Revealing the primary role of the V4+/V5+ cycle in InVO4 catalysts for promoting the photo-Fenton reaction†
Received
28th October 2023
, Accepted 29th December 2023
First published on 29th December 2023
Abstract
Surface oxygen and metal defects can promote the performance of photocatalysts significantly, but their role in the activation of hydrogen peroxide (H2O2) for pollutant degradation is not clear. Herein, the surface oxygen vacancies (VO) and vanadium vacancies (VV: V4+/V5+) of InVO4 catalysts were adjusted using different surfactants. Among them, a cationic surfactant with a hydrophilic head of quaternary ammonium ions facilitated the formation of surface vanadium vacancies (V4+/V5+) on the InVO4 crystal. This VV-dominated InVO4 exhibited the optimal degradation rate of rhodamine B and microcystin-LR (MC-LR) with H2O2 (0.045 M) at neutral pH under visible-light irradiation, which was ∼8.2-times versus 3.2-times larger than that of the Vo-dominated InVO4 system. The cycle of V4+/V5+ decomposed H2O2 to yield hydroxyl radicals (·OH) and superoxide radicals (·O2−) continuously, which degraded organic pollutants efficiently. This study provides insights into the primary role of the V4+/V5+ cycle in InVO4 catalysts for promoting the photo-Fenton reaction via adjuration of surfactants.
Environmental significance
Surface oxygen and metal defects can promote the performance of photocatalysts significantly, but their role in the activation of H2O2 for pollutant degradation is not clear. Herein, the surface oxygen vacancies (VO) and vanadium vacancies (VV: V4+/V5+) of InVO4 catalysts were adjusted using different surfactants. This study provides insights into the primary role of the V4+/V5+ cycle in InVO4 catalysts for promoting the photo-Fenton reaction via adjuration of surfactants.
|
1. Introduction
Advanced oxidation processes have been used widely as efficient treatment for the removal of organic pollutants. Hydroxyl radical (·OH)-based Fenton reaction1 with strong oxidation has attracted attention as a way to solve water pollution.2 Among the many types of Fenton systems, heterogeneous catalysts (especially photo-Fenton catalysts) can be used for the degradation of organic contaminants, due to their recyclability,3 low metal dissolution,4 wide pH applicability5 and use of nature light.6 Vanadate catalysts7 are promising visible-light photocatalysts8 for CO2 reduction,9 air purification,10 hydrogen production11 and water treatment.12 However, inadequate adsorption capacity,13 fast recombination of photogenerated electrons (e−) and holes (h+)14 and a narrow absorption15 range in visible light have hindered industrial application of vanadate photocatalysts. Hence, combining InVO4 photocatalysis with the Fenton reaction for wastewater treatment is a rational approach.
Unfortunately, activating peroxides and producing reactive oxygen species (ROS) to degrade organic compounds using pure InVO4 photocatalysis is difficult because of the high metal valence of vanadium (V5+). Ji and colleagues found that InVO4–BiVO4 having a high molar ratio of V4+/V5+ presented excellent photocatalytic activity in the presence of H2O2.16 In addition, Yao et al. found that V4+ rather than the oxygen vacancy (VO) of InVO4 could promote N2 activation to NH3, which was confirmed by control experiments and theoretical calculation.17 Hence, it was possible to form a high-efficiency photo-Fenton system for water treatment if surface (especially metal) defects18 (Vm) were introduced on the surface of photocatalysts.19 A surfactant was used commonly for the modification of photocatalytic materials and resulted in a vacancy on the material surface. Bi2O2CO3 photocatalysis obtained surface Vo defects by the addition of hexadecyl trimethyl ammonium bromide (CTAB).20 In addition, the photoelectric material BiVO4 was found to have VV defects on its surface during preparation using CTAB as a template. BiVO4 with VV defects showed better light absorption and higher efficiency of CO2 reduction than that in an unmodified system.21
In the present study, we prepared a series of InVO4 photocatalysts with different dominant surface defects (VO or VV) using different surfactants to investigate the effect of the surface defects of InVO4 catalysts on photo-Fenton activity. In particular, the activation mechanism of H2O2 by these surface defects was analyzed. Notably, combining optical microscopy and HRTEM methods, we studied (for the first time) the relationship between the spatial structure of surfactants and types of surface defects. Our data helped to reveal the primary role of the V4+/V5+ cycle in an InVO4 catalyst for promoting the photo-Fenton reaction.
2. Results and discussion
2.1 Characterizations
2.1.1 Comparison of surface VV and VO defects.
As described in section 2.1 of the ESI,† C-InVO4 and B-InVO4 were prepared with N-hexadecyltrimethylammonium chloride (CTAC) and bromohexadecyl pyridine (CPB), respectively. The structure of CTAC and CPB are displayed in Fig. 1. The N-InVO4 sample was synthesized without using a surfactant. Fig. 2a shows the XRD patterns of as-prepared InVO4 samples. Similar to N-InVO4 prepared without surfactant addition, C-InVO4 and B-InVO4 samples displayed sharp diffraction peaks at 20.85°, 31.07° and 33.05°, which could be assigned to the characteristic (020), (200) and (112) facets of the orthorhombic phase of InVO4 (JCPDF: 48-0898), respectively. These data indicated that modification of a cationic surfactant did not affect the crystal structure of InVO4 (which had good crystallinity). Notably, compared with N-InVO4, samples of B-InVO4 and C-InVO4 showed a non-ignorable shift at 33.05° (Fig. S1a;† which has been demonstrated to be due to the surface defects of the InVO4 lattice22) and a shift to the right for C-InVO4 and the left for B-InVO4 (which might denote different surface defects).
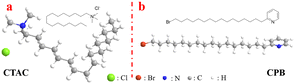 |
| Fig. 1 Structure of (a) CTAC and (b) CPB. | |
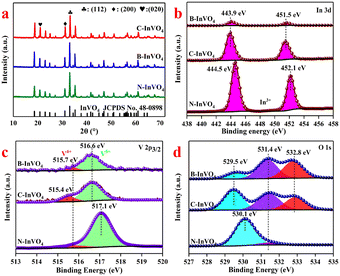 |
| Fig. 2 (a) XRD patterns of InVO4. XPS spectrum of as-prepared InVO4 samples: (b) In3d, (c) V 2p3/2 and (d) O1s. | |
XPS could be used to identify these defects. In the XPS spectra, a conspicuous doublet located at 444.5 eV (In 3d3/2) and 452.1 eV (In 3d5/2) were attributed to the surface In3+ species of the N-InVO4 sample (Fig. 2b). The binding energy of In shifted to its lower value (443.9 eV, In 3d3/2; 451.5 eV, In 3d5/2) with C-InVO4 and B-InVO4. Meanwhile, the two bands at 515.7 and 517.1 eV were assigned to the surface V4+ and V5+ species with N-InVO4, respectively (Fig. 2c). A similar lower value of V was observed on the surface of C-InVO4 (516.6 eV, V5+; 515.4 eV, V4+) and B-InVO4 (516.6 eV, V5+; 515.7 eV, V4+). The percentages of V4+ defects in C-InVO4 and B-InVO4 were calculated to be 18.3% and 12.57%, respectively, using an integral method (Table S1†),23 which were much higher than that of N-InVO4 (9.49%). These data indicated that the modification of cationic surfactants generated more surface Vv defects for the InVO4 catalyst, but did not affect the crystal phase or crystallinity. To confirm this finding, the photoluminescence (PL) emission spectra of InVO4 samples were also obtained. C-InVO4 and B-InVO4 samples showed a PL band at 469 nm, which was attributed to the intrinsic defects, including VV and VO (Fig. S1b†).24
As shown in Fig. 2d, there were three types of oxygen species: lattice oxygen (O1), bridging hydroxyl (O2) and the adsorption of O2 species on VOs (O3). These were centered at 530.1, 531.4 and 532.8 eV, respectively.25 The VOs concentrations (Table S1†) of C-InVO4 (25.47%) and B-InVO4 (40.44%) were higher than that of N-InVO4 (3.91%), confirming that modification with the cationic surfactant generated surface VO defects, which was in agreement with the literature.20 The formation of VOs arose from the charge balance of V5+/V4+.26
While VO and VV defects were present at the surfaces of C-InVO4 and B-InVO4, there were more VV defects at the C-InVO4 surface than those of B-InVO4. In contrast, many fewer VO defects of C-InVO4 were present than those of B-InVO4 (Table S1†). The differences between two samples in the concentration of surface defects aided distinguishing of the respective effects of VO and VV upon photocatalytic performance.
2.1.2 Structure of surfactant-mediated surface defects on InVO4.
We wished to gain insight into the formation mechanism of two types of surface defect. We divided the preparation process of InVO4 into four steps, and monitored each step using an optical microscope (Fig. S2†). When the reacting ingredients were mixed adequately, particles were not observed in the presence of CTAC (Fig. S2b1†) and CPB (Fig. S2c1†), and sheet or cube crystals formed in the absence of a surfactant (Fig. S2a1†). After adjustment of the pH to 9.28, an assembly structure was observed in the presence of the CTAC (Fig. S2b2†) and CPB systems (Fig. S2c2†), indicating that pH played a crucial part in the formation of precursors. In the non-surfactant system and cationic surfactant (CTAC and CPB) under hydrothermal treatment (Fig. S2a3, a4, b3, b4, c3 and c4†), eye-like and tree-like crystal nuclei formed gradually. They grew in an ordered way to form the tree-like crystals of InVO4. In the absence of a surfactant, the tree-like crystals exhibited some short and fragmentary branches (Fig. S2a4†). In the presence of CTAC, these crystals had “branches”, which then grew in an orderly manner along horizontal and vertical directions (Fig. S2b3†). These phenomena could be explained by the formation of oil-in-water spherical micelles at this concentration (Fig. S3a†). In contrast, in the presence of CPB, tree-like branches had many slender and curved branches (Fig. S2c4†) as rod micelles were formed (Fig. S3b†).
As described in Table S2,† the pore sizes of all samples ranged from 7 nm to 15 nm, and the pore volume increased from 0.008 cm3 g−1 for N-InVO4 catalyst to 0.019 cm3 g−1 for B-InVO4 and further to 0.084 cm3 g−1 for C-InVO4. In addition, the specific surface area of N-InVO4, B-InVO4 and C-InVO4 was 3.91, 10.95 and 23.08 m2 g−1, respectively. These results indicated that CTAC was most effective in modifying the structure of C-InVO4 to achieve a small crystal size (Fig. S4†), large porosity and good crystallinity. The largest specific surface area of C-InVO4 was conducive to providing more active sites and improving the photocatalytic performance. HRTEM (Fig. 3) further confirmed that the presence of surfactants affected the morphology and surface structure of InVO4 crystals. First, the images in Fig. 3a1–c1 showed that the particle size of C-InVO4 was the smallest, consistent with the analysis stated above. The presence of a surfactant in the InVO4 synthesis reduced the crystal size substantially, and the small crystals had a large surface to expose to reactants. Second, the HRTEM images of Fig. 3a2–c2 showed that the lattice spacing of N-InVO4 and C-InVO4 samples was 2.900 Å (assigned to the (200) plane of orthogonal InVO4 (2.876 Å)), and the lattice spacing of B-InVO4 sample was 4.246 Å (assigned to the (020) plane of orthogonal InVO4 (4.259 Å)). The main exposed plane of N-InVO4 and C-InVO4 was the (200) facet, whereas that of B-InVO4 was the (020) facet. These results indicated the one could tune the exposed facet of InVO4 using different surfactants. Consequently, the V4+ defects of C-InVO4 with a large surface area provided many active catalytic sites. VO defects were predominantly the surface vacancies of B-InVO4.
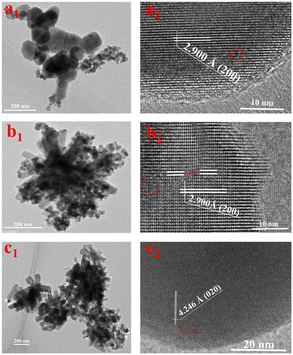 |
| Fig. 3 TEM images of (a1) N-InVO4, (b1) C-InVO4 and (c1) B-InVO4. HRTEM images of (a2) N-InVO4, (b2) C-InVO4 and (c2) B-InVO4. | |
CPB and CTAC had the same hydrophobic tails of the hexadecyl group, and their hydrophilic head groups were different (Fig. 1). The hydrophilic head of the surfactant interacted with the crystal nuclei and crystal facets of InVO4, which affected the growth of InVO4 crystals but also regulated their predominate facets.27 The hydrophilic head of CPB displayed a planar structure owing to a pyridine ring, whereas the hydrophilic quaternary ammonium ion of CTAC showed a tetrahedral spatial configuration. The planar structure head of CPB was favourable for forming InVO4 crystals with (020) facets. In contrast, the tetrahedral spatial structure head of CTAC favored the formation of InVO4 crystals with (200) facets. A possible formation mechanism is shown in Fig. 4.
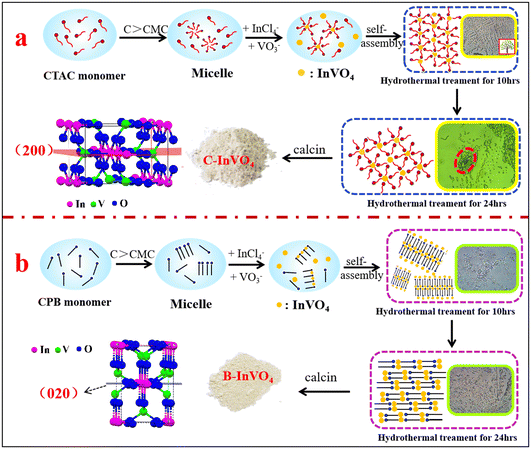 |
| Fig. 4 Tuning mechanism of a surfactant for the surface defects of (a) C-InVO4 and (b) B-InVO4. | |
2.1.3 Optical and electrochemical properties.
The optical properties of C-InVO4, B-InVO4 and N-InVO4 were illustrated in UV-vis DRS spectra (Fig. 5a). All samples had strong absorption in the visible-light region (520–580 nm). The bandgap energies (Eg) of InVO4 photocatalysts were obtained by their corresponding Tauc plots from UV-vis DRS.28 As shown in Fig. S5,† the bandgap Eg of C-InVO4, B-InVO4 and N-InVO4 was 2.37, 2.17 and 2.07 eV, respectively. Photocurrent response spectra (Fig. 5b) showed that the C-InVO4 photocatalyst exhibited the most significant photocurrent response, which resulted from the highest separation efficiency. The PL spectrum29 also confirmed that the C-InVO4 photocatalyst exhibited lower e−–h+ recombination30 compared with that for N-InVO4 and B-InVO4 (Fig. S6a†). Furthermore, electrochemical impedance spectroscopy (EIS) was introduced to evaluate the charge-carrier separation. A smaller semi-circular radius of C-InVO4 than that of N-InVO4 and B-InVO4 photocatalysts was documented (Fig. S6b†). This observation indicated that C-InVO4 had low resistance and was more helpful for the transference of interfacial charge, which conformed to the results for the photocurrent response and PL. All these properties showed a better photocatalytic performance of the C-InVO4 sample.
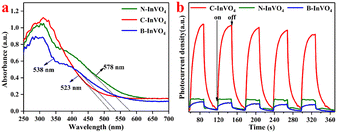 |
| Fig. 5 (a) Spectra of UV-vis DRS and (b) curves for transient photocurrent intensity versus time. | |
2.2 Photocatalytic activity
Azo dyes are present in untreated dye water and pose a serious threat to life.31 Degradation experiments of rhodamine B (RhB) using as-prepared samples with H2O2 under λ > 420 nm visible-light irradiation were carried out. As shown in Fig. 6a, there was negligible degradation of RhB (Ct/C0 = 5.6% and 18.7%, respectively) in blank experiments (only RhB or without catalyst) after 150 min illumination. Percent degradation of RhB using C-InVO4, B-InVO4 and N-InVO4 was 98.8%, 29.6% and 28.5%, respectively (Fig. 6a). Notably, the reaction rate constant of C-InVO4 (k = 0.0255 min−1) was much higher than that of B-InVO4 (k = 0.0031 min−1), and unmodified N-InVO4 (k = 0.0025 min−1) (Fig. 6b). The photocatalytic activity of C-InVO4 was nearly 8.2-times higher than that of B-InVO4.
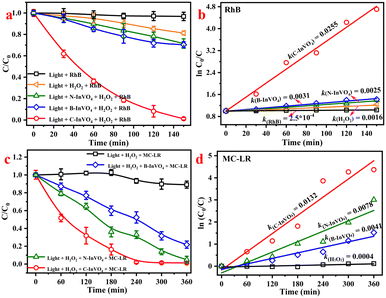 |
| Fig. 6 (a) Degradation curve of RhB (1.5 × 10−5 M) using InVO4/H2O2 (pH = 5.93) and (b) its pseudo-first-order kinetic curve. (c) Degradation curve of MC-LR (5 × 10−6 M) using InVO4/H2O2 (pH = 6.73) and (d) the corresponding pseudo-first-order kinetic curve. Reaction conditions: [catalyst] = 0.4 g L−1, [H2O2] = 0.045 M, T = 35.5 °C. | |
To show that the degradation stated above was photocatalysis rather than photosensitization, we conducted microcystin-LR (MC-LR) degradation (Fig. 6c). The photocatalytic activity of these samples was C-InVO4 > N-InVO4 > B-InVO4, and their rate constant was 0.0132, 0.0078 and 0.0041 min−1, respectively. In comparison, the constant for MC-LR/H2O2 system (without catalyst) was only 0.0004 min−1 (Fig. 6d). The degradation ability of N-InVO4 was higher than that of B-InVO4 for MC-LR. It was contrary to the degradation of RhB because the overloaded bulk oxygen defects in B-InVO4 acted as centers of charge recombination.17 This result demonstrated the improvement in the efficiency of degradation of organic pollutants caused by CTAC modification. Moreover, different photocatalysts for the removal of pollutants via a photo-Fenton system were compared (Table S3†). C-InVO4 was quite comparable with other photocatalysts. In addition, dark adsorption had a negligible role in the overall reaction. The adsorption reaction of RhB and MC-LR could reach equilibrium within 120 min under dark conditions (Fig. S7a and b†). Fig. S7c† revealed the role of C-InVO4 in H2O2 activation. Only 28.8% RhB was removed by C-InVO4 in the absence of H2O2 after 150 min illumination, whereas nearly 100% degradation of RhB was achieved in C-InVO4/H2O2 under visible-light irradiation. C-InVO4 exhibited restrictive activity for RhB degradation but could activate H2O2 for degradation. In addition, the C-InVO4/H2O2 system removed only 8.7% RhB in the dark (Fig. S7d†). Hence, H2O2 and visible light had crucial roles in RhB degradation.
2.3 Activation mechanism of H2O2 on the InVO4 surface
2.3.1 C-InVO4 exhibited the highest potential of H2O2 activation.
Fig. 7a showed the decomposition curve of H2O2 under irradiation for 150 min, and the percent decomposition values of C-InVO4, B-InVO4 and N-InVO4 samples were 4.98%, 2.49% and 3.18%, respectively, which were significantly higher than that of the control group (H2O2 only, 1.77%). Hence, InVO4 samples modified by CTAC with higher V4+ defects could promote H2O2 decomposition. The total quantity of H2O2 decomposed under irradiation is illustrated in Fig. S8.† The content of H2O2 decomposed by C-InVO4 (2.24 mM) was nearly two-times higher than that of B-InVO4 (1.12 mM), which further confirmed that C-InVO4 having more V4+ defects could activate H2O2. Interestingly, in the initial 40 min, the percent decomposition of H2O2 over C-InVO4 or B-InVO4 was negligible, much lower than that of N-InVO4 (Fig. 7a). There appeared to be competing regeneration and decomposition of H2O2 in the two modified InVO4 systems, and the regeneration rate of H2O2 over C-InVO4 was higher than the decomposition rate between 30 min and 60 min. After 60 min, H2O2 decomposition in C-InVO4/H2O2 and B-InVO4/H2O2 systems proceeded rapidly. Their decomposition rate constants after the initial 60 min were 0.027 and 0.020 h−1, respectively, which were much higher than that of N-InVO4/H2O2 (0.012 h−1) and the control experiment without a catalyst (H2O2 only, 0.00088 h−1) (Fig. 7b), further confirming the higher capacity of H2O2 activation by C-InVO4 owing to more surface V4+ defects.
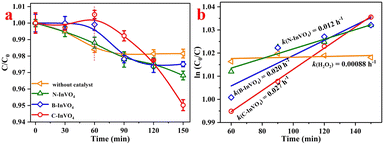 |
| Fig. 7 (a) Degradation curve of H2O2 with visible-light irradiation. (b) Pseudo-first-order kinetics with illumination from 60 to 150 min [catal] = 0.4 g L−1, [RhB] = 1.5 × 10−5 M, T = 33.2 °C, [H2O2] = 0.045 M, pH = 5.94. | |
2.3.2 Activation of H2O2via the V4+/V5+ cycle on the C-InVO4 surface.
H2O2 decomposition generated ROS. Trapping agents were used to identify the ROS produced over C-InVO4 (the most active catalyst). Ethylenediaminetetraacetic acid (EDTA),32 benzoquinone (BQ)33 and isopropyl alcohol (IPA)34 were used as trapping agents to capture metal ions, O2·− and ·OH, respectively. As depicted in Fig. S9,† only 37.6% of RhB was removed with EDTA addition, suggesting that metal ions (V4+) were important for RhB degradation. Meanwhile, the degradation efficiency was decreased to 85.4% in IPA, indicating that ·OH radicals were involved in the degradation of RhB. Interestingly, RhB degradation was accelerated with BQ. A similar phenomenon was found in the Fenton-like reaction.35 During this reaction, the cycle of Fe2+/Fe3+ was constructed through p-xylenol/BQ and ROS, and promoted photocatalytic activity. Therefore, we proposed that the reaction in the C-InVO4/H2O2 system followed a mechanism similar to the Fenton reaction, wherein the V4+/V5+ cycle was established. Furthermore, the successive production of ·OH and ·O2− in C-InVO4/H2O2 with or without a light system using terephthalic acid (TA) and nitro blue tetrazolium (NBT) as probes, respectively, was observed.3 As illustrated in Fig. S10,† 21.9 μmol L−1 of ·OH was produced, along with 2470 μmol L−1 H2O2 (5.49%) consumption without illumination. However, the generation of ·O2− was only 7.2 μmol L−1 in the dark. In the presence of visible light, much less H2O2 (4.98%, 2240 μmol L−1) was decomposed to produce a higher amount of ·OH (53.49 μmol L−1) and ·O2− (54.08 μmol L−1). In general, the consumption of H2O2 could be promoted by light radiation and more active radicals generated. Our unusual discovery could be explained by the regeneration of H2O2 in the C-InVO4 photo-Fenton, and this conclusion was demonstrated with the results shown in Fig. 7. In addition, the percentage of H2O2 converted to ·OH under visible light (2.39%) was 2.6-times higher than that in the dark (0.90%) (Fig. S10b†). The increasing concentration of ·OH and ·O2− in visible light also indicated the important role of two types of ROS in the degradation of organic pollutants.
The formation of active radicals was investigated further with ESR.36 As illustrated in Fig. 8a, weak quadruple signals with an intensity ratio 1.0
:
2.0
:
2.0
:
1.0 for DMPO–·OH adducts were observed in the C-InVO4/H2O2 system in the dark, and they increased significantly under visible-light irradiation due to greater generation of ·OH species. The generation of ·OH in the dark condition could indicate the V4+/V5+ cycle by H2O2 decomposition. In addition, DMPO/O2·− signals were not recorded in the dark, whereas DMPO/O2·− adducts appeared and increased (Fig. 8b) with increasing irradiation time, which indicated that visible light promoted the formation of O2·−. O2·− reacted with V5+ and water to regenerate H2O2 and V4+. Therefore, H2O2 could be activated and multiplied via the V4+/V5+ cycle under visible-light irradiation.
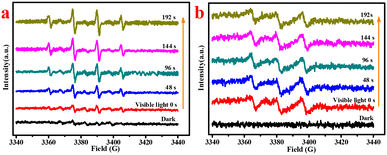 |
| Fig. 8 (a) ESR tests for DMPO–·OH in water and (b) DMPO–O2·− in methanol of the C-InVO4/H2O2 system, [catal] = 0.4 g L−1, [H2O2] = 0.045 M. | |
An acidic condition plays a crucial part in Fenton reactions.37 We investigated the effect of pH upon RhB degradation. Fig. 9a showed that the removal of RhB using the C-InVO4/H2O2 system could reach at least 82.11% in a wide range of pH (2.39 to 10.61). This finding indicated that the reaction mechanism did not merely involve the Fenton mechanism.
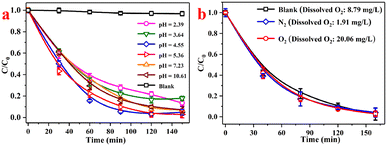 |
| Fig. 9 (a) Photodegradation curves of RhB solution under different pH conditions. (b) Photodegradation curves of RhB solution with different amounts of dissolved O2 (pH = 5.94). Reaction conditions: [catal] = 0.4 g L−1, [RhB] = 1.5 × 10−5 M, T = 33.7 °C, [H2O2] = 0.045 M. | |
2.3.3 Surface VO had a minimal role in H2O2 activation.
It has been reported that VO can activate the molecular O2 adsorbed38 on a surface to yield ROS,39 thereby resulting the pollutant degradation. Based on previous analyses, two types of defects (VV and VO) were formed on the C-InVO4 surface. In principle, the degradation ability of RhB could be improved with an increasing concentration of dissolved O2. However, the dissolved oxygen concentration (DOC) in this suspension barely affected the degradation of RhB (8.79, 1.91 and 20.06 mg L−1, respectively) (Fig. 9b). This finding indicated that activation of the O2 adsorbed at VO did not make an essential contribution to photocatalysis of the C-InVO4/H2O2 system. This observation was also consistent with the poor performance of B-InVO4, in which VO predominated. We also validated that H2O2 instead of O2 was the only source of ·O2−.40 Therefore, the V4+/V5+ cycle should be the primary driving force of photocatalysis. VV defects were more important active sites for H2O2 activation than VO defects.
XPS (V 2p3/2) analysis of C-InVO4, B-InVO4 and N-InVO4 samples after degradation (Fig. 10a) corroborated our conclusion. Degradation did not cause a substantial change in the content of V4+ defects in N-InVO4 (9.49% → 7.71%) and B-InVO4 (12.57% → 8.19%) systems. In contrast, surface V4+ defects of C-InVO4 decreased considerably from 18.3% (Table S1†) to 5.7% after degradation (Table S4†). Meanwhile, V5+ increased by 12.6%. These synergistic changes in V4+ and V5+ defects suggested that V4+ reacted with H2O2 to yield V5+ on the C-InVO4 surface.
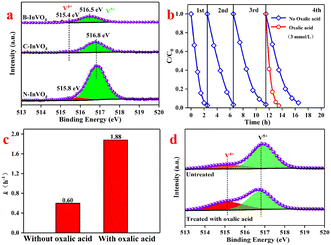 |
| Fig. 10 (a) XPS spectrum of C-InVO4, B-InVO4 and N-InVO4 samples after degradation: V 2p3/2. (b) Cycling performance of the C-InVO4 sample upon addition of oxalic acid. (c) Rate constant and (d) XPS V 2p3/2 spectrum of C-InVO4 treated/not treated with oxalic acid. Reaction conditions: [catal] = 0.4 g L−1, [RhB] = 1.5 × 10−5 M, T = 33.0 °C, [H2O2] = 0.045 M. | |
To provide further evidence for the proposed activation mechanism of H2O2, we undertook cyclic degradation experiments of RhB in the C-InVO4/H2O2 system. For four cycles, the degradation efficiency become slower and slower (Fig. 10b) because V4+ was converted persistently to V5+, which could not activate H2O2. To verify this hypothesis, oxalic acid (reactant) was added in the fourth cycle to reduce V5+ to V4+. This additive significantly enhanced the degradation rate of RhB over C-InVO4 in the presence of H2O2 (Fig. 10b). The reaction rate constant reached 1.88 h−1, which was 3.13-times greater than that of the untreated C-InVO4 system (k = 0.60 h−1) (Fig. 10c). This finding demonstrated the function of surface V4+ defects for the degradation capacity of C-InVO4, which reacted with H2O2 to yield V5+ and ROS. As shown in Fig. 10d, the higher concentration of V4+ defects (32.6%) of C-InVO4 treated with oxalic acid after the first degradation experiments of RhB in the C-InVO4/H2O2 system led to a higher degradation rate compared with that using untreated C-InVO4 (5.7%) (Table S4†). These data verified the decisive influence of V4+ on activation of H2O2. We also examined other reducing agents (e.g. ascorbic acid, sodium sulfite, and sodium citrate) on the fourth-cycle experiment, and identical results were obtained (Fig. S11†).
Overall, the data suggested that the surface V4+ defects of C-InVO4 reduced H2O2 to give ·OH, which was accelerated by irradiation. Simultaneously, visible-light irradiation enabled decomposition of H2O2 to yield ·O2−. A tentative photocatalytic activation mechanism of H2O2 by surface VV defects on C-InVO4 was proposed using these equations:
| V4+ + H2O2 → V5+ + ·OH + OH− | (1) |
| 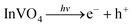 | (2) |
|  | (4) |
| ·O2− + V5+ + 2H2O → 2H2O2 + V4+ | (5) |
Surface V
4+ defects reacted with absorbed H
2O
2 (ref.
41) on the C-InVO
4 surface to give ·OH (
eqn (1)).
42 A photogenerated e
−–h
+ pair was separated by visible-light irradiation
43 (
eqn (2)), wherein H
2O
2 captured e
− (ref.
44) to produce ·OH (ref.
45–47) (
eqn (3)). Meanwhile, visible-light irradiation promoted the reaction of H
2O
2 with the ·OH radical to yield ·O
2− (
eqn (4)).
40 The V
5+ yielded reacted with ·O
2− to regenerate H
2O
2 (
eqn (5)), and the V
4+/V
5+ cycle was established (
Fig. 11).
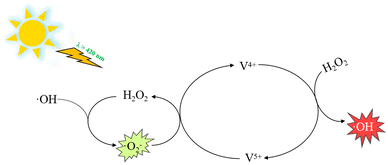 |
| Fig. 11 Photocatalytic mechanism of C-InVO4 in the presence of H2O2 under visible-light irradiation. | |
3. Conclusions
A series of InVO4 photocatalysts with different dominant surface defects (VO or VV) was synthesized using different surfactants to investigate the effect of the surface defects of InVO4 catalysts on photocatalytic activity. Among them, the CTAC surfactant with a hydrophilic head of quaternary ammonium cations facilitated small C-InVO4 crystals with (200) facets enriched with surface V4+ defects. This VV-dominated InVO4 exhibited the optimal degradation rate of RhB and microcystin-LR with H2O2 (0.045 M) at neutral pH under visible-light irradiation, which was ∼8.2-times versus ∼3.2-times larger than that of the VO-dominated InVO4 system. These VV defects could activate H2O2 to generate ·OH and O2·− under visible-light absorption, so these species could degrade organic pollutants efficiently. Our study provides insights into the primary role of the V4+/V5+ cycle in InVO4 catalyst for promoting the photo-Fenton reaction via adjuration of surfactants.
Author contributions
X. Liu and D. Huang conceived this project. L. Jin and H. Liu performed the experiments. X. Liu, Y. Huang, L. Ye and D. Huang analyzed the data and wrote the manuscript. All authors provided scientific input as well as editing and approving the final version of the manuscript.
Conflicts of interest
There are no conflicts of interest to declare.
Acknowledgements
This work was supported financially by NSFC (22136003, 21972073, 22076098, 21805166) and the 111 Project (D20015).
Notes and references
- Y. Liu and J. Wang, Multivalent metal catalysts in Fenton/Fenton-like oxidation system: A critical review, Chem. Eng. J., 2023, 466, 143147 CrossRef.
- Y. Liu, Y. Zhao and J. Wang, Fenton/Fenton-like processes with in-situ production of hydrogen peroxide/hydroxyl radical for degradation of emerging contaminants: Advances and prospects, J. Hazard. Mater., 2021, 404, 124191 CrossRef PubMed.
- Y. Shi, X. Wang, X. Liu, C. Liu, W. Shen and L. Zhang, Visible light promoted Fe3S4 Fenton oxidation of atrazine, Appl. Catal., B, 2020, 277, 119229 CrossRef CAS.
- Y. Chen, M. Xu, J. Wen, Y. Wan, Q. Zhao, X. Cao, Y. Ding, Z. Wang, H. Li and Z. Bian, Selective recovery of precious metals through photocatalysis, Nat. Sustain., 2021, 4, 618–626 CrossRef.
- J. Xu, Q. Zhang, X. Gao, P. Wang, H. Che, C. Tang and Y. Ao, Highly efficient FeIII-initiated self-cycled Fenton system in piezo-catalytic process for organic pollutants degradation, Angew. Chem., Int. Ed., 2023, 62, e2023070 Search PubMed.
- C. Dong, Y. Yang, X. Hu, Y. Cho, G. Jang, Y. Ao, L. Wang, J. Shen, J. H. Park and K. Zhang, Self-cycled photo-Fenton-like system based on an artificial leaf with a solar-to-H2O2 conversion efficiency of 1.46%, Nat. Commun., 2022, 13, 4982 CrossRef PubMed.
- P. Dhull, A. Sudhaik, V. Sharma, P. Raizada, V. Hasija, N. Gupta, T. Ahamad, V. Nguyen, A. Kim, M. Shokouhimehr, S. Kim, Q. V. Le and P. Singh, An overview on InVO4-based photocatalysts: Electronic properties, synthesis, enhancement strategies, and photocatalytic applications, Mol. Catal., 2023, 539, 113013 CrossRef.
- M. R. Tamtam, R. Koutavarapu and J. Shim, InVO4 nanosheets decorated with ZnWO4 nanorods: A novel composite and its enhanced photocatalytic performance under solar light, Environ. Res., 2023, 227, 115735 CrossRef PubMed.
- Q. Han, X. Bai, Z. Man, H. He, L. Li, J. Hu, A. Alsaedi, T. Hayat, Z. Yu, W. Zhang, J. Wang, Y. Zhou and Z. Zou, Convincing synthesis of atomically thin, single-crystalline InVO4 Sheets toward promoting highly selective and efficient solar conversion of CO2 into CO, J. Am. Chem. Soc., 2019, 141, 4209–4213 CrossRef PubMed.
- J. Hu, D. Chen, N. Li, Q. Xu, H. Li, J. He and J. Lu, Fabrication of grafitic-C3N4 quantum dots/graphene-InVO4 aerogel hybrids with enhanced photocatalytic NO removal under visible-light irradiation, Appl. Catal., B, 2018, 236, 45–52 CrossRef.
- Z. Kong, Y. Yuan, D. Chen, G. Fang, Y. Yang, S. Yang and D. Gao, Noble-metal-free MoS2 nanosheet modified-InVO4 heterostructure for enhanced visible-light-driven photocatalytic H2 production, Dalton Trans., 2017, 46, 2072–2076 RSC.
- J. Liu, S. Li, C. Meng, J. Sun, X. Li, Y. Hu and H. Cheng, Visible-light induced tetracycline degradation catalyzed by dual Z-scheme InVO4/CuBi2O4/BiVO4 composites: The roles of oxygen vacancies and interfacial chemical bonds, Chem. Eng. J., 2023, 473, 145278 CrossRef.
- Y. Yan, X. Liu, W. Fan, P. Lv and W. Shi, InVO4 microspheres: Preparation, characterization and visible-light-driven photocatalytic activities, Chem. Eng. J., 2012, 200–202, 310–316 CrossRef.
- A. Kumar, P. Prajapati, U. Pal and S. Jain, Ternary rGO/InVO4/Fe2O3 Z-Scheme heterostructured photocatalyst for CO2 reduction under visible light irradiation, ACS Sustainable Chem. Eng., 2018, 6, 8201–8211 CrossRef.
- B. Hu, F. Cai, J. Chen, M. Fan, C. Song, X. Yan and W. Shi, Hydrothermal synthesis g-C3N4/nano-InVO4 nanocomposites and enhanced photocatalytic activity for hydrogen production under visible light irradiation, ACS Appl. Mater. Interfaces, 2015, 7, 18247–18256 CrossRef PubMed.
- K. Ji, J. Deng, H. Zang, J. Han, H. Arandiyand and H. Dai, Fabrication and high photocatalytic performance of noble metal nanoparticles supported on 3DOM InVO4-BiVO4 for the visible-light-driven degradation of rhodamine B and methylene blue, Appl. Catal., B, 2015, 206, 300–307 Search PubMed.
- S. Yao, J. Liu, F. Liu, B. Wang, Y. Ding, L. Li, C. Liu, F. Huang, J. Fang, Z. Lin and M. Wang, Robust route to photocatalytic nitrogen fixation mediated by capitalizing on defect-tailored InVO4 nanosheets, Environ. Sci.: Nano, 2022, 9, 1996 RSC.
- H. Li, J. Li, Z. Ai, F. Jia and L. Zhang, Oxygen vacancy-mediated photocatalysis of BiOCl: reactivity, selectivity, and perspectives, Angew. Chem., Int. Ed., 2017, 57, 122–138 CrossRef PubMed.
- Y. Zhang, Z. Xu, G. Li, X. Huang, W. Hao and Y. Bi, Direct observation of oxygen vacancy self-healingon TiO2 photocatalysts for solar water splitting, Angew. Chem., Int. Ed., 2019, 58, 4229–14233 Search PubMed.
- Z. Zhao, Y. Zhou, F. Wang, K. Zhang, S. Yu and K. Cao, Polyaniline-decorated {001} facets of Bi2O2CO3 nanosheets: In situ oxygen vacancy formation and enhanced visible light photocatalytic activity, ACS Appl. Mater. Interfaces, 2015, 7, 730–737 CrossRef PubMed.
- S. Gao, B. Gu, X. Jiao, Y. Sun, X. Zu, F. Yang, W. Zhu, C. Wang, Z. Feng, B. Ye and Y. Xie, Highly efficient and exceptionally durable CO2 photoreduction to methanol over freestanding defective single-unit-cell bismuth vanadate layers, J. Am. Chem. Soc., 2017, 139, 3438–3445 CrossRef PubMed.
- H. Wang, Q. Chen, Q. Luan, R. Duan, R. Guan, X. Cao and X. Hu, Photocatalytic properties dependent on the interfacial defects of intergrains within TiO2 mesocrystals, Chem. – Eur. J., 2018, 24, 17105–17116 CrossRef PubMed.
- Y. Wang, J. Cai, M. Wu, J. Chen, W. Zhao, Y. Tian, T. Ding, J. Zhang, Z. Jiang and X. Li, Rational constructure of oxygen vacancies onto tungsten trioxide to improve visible light photocatalytic water oxidation reaction, Appl. Catal., B, 2018, 239, 398–407 CrossRef.
- L. Dai, X. Chen, W. Wang, T. Zhou and B. Hu, Growth and luminescence characterization of large-scale zinc oxide nanowires, J. Phys.: Condens. Matter, 2003, 15, 2221–2226 CrossRef.
- X. Chen, S. Guan, J. Zhou, H. Shang, J. Zhang, F. Lv, H. Yu, H. Li and Z. Bian, Photocatalytic free radical-controlled synthesis of high performance single-atom catalysts, Angew. Chem., Int. Ed., 2023, 62, e202312734 CrossRef PubMed.
- J. Zhu, J. Chen, H. Xu, S. Sun, Y. Xu, M. Zhou, X. Gao and Z. Sun, Plasma-introduced oxygen defects confined in Li4Ti5O12 nanosheets for boosting lithium-ion diffusion, ACS Appl. Mater. Interfaces, 2019, 11, 17384–17392 CrossRef PubMed.
- X. Shi, X. Chen, S. Zhou, S. Lou and Y. Wang, PVP assisted hydrothermal synthesis of BiOBr hierarchical nanostructures and high photocatalytic capacity, Chem. Eng. J., 2013, 222, 120–127 CrossRef.
- J. Xu, C. Liu, J. Niu and M. Chen, Preparation of In2S3 nanosheets decorated KNbO3 nanocubes composite photocatalysts with significantly enhanced activity under visible light irradiation, Sep. Sci. Technol., 2020, 230, 115861 Search PubMed.
- Y. Shi, H. Shou, H. Li, G. Zhan, X. Liu, Z. Yang, C. Mao, J. Cheng, X. Zhang, Y. Zhang, S. Zhao, J. Wang, X. Liu, L. Song, H. Sun and L. Zhang, Visible light-driven conversion of carbon-sequestrated seawater into stoichiometric CO and HClO with nitrogen-doped BiOCl atomic layers, Angew. Chem., Int. Ed., 2023, 62, e202302286 CrossRef PubMed.
- Y. Chen, S. H. Guan, H. Ge, X. Chen, Z. M. Xu, Y. H. Yue, H. Yamashita, H. Yu, H. X. Li and Z. F. Bian, Photocatalytic dissolution of precious Metals by TiO2 through photogenerated free radicals, Angew. Chem., Int. Ed., 2022, 61, e202213640 CrossRef PubMed.
- N. Shetti, S. Malode, R. Malladi, S. Nargund, S. Shukla and T. Aminabhavi, Electrochemical detection and degradation of textile dye Congo red at graphene oxide modified electrode, Microchem. J., 2019, 146, 387–392 CrossRef.
- S. Sun, C. Shan, Z. Yang, S. Wang and B. Pan, Self-enhanced selective oxidation of phosphonate into phosphate by Cu(II)/H2O2: performance, mechanism, and validation, Environ. Sci. Technol., 2022, 56, 634–641 CrossRef PubMed.
- Q. Wu, S. Chai, H. Yang, Z. Gao, R. Zhang, L. Wang and L. Kang, Enhancing visible-light driven photocatalytic performance of BiOBr by self-doping and in-situ deposition strategy: a synergistic effect between Bi5+ and metallic Bi, Sep. Purif. Technol., 2020, 253, 117388H CrossRef.
- Y. Wen, J. Chen, X. Gao, W. Liu, H. Che, B. Liu and Y. Ao, Two birds with one stone: Cobalt-doping induces to enhanced piezoelectric property and persulfate activation ability of ZnO nanorods for efficient water purification, Nano Energy, 2023, 107, 108173 CrossRef.
- F. Chen, W. Ma, J. He and J. Zhao, Fenton degradation of malachite green catalyzed by aromatic additives, J. Phys. Chem. A, 2002, 106, 9485–9490 CrossRef.
- X. Liu, W. Hou, Y. Huang, H. Zhao, Z. Song and Y. Huang, Facile and green synthesis of carbon nanopinnacles for the removal of chlortetracycline: performance, mechanism and biotoxicity, Chem. Eng. J., 2022, 433, 133822 CrossRef.
- X. Hou, W. Shen, X. Huang, Z. Ai and L. Zhang, Ascorbic acid enhanced activation of oxygen by ferrous iron: a case of aerobic degradation of rhodamine B, J. Hazard. Mater., 2016, 308, 67–74 CrossRef PubMed.
- Y. Shi, Z. Yang, L. Shi, H. Li, X. Liu, X. Zhang, J. Cheng, C. Liang, S. Cao, F. Guo, X. Liu, Z. Ai and L. Zhang, Surface boronizing can weaken the excitonic effects of BiOBr nanosheets for efficient O2 activation and selective NO oxidation under visible light irradiation, Environ. Sci. Technol., 2022, 56, 14478–14486 CrossRef PubMed.
- J. Cao, Z. Xu, Y. Chen, S. Li, Y. Jiang, L. Bai, Y. Yu, H. Li and Z. Bian, Tailoring the asymmetric structure of NH2-UiO-66 metal-organic frameworks for light-promoted selective and efficient gold extraction and separation, Angew. Chem., Int. Ed., 2023, 62, e202302202 CrossRef PubMed.
- L. Wang, X. Lan, W. Peng and Z. Wang, Uncertainty and misinterpretation over identification, quantification and transformation of reactive species generated in catalytic oxidation processes: a review, J. Hazard. Mater., 2020, 408, 124436 CrossRef PubMed.
- Y. Qin, F. Song, Z. Ai, P. Zhang and L. Zhang, Protocatechuic acid promoted alachlor degradation in Fe(III)/H2O2 Fenton system, Environ. Sci. Technol., 2015, 49, 7948–7956 CrossRef PubMed.
- C. Fang, Z. Hao, Y. Wang, Y. Huang, D. Huang and X. Liu, Carbon nanotube as a nanoreactor for efficient degradation of 3-aminophenol over CoOx/CNT catalyst, J. Cleaner Prod., 2023, 405, 136912 CrossRef.
- N. Alireza and K. Maryam, Decolorization of a binary azo dyes mixture using CuO incorporated nanozeolite-X as a heterogeneous catalyst and solar irradiation, Chem. Eng. J., 2013, 228, 631–641 CrossRef.
- A. Isari, S. Moradi, S. Rezaei, F. Ghanbari, E. Dehghanifard and B. Kakavandi, Peroxymonosulfate catalyzed by core/shell magnetic ZnO photocatalyst towards malathion degradation: Enhancing synergy, catalytic performance and mechanism, Sep. Purif. Technol., 2021, 275, 119163 CrossRef CAS.
- Z. Hao, W. Hou, C. Fang, Y. Huang and X. Liu, Sulfite activation by cobaltosic oxide nanohydrangeas for tetracycline degradation: performance, degradation pathways and mechanism, J. Hazard. Mater., 2022, 439, 129618 CrossRef CAS PubMed.
- X. Liu, Z. Hao, C. Fang, K. Pang, J. Yan, Y. Huang, D. Huang and D. Astruc, Using waste to treat waste: facile synthesis of hollowcarbonnanospheres from lignin for water decontamination, Chem. Sci., 2024, 15, 204–212 RSC.
- Q. Li, J. Ruan, X. Zhang, Y. Wang, H. Zhao, Y. Huang, D. Huang and X. Liu, Spatial Confinement of Co-N-C Catalyst in Carbon Nanocuboid for Water Decontamination: High Performance, Mechanism and Biotoxicity Assessment, Chem. Eng. J., 2024, 479, 147555 CrossRef CAS.
|
This journal is © The Royal Society of Chemistry 2024 |