DOI:
10.1039/D3SC01088K
(Perspective)
Chem. Sci., 2023,
14, 5827-5841
Lanthanide molecular cluster-aggregates as the next generation of optical materials
Received
27th February 2023
, Accepted 10th May 2023
First published on 10th May 2023
Abstract
In this perspective, we provide an overview of the recent achievements in luminescent lanthanide-based molecular cluster-aggregates (MCAs) and illustrate why MCAs can be seen as the next generation of highly efficient optical materials. MCAs are high nuclearity compounds composed of rigid multinuclear metal cores encapsulated by organic ligands. The combination of high nuclearity and molecular structure makes MCAs an ideal class of compounds that can unify the properties of traditional nanoparticles and small molecules. By bridging the gap between both domains, MCAs intrinsically retain unique features with tremendous impacts on their optical properties. Although homometallic luminescent MCAs have been extensively studied since the late 1990s, it was only recently that heterometallic luminescent MCAs were pioneered as tunable luminescent materials. These heterometallic systems have shown tremendous impacts in areas such as anti-counterfeiting materials, luminescent thermometry, and molecular upconversion, thus representing a new generation of lanthanide-based optical materials.
1. Introduction
Over the past few decades, the study of luminescent materials based on trivalent and divalent lanthanide ions has garnered much attention, with successful applications in diverse fields such as lighting, bioimaging, and the development of luminescent probes for temperature, ions, pH, pressure, and other analytes.1–35 Despite numerous reports published in this field, it remains an effervescent research topic with new developments reported frequently, including new applications and/or improved performance.
Although not restricted to nanoparticles36–40 and molecular complexes,41–45 both classes of materials represent the most studied systems for optical applications, each with its own merits and limitations. For example, much of the success in using nanoparticles relies on fine tunability of the composition due to the large number of metal ions within the crystalline lattice, enabling optimization of the optical properties through precise control of composition, with the main drawback still being the lack of homogeneity with size distribution. As a result, the observed property attained will be the result of different smaller parts. On the other hand, molecular compounds offer atomic-level control due to their well-defined structure. However, the limited number of metal ions in such compounds restricts the effectiveness of energy-transfer (ET) processes between different lanthanides, thereby limiting the possibilities for controlling optical output.
In this perspective, we will highlight an emerging class of lanthanide materials, molecular cluster-aggregates (MCAs), for their optical applications. We will discuss the advantages of this class of materials in terms of composition, ET, and control over their optical output. Our focus will be on recent achievements in the luminescence of trivalent lanthanide-based MCAs. Furthermore, we will not delve into the synthetic strategies46–50 or the theoretical aspects of lanthanides luminescence51–55 but rather we summarize and highlight some of the recent achievements in this area.
This article starts with a brief discussion about lanthanide-based MCAs (Section 2) and trivalent lanthanide luminescence (Section 3). We subsequently focused our attention on homometallic luminescent MCAs (Section 4) before discussing the impacts and potential that heterometallic MCAs could have in some applications which require effective control of ET processes, such as luminescent thermometers and anti-counterfeiting materials (Section 5). We conclude this perspective by providing an outlook on possible future directions and their potential implications for the field of optical materials.
2. Lanthanide-based molecular cluster-aggregates
Lanthanide-based MCAs are composed of rigid multinuclear metal cores encapsulated by organic ligands.46–50,56–73 These systems are arranged in a highly ordered structure in a crystal lattice, presenting a high uniformity due to the molecular origin. The nuclearity of MCAs can range from a few to hundreds of metal ions (Fig. 1), with larger ones comparable to the ultrasmall nanoparticles in size.74–78 In the late 90s, lanthanide-based MCAs gained attention due to their aesthetic beauty, remarkable physical properties such as luminescence and magnetism, as well as the synthetic challenge of producing large nuclearity systems.
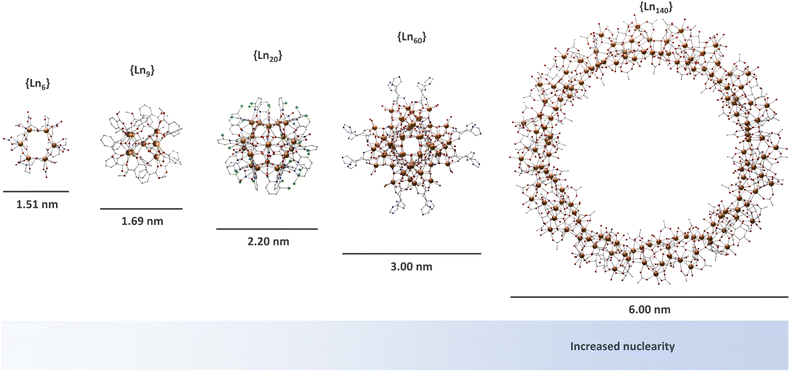 |
| Fig. 1 Molecular structure of selected MCAs. {Ln6} (CCDC number: 1958091);79{Ln9} (CCDC number: 956639);80{Ln20} (CCDC number: 2023766);81{Ln60} (CCDC number: 2152485);82 and {Ln140} (CCDC number: 1569507).83 Colour code: Dark orange: Ln3+; grey: carbon; red: oxygen; blue: nitrogen; light green: chlorine. Hydrogen atoms were omitted in all structures for clarity. | |
For the latter, Staples, Zheng, and co-workers paved the way in the study of the synthetic coordination chemistry of lanthanides at high pH by pioneering the control of hydrolysis and halide-templating in a pentadecanuclear lanthanide system.84–86 This resulted in new strategies for producing high-nuclearity MCAs with a certain degree of control. Since then, several research groups have successfully reported intriguing and impressive giant complexes such as {Er60},82,87–90{Nd104},91 and {Gd140},83 mostly being developed by Long, Zheng, Kong and co-workers.
Structurally, MCAs can be viewed as a merging of the nanoparticle and molecular complex realms (Scheme 1). The sizes and high nuclearities resemble those observed for ultrasmall nanoparticles while maintaining high homogeneity in size and number of metal ions due to the molecular nature. Therefore, achieving high performance and fine-tunability in the composition akin to nanoparticles is possible while alleviating issues related to size distribution.
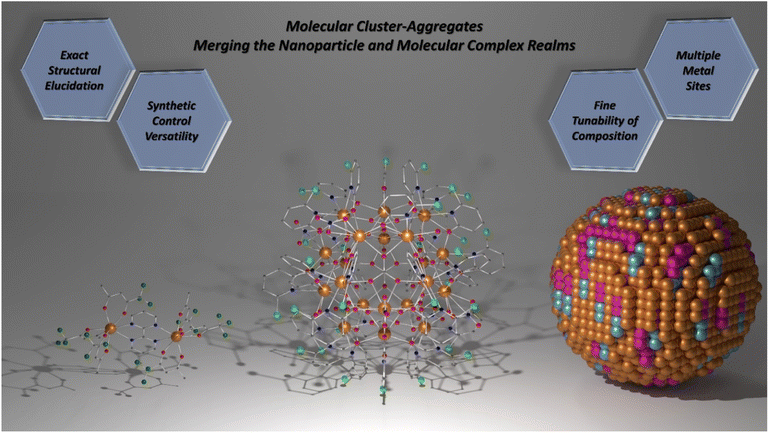 |
| Scheme 1 Molecular cluster-aggregates. The union between molecular complex and nanoparticle worlds. Left: [Dy2(bpm)(tfaa)6] complex (CCDC number: 1859276).98 Centre: {Eu20} MCA (CCDC number: 2023766).81 Right: Representation of a heterometallic spherical nanoparticle. Colour code for left and centre: dark orange: Ln3+; grey: carbon; red: oxygen; blue: nitrogen; light green: chlorine; dark green: fluoride. Hydrogen atoms were omitted in all structures for clarity. For the nanoparticle representation, colours were randomly chosen and have no meaning. | |
From the application perspective, earlier high-nuclearity MCAs were primarily designed for use as molecular magnetic coolers.62–70 Little attention was paid to exploring their potential as highly efficient optical materials. However, in recent years, our research group has been focused on developing heterometallic MCAs for various applications, unlocking the vast potential of this class of compounds.79,81,92–96
3. Luminescence of trivalent lanthanides
Lanthanides ions are well known for their remarkable and versatile luminescent properties. In this perspective article, a detailed discussion of lanthanide luminescence theory is out of our scope. For this topic, readers can find specialized and detailed reviews.51–55 We intend to marginally describe some essential features that will appear in examples discussed in Sections 4 and 5. Except for Ce3+ and some divalent lanthanide ions (such as Eu2+ and Yb2+), the typical luminescence observed for most trivalent lanthanide ions (Ln3+) originates from electronic transitions within the partially filled 4fn orbitals. An essential feature of lanthanides is the shielded nature of the 4f orbitals by the 5s25p6 subshells, thus resulting in an inner orbital character. This remarkable feature is responsible for the unique optical properties of the lanthanides and results in particularly sharp absorption and emission lines characteristic of each lanthanide. In terms of energetic position, the 4f–4f transitions are thus little sensitive to the external environment and have a fingerprint for different lanthanide ions. The characteristic emissions of the Ln3+ ions span from the ultraviolet-(UV) to the near-(NIR) and mid-infrared range of the electromagnetic spectrum, according to the individual ladder-like structure (Fig. 2) and are characterized by relatively long lifetimes (in the order of micro to milliseconds).
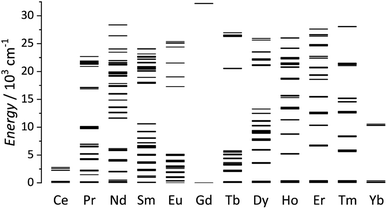 |
| Fig. 2 Partial energy-levels diagram for trivalent lanthanides. Energies obtained from Carnall.99 | |
Another crucial aspect of the 4f–4f transitions is the parity-forbidden nature of these transitions. Laporte's rule forbids intraconfigurational electric dipole transitions, such as 4f–4f.97 Consequently, due to the low transition probabilities, the absorption cross-sections for lanthanide ions are much smaller than other luminescent species. Hence, the relaxation of the selection rules is pivotal for making the 4f–4f transitions “less forbidden”.
This can be achieved by placing the lanthanide ion in a non-centrosymmetric coordination environment, resulting in mixing electronic and vibrational wavefunctions. Even though the wavefunctions mixing makes the 4f–4f transitions less forbidden, transition probabilities remain weak with molar absorption coefficients much smaller than 100 M−1 cm−1.
Considering this, some strategies have been developed to effectively harvest the energy to the emitter levels of each lanthanide. Some of the approaches relevant to understanding the examples in Sections 4 and 5 will be briefly discussed here.
When Ln3+ ions are placed in close proximity to each other, ET processes could be efficiently controlled to modulate the optical output.100–108 This is an easy task for nanoparticles, with hundreds to tens of thousands of metal ions, but more limited for low nuclearity molecular complexes. Since efficient ET transfer is at the crux of the upconversion (UC) mechanism (vide infra),109–112 it is easy to understand the supremacy of nanoparticles over molecular UC.
3.1 Antenna effect
The antenna effect, also known as ligand-mediated intramolecular ET, was first demonstrated in the seminal studies of Weissman and Crosby.113–115 This strategy relies on using an organic compound, which usually has a much larger absorption cross-section to absorb light and transfer it to Ln3+ ions (Fig. 3). Briefly, the ligand absorbs the light, populating the excited singlet states (Sn). An intersystem crossing (ISC) occurs, thus populating the ligand triplet state (T). Subsequently, the energy is transferred to the Ln3+ excited states that can radiatively emit via the individually characteristic 4f–4f transitions. From the ligand side, two critical conditions are required for an efficient ligand-to-Ln3+ ET to occur:
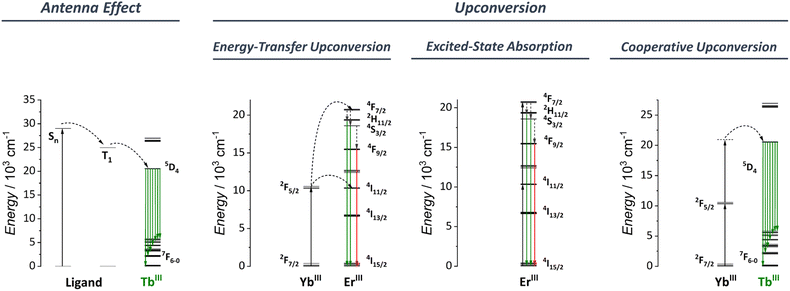 |
| Fig. 3 Depiction of some commonly observed luminescence mechanisms for Ln3+ ions. From left to right: Ligand-based antenna effect, Yb3+-Er3+-based energy-transfer upconversion, Er3+-based excited-state absorption, and Yb3+-Tb3+-based cooperative upconversion. | |
(1) An efficient ISC process: For this, an energy gap of at least 5000 cm−1 between the ligand S1 and T1 is required.116–118
(2) An efficient T1 to Ln3+ ET process: According to Latva's empirical rules, the optimal gap between the ligand T1 state and Ln3+ emitter level is around 2500–4000 cm−1. Energetic differences above 4000 cm−1 do not allow an efficient ET. Energetic differences below 2500 cm−1 result in a strong back energy-transfer (BET) from the Ln3+ ion to the ligand.119
3.2 Upconversion
Upconversion (UC) is an intriguing phenomenon involving the sequential absorption of two or more low-energy photons resulting in the emission of higher-energy photons. Unlike the nonlinear optics processes such as second-harmonic generation, UC is based on the cumulative effects of multiple first-order absorption processes such as excited-state absorption (ESA), energy transfer upconversion (ETU), and cooperative upconversion (CUC), showing a higher efficiency than the nonlinear processes.
Bloembergen first observed the phenomenon of upconversion in 1959,120 but it wasn't until the pioneering research of Auzel,121 Ovsyankin, and Feofilov122 in 1966 that an appropriate understanding and explanation was developed. Since then, UC has been extensively studied in low-phonon solid materials and nanoparticles, with applications in a variety of fields, including bioimaging,123–128 photodynamic therapy,129–133 optogenetics,134–138 upconversion lasing,139–143 super-resolution microscopy,144–148 luminescence thermometry,149–158 anti-counterfeiting materials,159–163 and many others.
Fig. 3 shows a simplified diagram for some of the UC mechanisms relevant to the discussion in Section 5. This perspective does not focus on reviewing the different UC mechanisms. For this, we recommend that readers to consider the review article by Auzel.109
Until the early 2000s, studies of the upconversion phenomena were mainly focused on Ln3+-based nanoparticles and low-phonon solid materials. Since then, some groups have started investigating the UC process in low-nuclearity molecular compounds to overcome some intrinsic issues inherent to nanoparticles (vide supra). Despite the success of the earlier works in observing UC in molecular species, the use of low-nuclearities compounds resulted in low upconversion quantum yields (UCQY),164–170 much below the values observed for nanoparticles and low-phonon solid materials.171–173
3.3 Why luminescent MCAs?
As mentioned in Section 2, MCAs represent the union of two of the most explored classes of lanthanide materials for luminescence, nanoparticles and molecular complexes. As such, the benefits of both classes can be attained in a single unit by using MCAs. Due to the chemical similarities, it is possible to replace one lanthanide with another within these molecular entities, thus, modifying the chemical composition, and consequently, the ET processes as seen for NPs.100–108 However, at the same time, the molecular nature allows for the precise determination of the lanthanide local symmetry/distortions, which is not entirely possible and understood through the huge surface metal sites in nanoparticles. Consequently, higher uniformity/homogeneity in the optical properties is expected for different synthetic batches of MCAs and at an individual level. Additionally, the presence of organic ligands as a constituent part of the MCA structure makes it possible for an efficient antenna effect without needing surface modification.
4. Optical properties of homometallic MCAs
The study of luminescent homometallic MCAs dates to the late 90s and early 2000s. The first reported example is related to a pentanuclear europium MCA assembled via a ligand-controlled hydrolysis using dibenzoylmethane (Hdbm) as the ligand.174 In this work, the Eu3+ ion luminescence was used only as a structural probe to confirm the observations that all five Eu3+ ions possess the exact local symmetry. Since then, several examples of luminescent homometallic with the most diverse nuclearities have been reported (Table 1). Most of these works report the presence of luminescence on the MCAs; however, some are important in understanding the potential of lanthanide-based MCAs as luminescent materials and will be discussed in this section. It should be noted that homometallic MCAs as luminescent materials are less attractive in application potential, as almost no differences will be observed compared to low-nuclearity molecular complexes. All the attractiveness of using MCAs as luminescent materials relies on the possibility of controlling the composition and ET processes (see next section).
Table 1 Summary of homometallic luminescent MCAsa
|
Capping ligand |
Emitter ion |
Lifetime/μs |
Quantum yield/% |
Reference |
PepCO2 = 2-[{3-(((tert-butoxycarbonyl)amino)methyl)benzyl}amino]acetic acid. L1 = 3,3′-((1E,1′E)-((4,5-dimethyl-1,2-phnylene)bis(azaneylylidene))bis(methaneylylidene))bis(benzene-1,2-diol).
|
{Ln5} |
Dibenzoylmethane |
Eu3+ |
0.06 |
— |
174
|
{Ln5} |
Dibenzoylmethane |
Eu3+ |
— |
— |
183
|
{Ln5} |
Dibenzoylmethane |
Tb3+ |
— |
— |
183
|
{Ln5} |
Dibenzoylmethane |
Nd3+ |
8.29 |
— |
184
|
{Ln5} |
Dibenzoylmethane |
Yb3+ |
9.95 |
— |
184
|
{Ln6} |
Triethanolamine |
Er3+ |
— |
— |
79
|
{Ln8} |
2,2,6,6-Tetramethylheptane-3,5-dione |
Eu3+ |
— |
— |
183
|
{Ln8} |
2,2,6,6-Tetramethylheptane-3,5-dione |
Tb3+ |
— |
— |
183
|
{Ln9} |
Benzoylacetone |
Eu3+ |
— |
— |
176
|
{Ln9Na10} |
5′-Methyl-2,2′-bipyridyl-6-phosphonic acid |
Eu3+ |
2370, 240 |
32 |
185
|
{Ln9Na10} |
5′-Methyl-2,2′-bipyridyl-6-phosphonic acid |
Yb3+ |
9.3 |
0.22 |
185
|
{Ln9} |
Hexylsalycilate |
Tb3+ |
1200 |
90 |
179
|
{Ln9} |
Hexylsalycilate |
Yb3+ |
2, 0.6 |
— |
179
|
{Ln9} |
Acetylacetonate |
Eu3+ |
— |
— |
183
|
{Ln9} |
Acetylacetonate |
Tb3+ |
— |
— |
183
|
{Y9-nLnn} |
Acetylacetonate |
Eu3+ |
— |
— |
186
|
{Ln9} |
2-(Hydroxymethyl)pyridine |
Eu3+ |
— |
— |
187
|
{Ln9} |
Methyl 4-methylsalicylate |
Tb3+ |
815 |
31 |
180
|
{Ln9} |
Methyl 5-methylsalicylate |
Tb3+ |
190 |
2.4 |
180
|
{Ln9} |
Methylsalicylate |
Tb3+ |
264 |
6.7 |
180
|
{Ln1Gd8} |
Butylsalicylate |
Tb3+ |
960 |
14 |
188
|
{Ln2Gd7} |
Butylsalicylate |
Tb3+ |
1060 |
23 |
188
|
{Ln5Gd4} |
Butylsalicylate |
Tb3+ |
1160 |
33 |
188
|
{Ln8Gd1} |
Butylsalicylate |
Tb3+ |
1170 |
40 |
188
|
{Ln9} |
Butylsalicylate |
Tb3+ |
1160 |
39 |
188
|
{Ln1Gd8} |
Butylsalicylate |
Yb3+ |
0.57 |
— |
189
|
{Ln3Gd6} |
Butylsalicylate |
Yb3+ |
0.56 |
— |
189
|
{Ln7Gd2} |
Butylsalicylate |
Yb3+ |
0.57 |
— |
189
|
{Ln1Lu8} |
Butylsalicylate |
Yb3+ |
0. 74 |
— |
189
|
{Ln3Lu6} |
Butylsalicylate |
Yb3+ |
0.63 |
— |
189
|
{Ln7Lu2} |
Butylsalicylate |
Yb3+ |
0.61 |
— |
189
|
{Ln9} |
Butylsalicylate |
Yb3+ |
0.60 |
— |
189
|
{Ln13} |
(Ph4Si4O8)4− |
Eu3+ |
884 |
50 |
181
|
{Ln14} |
Acetylacetonate |
Tb3+ |
— |
— |
190
|
{Ln14} |
Acetylacetonate |
Dy3+ |
— |
— |
190
|
{Ln14} |
2,3-Dihydroxybenzaldehyde |
Yb3+ |
7.83 |
0.24 |
191
|
{Ln14} |
2,3-Dihydroxybenzaldehyde |
Nd3+ |
|
|
192
|
{Ln15} |
PepCO2 and dibenzoylmethane |
Eu3+ |
— |
16 |
182
|
{Ln15} |
PepCO2 and dibenzoylmethane |
Tb3+ |
— |
<3 |
182
|
{Ln18} |
L1 and 2,3-dihydroxybenzaldehyde |
Yb3+ |
9.11 |
0.16 |
193
|
{Ln18} |
L1 and 2,3-dihydroxybenzaldehyde |
Nd3+ |
6.44 |
0.17 |
194
|
{Ln18} |
L1 and 2,3-dihydroxybenzaldehyde |
Eu3+ |
— |
— |
195
|
{Ln20} |
3,5-Bis[3-(pyrid-2-yl)-1,2,4-triazolyl]-pyridine |
Tb3+ |
605 |
— |
196
|
{Ln20} |
Chloro-2-pyridinol |
Eu3+ |
— |
— |
81
|
{Ln20} |
Chloro-2-pyridinol |
Tb3+ |
1314, 454, 107 |
55.8 |
81
|
{Ln42} |
2-Hydroxy-3-methoxybenzaldehyde |
Nd3+ |
6.37 |
0.76 |
197
|
{Ln42} |
2-Hydroxy-3-methoxybenzaldehyde |
Yb3+ |
11.65 |
0.20 |
198
|
{Ln42} |
2-Hydroxy-3-methoxybenzaldehyde |
Sm3+ |
15.65 |
0.33 |
198
|
{Ln42} |
2-Hydroxy-3-methoxybenzaldehyde |
Eu3+ |
22.8 |
1.74 |
199
|
{Ln42} |
2-Hydroxy-3-methoxybenzaldehyde |
Tb3+ |
— |
<0.1 |
199
|
{Ln48} |
2-Thiophenecarboxylic acid |
Eu3+ |
284 |
8.40 |
200
|
{Ln48} |
3-Furancarboxylic acid |
Tb3+ |
905 |
3.11 |
200
|
In 2020, our group reported the first demonstration of NIR-to-NIR emission on a water-soluble Er3+-based MCA.79 The [Er6(teaH)6(NO3)6] exhibit a wheel-like molecular structure in which each Er3+ is encapsulated by one teaH2− (doubly deprotonated triethanolamine) and one nitrate anion. We demonstrated the water stability via NMR of the Y3+ analogue and by TGA, powder X-ray, and FTIR analysis of the {Er6} MCA powder before and after a solubilization/drying procedure. By exciting the MCA with a 980 nm laser, we observed the characteristic telecom range 4I13/2 → 4I15/2 transition band centred at approximately 1530 nm. This was an exciting result as NIR-to-NIR emissions are critical for biomedical imaging, telecommunications, photonics and optoelectronics fields.175
Among all the possible nuclearities, nonanuclear hourglass-like MCAs are the most studied in luminescence. These robust systems can be seen as two square pyramids sharing the apical vertex (Fig. 4) and are easily synthesized, stable in several solvents, and the most attractive fact is that they can be prepared with different encapsulating ligands, promoting a toolbox to investigate the impact of other organic moieties on the MCAs luminescent properties.
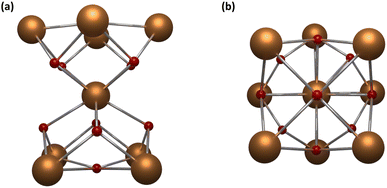 |
| Fig. 4 Metal core structure for {Ln9} MCAs. (a) Side view and (b) top view. CCDC number: 956639.80 Colour code: Dark orange: Ln3+; red: oxygen. Hydrogen atoms were omitted in the structure for clarity. | |
The first luminescent nonanuclear MCA was reported by Yan and co-workers in 2002.176 These MCAs, [Ln9(μ4-O)2(μ3-OH)8(μ-BA)8(BA)8]−[HN(CH2CH3)3]+·(CH3OH)2(CHCl3) (Ln = Sm3+, Eu3+, Gd3+, Dy3+, and Er3+) were assembled using benzoylacetone (BA) as the ligand. However, luminescent properties were reported only for the {Eu9} congener. This outcome is not surprising, as the triplet state of BA is positioned at around 21
700 cm−1,177,178 which makes it an ideal energetic position to sensitize Eu3+ ions, but not others.
On the other hand, in 2007, Kushida and co-workers reported Tb3+ and Yb3+ homometallic {Ln9} MCAs with the same metal core topology with hexylsalicylate as the capping ligand.179 The triplet state of this ligand is located approximately at 23
200 cm−1, 2800 cm−1 above the Tb3+ 5D4 emitter level, thus, acting as a highly efficient antenna for Tb3+ sensitization. Consequently, an impressive emission quantum yield of 90% was observed. For the {Yb9} analogue, the NIR emission was observed but too weak to allow a complete study.
Posteriorly, Hasegawa and co-workers published a report describing the same nonanuclear core-structure surrounded by methylsalycilate, methyl 4-methylsalicylate, and methyl 5-methylsalicylate.180 The triplet state of these ligands occurs at lower energies than the previous example (hexylsalicylate) and the energetic proximity of the triplet state and Tb3+ emitter level results in a more pronounced back-ET, and consequently, lower quantum yields.
These previous works show the elegance of the nonanuclear hourglass-like MCAs. By changing the capping ligand, while keeping the same metal-core structure, the first system is optimal for sensitizing Eu3+ while the second one acts as a better sensitizer for Tb3+ ions. The study of luminescent homometallic {Ln9} MCAs were further extended for systems containing several other ligands (Table 1).
In 2022, Sun and co-workers reported a {Eu13} nanocage exhibiting a Keggin-like structure, prepared with a highly symmetric (Ph4Si4O8)4− capping ligand.181 This MCA has a similar fourfold symmetry as that of the macrocyclic tetrasilane and shows potential for application in optical, catalysis, and photoelectric fields. The rigid structure in which the Eu3+ atoms are bridged by O2− rather than the OH− bridges commonly observed for MCAs results in a higher quantum yield (50%) due to the reduced vibrational quenching pathways.
In 2013, Roesky and co-workers described a pentadecanuclear system as the first MCA prepared with peptoids as supporting ligands for nanoscale bio-compatible applications.182 The [Ln15(μ3-OH)20(PepCO2)10(DBM)10Cl]4+ MCA is assembled via heterocubane subunits organized in a pentagonal ring arrangement. The photoluminescence studies of Eu3+ and Tb3+-based systems showed the expected emission in the visible range, and their applicability as probes in cell culture was evaluated using time-resolved luminescent microscopy. In vitro studies with HeLa tumour cells revealed an accumulation of the clusters in the endosomal-lysosomal system with moderate cytotoxicity for these cells.
Recently, our group started studying one of the most versatile MCA for optical applications, {Ln20}.81 This icosanuclear MCA consists of a rigid spherical metal-core surrounded by chloro-2-pyridinol (chp) ligands with the [Ln20(chp)30(CO3)12(NO3)6(H2O)6] chemical formula. This MCA resembles ultrasmall nanoparticles of size with a diameter of approximately 2.2 nm. Within the cluster, lanthanide ions are located in 4 different coordination sites with different local pseudo-symmetries (two distinct D3h sites, one Cs and one C4v site). We proved the solubility and stability of this MCA in acetonitrile and methanol by means of NMR and DLS techniques. The chp ligand triplet state is located up to 24
961 cm−1, enabling an efficient ET for Tb3+ ions but not for Eu3+ and Sm3+. The quantum yield for {Tb20} in solid-state was determined as 55.8% due to the efficient sensitization via chp ligands. The heterometallic {Ln20} MCAs represent a milestone in the optical applications of MCAs, as will be discussed in the next section.
Over the last few years, Schipper and Jones groups have developed nanoring-shaped homometallic MCAs with different nuclearities for optically sensing several analytes.191–195,197–199 Some remarkable examples include {Yb14}, {Yb18}, and {Nd42} for explosives sensing, {Nd14}, {Nd18}, and {Yb18} as antibiotics probes, {Nd14} for Cu2+, Co2+, H2PO4−, and F− detection, {Eu18} and {Yb18} for anthrax biomarkers sensing. These MCAs highlight the potential that this class of compound holds in the sensors field. However, as will be discussed in Section 5, we truly believe that studying heterometallic compositions for these nanorings could have more impact in consolidating MCAs as efficient optical materials.
More recently, Bu and co-workers reported the {Eu48} and {Tb48}, the largest luminescent MCA to date.200 These MCAs feature a nanopillar-like structure constructed from Ln3 triangles and Ln5 square pyramid units. The red and green characteristic emissions from Eu3+ and Tb3+ display a temperature-dependent behaviour over the 88–298 K range, which opens the possibility of using such nanoarchitecture as luminescent thermometers.
As discussed in this section, several examples homometallic luminescent MCAs are reported in the literature. While some unique and innovative systems have been reported, we believe that the true potential of MCAs can only be fully realized through heterometallic structures, which will be discussed in the following section.
5. Optical properties of heterometallic MCAs
Unlike the homometallic systems highlighted above, the use of heterometallic MCAs for optics is in its early stages, with only limited examples reported and discussed in this section. In addition to being intriguing, heterometallic MCAs are more appealing due to the ability to amalgamate different lanthanide ions, regulate ET processes and tuning emission properties for various applications.
The initial illustration of luminescent heterometallic MCA features the well-known nonanuclear hourglass-like MCA with acac− as the hydrolysis-limiting ligand.201 By synthesizing MCAs with varying Eu3+/Tb3+ ratios, the authors tuned the emission colour output. Despite the authors not fully recognizing its potential, this work marked the beginning of harnessing the power of heterometallic lanthanide-based MCAs for luminescence applications and stands as a milestone achievement in the field.
Inspired by this previous research, we questioned whether we could manipulate lanthanide–lanthanide ET processes within a single MCA unit.
To answer this question, we started our journey into heterometallic MCAs with the hexanuclear wheel, [Er3Yb3(teaH)6(NO3)6] (Fig. 5a). As aforementioned, the {Er6} wheel is the first example of a water-soluble MCA showing NIR-to-NIR emissions.79 In the same work, we demonstrated that by replacing three Er3+ atoms with Yb3+, an 85% enhancement of the NIR emission could be attained in the heterometallic {Er3Yb3} wheel owing to improved absorption of the 980 nm laser by Yb3+ ions (Fig. 5b). Furthermore, by changing the solvent to deuterated water, a significant emission increase was achieved, suggesting a strong interaction between the Er3+ ions with the OH oscillators from the solvent and pointing out that bulkier ligands would be more efficient in shielding the metal core from the external environment.
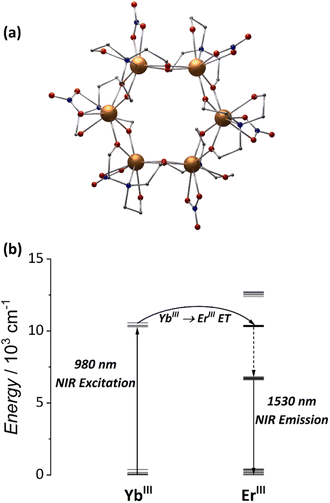 |
| Fig. 5 (a) Single-crystal structure for {Ln6} MCAs (CCDC number: 1958091). (b) Energy-transfer mechanism for the NIR-to-NIR emission.79 Colour code: Dark orange: Ln3+; grey: carbon; red: oxygen; blue: nitrogen. Hydrogen atoms were omitted in the structure for clarity. | |
Aiming to obtain molecular UC with MCAs, we synthesized a series of pentadecanuclear MCAs containing Yb3+ (absorber), Er3+ (emitter), and Y3+ (optically silent) ions.92 In these MCAs, 2,2-dimethylolpropionic acid (Hdmp) ligand was used to encapsulate the pentadecanuclear metal core (Fig. 6) in which the lanthanide ions are distributed in three different local symmetries (D4d, D2d, and C2v geometries in a ratio of 1
:
2
:
2). However, similarly to the teaH2− ligand in the {Er3Yb3} MCA, the use of Hdmp ligand do not effectively prevents interaction with the environment and the molecular UC was only observed at solid-state. Nevertheless, the UC quantum yields (UCQY) obtained in this work were unprecedented for the molecular UC field, reaching values of 10−4 to 10−3%. Although low, before this work, the maximum values obtained for UCQY were below 10−6%; hence, this work was pivotal in understanding the superiority of MCAs as molecular upconverters compared with low-nuclearity complexes.
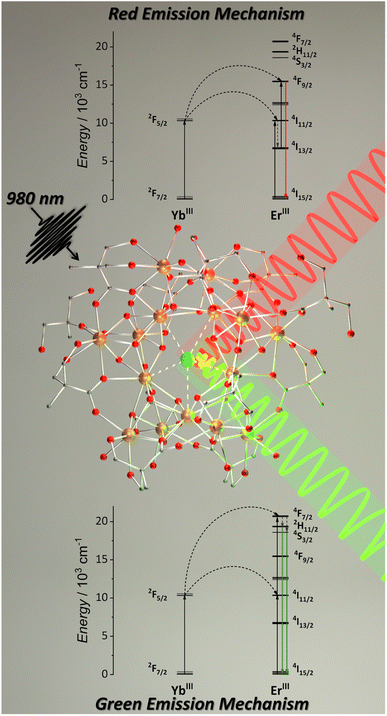 |
| Fig. 6 Single-crystal structure for {Ln15} MCAs (CCDC number: 2023378) and mechanism for red and green UC emissions.92 Colour code: Dark orange: Ln3+; grey: carbon; red: oxygen. Hydrogen atoms were omitted in the structure for clarity. | |
More specifically, in this work, we synthesized five different compositions, {Er2Yb13}, {Er10Yb5}, {Y10Er1Yb4}, {Y13Er2}, and {Y10Er5}. The first composition was synthesized to ensure an excess of donor atoms in relation to acceptors. The second composition aimed the opposite. The third composition added Y3+ as an optically inactive atom to distance the optically active atoms. The last two compositions were synthesized containing only Er3+ as an optically active ion targeting to evaluate the possibility of Er3+-based ESA UC. These last two compositions do not show any emission upon excitation at 980 and 808 nm, confirming the absence of the ESA mechanism. The green and red emission components from Er3+ were observed for the other three compositions, occurring via the ETU mechanism (Fig. 6). The MCAs show extremely high UCQY compared to other molecular upconverters. The values obtained were 8.3 × 10−3% for {Y10Er1Yb4}, 1.5 × 10−3 for {Er2Yb13}, and 2.8 × 10−4 for {Er10Yb5}. These results indicate that the higher UCQY value observed for {Y10Er1Yb4} is due to the separation between the optically active ions, reducing the possibility of migration and concentration quenching within the MCA, thus, highlighting the impact of composition control when aiming molecular UC with MCAs.
Simultaneously with the previous work, Charbonnière and co-workers investigated the molecular UC in nonanuclear hourglass-like MCAs.202 By synthesizing the [TbYb8(acac)16(OH)10] MCA, they reported the first MCA showing the CUC mechanism with a UCQY of 1 × 10−5%. More recently,203 by changing the composition to [Tb4Yb5(acac)16(OH)10] and deuterating the ligands, solvent, and the OH− bridges, the overall performance was significantly improved (UCQY = 2.8 × 10−4%). In this elegant study, the authors report, for the first time in solution, cooperative upconversion of luminescence with the {Yb9} MCA. This process results in an emission band at 503 nm when excited by a 980 nm light source.
As previously discussed, our team has made a significant contribution to the field by focusing studying of the {Ln20} class of MCAs (Fig. 7). In our initial investigation with heterometallic {Ln20} MCAs, we successfully isolated a range of Eu3+/Tb3+-based compounds, varying in composition from {Eu1Tb19} to {Eu6Tb14}.81 This allowed us to fine-tune the emission colour output from green to red. This work clearly shows the impact that MCAs can have on the anticounterfeiting materials field, with the development of optical barcodes with the emission colour output finely tuned within the same MCA unit. The success in achieving this resides in how the composition control impacts the Tb3+ to Eu3+ ET processes. The ET efficiency is more than double in {Eu6Tb14} than observed for {Eu2Tb18} due to the increased number of acceptors within the same MCA unit. We have also been able to observe Tb3+ to Sm3+ ET by exploring {Sm4Tb16}, {Sm10Tb10}, and {Sm16Tb4} compositions, each one containing different ratios between Sm3+ and Tb3+ emission components. Additionally, by finely controlling the ratio of Eu3, Gd3+, and Tb3+ atoms and precisely selecting a suitable excitation energy (355 nm) we have been able to obtain white-light emission with the {Eu3Gd5Tb12} composition due to a favoured blue emission component from the chp ligand when adding Gd3+ to the MCA.
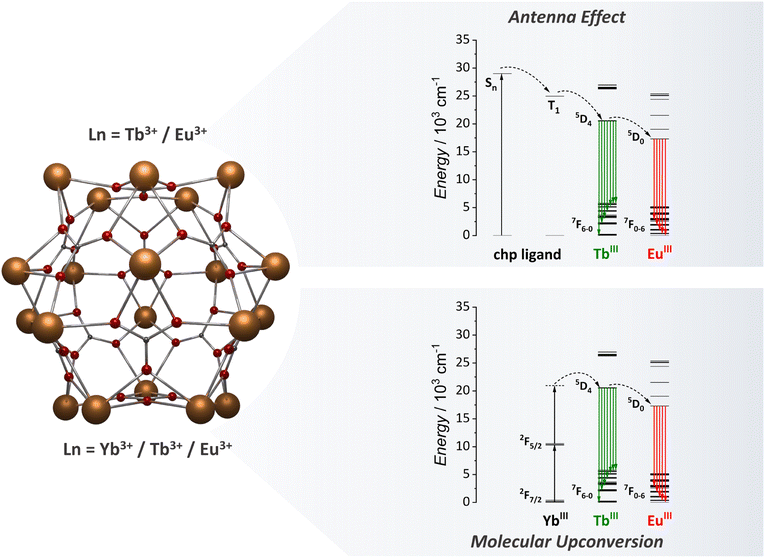 |
| Fig. 7 Metal core structure of the {Ln20} MCAs (CCDC number: 2023766)81 and the energy transfer mechanism for antenna effect and UC emissions.81,95 Colour code: Dark orange: Ln3+; grey: carbon; red: oxygen. Hydrogen atoms were omitted in the structure for clarity. | |
We further explored the Tb3+ to Eu3+ ET in {Ln20} MCAs for luminescence thermometry applications.93 We synthesized {Ln20} MCAs with four different compositions in which we kept the Eu3+/Tb3+ ratios constant (4
:
1). The {Eu4Tb16}, {Eu3Gd5Tb12}, {Eu2Gd10Tb8}, and {Eu1Gd15Tb4} compositions were synthesized, and the temperature-dependent emission properties were evaluated in an acetonitrile solution (0.1 mg mL−1). The increasing amount of Gd3+ atoms aimed for a physical distancing between the donor (Tb3+) and acceptor (Eu3+) atoms while keeping the ratios constant. Our results indicated a decrease in the Tb3 to Eu3+ ET efficiency from 86.7% for {Eu4Tb16} to 60.5% for {Eu1Gd15Tb4} MCA. This reduction of the ET efficiency negatively impacted the thermometric performance. For {Eu4Tb16}, a maximum relative sensitivity of 4.17% °C−1 was obtained, decreasing to 1.82% °C−1 for {Eu1Gd15Tb4}.
In this work, we also compared the photophysical and thermometric performances of {Eu4Tb16} in solution and solid-state, which shows no difference, suggesting a high composition homogeneity and proving that the ET processes operate solely within the MCA unit. The relative sensitivity obtained for these {Ln20} MCAs are in the same range as other Ln3+-based molecular luminescent ratiometric thermometers;204–219 however, the fact that the ET process occurs within the same cluster unit is a great advantage, as it allows us to control the performance at an individual cluster level.
More recently, our group used this same system to explore a new luminescence thermometry approach, the time-gated luminescence thermometry.96 We take advantage of the Tb3+ to Eu3+ ET processes and different decay dynamics for {Eu5Tb15} MCA to implement a strategy in which a pulsed lamp is used to excite the sample and various delays were employed on the detection channel. This promising new approach that can help move from laboratory equipment to real-life applications by eliminating any background emission that can plague the luminescence thermometry implementation. This approach also resulted in a 250% enhancement of the relative thermal sensitivity for {Eu5Tb15} MCA, from 2.79 to 9.78% °C−1.
After exploring the ligand-based sensitization for {Ln20} MCAs and understanding the composition control impact for optical barcoding81 and luminescence thermometry,93 we moved one step further, targeting the use of {Ln20} as molecular upconverters.95 To tap into this mechanism, we synthesized {Gd11Tb2Yb7}, aiming for a cooperative UC mechanism (Fig. 7). For this system, Yb3+ acts as the absorber (donor) species, Tb3+ as the emitter ion, and Gd3+ as an optically silent ion to guarantee a distancing of Tb3+ ions within the structure. Upconverted emission was successfully observed upon a 980 nm laser excitation. The rigid spherical shape of the {Ln20} MCA, which resembles spherical nanoparticles, enabled the reduction of molecular vibrations, minimizing possible vibrational mediated quenching pathways, thus resulting in a UCQY of 1.04 × 10−4%. Intending further to expand the possibilities in the molecular UC field, we target the demonstration of luminescence thermometry obtained via the molecular UC demonstrated in this work. It should be noted that prior to this work, molecular UC works were limited to demonstrating the presence of the mechanism, and there was no previous report of potential applications. To tap into the luminescence thermometry demonstration, we replaced some of the Gd3+ ions with Eu3+ intending to access the Yb3+ → Tb3+ → Eu3+ ET process (Fig. 7). Four different compositions were synthesized, namely, {Eu8Gd3Tb2Yb7}, {Eu9Gd2Tb2Yb7}, {Eu10Gd1Tb2Yb7}, and {Eu11Tb2Yb7}, and the temperature dependent UC was measured. We have observed considerable changes in the ratios of Tb3+ and Eu3+ emission bands and thermometric performances, reaching a maximum relative sensitivity of 3.05% °C−1 for {Eu9Gd2Tb2Yb7} MCA. The success in implementing molecular UC-based luminescence thermometry with {Ln20} opens new avenues for molecular upconverters.
After observing Yb3+/Tb3+-based cooperative UC with some of the {Ln20} MCAs, we decide to explore the Yb3+/Ho3+ pair (Fig. 8).94 Although widely demonstrated for nanoparticles, glasses, and other classes of low-phonon materials,220–224 Ho3+ UC was never observed with a molecular system. The main reason is the mismatching between Yb3+ (donor) and Ho3+ (acceptor) electronic states. In this work, we have shown for the first-time molecular UC (Fig. 8a) and molecular NIR-to-NIR emission (Fig. 8b) based on the Yb3+/Ho3+ pair. The UC process occurs via the ETU mechanism with the absorption of two 980 nm photons, thus populating Ho3+ green emitter states (5S2 and 5F4). Photons can be emitted by this state or non-radiatively decay to the red emitter state (5F5). Additionally, we proved that molecular vibrations are not always the villains for molecular UC with the νC
O and/or νC
Caromatic at ∼1595 cm−1 helping to bridge the gap between donor and acceptor levels (Fig. 8c and d) and allowing the energy-transfer UC mechanism. For the {Gd8Ho2Yb10} MCA, a UCQY of 5.24 × 10−6% was observed, thus expanding the molecular UC possibilities by adding another emitter ion as a potential candidate.
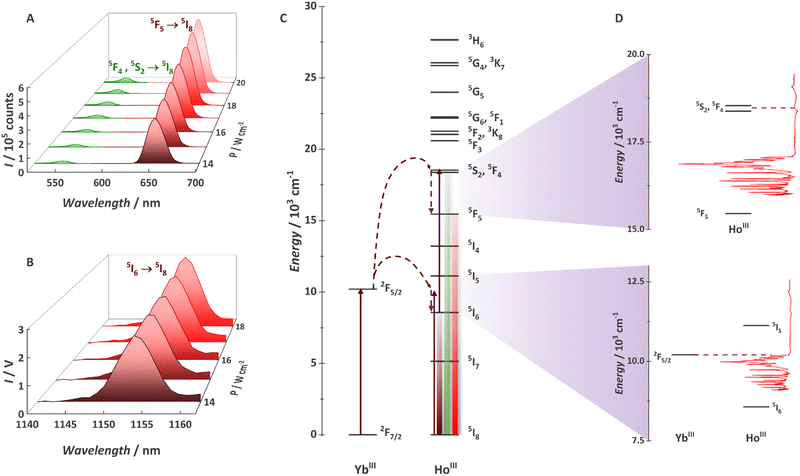 |
| Fig. 8 (A) Upconversion spectra for a 0.1 mg mL−1 deuterated methanol solution of {Gd8Ho2Yb10} obtained at 20 °C with the incident laser power (λexc = 980 nm/νex = 10 204 cm−1) focused on a spot size of ∼0.03 cm2. (B) NIR-to-NIR spectra of {Gd8Ho2Yb10}. (C) Partial energy level diagram for the MCA showing YbIII and HoIII levels. (D) Coupling between the vibration and electronic levels. Adapted from ref. 94 with permission from the Royal Society of Chemistry. | |
These previous works highlight the versatility of heterometallic MCAs as highly efficient optical materials. The {Ln20} MCA has been demonstrated as an impressive toolbox for both ligand-sensitized and UC luminescence, with a significant impact in applications which require fine control of ET processes such as luminescent thermometry and anti-counterfeiting materials.
6. Conclusions and outlook
In this perspective, we highlighted recent advances in using high-nuclearity lanthanide-based MCAs as efficient optical materials. Our research group has shown that these materials have the potential to be the next generation of highly emissive compounds with unprecedented composition control and fine tuning of energy transfer (ET) process. This precise control of ET has significant implications in attaining high-performance luminescent materials, expanding the possibilities of applications in which colour output and/or ET processes play pivotal roles as luminescent thermometers and optical barcodes for anti-counterfeiting applications.
As discussed in Section 4 and summarized in Table 1, there are a small, but much higher number of examples related to homometallic luminescent MCAs. Further exploration and consolidation of this class of compounds could be achieved by evaluating these systems with mixed metal strategy. We believe that some of these underexplored homometallic systems, could result in excellent materials for luminescence thermometry, optical barcodes, and molecular upconverters upon investigation of their heterometallic counter parts.
In the field of molecular UC, MCAs already represent a turning point. The rigid metal core structure and the multiple metal ions enabled four orders of magnitude enhancement for UCQY when compared with low-nuclearity molecular upconverters, approaching the UCQY values observed for ultrasmall nanoparticles.
As in the case of all emerging fields, much more can and needs to be done to its consolidation. Up to now, the highest nuclearity explored for heterometallic luminescent MCAs is twenty. Efforts should be focused on understanding whether higher nuclearities can be beneficial or detrimental to the ET processes within the metal core. From one side, one can expect that more metal in the core will allow greater control of the ET and colour outputs, being valuable for thermometry and anti-counterfeiting applications. However, the great majority of highest-nuclearity MCAs (60 and higher metal ions) show a hollow structure with a void space on the centre of the cluster; thus, it is also possible that increasing the nuclearities in hollow MCAs can result in a detrimental distancing of the optically active ions, resulting in a poorer ET efficiency. Therefore, systematic studies with higher nuclearity heterometallic MCAs will dictate the future directions of the field in the coming years.
Data availability
As a perspective article, no new experimental or computational data are included.
Author contributions
All authors equally contributed to the manuscript preparation and writing.
Conflicts of interest
There are no conflicts to declare.
Acknowledgements
We thank the University of Ottawa, the CFI, and the NSERC for financial support of this work.
References
- J.-C. G. Bünzli and S. V. Eliseeva, Chem. Sci., 2013, 4, 1939 RSC
.
- A. de Bettencourt-Dias, Dalton Trans., 2007, 2007, 2229 RSC
.
- X. Qin, X. Liu, W. Huang, M. Bettinelli and X. Liu, Chem. Rev., 2017, 117, 4488 CrossRef CAS PubMed
.
- Z. Xia and Q. Liu, Prog. Mater. Sci., 2016, 84, 59 CrossRef CAS
.
- S. V. Eliseeva and J.-C. G. Bünzli, Chem. Soc. Rev., 2010, 39, 189 RSC
.
- J.-C. G. Bünzli, Trends Chem., 2019, 1, 751 CrossRef
.
- X. Dong Wang, O. S. Wolfbeis and R. J. Meier, Chem. Soc. Rev., 2013, 42, 7834 RSC
.
- L. H. Fischer, G. S. Harms and O. S. Wolfbeis, Angew. Chem., Int. Ed., 2011, 50, 4546 CrossRef CAS PubMed
.
- M. L. Cable, J. P. Kirby, H. B. Gray and A. Ponce, Acc. Chem. Res., 2013, 46, 2576 CrossRef CAS PubMed
.
- C. D. S. Brites, P. P. Lima, N. J. O. Silva, A. Millán, V. S. Amaral, F. Palacio and L. D. Carlos, Nanoscale, 2012, 4, 4799 RSC
.
- C. D. S. Brites, S. Balabhadra and L. D. Carlos, Adv. Opt. Mater., 2019, 7, 1801239 CrossRef
.
- A. Ćirić, S. Stojadinović and M. D. Dramićanin, J. Lumin., 2019, 216, 116749 CrossRef
.
- C. D. S. Brites, P. P. Lima, N. J. O. Silva, A. Millan, V. S. Amaral, F. Palacio and L. D. Carlos, New J. Chem., 2011, 35, 1177 RSC
.
- P. Netzsch, M. Hämmer, E. Turgunbajew, T. P. van Swieten, A. Meijerink, H. A. Höppe and M. Suta, Adv. Opt. Mater., 2022, 10, 2200059 CrossRef CAS
.
- A. A. Ansari, A. K. Parchur, M. K. Nazeeruddin and M. M. Tavakoli, Coord. Chem. Rev., 2021, 444, 214040 CrossRef CAS
.
- J. Rocha, C. D. S. Brites and L. D. Carlos, Chem.–Eur. J., 2016, 22, 14782 CrossRef CAS PubMed
.
- G. Brunet, R. Marin, M.-J. Monk, U. Resch-Genger, D. A. Gálico, F. A. Sigoli, E. A. Suturina, E. Hemmer and M. Murugesu, Chem. Sci., 2019, 10, 6799 RSC
.
- D. A. Gálico, R. Marin, G. Brunet, D. Errulat, E. Hemmer, F. A. Sigoli, J. O. Moilanen and M. Murugesu, Chem.–Eur. J., 2019, 25, 14625 CrossRef PubMed
.
- R. M. Diaz-Rodriguez, D. A. Gálico, D. Chartrand, E. A. Suturina and M. Murugesu, J. Am. Chem. Soc., 2022, 144, 912 CrossRef CAS PubMed
.
- F. M. Cabral, D. A. Gálico, I. O. Mazali and F. A. Sigoli, Inorg. Chem. Commun., 2018, 98, 29 CrossRef CAS
.
- D. Parker, Coord. Chem. Rev., 2000, 205, 109 CrossRef CAS
.
- C. M. G. dos Santos, A. J. Harte, S. J. Quinn and T. Gunnlaugsson, Coord. Chem. Rev., 2008, 252, 2521 Search PubMed
.
- S. Shinoda and H. Tsukube, Analyst, 2011, 136, 431 RSC
.
- B. V. Harbuzaru, A. Corma, F. Rey, J. L. Jordá, D. Ananias, L. D. Carlos and J. Rocha, Angew. Chem., Int. Ed., 2009, 48, 6476 CrossRef CAS PubMed
.
- A. M. Kaczmarek, Y. -Y. Liu, C. Wang, B. Laforce, L. Vincze, P. Van Der Voort, K. Van Hecke and R. Van Deun, Adv. Funct. Mater., 2017, 27, 1700258 CrossRef
.
- E. J. New, D. Parker, D. G. Smith and J. W. Walton, Curr. Opin. Chem. Biol., 2010, 14, 238 CrossRef CAS PubMed
.
- Y. Zhao and D. Li, J. Mater. Chem. C, 2020, 8, 12739 RSC
.
- X. Wang, H. Chang, J. Xie, B. Zhao, B. Liu, S. Xu, W. Pei, N. Ren, L. Huang and W. Huang, Coord. Chem. Rev., 2014, 273, 201 CrossRef
.
- L.-N. Sun, H. Peng, M. I. J. Stich, D. Achatz and O. S. Wolfbeis, Chem. Commun., 2009, 33, 5000 RSC
.
- M. G. Lahoud, R. C. G. Frem, D. A. Gálico, G. Bannach, M. M. Nolasco, R. A. S. Ferreira and L. D. Carlos, J. Lumin., 2016, 170, 357 CrossRef CAS
.
- L. Li, Q. Chen, Z. Niu, X. Zhou, T. Yang and W. Huang, J. Mater. Chem. C, 2016, 4, 1900 RSC
.
- H. Wang, X. Wang, M. Liang, G. Chen, R.-M. Kong, L. Xia and F. Qu, Anal. Chem., 2020, 92, 3366 CrossRef CAS PubMed
.
- M. Runowski, P. Woźny and I. R. Martín, J. Mater. Chem. C, 2021, 9, 4643 RSC
.
- M. Runowski, P. Woźny, N. Stopikowska, Q. Guo and S. Lis, ACS Appl. Mater. Interfaces, 2019, 11, 4131 CrossRef CAS PubMed
.
- M. Runowski, P. Woźny, N. Stopikowska, I. R. Martín, V. Lavín and S. Lis, ACS Appl. Mater. Interfaces, 2020, 12, 43933 CrossRef CAS PubMed
.
- K. Du, J. Feng, X. Gao and H. Zhang, Light Sci. Appl., 2022, 11, 222 CrossRef CAS PubMed
.
- W. Yang, X. Li, D. Chi, H. Zhang and X. Liu, Nanotechnol, 2014, 25, 482001 CrossRef PubMed
.
- F. Wang, D. Banerjee, Y. Liu, X. Chen and X. Liu, Analyst, 2010, 135, 1839 RSC
.
- S. Han, R. Deng, X. Xie and X. Liu, Angew. Chem., Int. Ed., 2014, 53, 11702 CrossRef CAS PubMed
.
- F. Wang, Y. Han, C. S. Lim, Y. Lu, J. Wang, J. Xu, H. Chen, C. Zhang, M. i. Hong and X. Liu, Nature, 2010, 463, 1061 CrossRef CAS PubMed
.
- J.-C. G. Bünzli, Coord. Chem. Rev., 2015, 293, 19 CrossRef
.
- C. Wei, L. Ma, H. B. Wei, Z. W. Liu, Z. Q. Bian and C. H. Huang, Sci. China: Technol. Sci., 2018, 61, 1265 CrossRef CAS
.
- S. Faulkner, S. J. A. Pope and B. P. Burton-Pye, Appl. Spectrosc. Rev., 2005, 40, 1 CrossRef CAS
.
- C. Andraud and O. Maury, Eur. J. Inorg. Chem., 2009, 2009, 4357 CrossRef
.
- L. Armelao, S. Quici, F. Barigelletti, G. Accorsi, G. Bottaro, M. Cavazzini and E. Tondello, Coord. Chem. Rev., 2010, 254, 487 CrossRef CAS
.
- X.-Y. Zheng, J. Xie, X.-J. Kong, L.-S. Long and L.-S. Zheng, Coord. Chem. Rev., 2019, 378, 222 CrossRef CAS
.
- A. T. Wagner and P. W. Roesky, Eur. J. Inorg. Chem., 2016, 2016, 782 CrossRef CAS
.
- Z. Zhang, Y. Zhang and Z. Zheng, Struct. Bonding, 2017, 173, 1 CAS
.
- A. V. Virovets, E. Peresypkina and M. Scheer, Chem. Rev., 2021, 121, 14485 CrossRef CAS PubMed
.
- X.-M. Luo, Y.-K. Li, X.-Y. Dong and S.-Q. Zang, Chem. Soc. Rev., 2023, 52, 383 RSC
.
-
J.-C. G. Bünzli and S. V. Eliseeva, in Lanthanide Luminescence, ed. P. Hänninen and H. Härmä, Springer Berlin Heidelberg, Berlin, Heidelberg, 2010, vol. 7, pp. 1–45 Search PubMed
.
-
C. Görller-Walrand and K. Binnemans, in Handbook on the Physics and Chemistry of Rare Earths, ed. K. A. Gschneidner Jr and L. R. Eyring, Elsevier Science B. V., 1998, vol. 25, pp. 101–264 Search PubMed
.
-
C. Görller-Walrand and K. Binnemans, in Handbook on the Physics and Chemistry of Rare Earths, ed. K. A. Gschneidner Jr and L. Eyring, Elsevier Science B. V., 1996, vol. 23, pp. 121–283 Search PubMed
.
- K. Binnemans, Coord. Chem. Rev., 2015, 295, 1 CrossRef CAS
.
- J.-C. G. Bünzli and C. Piguet, Chem. Soc. Rev., 2005, 34, 1048 RSC
.
- L. Qin, G.-J. Zhou, Y.-Z. Yu, H. Nojiri, C. Schröder, R. E. P. Winpenny and Y.-Z. Zheng, J. Am. Chem. Soc., 2017, 139, 16405 CrossRef CAS PubMed
.
- R. Sen, D. K. Hazra, M. Mukherjee and S. Koner, Eur. J. Inorg. Chem., 2011, 2011, 2826 CrossRef CAS
.
- Y.-L. Li, H.-L. Wang, Z.-H. Zhu, F.-P. Liang and H.-H. Zou, Inorg. Chem., 2022, 61, 10101 CrossRef CAS PubMed
.
- M. Wu, F. Jiang, D. Yuan, J. Pang, J. Qian, S. A. AL-Thabaitic and M. Hong, Chem. Commun., 2014, 50, 1113 RSC
.
- L. Qin, Y.-Z. Yu, P.-Q. Liao, W. Xue, Z. Zheng, X.-M. Chen and Y.-Z. Zheng, Adv. Mater., 2016, 28, 10772 CrossRef CAS PubMed
.
- X.-Y. Li, H.-F. Su, Q.-W. Li, R. Feng, H.-Y. Bai, H.-Y. Chen, J. Xu and X.-H. Bu, Angew. Chem., Int. Ed., 2019, 58, 10184 CrossRef CAS PubMed
.
- X.-Y. Zheng, X.-J. Kong, Z. Zheng, L.-S. Long and L.-S. Zheng, Acc. Chem. Res., 2018, 51, 517 CrossRef CAS PubMed
.
- Z.-M. Zhang, K. H. Zangana, A. K. Kostopoulos, M.-L. Tong and R. E. P. Winpenny, Dalton Trans., 2016, 45, 9041 RSC
.
- F.-S. Guo, Y.-C. Chen, L.-L. Mao, W.-Q. Lin, J.-D. Leng, R. Tarasenko, M. Orendáč, J. Prokleška, V. Sechovský and M.-L. Tong, Chem.–Eur. J., 2013, 19, 14876 CrossRef CAS PubMed
.
- X.-Y. Zheng, J.-B. Peng, X.-J. Kong, L.-S. Long and L.-S. Zheng, Inorg. Chem. Front., 2016, 3, 320 RSC
.
- J.-B. Peng, Q.-C. Zhang, X.-J. Kong, Y.-P. Ren, L.-S. Long, R.-B. Huang, L.-S. Zheng and Z. Zheng, Angew. Chem., Int. Ed., 2011, 50, 10649 CrossRef CAS PubMed
.
- W. Huang, Z. Zhang, Y. Wu, W. Chen, D. A. Rotsch, L. Messerle and Z. Zheng, Inorg. Chem. Front., 2021, 8, 26 RSC
.
- T.-Q. Song, J. Dong, A.-F. Yang, X.-J. Che, H.-L. Gao, J.-Z. Cui and B. Zhao, Inorg. Chem., 2018, 57, 3144 CrossRef CAS PubMed
.
- Y.-L. Li, H.-L. Wang, Z.-H. Zhu, J. Li, H.-H. Zou and F.-P. Liang, Inorg. Chem., 2021, 60, 16794 CrossRef CAS PubMed
.
- P. Hu, S. Li, L. Cao, A. Liu, G.-L. Zhuang, L. Ji and B. Li, ACS Omega, 2022, 7, 38782 CrossRef CAS PubMed
.
- Z. Li, D. Wang, Z. Zhou, G. Zhao, Q. Li, Y. Bi and Z. Zheng, Inorg. Chem., 2022, 61, 20814 CrossRef CAS PubMed
.
- T.-Q. Lu, H. Xu, L.-T. Cheng, X.-T. Wang, C. Chen, L. Cao, G.-L. Zhuang, J. Zheng and X.-Y. Zheng, Inorg. Chem., 2022, 61, 8861 CrossRef CAS PubMed
.
- Z.-R. Luo, H.-L. Wang, Z.-H. Zhu, T. Liu, X.-F. Ma, H.-F. Wang, H.-H. Zou and F.-P. Liang, Commun. Chem., 2020, 3, 30 CrossRef CAS PubMed
.
- J. R. McBride, A. D. Dukes III, M. A. Schreuder and S. J. Rosenthal, Chem. Phys. Lett., 2010, 498, 1 CrossRef CAS PubMed
.
- B. H. Kim, M. J. Hackett, J. Park and T. Hyeon, Chem. Mater., 2014, 26, 59 CrossRef CAS
.
- K. Zarschler, L. Rocks, N. Licciardello, L. Boselli, E. Polo, K. P. Garcia, L. De Cola, H. Stephan and K. A. Dawson, Nanomedicine, 2016, 12, 1663 CrossRef CAS PubMed
.
- I. Halimi, E. M. Rodrigues, S. L. Maurizio, H.-Q. T. Sun, M. Grewal, E. M. Boase, N. Liu, R. Marin and E. Hemmer, J. Mater. Chem. C, 2019, 7, 15364 RSC
.
- N. Liu, N. Gobeil, P. Evers, I. Gessner, E. M. Rodrigues and E. Hemmer, Dalton Trans., 2020, 49, 16204 RSC
.
- D. A. Gálico, J. S. Ovens and M. Murugesu, Nanoscale, 2020, 12, 11435 RSC
.
- T. Nakanishi, Y. Suzuki, Y. Doi, T. Seki, H. Koizumi, K. Fushimi, K. Fujita, Y. Hinatsu, H. Ito, K. Tanaka and Y. Hasegawa, Inorg. Chem., 2014, 53, 7635 CrossRef CAS PubMed
.
- D. A. Gálico, A. A. Kitos, J. S. Ovens, F. A. Sigoli and M. Murugesu, Angew. Chem., Int. Ed., 2021, 60, 6130 CrossRef PubMed
.
- W. Huang, W. Chen, Q. Bai, Z. Zhang, M. Feng and Z. Zheng, Angew. Chem., Int. Ed., 2022, 134, e202205385 Search PubMed
.
- X.-Y. Zheng, Y.-H. Jiang, G.-L. Zhuang, D.-P. Liu, H.-G. Liao, X.-J. Kong, L.-S. Long and L.-S. Zheng, J. Am. Chem. Soc., 2017, 139, 18178 CrossRef CAS PubMed
.
- R. Wang, Z. Zheng, T. Jin and R. J. Staples, Angew. Chem., Int. Ed., 1999, 38, 1813 CrossRef CAS PubMed
.
- R. Wang, H. D. Selby, H. Liu, M. D. Carducci, T. Jin, Z. Zheng, J. W. Anthis and R. J. Staples, Inorg. Chem., 2002, 41, 278 CrossRef CAS PubMed
.
- Z. Zheng and R. Wang, Comments Inorg. Chem., 2000, 22, 1 CrossRef CAS
.
- J. Dong, P. Cui, P.-F. Shi, P. Cheng and B. Zhao, J. Am. Chem. Soc., 2015, 137, 15988 CrossRef CAS PubMed
.
- X.-M. Luo, Z.-B. Hu, Q.-F. Lin, W. Cheng, J.-P. Cao, C.-H. Cui, H. Mei, Y. Song and Y. Xu, J. Am. Chem. Soc., 2018, 140, 11219 CrossRef CAS PubMed
.
- X.-M. Luo, N.-F. Li, Q.-F. Lin, J.-P. Cao, P. Yuan and Y. Xu, Inorg. Chem. Front., 2020, 7, 2072 RSC
.
- X.-J. Kong, Y. Wu, L.-S. Long, L.-S. Zheng and Z. Zheng, J. Am. Chem. Soc., 2009, 131, 6918 CrossRef CAS PubMed
.
- J.-B. Peng, X.-J. Kong, Q.-C. Zhang, M. Orendac, J. Prokleska, Y. P. Ren, L.-S. Long, Z. Zheng and L.-S. Zheng, J. Am. Chem. Soc., 2014, 136, 17938 CrossRef CAS PubMed
.
- D. A. Gálico, J. S. Ovens, F. A. Sigoli and M. Murugesu, ACS Nano, 2021, 15, 5580 CrossRef PubMed
.
- D. A. Gálico and M. Murugesu, ACS Appl. Mater. Interfaces, 2021, 13, 47052 CrossRef PubMed
.
- D. A. Gálico, R. Ramdani and M. Murugesu, Nanoscale, 2022, 14, 9675 RSC
.
- D. A. Gálico and M. Murugesu, Angew. Chem., Int. Ed., 2022, 61, e202204839 CrossRef PubMed
.
- D. A. Gálico and M. Murugesu, Nanoscale, 2023, 15, 5778 RSC
.
- O. Laporte and W. F. Meggers, J. Opt. Soc. Am., 1925, 11, 459 CrossRef CAS
.
- D. Errulat, R. Marin, D. A. Gálico, K. L. M. Harriman, A. Pialat, B. Gabidullin, F. Iikawa, O. D. D. Couto Jr, J. O. Moilanen, E. Hemmer, F. A. Sigoli and M. Murugesu, ACS Cent. Sci., 2019, 5, 1187 CrossRef CAS PubMed
.
-
W. T. Carnall, P. R. Fields and K. Rajnak, Argonne National Laboratory – Technical Report, 1968 Search PubMed
.
- F. Wang, R. Deng, J. Wang, Q. Wang, Y. Han, H. Zhu, X. Chen and X. Liu, Nat. Mater., 2011, 10, 968 CrossRef CAS PubMed
.
- F. Wang and X. Liu, J. Am. Chem. Soc., 2008, 130, 5642 CrossRef CAS PubMed
.
- X. Qin, J. Xu, Y. Wu and X. Liu, ACS Cent. Sci., 2019, 5, 29 CrossRef CAS PubMed
.
- S. Wen, J. Zhou, K. Zheng, A. Bednarkiewicz, X. Liu and D. Jin, Nat. Commun., 2018, 9, 2415 CrossRef PubMed
.
- Z. Lei, X. Ling, Q. Mei, S. Fu, J. Zhang and Y. Zhang, Adv. Mater., 2020, 32, 1906225 CrossRef CAS PubMed
.
- Y. Zhong, I. Rostami, Z. Wang, H. Dai and Z. Hu, Adv. Mater., 2015, 27, 6418 CrossRef CAS PubMed
.
- G. Bao, K.-L. Wong, D. Jin and P. A. Tanner, Light Sci. Appl., 2018, 7, 96 CrossRef PubMed
.
- Y. Kitagawa, K. Matsuda, P. P. F. da Rosa, K. Fushimi and Y. Hasegawa, Chem. Commun., 2021, 57, 8047 RSC
.
- S. Faulkner and S. J. A. Pope, J. Am. Chem. Soc., 2003, 125, 10526 CrossRef CAS PubMed
.
- F. Auzel, Chem. Rev., 2004, 104, 139 CrossRef CAS PubMed
.
- S. Liu, L. Yan, J. Huang, Q. Zhang and B. Zhou, Chem. Soc. Rev., 2022, 51, 1729 RSC
.
- H. Dong, L.-D. Sun and C.-H. Yan, Nanoscale, 2013, 5, 5703 RSC
.
- B. Zhou, B. Shi, D. Jin and X. Liu, Nat. Nanotechnol., 2015, 10, 924 CrossRef CAS PubMed
.
- S. I. Weissman, J. Chem. Phys., 1942, 10, 214 CrossRef CAS
.
- G. A. Crosby, R. E. Whan and R. M. Alire, J. Chem. Phys., 1961, 34, 743 CrossRef CAS
.
- G. A. Crosby, R. E. Whan and J. J. Freeman, J. Phys. Chem., 1962, 66, 2493 CrossRef CAS
.
- F. J. Steemers, W. Verboom, D. N. Reinhoudt, E. B. van der Tol and J. W. Verhoeven, J. Am. Chem. Soc., 1995, 117, 9408 CrossRef CAS
.
- M. J. Beltrán-Leiva, E. Solis-Céspedes and D. Páez-Hernández, Dalton Trans., 2020, 49, 7444 RSC
.
- D. A. Gálico, E. R. Souza, I. O. Mazali and F. A. Sigoli, J. Lumin., 2019, 210, 397 CrossRef
.
- M. Latva, H. Takalo, V.-M. Mukkala, C. Matachescu, J. C. Rodríguez-Ubis and J. Kankare, J. Lumin., 1997, 75, 149 CrossRef CAS
.
- N. Bloembergen, Phys. Rev. Lett., 1959, 2, 84 CrossRef CAS
.
- F. Auzel, C. R. Acad. Sci., 1966, 263, 819 Search PubMed
.
- V. V. Ovsyakin and P. P. Feoflov, JETP Lett., 1966, 4, 317 Search PubMed
.
- R. Rafique, S. K. Kailasa and T. J. Park, Trends Anal. Chem., 2019, 120, 115646 CrossRef
.
- M. Zhao, B. Li, P. Wang, L. Lu, Z. Zhang, L. Liu, S. Wang, D. Li, R. Wang and F. Zhang, Adv. Mater., 2018, 30, 1804982 CrossRef PubMed
.
- Q. Liu, W. Feng and F. Li, Coord. Chem. Rev., 2014, 273, 100 CrossRef
.
- Y.-F. Wang, G.-Y. Liu, L.-D. Sun, J.-W. Xiao, J.-C. Zhou and C.-H. Yan, ACS Nano, 2013, 7, 7200 CrossRef CAS PubMed
.
- M. V. Da Costa, S. Doughan, Y. Han and U. J. Krull, Anal. Chim. Acta, 2014, 832, 1 CrossRef CAS PubMed
.
- S. Xu, S. Huang, Q. He and L. Wang, Trends Anal. Chem., 2015, 66, 72 CrossRef CAS
.
- Y. Xie, Y. Sun, J. Sun, Y. Wang, S. Yu, B. Zhou, B. Xue, X. Zheng, H. Liu and B. Dong, Inorg. Chem. Front., 2023, 10, 93 RSC
.
- N. M. Idris, M. K. Gnanasammandhan, J. Zhang, P. C. Ho, R. Mahendran and Y. Zhang, Nat. Med., 2012, 18, 1580 CrossRef CAS PubMed
.
- M. R. Hamblin, Dalton Trans., 2018, 47, 8571 RSC
.
- Y. Liu, X. Meng and W. Bu, Coord. Chem. Rev., 2019, 379, 82 CrossRef CAS
.
- Y. Liu, Y. Liu, W. Bu, C. Cheng, C. Zuo, Q. Xiao, Y. Sun, D. Ni, C. Zhang, J. Liu and J. Shi, Angew. Chem., Int. Ed., 2015, 54, 8105 CrossRef CAS PubMed
.
- B. Zheng, H. Wang, H. Pan, C. Liang, W. Ji, L. Zhao, H. Chen, X. Gong, X. Wu and J. Chang, ACS Nano, 2017, 11, 11898 CrossRef CAS PubMed
.
- S. Chen, A. Z. Weitemier, X. Zeng, L. He, X. Wang, Y. Tao, A. J. Y. Huang, Y. Hashimotodani, M. Kano, H. Iwasaki, L. K. Parajuli, S. Okabe, D. B. L. Teh, A. H. All, I. Tsutsui-Kimura, K. F. Tanaka, X. Liu and T. J. McHugh, Science, 2018, 359, 679 CrossRef CAS PubMed
.
- A. H. All, X. Zeng, D. B. L. Teh, Z. Yi, A. Prasad, T. Ishizuka, N. Thakor, Y. Hiromu and X. Liu, Adv. Mater., 2019, 31, 180347 CrossRef PubMed
.
- Z. Wang, M. Hu, X. Ai, Z. Zhang and B. Xing, Adv. Biosyst., 2019, 3, 1800233 CrossRef PubMed
.
- X. Wu, Y. Zhang, K. Takle, O. Bilsel, Z. Li, H. Lee, Z. Zhang, D. Li, W. Fan, C. Duan, E. M. Chan, C. Lois, Y. Xiang and G. Han, ACS Nano, 2016, 10, 1060 CrossRef CAS PubMed
.
- T. Hebert, R. Wannemacher, W. Lenth and R. M. Macfarlane, Appl. Phys. Lett., 1990, 57, 1727 CrossRef
.
- H. Zhu, X. Chen, L. M. Jin, Q. J. Wang, F. Wang and S. F. Yu, ACS Nano, 2013, 7, 11420 CrossRef CAS PubMed
.
- T. Wang, H. Yu, C. K. Siu, J. B. Qiu, X. H. Xu and S. F. Yu, ACS Photonics, 2017, 4, 1539 CrossRef CAS
.
- A. Fernandez-Bravo, K. Yao, E. S. Barnard, N. J. Borys, E. S. Levy, B. Tian, C. A. Tajon, L. Moretti, M. V. Altoe, S. Aloni, K. Beketayev, F. Scotognella, B. E. Cohen, E. M. Chan and P. J. Schuck, Nat. Nanotechnol., 2018, 13, 572 CrossRef CAS PubMed
.
- L. Jin, Y. Wu, Y. Wang, S. Liu, Y. Zhang, Z. Li, X. Chen, W. Zhang, S. Xiao and Q. Song, Adv. Mater., 2019, 31, 1807079 CrossRef PubMed
.
- Y. Liu, Y. Lu, X. Yang, X. Zheng, S. Wen, F. Wang, X. Vidal, J. Zhao, D. Liu, Z. Zhou, C. Ma, J. Zhou, J. A. Piper, P. Xi and D. Jin, Nature, 2017, 543, 229 CrossRef CAS PubMed
.
- X. Peng, B. Huang, R. Pu, H. Liu, T. Zhang, J. Widengren, Q. Zhan and H. Ågren, Nanoscale, 2019, 11, 1563 RSC
.
- C. Chen, B. Liu, Y. Liu, J. Liao, X. Shan, F. Wang and D. Jin, Adv. Mater., 2021, 33, 2008847 CrossRef CAS PubMed
.
- H. Dong, L.-D. Sun and C.-H. Yan, Front. Chem., 2021, 8, 619377 CrossRef PubMed
.
- C. Chen, L. Ding, B. Liu, Z. Du, Y. Liu, Xi. Di, X. Shan, C. Lin, M. Zhang, X. Xu, X. Zhong, J. Wang, L. Chang, B. Halkon, X. Chen, F. Cheng and F. Wang, Nano Lett., 2022, 22, 7136 CrossRef CAS PubMed
.
- D. Jaque and F. Vetrone, Nanoscale, 2012, 4, 4301 RSC
.
- F. Vetrone, R. Naccache, A. Zamarrón, A. J. de la Fuente, F. Sanz-Rodríguez, L. M. Maestro, E. Martin Rodriguez, D. Jaque, J. G. Sole and J. A. Capobianco, ACS Nano, 2010, 4, 3254 CrossRef CAS PubMed
.
- E. M. Rodrigues, D. A. Gálico, I. O. Mazali and F. A. Sigoli, Sens. Actuators, A, 2019, 291, 1 CrossRef CAS
.
- C. Hazra, A. Skripka, S. J. L. Ribeiro and F. Vetrone, Adv. Opt. Mater., 2020, 8, 2001178 CrossRef CAS
.
- C. Krishnaraj, H. Rijckaert, H. S. Jena, P. Van Der Voort and A. M. Kaczmarek, ACS Appl. Mater. Interfaces, 2021, 13, 47010 CrossRef CAS PubMed
.
- O. A. Savchuk, J. J. Carvajal, C. D. S. Brites, L. D. Carlos, M. Aguilo and F. Diaz, Nanoscale, 2018, 10, 6602 RSC
.
- M. Runowski, N. Stopikowska, D. Szeremeta, S. Goderski, M. Skwierczyńska and S. Lis, ACS Appl. Mater. Interfaces, 2019, 11, 13389 CrossRef CAS PubMed
.
- C. D. S. Brites, X. Xie, M. L. Debasu, X. Qin, R. Chen, W. Huang, J. Rocha, X. Liu and L. D. Carlos, Nat. Nanotechnol., 2016, 11, 851 CrossRef CAS PubMed
.
- E. M. Rodrigues, D. A. Gálico, M. A. Lemes, J. Bettini, E. T. Neto, I. O. Mazali, M. Murugesu and F. A. Sigoli, New J. Chem., 2018, 42, 13393 RSC
.
- E. M. Rodrigues, D. A. Gálico, I. O. Mazali and F. A. Sigoli, Methods Appl. Fluoresc., 2017, 5, 024012 CrossRef PubMed
.
- H. Suo, Q. Zhu, X. Zhang, B. Chen, J. Chen and F. Wang, Mater. Phys. Today, 2021, 21, 100520 CrossRef CAS
.
- T. Sun, B. Xu, B. Chen, X. Chen, M. Li, P. Shi and F. Wang, Nanoscale, 2017, 9, 2701 RSC
.
- X. Liu, Y. Wang, X. Li, Z. Yi, R. Deng, L. Liang, X. Xie, D. T. B. Loong, S. Song, D. Fan, A. H. All, H. Zhang, L. Huang and X. Liu, Nat. Commun., 2017, 8, 899 CrossRef PubMed
.
- S. Liu, L. Yan, Q. Li, J. Huang, L. Tao and B. Zhou, Chem. Eng. J., 2020, 397, 125451 CrossRef CAS
.
- R. Huang, S. Liu, J. Huang, H. Liu, Z. Hu, L. Tao and B. Zhou, Nanoscale, 2021, 13, 4812 RSC
.
- B. Golesorkhi, S. Naseri, L. Guenee, I. Taarit, F. Alves, H. Nozary and C. Piguet, J. Am. Chem. Soc., 2021, 143, 15326 CrossRef CAS PubMed
.
- Y. Suffren, B. Golesorkhi, D. Zare, L. Guénée, H. Nozary, S. V. Eliseeva, S. Petoud, A. Hauser and C. Piguet, Inorg. Chem., 2016, 55, 9964 CrossRef CAS PubMed
.
- B. Golesorkhi, H. Nozary, A. Fürstenberg and C. Piguet, Mater. Horiz., 2020, 7, 1279 RSC
.
- B. Golesorkhi, A. Fürstenberg, H. Nozary and C. Piguet, Chem. Sci., 2019, 10, 6876 RSC
.
- N. Souri, P. Tian, C. Platas-Iglesias, K.-L. Wong, A. Nonat and L. J. Charbonnière, J. Am. Chem. Soc., 2017, 139, 1456 CrossRef CAS PubMed
.
- A. Nonat, S. Bahamyirou, A. Lecointre, F. Przybilla, Y. Mély, C. Platas-Iglesias, F. Camerel, O. Jeannin and L. J. Charbonnière, J. Am. Chem. Soc., 2019, 141, 1568 CrossRef CAS PubMed
.
- A. M. Nonat and L. J. Charbonnière, Coord. Chem. Rev., 2020, 409, 213192 CrossRef CAS
.
- M. Kaiser, C. Würth, M. Kraft, I. Hyppänen, T. Soukka and U. Resch-Genger, Nanoscale, 2017, 9, 10051 RSC
.
- C. Homann, L. Krukewitt, F. Frenzel, B. Grauel, C. Würth, U. Resch-Genger and M. Haase, Angew. Chem., Int. Ed., 2018, 57, 8765 CrossRef CAS PubMed
.
- C. Würth, S. Fischer, B. Grauel, A. P. Alivisatos and U. Resch-Genger, J. Am. Chem. Soc., 2018, 140, 4922 CrossRef PubMed
.
- R.-G. Xiong, J.-L. Zuo, Z. Yu, X.-Z. You and W. Chen, Inorg. Chem. Commun., 1999, 2, 490 CrossRef CAS
.
- J.-C. G. Bünzli and S. V. Eliseeva, J. Rare Earths, 2010, 28, 824 CrossRef
.
- G. Xu, Z.-M. Wang, Z. He, Z. Lü, C.-S. Liao and C.-H. Yan, Inorg. Chem., 2002, 41, 6802 CrossRef CAS PubMed
.
- S. A. Junior, F. V. de Almeida, G. F. de Sá and C. M. Donegá, J. Lumin., 1997, 72, 478 CrossRef
.
- D. A. Gálico, I. O. Mazali and F. A. Sigoli, J. Lumin., 2017, 192, 224 CrossRef
.
- K. Manseki, Y. Hasegawa, Y. Wada, H. Ichida, Y. Kanematsu and T. Kushida, J. Lumin., 2007, 122, 262 CrossRef
.
- S. Omagari, T. Nakanishi, T. Seki, Y. Kitagawa, Y. Takahata, K. Fushimi, H. Ito and Y. Hasegawa, J. Phys. Chem. A, 2015, 119, 1943 CrossRef CAS PubMed
.
- K. Sheng, W.-D. Si, R. Wang, W.-Z. Wang, J. Dou, Z.-Y. Gao, L.-K. Wang, C.-H. Tung and D. Sun, Chem. Mater., 2022, 34, 4186 CrossRef CAS
.
- D. T. Thielemann, A. T. Wagner, E. Rösch, D. K. Kölmel, J. G. Heck, B. Rudat, M. Neumaier, C. Feldmann, U. Schepers, S. Bräse and P. W. Roesky, J. Am. Chem. Soc., 2013, 135, 7454 CrossRef CAS PubMed
.
- S. Petit, F. Baril-Robert, G. Pilet, C. Reber and D. Luneau, Dalton Trans., 2009, 34, 6809 RSC
.
- X.-Y. Chen, X. Yang and B. J. Holliday, Inorg. Chem., 2010, 49, 2583 CrossRef CAS PubMed
.
- S. Comby, R. Scopelliti, D. Imbert, L. Charbonnière, R. Ziessel and J.-C. G. Bünzli, Inorg. Chem., 2006, 45, 3158 CrossRef CAS PubMed
.
- F. Baril-Robert, S. Petit, G. Pilet, G. Chastanet, C. Reber and D. Luneau, Inorg. Chem., 2010, 49, 10970 CrossRef CAS PubMed
.
- D. I. Alexandropoulos, S. Mukherjee, C. Papatriantafyllopoulou, C. P. Raptopoulou, V. Psycharis, V. Bekiari, G. Christou and T. C. Stamatatos, Inorg. Chem., 2011, 50, 11276 CrossRef CAS PubMed
.
- S. Omagari, T. Nakanishi, Y. Kitagawa, T. Seki, K. Fushimi, H. Ito, A. Meijerink and Y. Hasegawa, Sci. Rep., 2016, 6, 1 CrossRef PubMed
.
- S. Omagari, T. Nakanishi, Y. Kitagawa, T. Seki, K. Fushimi, H. Ito, A. Meijerink and Y. Hasegawa, J. Lumin., 2018, 201, 170 CrossRef CAS
.
- X.-L. Li, L.-F. He, X.-L. Feng, Y. Song, M. Hu, L.-F. Han, X.-J. Zheng, Z.-H. Zhang and S.-M. Fang, CrystEngComm, 2011, 13, 3643 RSC
.
- D. Shi, X. Yang, Y. Ma, M. Niu and R. A. Jones, Inorg. Chem., 2020, 59, 14626 Search PubMed
.
- W. Hao, X. Yang, Y. Ma, M. Niu, D. Shi and D. Schipper, Dalton Trans., 2021, 50, 5865 RSC
.
- Y. Ma, X. Yang, M. Niu, D. Shi and D. Schipper, J. Lumin., 2022, 241, 118494 CrossRef CAS
.
- Y. Ma, X. Yang, D. Shi, M. Niu and D. Schipper, Inorg. Chem., 2020, 59, 17608 CrossRef CAS PubMed
.
- Y. Ma, X. Yang, Z. Xiao, X. Liu, D. Shi, M. Niu and D. Schipper, Chem. Commun., 2021, 57, 7316 RSC
.
- W. -Q. Lin, X. -F. Liao, J. -H. Jia, J. -D. Leng, J. -L. Liu, F. -S. Guo and M. -L. Tong, Chem.–Eur. J., 2013, 19, 12254 CrossRef CAS PubMed
.
- D. Shi, X. Yang, H. Chen, D. Jiang, J. Liu, Y. Ma, D. Schipper and R. A. Jones, Chem. Commun., 2019, 55, 13116 RSC
.
- D. Shi, X. Yang, H. Chen, Y. Ma, D. Schipper and R. A. Jones, J. Mater. Chem. C, 2019, 7, 13425 RSC
.
- T. L. King, O. E. Palomero, A. B. Bard, J. A. Espinoza Jr, H. Guo, D. Schipper, X. Yang, L. J. DePue, E. L. Que and R. A. Jones, J. Coord. Chem., 2021, 74, 92 CrossRef CAS
.
- X.-Y. Li, Y. Jing, J. Zheng, H. Ding, Q. Li, M.-H. Yu and X.-H. Bu, Cryst. Growth Des., 2020, 20, 5294 CrossRef CAS
.
- D. Guettas, C. M. Balogh, C. Sonneville, Y. Malicet, F. Lepoivre, E. Onal, A. Fateeva, C. Reber, D. Luneau, O. Maury and G. Pilet, Eur. J. Inorg. Chem., 2016, 2016, 3932 CrossRef CAS
.
- R. C. Knighton, L. K. Soro, A. Lecointre, G. Pilet, A. Fateeva, L. Pontille, L. Francés-Soriano, N. Hildebrandt and L. J. Charbonniere, Chem. Commun., 2021, 57, 53 RSC
.
- R. C. Knighton, L. K. Soro, L. Francés-Soriano, A. Rodríguez-Rodríguez, G. Pilet, M. Lenertz, C. Platas-Iglesias, N. Hildebrandt and L. J. Charbonniere, Angew. Chem., Int. Ed., 2022, 61, e202113114 CrossRef CAS PubMed
.
- A. N. C. Neto, E. Mamontova, A. M. P. Botas, C. D. S. Brites, R. A. S. Ferreira, J. Rouquette, Y. Guari, J. Larionova, J. Long and L. D. Carlos, Adv. Opt. Mater., 2022, 10, 2101870 CrossRef
.
- C. Krishnaraj, H. Rijckaert, H. S. Jena, P. Van Der Voort and A. M. Kaczmarek, ACS Appl. Mater. Interfaces, 2021, 13, 47010 CrossRef CAS PubMed
.
- A. M. Kaczmarek, Y.-Y. Liu, M. K. Kaczmarek, H. Liu, F. Artizzu, L. D. Carlos and P. van Der Voort, Angew. Chem., Int. Ed., 2020, 132, 1948 CrossRef
.
- A. Cadiau, C. D. S. Brites, P. M. J. Costa, R. A. S. Ferreira, J. Rocha and L. D. Carlos, ACS Nano, 2013, 7, 7213 CrossRef CAS PubMed
.
- V. Trannoy, A. N. Carneiro Neto, C. D. S. Brites, L. D. Carlos and H. Serier-Brault, Adv. Opt. Mater., 2021, 9, 2001938 CrossRef CAS
.
- K. Karachousos-Spiliotakopoulos, V. Tangoulis, N. Panagiotou, A. Tasiopoulos, E. Moreno-Pineda, W. Wernsdorfer, M. Schulze, A. M. P. Botas and L. D. Carlos, Dalton Trans., 2022, 51, 8208 RSC
.
- R. A. S. Ferreira, E. Mamontova, A. M. P. Botas, M. Shestakov, J. Vanacken, V. Moshchalkov, Y. Guari, L. F. Chibotaru, D. Luneau, P. S. André, J. Larionova, J. Long and L. D. Carlos, Adv. Opt. Mater., 2021, 9, 2101495 CrossRef CAS
.
- J. Wang, J. J. Zakrzewski, M. Heczko, M. Zychowicz, K. Nakagawa, K. Nakabayashi, B. Sieklucka, S. Chorazy and S.-I. Ohkoshi, J. Am. Chem. Soc., 2020, 142, 3970 CrossRef CAS PubMed
.
- D. M. Lyubov, A. N. Carneiro Neto, A. Fayoumi, K. A. Lyssenko, V. M. Korshunov, I. V. Taydakov, F. Salles, Y. Guari, J. Larionova, L. D Carlos, J. Long and A. A. Trifonov, J. Mater. Chem. C, 2022, 10, 7176 RSC
.
- D. A. Gálico, I. O. Mazali and F. A. Sigoli, New J. Chem., 2018, 42, 18541 RSC
.
- D. A. Gálico, I. O. Mazali and F. A. Sigoli, Int. J. Mol. Sci., 2022, 23, 14526 CrossRef PubMed
.
- A. A. Kitos, D. A. Gálico, R. Castañeda, J. S. Ovens, M. Murugesu and J. Brusso, Inorg. Chem., 2020, 59, 11061 CrossRef CAS PubMed
.
- A. A. Kitos, D. A. Gálico, N. Mavragani, R. Castañeda, J. O. Moilanen, J. L. Brusso and M. Murugesu, Chem. Commun., 2021, 57, 7818 RSC
.
- X.-Q. Guo, L.-P. Zhou, L.-X. Cai and Q.-F. Sun, Chem.–Eur. J., 2018, 24, 6936 CrossRef CAS PubMed
.
- P. G. Derakhshandeh, S. Abednatanzi, L. Bourda, S. Dalapati, A. Abalymov, M. Meledina, Y.-Y. Liu, A. G. Skirtach, K. Van Hecke, A. M. Kaczmarek and P. Van Der Voort, J. Mater. Chem. C, 2021, 9, 6436 RSC
.
- R. Marin, D. A. Gálico, R. Gayfullina, J. O. Moilanen, L. D. Carlos, D. Jaque and M. Murugesu, J. Mater. Chem. C, 2022, 10, 13953 Search PubMed
.
- A. Pilch, C. Würth, M. Kaiser, D. Wawrzyńczyk, M. Kurnatowska, S. Arabasz, K. Prorok, M. Samoć, W. Strek, U. Resch-Genger and A. Bednarkiewicz, Small, 2017, 20, 1701635 CrossRef PubMed
.
- A. Pilch, D. Wawrzyńczyk, M. Kurnatowska, B. Czaban, M. Samoć, W. Strek and A. Bednarkiewicz, J. Lumin., 2017, 182, 114 CrossRef CAS
.
- R. Naccache, F. Vetrone, V. Mahalingam, L. A. Cuccia and J. A. Capobianco, Chem. Mater., 2009, 21, 717 CrossRef CAS
.
- P. Tadge, R. S. Yadav, P. K. Vishwakarma, S. B. Rai, T.-M. Chen, S. Sapra and S. Ray, J. Alloys Compd., 2020, 821, 153230 CrossRef CAS
.
- R. Joshi, S. Patra, M. Srivastava, B. P. Singh, A. Chakraborty, S. B. Shelar, R. Chakravarty, S. Chakraborty and R. S. Ningthoujam, ACS Appl. Nano Mater., 2022, 5, 12962 CrossRef CAS
.
|
This journal is © The Royal Society of Chemistry 2023 |
Click here to see how this site uses Cookies. View our privacy policy here.