DOI:
10.1039/D4AN00494A
(Paper)
Analyst, 2024,
149, 3302-3308
Remediate and sense: alginate beads empowered by portable electrochemical strips for copper ion removal and detection at environmental sites
Received
30th March 2024
, Accepted 1st May 2024
First published on 1st May 2024
Abstract
The contamination of environmental sites due to the presence of persistent species represents an important issue to be tackled. In particular, the presence of high levels of metals in soil and surface water is more frequent. One of the metals that sometimes exceeds the permissible limit set by regulatory authorities is copper. For instance, copper-based fungicides are widely used in viticulture. However, copper ions remain in soil and can enter the food chain, posing threats to human health and environmental safety. Although the rapid detection of copper ions using portable sensors is effective in enhancing early warning, it sometimes solves only half of the problem as remediation is not considered. In this paper, we present a novel integrated/portable approach that merges the remediation and sensing of metals by proposing a remediate-and-sense concept. In order to realize this concept, alginate beads were coupled with printed electrochemical strips for on-site copper detection. Within the same architecture, alginate beads were used to remove copper ions from the soil, and printed electrochemical strips were used to evaluate the efficacy of remediation at the point of need. The concept was applied towards soil containing copper ions at the parts per billion level; with few alginate beads and in the absence of additional species, copper ions were quantitatively removed from the matrix; and 3D printing allowed us to combine the printed strips and spheres within a unique tool. The architecture was optimized and the results were compared to inductively coupled plasma-mass spectrometry (ICP-MS) measurements with a recovery percentage of 90%–110%. It should be noted that this novel portable approach may be applied to other pollutants, opening new possibilities for integrated remediation and sensing.
Introduction
Copper (Cu) is classified as an essential trace element as it is required for a variety of metabolic and physiological processes in humans, animals and plants.1 It is associated with bone health, immune function, cardiovascular risk and alterations in cholesterol metabolism.2 It is necessary for human brain development and maturation and functions as a cofactor of key metabolic enzymes and as a signalling and regulatory molecule. The amount of copper ions in organisms affects infant growth, host defence mechanisms and brain development.3,4 Copper is commonly used in agriculture as a standard bactericide/fungicide owing to its low cost, superior efficacy in disease control and relatively low toxicity towards humans.5 The main advantage of copper is its broad spectrum of activity against bacteria such as those causing downy mildew of grape, late blight of potatoes, and secondary diseases in minor crops such as vegetables and special fruits.6 For example, copper compounds are effective against numerous foliar mycoses, particularly those caused by downy mildew, one of the most serious diseases of grapevine worldwide.7 However, the extensive use of copper has resulted in the development of copper-tolerant microorganisms and negative environmental impacts due to its accumulation in soil and water bodies.8 The exposure of crops and vegetables to high levels of copper in soil can cause direct toxicity towards plants, whereas chronic exposure to moderate levels of copper can affect reproductive rates and vegetative growth (e.g. biomass of aerial and underground structures).9 With the aim to limit copper intake, the World Health Organization recommends a maximum of 0.9 mg per day of copper for adults.10 For these reasons, continuous monitoring of copper in agricultural soil and plants is necessary to keep its level under control and increase environmental quality/human wellness. Conventional analytical techniques used for copper analysis are mainly atomic absorption spectroscopy (AAS), inductively coupled plasma-mass spectrometry (ICP-MS) and inductively coupled plasma-optical emission spectroscopy (ICP-OES).11,12 Although these methods are characterized by high performance, sensitivity and accuracy, their major drawbacks lie in the expensive/sophisticated instrumentation, the need for specialized personnel and time-consuming procedures; the requirement for a specialized laboratory limits the application of these approaches for in situ testing/screening.13,14 In this regard, the existence of portable analytical devices that would allow everyone, with a major focus on non-specialized users, to be informed about the pollution and/or the presence of specific species in the environment, might represent an opportunity to monitor ecosystem and consumer health. The design and application of sensors and biosensors is of fundamental importance for this goal, and the use of electrochemical ones is preferable to colorimetric/fluorimetric methods, due to their advantages when coloured/turbid matrices are analyzed; in fact, the electrochemical methods are not affected by measuring non-treated matrices, i.e. blood, soil, and wastewater. With a major focus on the detection of metals, various electrochemical approaches have been reported: Bobrowski et al. evaluated the functionality of a screen-printed carbon electrode coated with an antimony film to detect Cu(II) in treated soil samples, and by differential pulse anodic stripping voltammetry, a detection limit of 0.14 μg L−1 and a linearity up to 150 μg L−1 were obtained.15 Adarakatti et al. designed a screen-printed platform using calixarene to detect lead, copper and mercury ions. The three metals were successfully detected in industrial effluents and wastewater samples, with detection limits of 38, 40 and 48 μg L−1, respectively for lead, copper and mercury.16 Electrodes were also screen-printed onto office paper-based substrates to detect copper in serum samples, down to 4 ppb using gold nanoparticles as the electrode modifier.17 Recently Nguyen et al. have modified a silver-based electrode using MnO2 as a modifier. Copper ions were detected in water sources down to 0.5 nM with a linearity comprised between 0.625–15 nM.18 It is without any doubt that these portable approaches can be of high relevance in environmental and other fields. However, the effectiveness of a decentralized detection system should be empowered by the possibility of remediating an environmental site of interest, and in this regard, several methods have been studied to remove metal pollutants from the environment. Remediation techniques include different approaches such as physical, chemical, and biological methods. Physical methods combine soil substitution and hot desorption processes including washing, soil replacement and isolation, surface capping, electrokinetic remediation and encapsulation. Chemical methods include vitrification, precipitation, ion exchange and adsorption. Biological methods are based on the use of microorganisms such as microbes and plant species, and depending on the site where the waste is removed, they are classified into in situ or ex situ.19,20 Although many previously listed physical, chemical and biological methods have been applied for the removal of heavy metals, chemical adsorption methods have been highlighted as efficient, inexpensive and easy to apply.21 Adsorption is a process in which a solid or liquid substance accumulates molecules or particles of another substance on its surface.22 Chemical adsorption is more popular for heavy metal removal because it has stronger interactions and higher adsorption capacity towards heavy metals.23 With regard to these approaches, the use of alginate, a natural anionic polysaccharide containing free hydroxyl and carboxyl groups, has been highlighted due to its abundance, non-toxicity and biocompatibility.24 The green features of alginate and alginate-based composites have been reported to be applied towards metal removal up to the parts per million level: various applications towards the detection of copper, cadmium, lead, chromium and mercury ions, using alginate as hydrogel beads and also in the presence of chitosan, polyaniline, polypyrrole, graphene, conductive polymers, etc., were reported.25–28 However, even if alginate represents a promising and environment-friendly solution for remediation, the success of metal removal needs to be evaluated by external analytical methods. It should also be considered that an in situ solution would be highly effective for the real-time surveillance of environmental sites. As per the theranostic application in clinical field, i.e. the possibility for diagnosing and providing a therapy for a certain disease using the same platform, a concept similar to remediate-and-sense, would have tremendous impacts, particularly to assure a prompt intervention in case of excessive pollution. In light of this, the goal of the present work was to develop for the first time a combined tool for the removal and sensing of metal ions in environmental matrices, using copper ions as the case of study. Briefly, an integrated device including alginate beads to remediate copper ions and screen-printed electrochemical strips was developed and characterized for its application to liquid and solid matrices, i.e. water and soil. The removal ability of alginate beads was evaluated via the optimization of the amount, size and time of contact with the polluted matrices. In particular, an experimental protocol was developed avoiding complex and invasive procedures, making the entire system a user-friendly platform for use in a decentralized context. The combination with printed-electrochemical strips for the rapid detection of metal ions is of particular importance if the on-site application is considered, and it represents a starting point towards further application in the environmental field, but not limited to this. It can be a starting point for converging towards precision and sustainable agriculture, reducing time and costs for on-site measurements and limiting the use of species that may be harmful to human health and the environment.29,30
Experimental
Chemicals and apparatuses
Conductive inks (Ag/AgCl and graphite) were purchased from Acheson (Milan, Italy), hydrochloric acid and alginic acid sodium salt from brown algae (A0682, low viscosity 4–12 cP for a 1% aqueous solution at 25 °C) from Sigma-Aldrich (St Louis, MO, USA), standard copper solutions (1000 mg L−1) from Fisher Scientific (Milan, Italy), and calcium chloride anhydrous from Merck Co. (Darmstadt, Germany). All reagents were characterized by a purity higher than 98%, as indicated by the producer. Soil was sampled from a garden pot. For the flexible polyester-based substrate, we used Autostat HT5 (125 μm), MacDermid, UK. This substrate was chosen for its robust and flexible characteristics, which are essential for our environmental applications. To insulate the printed electrodes, a clear adhesive tape as used in packaging was used. All measurements were done using a portable potentiostat, EmStat3 (PalmSens, The Netherlands) connected to a laptop.
Fabrication of electrochemical strips
Screen-printed electrodes were produced by manual screen printing on a flexible polyester-based substrate, using conductive inks: Ag/AgCl (Electrodag 477 SS) for the reference electrode and using graphite-based conductive inks (Electrodag 421) for the working and counter electrodes. After each printing step, the electrodes were cured in an oven at 80 °C for 20 min. Adhesive tape (commercially available) was used to insulate the printed electrodes. The diameter of the working electrode was 0.4 cm, and the electrochemical strips were 2.5 cm × 1 cm, respectively length x width.31 The shelf life of the strips is around 1 year if they are stored in vacuum packs away from light and at room temperature.
AuNP synthesis
To improve the analytical performance of the sensor toward copper measurement, gold nanoparticles were used, synthesized using chloroauric acid (HAuCl4) used as a precursor, sodium citrate as a capping agent and sodium boron hydride (NaBH4) as a reducing agent. Before proceeding with the synthesis, all the glassware and magnetic anchor used were washed thoroughly, first with aqua regia (HCl/HNO3 = 3
:
1, v/v) three times, rinsed in double-distilled water, washed with a piranha solution (H2SO4/H2O2 = 7
:
3, v/v) three times, and finally rinsed again with double-distilled water before use; both steps were repeated twice. The synthesis was conducted using 9 mL of double-distilled water to which 1 mL of the 10 mg mL−1 solution of chloroauric acid was added. Next, 2 mL of 10 mg mL−1 sodium citrate was added, and finally, 500 μL of 20 mM of the reducing agent was added dropwise. The reaction took place at room temperature overnight.32,33 Once the synthesis was completed and the gold nanoparticles were obtained, they were analyzed by UV/Vis spectroscopy in order to evaluate the successful synthesis.
Alginate bead synthesis
Calcium alginate beads were prepared by means of ionic cross-linking, starting from a sodium alginate solution (1% w/v) obtained by dissolution in distilled water, under continuous stirring, at room temperature, following a procedure adapted from previous studies.34,35 The obtained solution was loaded in a plastic syringe (Terumo, 5 mL), supplied with a metallic needle (diameter size = 18G or 23G), and then extruded into an anhydrous calcium chloride gelling bath (1.0 M) through an infusion pump (KDScientific, MA, USA). The following parameters were selected: distance between the syringe and gelation media was 3 cm and the flow rate was 10 mL h−1. The produced beads were cured in the gelation medium for around 15 min, under constant stirring, and then taken out and washed several times with distilled water in order to remove possible residual calcium chloride. The average sizes of the obtained beads were measured from the micrographs acquired using an optical microscope, using the ImageJ (NIH) software. The surface of the beads was investigated by field emission scanning electron microscopy (FEG-SEM, Leo Supra 35, UK). The swelling test was carried out by dipping pre-weighed beads in distilled water (1 bead per mL distilled water), within a humidity-controlled incubator (temperature = 37 °C and humidity = 98%). The beads were extracted at regular intervals (5 to 360 minutes), cleaned superficially to remove surface water, accurately weighed, and observed using a digital microscope (Jusion 40) at selected time points. The swelling percentage (SP) was determined using the following expression: SP = (Mt–M0) x 100/M0, where M0 and Mt are the initial mass and mass at time t, respectively. The measurements were made in triplicate, and the average data were provided.
Cu(II) measurements
Linear sweep-anodic stripping voltammetry (LS-ASV) was performed for copper detection, which first involves using a deposition potential to reduce copper in the solution and then oxidizing it. Measurements were conducted using a 0.1 M hydrochloric acid solution as the working solution, which was also used as the supporting electrolyte for copper detection. The use of an acid is necessary because the analysis of stripping is performed in the presence of acidic media, which are necessary to have metals in their oxidized forms.36 In anodic stripping voltammetry, the anodic peak potential for the re-oxidation of copper ions was observed around 0.3 V vs. Ag/AgCl. The experimental parameters were optimized as follows: a deposition potential of −0.4 V was applied for 300 s, in the potential range from 0.1 to 0.6 V, with an E step of 0.01 V and a scan rate of 0.5 V s−1.17
ICP-MS measurements
In order to evaluate the trueness of the platforms, all the samples were analyzed by ICP-MS. Briefly, samples were mineralized: 250 microliters were transferred into a 7 mL Pyrex. Hydrochloric acid and nitric acid were added to each sample (3
:
1 v/v). The reaction was conducted for 16 h at 90 °C, and after digestion the samples were diluted to 15 mL in ultrapure water. Measurements were performed using an Agilent 7700 ICP-MS instrument (Agilent Technologies) equipped with a frequency-matching radio frequency (RF) generator and a 3rd-generation Octopole Reaction System (ORS3) operating with helium gas in ORF. The following parameters were used: RF power = 1550 W; plasma gas flow = 14 L min−1; carrier gas flow = 0.99 L min−1; He gas flow = 4.3 mL min−1. In this experiment, 103Rh was used as an internal standard. Lead and cadmium concentrations were measured with three replicates.
Remediate-and-sense
In order to realize a unique platform for both remediating and sensing copper ions in environmental matrices, i.e., water and soil, an easy and integrated approach was adopted. Briefly, the chosen environmental matrix was allowed to enter into contact with the alginate beads without any treatment, i.e. acid addition, heating, etc., and consequently a printed strip was used to in situ monitor the presence of copper ions, as displayed in Fig. 1.
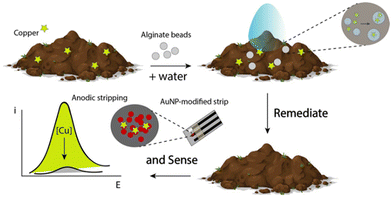 |
| Fig. 1 Architecture mechanism of the remediate-and-sense concept carried out in soil samples. | |
Results and discussion
AuNP-modified printed strips for copper detection
As reported in our previous findings, copper ions were successfully detected in biological matrices using paper-based electrochemical strips.17 In this case, a flexible substrate made of polyester was used to realize the printed strips: the choice was due to the need for flexibility and mechanical robustness for in situ application in environmental settings. However, even if the role of AuNPs was demonstrated to be fundamental for the analysis of copper ions, and other ionic species, e.g. mercury, arsenic, and iron, it should be noted that the deposition of AuNPs might change among different substrates, due to different porosities of conductive inks that are printed. The first investigation was about the modification of the strip surface with various amounts of AuNPs. As observed in the inset i of Fig. 2, by carrying out cyclic voltammetry experiments in the presence of 5 mM potassium ferricyanide in a 0.1 M potassium chloride solution, it appeared obvious how the presence of AuNPs improved the performance of the system. The surface of the strip was modified with different volumes of gold nanoparticles, namely 2 and 8 μL, and it was clear how their presence was effective with the increase in the electrochemical performance, as demonstrated by the higher intensity of peaks and the lower potential difference between the oxidation and reduction peaks. In particular, the potential difference is a good indicator of the quality during the electrochemical process of electron exchange at the electrode surface: as a result, 2 μL was selected as the optimal compromise among electrochemical performance, time to modification and amount of modifiers. The next step involved the evaluation of the analytical performance in the presence of copper ions in a wide range of concentrations, namely up to 400 ppb. The anodic stripping voltammetry (ASV) was adopted to obtain a calibration curve under standard conditions, using 0.1 M hydrochloric acid as the working solution.
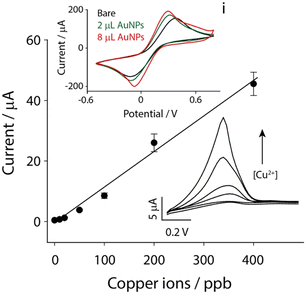 |
| Fig. 2 Calibration curve for copper ions in a standard solution, i.e. 0.1 M HCl, obtained by modifying the electrochemical strips with 2 μL of AuNPs. Experimental parameters: E dep = −0.4 V; t dep = 300 s; E begin = 0.1 V; E end = 0.6 V; E step = 0.01 V; scan rate = 0.5 V s−1. The voltammetric curves relating to the measured concentrations for copper are also reported. Inset i: Cyclic voltammograms for the evaluation of the optimal amount of AuNPs, between 2 and 8 μL. The measurements were conducted in triplicate in the presence of 5 mM potassium ferricyanide in a 0.1 M potassium chloride solution. | |
As reported in Fig. 2, a linear correlation was obtained between the height of the current peaks and the concentration of copper ions. The relationship is described using the following equation: y = 0.12x − 0.88, R2 = 0.983, (where y represents the current difference between metal ions and the blank solution, and x represents the metal ion level expressed in ppb).
The limit of detection (LOD), calculated as 3σB/slope (σB is the standard deviation calculated for several blank measurements and the slope is relative to y/x in the calibration curve), was found to be ca. 1 ppb, while the limit of quantification (LOQ) was found to be ca. 4 ppb, with a relative standard deviation (RSD%) lower than 10%.
Alginate beads as remediating agents
In order to evaluate the capability of alginate beads to remediate copper ions, two different types of alginate beads, obtained using two different needles (18G and 23G), were used, and named 18G alginate beads and 23G alginate beads, respectively. In particular, the size and amount of alginate beads, including the time of interaction between copper ions and beads, were evaluated.
Namely, the remediation efficacy of alginate beads 18G and 23G was investigated in the presence of a 100 ppb solution of copper ions dissolved in water. In detail, different numbers of beads (from 5 to 20) were used to identify the optimal density to be used for successive remediation studies, as shown in Fig. 3A and B. Before evaluation, the beads were allowed to dry for approximately 40 minutes at room temperature in contact with an adsorbent pad. Successively, various numbers of beads, between 5 and 20, were put in contact with 200 microliter of a 100 ppb copper ion solution.
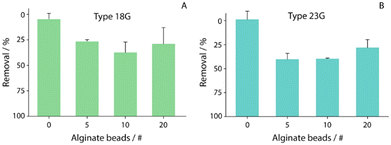 |
| Fig. 3 Optimization of the size and quantity of alginate beads placed in contact with a 100 ppb copper ion solution. (A) Evaluation of the ability of 18G alginate beads to remediate copper ions, using different bead quantities, ranging from 5 to 20. (B) Evaluation of the ability of 23G alginate beads to remediate copper ions using different bead quantities, ranging from 5 to 20. | |
For the first evaluation, 180 min interaction among copper ions and alginate beads was considered (the duration was chosen after assessing the removal capability within a wide range of times, data not shown). The efficacy of copper removal was studied through the adoption of stripping voltammetry after the sampled solution was acidified to pH 1 with hydrochloric acid. The 23G alginate beads showed a slightly better removal efficiency than that of the 18G ones, perhaps due to the average size (1.75 ± 0.02 mm vs. 2.75 ± 0.02 mm, respectively), while for both types of beads, 10 of them resulted with the highest removal, with percentages of ca. 40 and 50, respectively for 18G and 23G.
According to these findings, further studies were performed using the 23G alginate beads. As far as the formation of copper [CuCl4]2− complexes is concerned, this is more likely at higher concentrations of hydrochloric acid. At concentrations of 0.1 M, the probability of forming [CuCl4]2− complexes is low compared to solutions with much higher HCl concentrations. However, it is important to note that the formation of such complexes can still occur to some extent, particularly under certain conditions such as high temperatures or long reaction times. The predominance of [CuCl4]2− complexes generally increases with the increase in HCl concentration.37
Alginate bead morphology
Following the identification of the optimal alginate beads, they were characterized. It should be noted that homogeneous beads were achieved, and in the performed swelling tests, the alginate beads showed slow water absorption in the initial phase, whereas, after 3 hours, there was a drastic increase in water absorption and in the next 2 hours the beads reached their maximum swelling. In detail, they were characterised by a maximum water absorption of 250%, reaching an equilibrium between 240 minutes and 360 minutes, with the increase in size as high as 60% of the initial diameter (Fig. 4A). In addition, the surface of the produced beads was homogenous and rough, promoting the metal adsorption, as evident from the acquired SEM micrograph displayed in Fig. 4B.
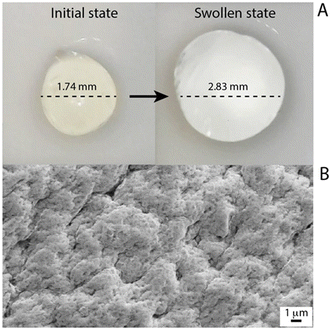 |
| Fig. 4 (A) Micrographs of the alginate beads in the initial state (left) and the swollen state. (B) SEM images of the alginate bead surface. | |
Remediate-and-sense in soil samples
The developed system that combines the possibility of removing copper ions and evaluating the performance of remediation in real time with an integrated strip was applied towards contaminated soil. However, before evaluating the performance for remediation, the electrochemical strip performance obtained in the standard solution was compared with those obtained in soil. In particular, a gram of soil was acidified with 5 mL of 0.1 M hydrochloric acid. Successively, a fraction was used for the direct measurement, while the remaining amount of acidified soil was filtered using a syringe with a 0.45 μm membrane, to remove the gross impurities. As reported in Fig. 5, all the “blank” solutions were spiked with the increasing amount of copper ions in the 0–80 ppb range, to evaluate the difference in sensitivity for future applications.
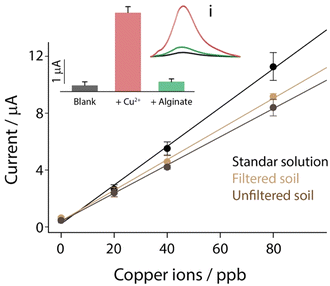 |
| Fig. 5 Study of the effect of the soil matrix on the sensitivity of the electrochemical strip. Three different calibration curves were obtained for copper ions in the range of 0 to 80 ppb in the standard solution, i.e. 0.1 M HCl, filtered and unfiltered soil. All measurements were carried out in triplicates. Inset: Comparison of the detected copper ions in three different samples: blank soil, 50 ppb copper-spiked soil and 50 ppb copper-spiked soil with the addition of 10 alginate beads. Inset i: Voltammetric curves related to the measured concentrations of copper. | |
By observing Fig. 5, it should be noted that a slight decrease in sensitivity was obtained by comparing the measurements carried out with and without soil, and this is ascribed to the presence of a complex matrix like soil. In particular, the sensitivity obtained in a standard solution of 0.1 M hydrochloric acid was equal to 0.14 μA ppb−1, while the sensitivity obtained after soil contact were equal to 0.11 and 0.10 μA ppb−1, respectively to filtered and unfiltered soils. All measurements were carried out in triplicate, and all the correlations were characterized by an R2 value of 0.993. All the metals inside the soil were quantified by ICP-MS measurements, and the presence of copper ions was not high enough to be detected by the present method. Successively the optimized type and amount of alginate beads were applied to copper-spiked soil as a proof of concept. Prior to start, the soil was spiked with 50 ppb of copper ions. Successively 10 alginate beads were added to the soil together with distilled water. Only water and alginate beads were used to evaluate the removal activity in the presence of contaminated soil. As reported in the inset of Fig. 5, three samples were compared: blank soil, 50 ppb copper-spiked soil and 50 ppb copper-spiked soil with the addition of 10 alginate beads. After two hours of interaction, the solution was taken without filtration and the pH was adjusted to 1 using hydrochloric acid on the strip, avoiding any contact with the soil. What has been achieved was a quantitative removal, which reported the signals for spiked soil similar to those obtained for blank samples, and using 3D printing, it was possible to design and realize an ad hoc case for the complete integration of the remediate-and-sense platform into a unique and compact device, as shown in Fig. 6.
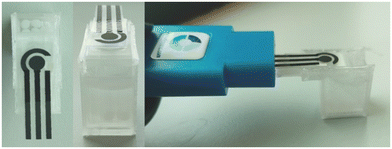 |
| Fig. 6 Three-dimensional printed case for the integration of the printed electrode and alginate spheres. | |
In addition, diverse spiking of copper ions was used in the soil, with levels between 50 and 200 ppb, and the efficiency of the approach of remediate-and-sense was evaluated by ICP-MS analyses of spiked soil, as shown in Table 1, demonstrating the satisfactory trueness of the method.
Table 1 Correlation among ICP-MS and electrochemical strips of various Cu-spiked soil concentrations and apparent recoveries of the two methods
Spiked concentration (ppb) |
ICP-MS (ppb) |
ICP-MS app. recovery (%) |
E-strip (ppb) |
E-strip app. recovery (ppb) |
Correlation (%)a |
Correlation % was calculated as (E-strip (ppb)/ICP-MS (ppb)) × 100.
|
50 |
50 ± 1 |
100 |
55 ± 5 |
110 |
110 |
100 |
102 ± 2 |
102 |
97 ± 8 |
97 |
95 |
150 |
149 ± 3 |
99 |
140 ± 11 |
93 |
94 |
200 |
205 ± 3 |
102 |
220 ± 20 |
110 |
107 |
Conclusions
The present work highlighted the opportunity to combine a remediation strategy with a portable analytical tool, to realize the so-called remediate-and-sense architecture. The approach was obtained by merging the efficacy of alginate microbeads and printed electrochemical strips to remediate copper ions and evaluate the efficacy with electrochemical measurements, respectively. The efficacy of alginate beads was evaluated by characterizing different-sized beads, using copper ions as the metal model to be remediated. In particular, the system was applied to a complex environmental matrix like soil. As a proof of concept, spiked soil was quantitatively remediated using just 10 alginate microbeads, avoiding complex tasks and invasive procedures. In addition, the analytical performance of the strip was satisfactorily evaluated by a complementary analysis of ICP-MS, with the correlation between ca. 95 and 110%. This study represents the first step towards the development of a fully printed analytical platform, where alginate might represent the substrate to screen-print electrochemical strips. A widely possible application can be made with this new architecture; in particular, it can represent a promising starting point for applications combined with pollutant removal methods, in order to converge towards precision and sustainable agriculture, limiting the use of species that may be harmful to human and environmental health.
Conflicts of interest
There are no conflicts to declare.
References
- X. Wang, M. F. de Souza, M. J. Mench, H. Li, Y. S. Ok, F. M. G. Tack and E. Meers, Environ. Pollut., 2022, 294, 118627 CrossRef CAS PubMed
.
- M. Bost, S. Houdart, M. Oberli, E. Kalonji, J.-F. Huneau and I. Margaritis, J. Trace Elem. Med. Biol., 2016, 35, 107–115 CrossRef CAS PubMed
.
- S. Lutsenko, C. Washington-Hughes, M. Ralle and K. Schmidt, J. Biol. Inorg. Chem., 2019, 24, 1179–1188 CrossRef CAS PubMed
.
- M. Tarnowska and T. Krawczyk, Biosens. Bioelectron., 2020, 169, 112614 CrossRef CAS PubMed
.
- J. Pereira, A. King, M. G. N. Campos and S. Santra, Curr. Nanosci., 2022, 18, 410–424 CrossRef CAS
.
- L. Tamm, B. Thuerig, S. Apostolov, H. Blogg, E. Borgo, P. E. Corneo, S. Fittje, M. de Palma, A. Donko, C. Experton, É. Alcázar Marín, Á. M. Pérez, I. Pertot, A. Rasmussen, H. Steinshamn, A. Vetemaa, H. Willer and J. Herforth-Rahmé, Agronomy, 2022, 12, 673 CrossRef CAS
.
- S. Dagostin, H.-J. Schärer, I. Pertot and L. Tamm, Crop Prot., 2011, 30, 776–788 CrossRef CAS
.
- J. R. Lamichhane, E. Osdaghi, F. Behlau, J. Köhl, J. B. Jones and J.-N. Aubertot, Agron. Sustainable Dev., 2018, 38, 28 CrossRef
.
- Z. Shabbir, A. Sardar, A. Shabbir, G. Abbas, S. Shamshad, S. Khalid, Natasha, G. Murtaza, C. Dumat and M. Shahid, Chemosphere, 2020, 259, 127436 CrossRef CAS PubMed
.
-
W. H. Organization and WHO, Guidelines for Drinking-water Quality, World Health Organization, 2004 Search PubMed
.
- L. A. Malik, A. Bashir, A. Qureashi and A. H. Pandith, Environ. Chem. Lett., 2019, 17, 1495–1521 CrossRef CAS
.
- M. Mittal, K. Kumar, D. Anghore and R. K. Rawal, Curr. Drug Discovery Technol., 2017, 14, 106–120 CrossRef CAS PubMed
.
- J. A. Buledi, S. Amin, S. I. Haider, M. I. Bhanger and A. R. Solangi, Environ. Sci. Pollut. Res., 2021, 28, 58994–59002 CrossRef PubMed
.
- A. P. F. Turner, ECS Sens. Plus, 2022, 1, 011601 CrossRef
.
- A. Bobrowski, M. Maczuga, A. Królicka, E. Konstanteli, C. Sakellaropoulou and A. Economou, Anal. Lett., 2017, 50, 2920–2936 CrossRef CAS
.
- P. S. Adarakatti, C. W. Foster, C. E. Banks, N. S. Arun Kumar and P. Malingappa, Sens. Actuators, A, 2017, 267, 517–525 CrossRef
.
- A. Miglione, M. Spinelli, A. Amoresano and S. Cinti, ACS Meas. Au, 2022, 2, 177–184 CrossRef CAS PubMed
.
- H. T. T. Nguyen, N. T. H. Chu and H. T. T. Le, J. Chem., 2023, 2023, e3855430 Search PubMed
.
- S. Rajendran, T. A. K. Priya, K. S. Khoo, T. K. A. Hoang, H.-S. Ng, H. S. H. Munawaroh, C. Karaman, Y. Orooji and P. L. Show, Chemosphere, 2022, 287, 132369 CrossRef CAS PubMed
.
- C. Li, K. Zhou, W. Qin, C. Tian, M. Qi, X. Yan and W. Han, Soil Sediment Contam.: Int. J., 2019, 28, 380–394 CrossRef CAS
.
- M. Ince and O. K. İnce, Int. J. Pure Appl. Sci., 2017, 3, 10–19 CrossRef
.
- K. C. Khulbe and T. Matsuura, Appl. Water Sci., 2018, 8, 19 CrossRef
.
- T. S. Vo, M. M. Hossain, H. M. Jeong and K. Kim, Nano Convergence, 2020, 7, 36 CrossRef CAS PubMed
.
- Z. Xiao, L. Zhang, L. Wu and D. Chen, J. Taiwan Inst. Chem. Eng., 2019, 102, 110–117 CrossRef CAS
.
- R. R. Pawar, Lalhmunsiama, P. G. Ingole and S.-M. Lee, Int. J. Biol. Macromol., 2020, 164, 3145–3154 CrossRef CAS PubMed
.
- I. Ayouch, I. Barrak, Z. Kassab, M. El Achaby, A. Barhoun and K. Draoui, Environ. Technol. Innovation, 2020, 20, 101157 CrossRef CAS
.
- M. Kuczajowska-Zadrożna, U. Filipkowska and T. Jóźwiak, J. Environ. Chem. Eng., 2020, 8, 103878 CrossRef
.
- W. Zhang, J. Ou, B. Wang, H. Wang, Q. He, J. Song, H. Zhang, M. Tang, L. Zhou, Y. Gao and S. Sun, J. Hazard. Mater., 2021, 418, 126358 CrossRef CAS PubMed
.
- S. C. Teixeira, N. O. Gomes, M. L. Calegaro, S. A. S. Machado, T. V. de Oliveira, N. de F. F. Soares and P. A. Raymundo-Pereira, Biomater. Adv., 2023, 155, 213676 CrossRef CAS PubMed
.
- S. C. Teixeira, N. O. Gomes, T. V. de Oliveira, P. Fortes-Da-Silva, N. de F. F. Soares and P. A. Raymundo-Pereira, Biosens. Bioelectron.: X, 2023, 14, 100371 CAS
.
- A. Raucci, A. Miglione, M. Spinelli, A. Amoresano and S. Cinti, J. Electrochem. Soc., 2022, 169, 037516 CrossRef CAS
.
- V. Ortone, L. Matino, F. Santoro and S. Cinti, Chem. Commun., 2021, 57, 7100–7103 RSC
.
- C. D. De Souza, B. R. Nogueira and M. E. C. M. Rostelato, J. Alloys Compd., 2019, 798, 714–740 CrossRef
.
- I. Cacciotti, C. Ceci, A. Bianco and G. Pistritto, Mater. Sci. Eng., C, 2017, 81, 32–38 CrossRef CAS PubMed
.
- M. Barbieri, F. Cellini, I. Cacciotti, S. D. Peterson and M. Porfiri, J. Mater. Sci., 2017, 52, 12506–12512 CrossRef CAS
.
- S. Cinti, D. Talarico, G. Palleschi, D. Moscone and F. Arduini, Anal. Chim. Acta, 2016, 919, 78–84 CrossRef CAS PubMed
.
- T. Sato, M. Ito, T. Sakamoto and R. Otsuka, Hydrometallurgy, 1987, 18, 105–115 CrossRef CAS
.
|
This journal is © The Royal Society of Chemistry 2024 |
Click here to see how this site uses Cookies. View our privacy policy here.