K. Sakamoto, F. Hayashi, K. Sato, M. Hirano and N. Ohtsu, XPS spectral analysis for a multiple oxide comprising NiO, TiO2, and NiTiO3, Appl. Surf. Sci., 2020, 526, 146729 CrossRef CAS.
- J. Wang, Y. Asakura and S. Yin, Preparation of (Zn1+xGe)(N2Ox) nanoparticles with enhanced NOx decomposition activity under visible light irradiation by nitridation of Zn2GeO4 nanoparticles designed precisely, Nanoscale, 2019, 11, 20151–20160 RSC.
- S. Sun, H. Ding, L. Mei, Y. Chen, Q. Hao, W. Chen, Z. Xu and D. Chen, Construction of SiO2-TiO2/g-C3N4 composite photocatalyst for hydrogen production and pollutant degradation: Insight into the effect of SiO2, Chin. Chem. Lett., 2020, 31, 2287–2294 CrossRef CAS.
- M. Xu, D. Li, K. Sun, L. Jiao, C. Xie, C. Ding and H. Jiang, Interfacial Microenvironment Modulation Boosting Electron Transfer between Metal Nanoparticles and MOFs for Enhanced Photocatalysis, Angew. Chem., 2021, 60, 16372–16376 CrossRef CAS PubMed.
- T. Dong, G. Jiang, Y. He, L. Yang, G. Wang and Y. Li, A novel BiOX photocatalyst for the “green” degradation of polymers used in oilfields, J. Hazard. Mater., 2022, 428, 128207 CrossRef CAS PubMed.
- Y. Liu, Y. Mao, X. Tang, Y. Xu, C. Li and F. Li, Synthesis of Ag/AgCl/Fe-S plasmonic catalyst for bisphenol A degradation in heterogeneous photo-Fenton system under visible light irradiation, Chin. J. Catal., 2017, 38, 1726–1735 CrossRef CAS.
- S. Horikoshi, T. Miura, M. Kajitani, N. Horikoshi and N. Serpone, Photodegradation of tetrahalobisphenol-A (X=Cl, Br) flame retardants and delineation of factors affecting the process, Appl. Catal., B, 2008, 84, 797–802 CrossRef CAS.
- J. L. Wang and S. Z. Wang, Reactive species in advanced oxidation processes: Formation, identification and reaction mechanism, Chem. Eng. J., 2020, 401, 126158 CrossRef CAS.
- T. Wu, G. Liu, J. Zhao, H. Hidaka and N. Serpone, Photoassisted Degradation of Dye Pollutants. V. Self-Photosensitized Oxidative Transformation of Rhodamine B under Visible Light Irradiation in Aqueous TiO2 Dispersions, J. Phys. Chem. B, 1998, 102, 5845–5851 CrossRef CAS.
- R. Li, J. Hu, M. Deng, H. Wang, X. Wang, Y. Hu, H. Jiang, J. Jiang, Q. Zhang, Y. Xie and Y. Xiong, Integration of an Inorganic Semiconductor with a Metal–Organic Framework: A Platform for Enhanced Gaseous Photocatalytic Reactions, Adv. Mater., 2014, 26, 4783–4788 CrossRef CAS PubMed.
- B. Su, M. Zheng, W. Lin, X. F. Lu, D. Luan, S. Wang and X. W. Lou, S-Scheme Co9S8@Cd0.8Zn0.2S-DETA Hierarchical Nanocages Bearing Organic CO2 Activators for Photocatalytic Syngas Production, Adv. Energy Mater., 2023, 13, 2203290 CrossRef CAS.
- J. Wang, Y. Asakura, T. Hasegawa and S. Yin, High-concentration N-doped La2Ti2O7 nanocrystals: Effects of nano-structuration and doping sites on enhancing the photocatalytic activity, Chem. Eng. J., 2021, 423, 130220 CrossRef CAS.
- B. Li, W. Wang, J. Zhao, Z. Wang, B. Su, Y. Hou, Z. Ding, W.-J. Ong and S. Wang, All-solid-state direct Z-scheme NiTiO3/Cd0.5Zn0.5S heterostructures for photocatalytic hydrogen evolution with visible light, J. Mater. Chem. A, 2021, 9, 10270–10276 RSC.
|
This journal is © The Royal Society of Chemistry 2024 |