DOI:
10.1039/D2VA00168C
(Perspective)
Environ. Sci.: Adv., 2022,
1, 612-619
Below zero†
Received
23rd July 2022
, Accepted 26th September 2022
First published on 4th October 2022
Abstract
The current climate debate focuses on how to reach net zero latest by 2050. Most transformation pathways rely on negative emissions to compensate “hard-to-avoid” emissions, for example in aviation, industry or livestock farming. However, even a constant global heating at 1.5 °C may trigger climate tipping points, such as the loss of cryosphere, permafrost or ecosystems. It therefore becomes necessary to achieve “below zero” with large-scale negative emissions, reducing atmospheric CO2 concentration and climate forcing. This paper argues for a systemic view and shows with a comparison of past, current and future carbon stocks and flows that storing the minimally necessary removals will already be challenging. Consequently, continued fossil emissions shall be avoided completely, as their compensation increases removals and binds societal resources. For delivering the required scale and speed of negative emissions, scalable technical solutions will have to developed, as bio-based solutions are limited though essential for reverting land use impacts and safeguarding biodiversity. In this context, it is important to investigate the potential of a circular carbon economy, storing carbon in safe and reliable material cycles.
Environmental significance
Negative emissions are foreseen at large scale to achieve the intermediary target of net zero emissions. Even though politically endorsed and dominating the debate, the net zero narrative disregards the required reduction of atmospheric carbon to achieve long-term climate stability and the cumulative storage capacity for negative emissions. From an Earth system perspective, compensating “hard-to-avoid” emissions cannot be sustained indefinitely and distracts from returning to a safe climate regime as it binds materials, energy and societal resources. Furthermore, it reveals the limited, though important, potential of bio-based solutions, necessitating to design and investigate scalable and reliable technical carbon storage.
|
Introduction
Earth is experiencing rapid loss of ice and permafrost,1,2 increase in weather and ocean extremes,3,4 declining biological productivity5,6 and many more severe consequences already now at only 1.19 °C global heating.7 The international, political consensus is to limit global heating to well below 2
°C and preferably 1.5 °C,8 which means a further substantial increase compared to today. Though still attainable in principle,9 sluggish climate action requires ever faster and more ambitious strategies.10 While it is most urgent to limit peak heating to prevent severe short term damages, it is insufficient to avoid climate tipping with high confidence.11,12
During the past one million years, atmospheric CO2 concentration had been between 180
ppm in ice ages and 280
ppm in warm periods.13 Anthropogenic CO2 emissions are accumulating in the atmosphere and upper oceans, leading to an increase in atmospheric CO2 concentration, the main driver for global heating.14 It is rising faster than ever and currently crossing 417
ppm.15 For limiting peak heating, it is imperative to minimize cumulative emissions. However, constant global heating at 1.5 °C may still exceed vital limits for other climate impacts—such as sea level rise, ocean acidification or decline in biological productivity12—and trigger a tipping cascade, inducing runaway heating with disastrous consequences.1,11,16,17 Consequently, it is necessary to actively remove CO2 from the system in order to reduce the induced heating and halt or even revert the loss of cryosphere, forests and other essential Earth systems.1 An atmospheric CO2 concentration of 350
ppm has been proposed as a safe level for long term climate stability.18–20 Reaching 350
ppm—or any other long term climate target—inevitably requires below zero emissions at a massive scale.21,22
The current debate on climate action centres around reaching net zero emissions globally in about 2050. In this narrative, which can be summarized as “Do your best, remove the rest”,23 “hard-to-avoid” emissions can be continuously compensated by negative emissions.24 What is considered “hard-to-avoid” is currently discussed in a socio-economic perspective: either substitution with emission free alternatives is considered “too costly” (e. g. hydrogen reduced steel,25 synthetic fuels and chemicals26) or shifting and reducing consumption, often related to affluence,27,28 “too inconvenient” (e. g. shift to predominantly vegan diet29 or alignment of energy demand with solar supply30). Compensation is assumed as possible between all kinds of greenhouse gas emissions and across different locations and time scales.31 It leads to delaying actions for avoiding emissions, which has been termed mitigation deterrence.32 It further gives rise to concerns such as possibly negative effects on biodiversity, infringement of indigenous rights or “climate-colonialism”,33–35 for a minority of rich individuals, companies and countries compensates lifestyle-dependent emissions on foreign land.27
In transition pathways aiming at limiting peak heating to 1.5 °C considered by IPCC, for example, negative C emissions have to start this decade and increase to approximately −3 Gt/a in 2050.36 This is necessary to compensate the remaining fossil emissions of equal magnitude36 (i. e. 27% of current fossil emissions24). After this important milestone is reached, fossil emissions decrease only slightly, while negative emissions increase to about −5 Gt/a in 2100 (Fig. S1 and Section
S2†). Global temperature correlates almost linearly with increasing cumulative emissions14,37 and non-linearly (i. e. with a hysteresis) with decreasing ones.38–40 Until 2100, negative C emissions cumulate between −220
Gt and −260
Gt in IPCC pathways (Fig.
S2†). Yet, only 1/3 (−70
Gt to −90
Gt) reduce climate forcing and are thus truly negative emissions, while the rest (−140
Gt to −180
Gt) is compensating continued fossil emissions (Fig. 1, S1 and S2, and Table
S1†). As a consequence, these projected negative emissions will have little effect on global temperature reduction despite tremendous efforts (260
Gt is as much C as had been emitted over the past 30 years).
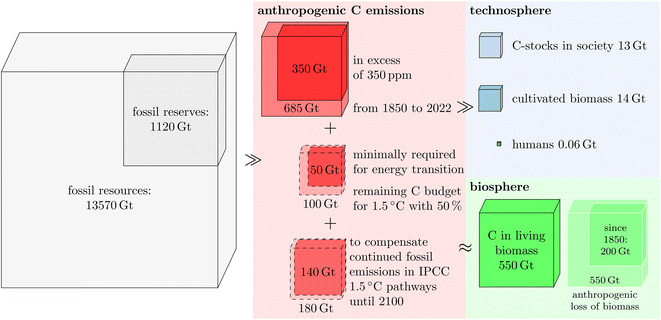 |
| Fig. 1 Comparison of anthropogenic C emissions (red) with C stocks in fossil fuels (grey, more than one order of magnitude larger), technosphere (blue, one order of magnitude smaller) and biosphere (green, the same order of magnitude). Solid black lines denote current, dashed lines future and white lines past C stocks. | |
Regardless of with or without compensation, emissions need to reduce to (net) zero soon to limit peak heating. For stabilizing the climate in the long term, “cleaning-up” the atmosphere and returning to 350
ppm inevitably requires below zero emissions at a large scale. The question is, if and how much hard-to-avoid emissions society can and wants to afford, which need to be continuously compensated in addition. Avoiding emissions completely will remove the underlying cause for climate change and necessitates faster actions.32,41 Yet, fossil fuels cannot be switched off immediately, as the replacing renewable energy system first needs to be built. Installing the necessary infrastructure requires energy in addition to (reduced) societal demand. In the beginning of the transition, it can only come from the fossil energy system.9,21,30 A minimum of 50
Gt of C has to be emitted to achieve the energy transition.9 When exhausting the remaining carbon budget for 1.5 °C with 50% confidence, this increases to 100
Gt of C (Fig. 1 and Section
S1†). Together with the 350
Gt C already in excess in the atmosphere and upper oceans, at least 400
Gt to 450
Gt has to be removed and stored safely as below zero emissions to reach 350
ppm. For comparison, this is about as much pure carbon (C) than the mass of all concrete in use in society today42,43 (Fig. 2). The required scale of negative emissions is thereby one order of magnitude larger than C currently contained in or managed by the technosphere, in the same order of magnitude than C contained in living biomass and two orders of magnitude smaller than fossil fuel resources (Fig. 1). This is—simply put—a gargantuan task ahead. Hard-to-avoid emissions in IPCC pathways36 necessitate to increase negative emissions by 40% within this century and more thereafter.
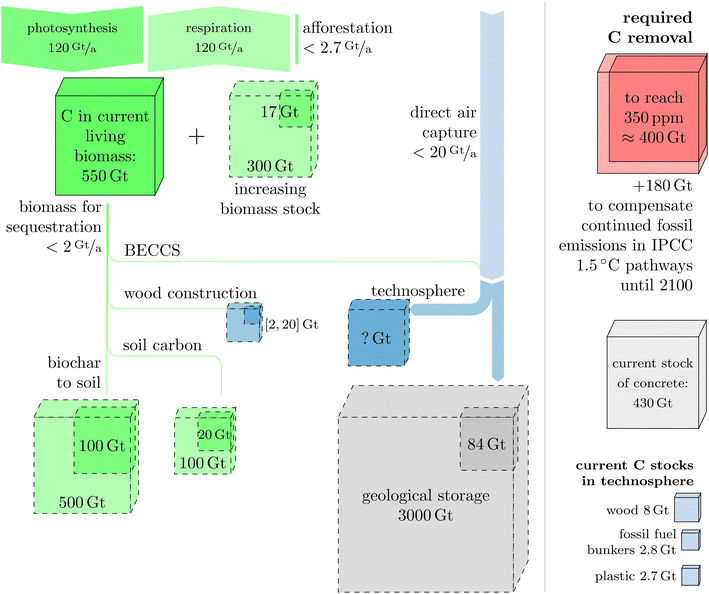 |
| Fig. 2 Comparison of carbon stocks (cubes) and flows (Sankey) for different negative emissions routes. All stocks and flows are representing C mass content (except concrete). For reaching 350 ppm, CO2 currently in excess and minimally required emissions during the transition (red cube) have to be removed. Continued fossil emissions have to be removed in addition (light red cube). This is comparable to the mass of current concrete stocks,43 while two orders of magnitude above current C stocks in society: wood, fossil fuels bunkers and plastics (right). Current living biomass processes around 120 Gt/a of C (photosynthesis and respiration)44 and may provide a limited flow of C for sequestration. This flow can be stored either in wood construction,45 through bioenergy with carbon capture and storage (BECCS) in geological or technical stores, as biochar in soil or by increasing soil carbon sequestration (SCS). Biochar and SCS are limited by the maximum C content of soil.46 Afforestation can increase C in living biomass. Direct air capture (DAC) is limited by the sustainable potential of renewable energy21 and by far the largest potential NET flux. C from DAC and BECCS can be transported and stored in geological storage or incorporated in products and cycled within the technosphere. | |
Negative emission routes
Different technical and nature based negative emission technologies (NET) are being discussed in literature (Fig. 2).47,48 Most of them remove CO2 by reverting the mode of release (i. e. biomass growth and direct air capture), while some propose new routes, such as enhanced weathering49,50 or ocean fertilisation.51 Considering that fossil energy use created the climate crisis unintendedly, the potential risks and side effects of new geo-engineering experiments are high.48 Consequently and in precaution, reverting anthropogenic emissions shall be preferred through their mode of release, i. e. technical and biological NETs for fossil and land use emissions. In the following, the possible scales for such NETs are put in perspective with past, current and future carbon stocks and flows (Fig. 2 and S3 and Table
S2†) applying an Earth systems perspective irrespective of social and economic aspects. Negative emission potentials are counted as the actual removal capacity, disregarding indirect substitution effects e. g. resulting from replacing fossil energy through bioenergy with carbon capture and storage (BECCS) or concrete with wood as a construction material.
Increase C stocks in living biomass
Restoring and increasing C stocks in the biosphere, e. g. through afforestation,52 can, if implemented properly, safeguard biodiversity and restore ecosystem integrity in addition. The C-flux for afforestation is limited by the available land and the growth dynamics of forests and may range between 0.12 Gt/a and 2.7 Gt/a.48,52–56 Total storage potential is limited due to saturation of C-uptake in mature forests after approximately one century.56 Estimates for the cumulative storage potential vary widely in the literature between 17 Gt and 300 Gt.53,56 For comparison, land use change has emitted about 200 Gt of C since 1850,57 which is within the range for afforestation potential (Fig. 2). Even though difficult to achieve, reverting land use change to pre-industrial levels can at best remove half the C necessary to reach 350
ppm. It would be insufficient to deliver the negative emissions necessary in IPCC 1.5 °C pathways alone (260
Gt of C until 2100). Today, the biosphere contains 550
Gt of C in living biomass, the majority in forests ecosystems.44,58 Bar-On et al.58 estimate that living biomass halved since humanity's existence, i. e. living biomass has been reduced by 550
Gt. The even more hypothetical case of restoring biomass to pre-human level could just be sufficient to reach 350
ppm.
Biomass for sequestration
In addition to increasing biomass stocks, the biosphere can also provide a C-flux for permanent sequestration through wood for construction,45 BECCS,53,59 soil carbon sequestration (SCS) or biochar to soils.46 Sustainable wood production, useable for wood construction, BECCS and biochar, is limited to a C-flux of 0.6 Gt/a.60,61 Agricultural residues (currently 2.46 Gt/a (ref. 62)) and dedicated biomass production on marginal land may increase the C-flux for sequestration.53 However, human appropriation of net primary production is already substantial63 and causing severe pressure on planetary boundaries.29,64,65 Estimates for biomass sequestration C-flux in literature range between 0.14 Gt/a (ref. 48) to 3.3 Gt/a (ref. 54) (up to 11.6 Gt/a,53 which seems unrealistic in comparison to total current C harvest of 8 Gt/a (ref. 62)). Considering decline in biological productivity, loss of fertile land and increased desertification5 and a sequestration efficiency of about 50%,53 C-flux of biomass sequestration is unlikely to exceed <2 Gt/a. Throughout Earth's history, the size of this biological “leak” has been four orders of magnitude smaller. During the Carbon period (350 to 250 million years before now), net surplus produced by forests transferred 12
300
Gt of C to coal deposits.66–68 The average C-flux to coal had been 0.000123 Gt/a over this entire period. Similarly, oil and gas deposits had formed during the Jura period (190 to 175 million years ago) and stored 550
Gt in oil and 1
820
Gt in gas.66–68 The average C-flux into these deposits had been 0.000158 Gt/a. This comparison suggests that unperturbed natural ecosystems may have a long term potential to remove atmospheric C of around 0.00016 Gt/a only, making it necessary to investigate in detail if 2 Gt/a could even be sustained.
The cumulative storage potential of biomass sequestration varies for each route. Wood construction may increase C stock by 2 Gt to 20 Gt this century, depending on construction demand and wood content.45 This may roughly double the wood stock in society (currently 8 Gt (ref. 42)). Carbon captured with BECCS has to be stored in technical or geological storage (see below), whereas SCS and biochar increase the C stock in soil. Currently, soils are estimated to hold between 1
500
Gt and 2
400
Gt of C (excluding living biomass) and permafrost may contain 1
700
Gt in addition44 (Fig.
S3†). Biochar may add 100
Gt to 500
Gt (ref. 53 and 56) and SCS 20 Gt to 100 Gt (ref. 56) to soils (Fig. 2). Biochar may contribute a significant storage potential, still impacts of application at scale on soil productivity, biodiversity, C release and resistance to extreme events—like wildfires—remain to be investigated.
Direct air capture
Capturing CO2 directly from the ambient air is energy intensive, but in contrast to bio-based solutions nearly independent of land availability.69 When powering direct air capture (DAC) with solar PV from the already built environment,21 additional land conversion is negligible.70 There are different DAC technologies available, some already at pilot scale and others in development.71–76 When powered with renewable energy, DAC has negligible C emissions stemming from the production of the materials contained in the infrastructure,77,78 which can also be avoided by decarbonising the supply chains. Resources required for the plant and sorbent are considered uncritical.78,79 For these reasons, DAC are foreseen as a major part of future energy systems,21,69,80–82 yet it faces the challenge of upscaling from pilot scale to a global industry.56,83 Furthermore, DAC may be constrained by economic and social limitations,48,54 however, they can be overcome in principle.47,84,85 C-flux from DAC is ultimately limited by the availability of excess solar energy on the already sealed surface of the built environment. At constant or decreasing energy demand from society, solar PV could power a C-flux from DAC of <20 Gt/a (Section
S4†),21 one order of magnitude larger than for biomass sequestration or afforestation. Yielding this potential will depend on the mobilisation of resources building the required solar infrastructure, the subsequent handling (e. g. transport) as well as the energy and resource requirements for technical and geological storage.
Geological storage
The principal challenge of BECCS and DACCS is the long-term safe storage of technically captured CO2.86 Geological storage is the injection of CO2 into geological formations, which may hold CO2 over centuries and millennia.86,87 Under certain conditions, CO2 reacts with the surrounding rock to form carbonates.88 Current geological storage projects are, however, often associated with oil and gas production.89 One example is the Sleipner project in Norway.90 This offshore gas field has a high content of CO2 in the gas, which is separated and injected back into the gas field to recover more gas (“enhanced oil/gas recovery”). In the first 20 years of operation, the Sleipner project stored 4.4
Mt of C. During the same time (1996–2016), oil and gas had been extracted worth 48
Mt of C emissions,91 paradoxically with the help of the separated and injected CO2. Consequently, the project avoided 9% emissions. Other projects without enhanced oil/gas recovery are currently under development (e. g. Climeworks in Iceland92). At the scale of injections at Sleipner, about 104 similar sized storage operations would be necessary to store the minimally required 400
Gt of C before the end of this century, highlighting the challenge of upscaling storage.
The Global CCS Institute estimates the global potential for C storage in saline formations to 84
Gt,93 while IPCC estimate the total geological potential to be in the range of 500 Gt to 3
000
Gt.75 Even if the cumulative storage potential may be sufficient, it may be difficult to find enough suitable and safe geological formations for permanent CO2 storage in time. Additionally, leakage has to be stored again, increasing the required C-flux.94
Technical storage
Geological storage is an end-of-pipe solution, as such a burden (or “cost”) to society. In contrast, incorporating C in products and cycling C in the technosphere can create value. A “circular carbon” economy may make excess C the main constituent of the socio-economic metabolism. Currently, the technosphere holds about 13
Gt of C, mostly in wood and paper products (8
Gt (ref. 43)), plastics (2.7
Gt (ref. 43)) and fossil fuel bunkers (2.8
Gt (ref. 67) Fig. S3†). In contrast to today, C would need to be cycled within the technosphere, preventing leakage to the atmosphere. It would also need to increase C stocks in the technosphere by more than one order of magnitude, for example by incorporating it in long lived products, such as buildings and infrastructure. This, however, has to go much beyond current efforts of wood construction (see above) and “CO2 binding concrete”. Concrete takes up about 10% of CO2 emissions previously released in cement production during the service life of buildings. This may be increased to <30% during recycling, when exposing crushed concrete under increased pressure and concentration of CO2.95–97 If all current concrete (430
Gt (ref. 43)) would take up 30% of CO2 emissions from their production (about 30 kg C t−1
concrete98), it could remove about 4
Gt of C. Consequently, CO2 binding concrete may at best contribute <1% to below zero. Using captured C in synthetic fuels or short lived products (e. g. carbonated drinks, single use plastics) has a storage potential proportional to the stocks of these products. For example, bunkering synfuels to the equivalent of one year's consumption of today's fossil fuels, would only hold 13
Gt of C out of the atmosphere. Consequently, storage of C in short lived products and synfuels may only make a minor contribution to C storage, while greatly increasing C circulation from and to the atmosphere and its associated energy demand.
In contrast, it would be necessary that C becomes the main constituent of any bulk material were we to store a significant fraction of removed C in the technosphere. Research is necessary for finding practical means to convert atmospheric CO2 into synthetic polymers, graphite, graphene, diamonds or other C containing materials and keeping them out of the atmosphere for centuries at low energetic costs.
Conclusions
Below zero emissions are inevitable to reduce atmospheric CO2 concentration and stabilize the climate. A minimum of 400
Gt of C has to be removed and stored permanently and safely. This is as much pure C as all the concrete in society or almost as much as contained in currently living biomass. Negative emissions for compensating continued fossil emissions have to be stored in addition. Already for the minimally required negative emissions, finding practical solutions at scale is a challenge. Consequently, the notion of “hard-to-avoid” emissions has to be rethought, finding ways to avoid them by substitution with expensive but emission-free technology as well as shifting and reducing consumption. By looking beyond net zero and applying a systems perspective, our strategy has to change: compensation of continued fossil emissions is no longer viable, in contrast, it distracts from the major task of returning to safe climate conditions. It conveys a false hope, leads to stranded investments, binds materials, requires energy and generates continuous need for storing C. These resources are more urgently needed for building the replacing renewable energy infrastructure and removing excess CO2 from the atmosphere to stabilize the climate in the long run.21 Restoring the biosphere has co-benefits of safeguarding biodiversity along with storing C. As the biosphere's stock and flow capacities are limited, it is relevant to design and investigate a leading role of direct air capture, which has the potential to remove C one order of magnitude faster than bio-based NETs (Fig. 2). Safe, reliable and scalable storage possibilities at low energy costs have to be developed, e.
g. as circular carbon materials in the technosphere. While the remaining resource flows have to drastically decrease to return to the safe operating space for humanity,99 C-fluxes out of the atmosphere into stocks in the technosphere will have to increase: a huge potential market that will have to grow fast.
Author contributions
Conceptualization, H. D.; method, H. D.; validation, H. D.; formal analysis, H. D.; investigation, H. D.; visualization, H. D.; writing–original draft preparation, H. D.; writing–review and editing, H. D.
Conflicts of interest
The author declares no known competing interests, which could have appeared to influence the work reported in this paper.
Acknowledgements
The author thanks Martin Gasser, Charles Marmy, Marta Roca Puigròs, Patrick Wäger and Rolf Widmer for comments and discussions when preparing this manuscript.
References
-
IPCC. The Ocean and Cryosphere in a Changing Climate Report (IPCC, 24 September 2019, 2019) Search PubMed
.
- T. Slater,
et al., Review article: Earth's ice imbalance, Cryosphere, 2021, 15, 233–246 CrossRef
issn: 1994-0424..
-
World Meterological Organization. State of the Global Climate 2020 Report WMO-Nr. 1264 (WMO, 2021). https://library.wmo.int/doc_num.php?explnum_id=10618 Search PubMed
.
- N. Gruber, P. W. Boyd, T. L. Frolicher and M. Vogt, Biogeochemical extremes and compound events in the ocean, Nature, 2021, 600, 395–407 CrossRef CAS PubMed
issn: 1476-4687 (Electronic) 0028-0836 (Linking)..
-
IPCC, IPCC Special Report on Climate Change, Desertification, Land Degradation, Sustainable Land Management, Food Security, and Greenhouse Gas Fluxes in Terrestrial Ecosystems Report (Intergovernmental Panel for Climate Change, 2019) Search PubMed
.
- H. Bugmann and C. Bigler, Will the CO2 fertilization effect in forests be offset by reduced tree longevity?, Oecologia, 2011, 165, 533–544 CrossRef PubMed
issn: 1432-1939 (Electronic) 0029-8549 (Linking)..
-
R. Lindsey & L. DahlmanClimate Change: Global Temperature Web Page. 2021. https://www.climate.gov/news-features/understanding-climate/climate-change-global-temperature Search PubMed
.
-
United Nations Framework Convention on Climate Change. Paris Agreement Generic. 2015/12/12/, 2015. https://unfccc.int/process-and-meetings/the-paris-agreement/the-paris-agreement Search PubMed
.
- H. Desing and R. Widmer, Reducing climate risks with fast and complete energy transitions: applying the precautionary principle to the Paris agreement, Environ. Res. Lett., 2021, 16(12), 121002 CrossRef CAS
. issn: 1748-9326..
-
IRENA. World, Energy Transitions Outlook: 1.5 Pathway Report, (International renewable energy agency, 2022), ISBN 978-92-9260-429-5, https://www.irena.org/publications/2022/Mar/World-Energy-Transitions-Outlook-2022 Search PubMed
.
- C. Heinze,
et al., The quiet crossing of ocean tipping points, Proc. Natl. Acad. Sci. U. S. A., 2021, 118, 1–9 CrossRef PubMed
. issn: 1091-6490 (Electronic) 0027-8424 (Linking)..
- M. Steinacher, F. Joos and T. F. Stocker, Allowable carbon emissions lowered by multiple climate targets, Nature, 2013, 499, 197–201 CrossRef CAS PubMed
. issn: 1476-4687 (Electronic) 0028-0836 (Linking)..
-
A. Berger, F. Mesinger & D. SijackiClimate Change – Inferences from Paleoclimate and Regional Aspects, (Springer, 2012), isbn: 978-3-7091-0972-4. DOI:10.1007/978-3-7091-0973-1
.
-
IPCC. Sixth Assessment Report: Physical Science Basis Report (IPCC, 2021). https://www.ipcc.ch/report/sixth-assessment-report-working-group-i/ Search PubMed
.
-
E. Dlugokencky & P. TansNOAA Trends in Atmospheric Carbon Dioxide Web Page. 2022. https://www.esrl.noaa.gov/gmd/ccgg/trends/ Search PubMed
.
- W. Steffen,
et al., Trajectories of the Earth System in the Anthropocene, Proc. Natl. Acad. Sci. U. S. A., 2018, 115, 8252–8259 CrossRef CAS PubMed
. issn: 1091-6490 (Electronic) 0027-8424 (Linking)..
- T. M. Lenton,
et al., Climate tipping points – too risky to bet against, Nature, 2019, 575, 592–595 CrossRef CAS PubMed
. issn: 1476-4687..
- J. Hansen,
et al., Assessing ”dangerous climate change”: required reduction of carbon emissions to protect young people, future generations and nature, PLoS One, 2013, 8, e81648 CrossRef PubMed
. issn: 1932-6203 (Electronic) 1932-6203 (Linking)..
- W. Steffen,
et al., Sustainability. Planetary boundaries: guiding human development on a changing planet, Science, 2015, 347, 1259855 CrossRef PubMed
. issn: 1095-9203 (Electronic) 0036-8075 (Linking)..
-
Center for Biological Diversity and 350.org. Not just a number: Achieving A CO2 Concentration of 350 ppm or Less To Avoid Catastrophic Climate Impacts Report. https://www.biologicaldiversity.org/programs/climate_law_institute/350_or_bust/pdfs/Not_Just_a_Number-v3.pdf Search PubMed.
- H. Desing, A. Gerber, R. Hischier, P. Wäger and R. Widmer, The 3-machines energy transition model: exploring the energy frontiers for restoring a habitable climate, Earth’s Future, 2022 DOI:10.1029/2022ef002875
.
-
P. Richner
CO2 Must Be Fairly Priced Interview. 2022-04-11, 2022. https://www.empa.ch/web/s604/eq75-price-for-co2 Search PubMed
.
-
SwissRE. Do Our Best, Remove the Rest – Insurers Role in Growing the Carbon Removal Industry Web Page. 2021. https://www.swissre.com/risk-knowledge/mitigating-climate-risk/do-our-best-remove-the-rest.html Search PubMed
.
- S. J. Davis,
et al., Net-zero emissions energy systems, Science, 2018, 360, 1–9 CrossRef PubMed
. issn: 1095-9203..
-
M. Ahman
et al.
Hydrogen Steelmaking for a Low Carbon Economy Report (Stockholm Environmental Institute, Lund University, 2018) Search PubMed
.
-
J. Perner, M. Unteutsch & A. LvenichThe Future Cost of Electricity-Based Synthetic Fuels Report (Agora Energiewende, Frontier Economics Ltd, 2018) Search PubMed
.
- Y. Oswald, A. Owen and J. K. Steinberger, Large inequality in international and intranational energy footprints between income groups and across consumption categories, Nat. Energy, 2020, 5, 231–239 CrossRef
. issn: 2058-7546..
- T. Wiedmann, M. Lenzen, L. T. Keysser and J. K. Steinberger, Scientists' warning on affluence, Nat. Commun., 2020, 11, 3107 CrossRef CAS PubMed
. issn: 2041-1723..
- W. Willett,
et al., Food in the Anthropocene: the EATLancet Commission on healthy diets from sustainable food systems, Lancet, 2019, 393, 447–492 CrossRef
. issn: 01..
- H. Desing and R. Widmer, How Much Energy Storage can We Afford? On the Need for a Sunflower Society, Aligning Demand with Renewable Supply, Biophysical Economics and Sustainability, 2022, 7(3), 1–15 Search PubMed
.
- K. Anderson and G. Peters, The trouble with negative emissions, Science, 2016, 354, 182–183 CrossRef CAS PubMed
. issn: 1095-9203 (Electronic) 0036-8075 (Linking)..
- N. Grant, A. Hawkes, S. Mittal and A. Gambhir, Confronting mitigation deterrence in low-carbon scenarios, Environ. Res. Lett., 2021, 16(6), 1–13 Search PubMed
. issn: 1748-9326..
-
O. O. Tw
Climate Colonialism and Large-Scale Land Acquisitions Blog. 2019. https://www.c2g2.net/climate-colonialism-and-large-scale-land-acquisitions/ Search PubMed
.
-
S. Heiba
How the EU Green Deal Perpetuates Climate Colonialism Newspaper Article. 2021-02-03, 2021. https://earth.org/eu-green-deal-perpetuates-climate-colonialism/ Search PubMed
.
-
G. Monbiot
Carbon offsetting Is Not Warding off Environmental Collapse – its Accelerating it Newspaper Article. 2022-01-26, 2022. https://www.theguardian.com/commentisfree/2022/jan/26/carbon-offsetting-environmental-collapse-carbon-land-grab?f=outliner Search PubMed
.
-
IPCC. Global Warming of 1.5 °C. An IPCC Special Report on the impacts of global warming of 1.5 °C above pre-industrial levels and related global greenhouse gas emission pathways, in The Context of Strengthening the Global Response to the Threat of Climate Change, Sustainable Development, and Efforts to Eradicate Poverty Report (Intergovernmental Panel for Climate Change, 2018) Search PubMed
.
- R. Knutti and J. Rogelj, The legacy of our CO2 emissions: a clash of scientific facts, politics and ethics, Clim. Change, 2015, 133, 361–373 CrossRef CAS
. issn: 0165-0009 1573-1480..
- K. Zickfeld, D. Azevedo, S. Mathesius and H. D. Matthews, Asymmetry in the climatecarbon cycle response to positive and negative CO2 emissions, Nat. Clim. Change, 2021, 11, 613–617 CrossRef CAS
. issn: 1758-678X 1758-6798..
- K. Zickfeld, A. H. MacDougall and H. D. Matthews, On the proportionality between global temperature change and cumulative CO 2 emissions during periods of net negative CO 2 emissions, Environ. Res. Lett., 2016, 11, 1–10 Search PubMed
. issn: 1748-9326..
- A. Jeltsch-Thömmes, T. F. Stocker and F. Joos, Hysteresis of the Earth system under positive and negative CO2 emissions, Environ. Res. Lett., 2020, 15(12) DOI:10.1088/1748-9326/abc4af
. issn: 1748-9326..
- D. P. Van Vuuren, A. F. Hof, M. A. van Sluisveld and K. Riahi, Open discussion of negative emissions is urgently needed, Nat. Energy, 2017, 2, 902–904 CrossRef
. issn: 2058-7546..
- F. Krausmann, C. Lauk, W. Haas and D. Wiedenhofer, From resource extraction to outflows of wastes and emissions: The socioeconomic metabolism of the global economy, 19002015, Global Environ. Change, 2018, 52, 131–140 CrossRef PubMed
.
- D. Wiedenhofer,
et al., Prospects for a saturation of humanity resource use? An analysis of material stocks and flows in nine world regions from 1900 to 2035, Global Environ. Change, 2021, 71, 1–15 Search PubMed
. issn: 0959..
- P. Friedlingstein,
et al., Global Carbon Budget 2020, Earth Syst. Sci. Data, 2020, 12, 3269–3340 CrossRef
. issn: 1866-3516..
- G. Churkina,
et al., Buildings as a global carbon sink, Nat.
Sustain., 2020, 3, 269–276 CrossRef
.
- P. Smith, Soil carbon sequestration and biochar as negative emission technologies, Global Change Biol., 2016, 22, 1315–1324 CrossRef PubMed
. issn: 1365-2486 (Electronic) 1354-1013 (Linking)..
- J. C. Minx,
et al., Negative emissionsPart 1: Research landscape and synthesis, Environ. Res. Lett., 2018, 13(6), 1–30 Search PubMed
. issn: 1748-9326..
- S. Fuss,
et al., Negative emissionsPart 2: Costs, potentials and side effects, Environ. Res. Lett., 2018, 13(6), 1–48 Search PubMed
. issn: 1748-9326..
- D. J. Beerling,
et al., Potential for large-scale CO2 removal via enhanced rock weathering with croplands, Nature, 2020, 583, 242–248 CrossRef CAS PubMed
. issn: 1476-4687..
- D. S. Goll,
et al., Potential CO2 removal from enhanced weathering by ecosystem responses to powdered rock, Nat. Geosci., 2021, 14, 545–549 CrossRef CAS
. issn: 1752-0894 1752-0908..
- R. S. Lampitt,
et al., Ocean fertilization: a potential means of geoengineering?, Philos. Trans. R. Soc., A, 2008, 366, 3919–3945 CrossRef CAS PubMed
. issn: 1364-503X (Print) 1364-503X (Linking)..
- S. C. Cook-Patton,
et al., Mapping carbon accumulation potential from global natural forest regrowth, Nature, 2020, 585, 545–550 CrossRef CAS PubMed
. issn: 1476-4687 (Electronic) 0028-0836 (Linking)..
- T. M. Lenton, The potential for land-based biological CO2 removal to lower future atmospheric CO2 concentration, Carbon Manage., 2014, 1, 145–160 CrossRef
. issn: 1758-3004 1758-3012..
- P. Smith,
et al., Biophysical and economic limits to negative CO2 emissions, Nat. Clim. Change, 2015, 6, 42–50 CrossRef
. issn: 1758-678X 1758-6798..
- B. W. Griscom,
et al., Natural climate solutions, Proc. Natl. Acad. Sci. U. S. A., 2017, 114, 11645–11650 CrossRef CAS PubMed
. issn: 1091-6490 (Electronic) 0027-8424 (Linking)..
-
O. Rueda, J. M. Mogolln, A. Tukker & L. SchererNegative-emissions Technology Portfolios to Meet the 1.5 C Target, Global Environmental Change, 2021, DOI:10.1016/j.gloenvcha.2021.102238, issn: 09593780
.
- P. Friedlingstein,
et al., Global Carbon Budget 2019, Earth Syst. Sci. Data, 2019, 11, 1783–1838 CrossRef
. issn: 1866-3516..
- Y. M. Bar-On, R. Phillips and R. Milo, The biomass distribution on Earth, Proc. Natl. Acad. Sci. U. S. A., 2018, 115, 6506–6511 CrossRef CAS PubMed
. issn: 1091-6490 (Electronic) 0027-8424 (Linking)..
- A. Babin, C. Vaneeckhaute and M. C. Iliuta, Potential and challenges of bioenergy with carbon capture and storage as a carbon-negative energy source: A review, Biomass Bioenergy, 2021, 146, 105968 CrossRef CAS
. issn: 09619534..
-
M. O'Brien
Timber Consumption and Sustainable Forest Use: Assessing the EUs Current and Expected Consumption of Global Timber in Relation to the Global Capacity for Sustainable Supply Thesis, 2015 Search PubMed
.
- H. Desing, R. Widmer, D. Beloin-Saint-Pierre, R. Hischier and P. Wäger, Powering a Sustainable and Circular EconomyAn Engineering Approach to Estimating Renewable Energy Potentials within Earth System Boundaries, Energies, 2019, 12, 1–18 CrossRef
. issn: 1996-1073..
- H. Haberl,
et al., Quantifying and mapping the human appropriation of net primary production in earth's terrestrial ecosystems, Proc. Natl. Acad. Sci. U. S. A., 2007, 104, 12942 CrossRef CAS PubMed
.
- F. Krausmann,
et al., Global human appropriation of net primary production doubled in the 20th century, Proc. Natl. Acad. Sci. U. S. A., 2013, 110, 10324–10329 CrossRef CAS PubMed
. issn: 1091-6490 (Electronic) 0027-8424 (Linking)..
- D. Gerten,
et al., Feeding ten billion people is possible within four terrestrial planetary boundaries, Nat. Sustain., 2020, 3, 200–208 CrossRef
. issn: 2398-9629..
- M. Springmann,
et al., Options for keeping the food system within environmental limits, Nature, 2018, 562, 519–525 CrossRef CAS PubMed
. issn: 1476-4687..
-
P. Kausch, M. Bertau, J. Gutzmer & J. MatschullatEnergie und Rohstoffe, (Spektrum, 2011), isbn: 978-3-8274-2797-7 Search PubMed
.
-
British Petroleum (BP). Statistical Review of World Energy Web Page. 2020. https://www.bp.com/en/global/corporate/energy-economics/statistical-review-of-world-energy.html Search PubMed
.
- Spliethoff. Vorelsungsskript Energietechnik 1 Manuscript. 2011.
- C. Breyer, M. Fasihi and A. Aghahosseini, Carbon dioxide direct air capture for effective climate change
mitigation based on renewable electricity: a new type of energy system sector coupling, Mitig. Adapt. Strategies Glob. Change, 2019, 25, 43–65 CrossRef
. issn: 1381-2386 1573-1596..
- M. Ozkan, S. P. Nayak, A. D. Ruiz and W. Jiang, Current status and pillars of direct air capture technologies, iScience, 2022, 25, 103990 CrossRef CAS PubMed
. issn: 2589-0042..
-
American Physial Society. Direct Air Capture of CO2 with Chemicals – A Technology Assessment for the APS Panel on Public Affairs Report (APS, 2011). https://www.aps.org/policy/reports/popa-reports/loader.cfm?csModule=security/getfile%26PageID=244407 Search PubMed
.
- C. Antonini,
et al., Hydrogen production from natural gas and biomethane with carbon capture and storage A techno-environmental analysis, Sustainable Energy Fuels, 2020, 4, 2967–2986 RSC
. issn: 2398-4902..
- M. Fasihi, O. Efimova and C. Breyer, Techno-economic assessment of CO2 direct air capture plants, J. Cleaner Prod., 2019, 224, 957–980 CrossRef CAS
. issn: 09596526..
- A. Gambhir and M. Tavoni, Direct Air Carbon Capture and Sequestration: How It Works and How It Could Contribute to Climate-Change Mitigation, One Earth, 2019, 1, 405–409 CrossRef
. issn: 25903322..
-
IPCC. IPCC Special Report on Carbon Dioxide Capture and Storage Report (Intergovernmental Panel on Climate Change, 2006), ISBN-13 978-0-521-86643-9 Search PubMed
.
- S. Sgouridis, M. Carbajales-Dale, D. Csala, M. Chiesa and U. Bardi, Comparative net energy analysis of renewable electricity and carbon capture and storage, Nat. Energy, 2019, 4, 456–465 CrossRef CAS
. issn: 2058-7546..
- M. M. J. De Jonge, J. Daemen, J. M. Loriaux, Z. J. N. Steinmann and M. A. J. Huijbregts, Life cycle carbon efficiency of Direct Air Capture systems with strong hydroxide sorbents, Int. J. Greenhouse Gas Control, 2019, 80, 25–31 CrossRef CAS
. issn: 1750583..
- S. Deutz and A. Bardow, Life-cycle assessment of an industrial direct air capture process based on temperature vacuum swing adsorption, Nat. Energy, 2021, 6, 203–213 CrossRef CAS
. issn: 2058-7546..
- X. Shi,
et al., Sorbents for the Direct Capture of CO2 from Ambient Air, Angew. Chem., Int. Ed. Engl., 2020, 59, 6984–7006 CrossRef CAS PubMed
. issn: 1521-3773..
- C. Beuttler, L. Charles and J. Wurzbacher, The Role of Direct Air Capture in Mitigation of Anthropogenic Greenhouse Gas Emissions, Front. Clim., 2019, 1, 10 CrossRef
. issn: 2624-9553..
- R. Hanna, A. Abdulla, Y. Xu and D. G. Victor, Emergency deployment of direct air capture as a response to the climate crisis, Nat. Commun., 2021, 12, 368 CrossRef CAS PubMed
. issn: 2041-1723..
- G. Realmonte,
et al., An inter-model assessment of the role of direct air capture in deep mitigation pathways, Nat. Commun., 2019, 10, 3277 CrossRef CAS PubMed
. issn: 2041-1723..
- G. F. Nemet,
et al., Negative emissionsPart 3: Innovation and upscaling, Environ. Res. Lett., 2018, 13(6), 1–31 Search PubMed
. issn: 1748-9326..
-
IPCC. Assessment Report 5: Climate Change 2013, the Physical Science Basis Report (IPCC, 2013) Search PubMed
.
- N. McQueen,
et al., A review of direct air capture (DAC): scaling up commercial technologies and innovating for the future, Progress in Energy, 2021, 3, 1–23 CrossRef
. issn: 2516-1083..
-
National Energy Technology Laboratory. Safe Geological Storage of Captured Carbon Dioxide: Two Decades of DOE's Carbon Storage R&D Program in Review Report 2130.104.003.001 (NETL, 2020). https://northernlightsccs.com/wp-content/uploads/2021/03/Safe-Geologic-Storage-of-Captured-Carbon-Dioxide_April_15_2020_FINAL.pdf Search PubMed
.
- K. Z. House, D. P. Schrag, C. F. Harvey and K. S. Lackner, Permanent carbon dioxide storage in deep-sea sediments, Proc. Natl. Acad. Sci. U. S. A., 2006, 103, 12291–12295 CrossRef CAS PubMed
. issn: 0027-8424 (Print) 0027-8424 (Linking)..
- J. M. Matter,
et al., Rapid carbon mineralization for permanent disposal of anthropogenic carbon dioxide emissions, Science, 2016, 352, 1312–1314 CrossRef CAS PubMed
. issn: 1095-9203 (Electronic) 0036-8075 (Linking)..
- M. Bui,
et al., Carbon capture and storage (CCS): the way forward, Energy Environ. Sci., 2018, 11, 1062–1176 RSC
. issn: 1754-5692 1754-5706..
-
O. Skalmeraas
Sleipner Carbon Capture and Storage Project Web Page. 2017. https://www.ice.org.uk/knowledge-and-resources/case-studies/sleipner-carbon-capture-storage-project Search PubMed
.
-
Norsk Petroleum. Sleipner West Gas Field Statistics Web Page. 2022. https://www.norskpetroleum.no/en/facts/field/sleipner-vest/ Search PubMed.
-
Climeworks. CO2 Storage Solution Web Page. 2022. https://climeworks.com/co2-storage-solutions Search PubMed.
-
Global CCS Institute. Global Status Report 2019 Report, 2019. https://www.globalccsinstitute.com/wp-content/uploads/2019/12/GCC_GLOBAL_STATUS_REPORT_2019.pdf Search PubMed
.
- A. Vinca, J. Emmerling and M. Tavoni, Bearing the Cost of Stored Carbon Leakage, Front. Energy Res., 2018, 6, 40 CrossRef
. issn: 2296-598X..
-
P. V. Nygaard & A. LeemannKohlendioxidaufnahme von Stahlbetonbauten durch Karbonatisierung Report, (Empa, Cemsuisse, 2012). https://www.cemsuisse.ch/app/uploads/2020/04/<?pdb_no 2012?>2012<?pdb END?>_Kohlendioxidaufnahme-von-Stahlbetonbauten-durch-Karbonatisierung_Dr-Nygaard_Dr-Leemann.pdf Search PubMed
.
-
A. G. Sika. Betonrecycling alle Lsungen fr CO2-rmeren Beton Web Page. 2020. https://www.sika.com/de/annual-report/annual-report-2020/sika-as-enabler/concrete-recycling.html Search PubMed
.
-
Neustark GmbH. Concrete Solutions. TODAY. Web Page. 2021. https://www.neustark.com/ Search PubMed
.
- G. Wernet,
et al., The ecoinvent database version 3 (part I): overview and methodology, Int. J. Life Cycle Assess., 2016, 21, 1218–1230 CrossRef
. issn: 0948-3349 1614-7502..
- H. Desing, G. Braun and R. Hischier, Ecological resource availability: a method to estimate resource budgets for a sustainable economy, Glob. Sustain., 2020, 3, 1–11 CrossRef
. issn: 2059-4798..
|
This journal is © The Royal Society of Chemistry 2022 |
Click here to see how this site uses Cookies. View our privacy policy here.