DOI:
10.1039/C4RA05174B
(Paper)
RSC Adv., 2014,
4, 44692-44699
Synthesis of a hierarchically meso-macroporous TiO2 film based on UV light-induced in situ polymerization: application to dye-sensitized solar cells†
Received
31st May 2014
, Accepted 18th August 2014
First published on 18th August 2014
Abstract
Hierarchically meso-macroporous TiO2 films were prepared via a UV light-induced in situ polymerization of hybrid organic–inorganic films containing propoxylated glyceryltriacrylate monomers and titania precursors. These films were composed of well-connected, net-like frameworks with a large open cavity (0.2–1.5 μm), which were assembled by titania nanocrystals with a size of ∼25 nm and that possessed relatively rich inner crystal mesopores of about 4 nm. The particular net-like frameworks make the film a promising candidate for use as the scattering layer in dye-sensitized solar cells (DSSCs). A bilayer structured photo-electrode, consisting of the net-like framework layer on top of the P25 film, was prepared and exhibited good overall conversion efficiency. Interestingly, the photovoltaic conversion efficiency (η) was improved to 6.95%, much higher than that of P25 single-layer film (6.17%).
1 Introduction
Since the breakthrough work into dye-sensitized solar cells (DSSCs) in 1991, much attention and various attempts have been made to improve the efficiency of DSSCs in the past decades.1–10 As the core component in DSSCs, the structures and properties of photoanodes directly affect the photoelectron conversion efficiency. Recently, various novel photoanode materials have been developed towards improving the utilization of photoelectrons.5–10
Nanocrystalline TiO2 has been the most widely used photoanode material for DSSCs, due to its superior stability and efficient electron injection efficiency.11 Such photoanode materials normally possess large surface areas to maximize dye absorption for light harvesting. Usually, TiO2 thin films composed of small NPs (20–30 nm) have higher surface area, which leads to enhanced light-harvesting efficiency but, unfortunately, decreased light scattering. The addition of a scattering layer has been attracting much attention as an effective solution to this problem.10,12–16 Currently, light-scattering layers can be formed in the following ways: (1) mixing TiO2 particles of different sizes, with the larger particles used as scattering centers;17 (2) using polystyrene spheres as a template to form a film, and then removing the polystyrene spheres to produce spherical voids that act as scattering layer in the electrode;18,19 (3) TiO2 nanorods and TiO2 microplates used as a scattering layer.20–22 As is well known, the introduction of large particles would reduce the dye-loading capacity of the electrode. Therefore, a film-type scattering layer is more favorable for light harvesting.
As a result, more efforts have been devoted to preparing porous TiO2 film with a large surface area. Highly organized and oriented mesoporous TiO2 thin films with various mesostructures, such as p6m, Ia3d, or Im3m, have already been prepared.23–25 Two groups, those of Grätzel and Li, have investigated mesoporous TiO2 films exhibiting greatly enhanced performance in dye-sensitized solar cells, but the performance of the actual cells is limited by incomplete filling of mesopores due to the smaller pore size (<10 nm),26,27 and the existing preparation methods are complex, so not suitable for large-scale production.
UV curing is a facile way to prepare polymeric films from photosensitive monomers, and the properties of the synthesized film can be easily controlled using different photosensitive monomers and irradiation conditions.28 In the present work, hierarchically mesoporous TiO2 films with controllable hierarchical structure and properties were fabricated through UV light-induced in situ polymerization of hybrid organic–inorganic films containing photosensitive monomers and using titania precursors with net-like frameworks. The as-prepared, net-like framework (NF) film was further used as the scattering layer to construct a novel double-layer (DL) film with the NF layer on top of the P25 layer. The introduction of the NF layer increased the light-scattering capacity at around 300–500 nm, owing to its branched network structure. As a result, the photovoltaic conversion efficiency (η) was improved to 6.95%, much higher than that of P25 single-layer film (6.17%). On the fluorine-doped tin oxide (FTO) substrate without the NF layer, only a small fraction of the light is reflected, and most of the solar energy is transmitted out of the system. Hence, due to the enhanced light-scattering by the NF layer, more electrons can be excited, and thus the photocurrent and photoelectrical conversion efficiency are increased.
2 Experimental section
2.1 Materials
Titanium tetrabutoxide (TTB), anhydrous ethanol, N,N-dimethylformamide (DMF), and polyvinylpyrrolidone (PVP, molecular weight ∼30
000) were all purchased from Beijing Chemical Reagents Co. Ltd. Propoxylated glyceryltriacrylate (POGTA) was purchased from Tianjin Tianjiao Chemical Co. Ltd. 2,2-Dimethoxy-2-phenylacetophenone (Irgacure651), as photoinitiator, was purchased from Jingjiang Hongtai Chemical Engineering Co. Ltd. All the chemicals were used without further purification.
2.2 Preparation of initial precursor solutions
A typical precursor solution was synthesized as follows: TTB was dissolved in the mixture of ethanol and DMF and stirred for 10 min. Then, the mixture of nitric acid and water was added under ice–water cooling, in order to hydrolyze the inorganic precursor slowly. After removal from the ice–water cooling, POGTA, Irgacure 651 (1 wt% of POGTA), and PVP (2 wt% of the whole above sol) were slowly added and stirred until fully dissolved, respectively. The molar ratio of TTB, ethanol, DMF, HNO3, H2O and POGTA was 1
:
8
:
4
:
0.5
:
3
:
0.9.
2.3 Preparation of bilayer film
The bilayer-structured composite electrode was prepared as follows. To obtain the composite electrode, Degussa P25 (25 nm-sized TiO2 nanoparticles) was firstly coated over the fluorine-doped tin oxide (FTO) substrate by the doctor blade technique. Then, TiO2 films with net-like framework were deposited by dip-coating the P25/FTO substrate at constant withdrawal rate of 2 cm min−1. Immediately, the as-obtained fresh films were cured by UV light (generated by a 16W low pressure mercury lamp, λmax = 253.7 nm; the distance between films and lamp was about 5 cm) for 5 min, and the resultant gel films were calcined at 200 °C for 20 min and then at 500 °C for 60 min. The whole procedure is displayed in Scheme 1.
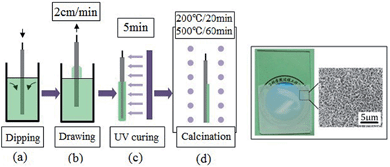 |
| Scheme 1 The preparation procedure of porous TiO2 film with net-like frameworks: (a) dipping in the precursor solutions for TiO2 coatings, (b) withdrawal step, with a lifting speed of 2 cm min−1, (c) UV curing step and formation of the gel film on the glass slide, (d) calcination steps to form the porous TiO2 film with net-like frameworks, treating the gel film at 200 °C for 20 min and then at 500 °C for 60 min, (e) porous TiO2 film obtained by dip-coating from precursor solutions. | |
2.4 Characterization
SEM images were obtained using a JEOL JSM-6700F scanning electron microscope at 3.0 kV. UV-vis spectrophotometry was carried out with UNICO UV-2802PC. Nitrogen adsorption–desorption isotherms were collected using Quantachrome Instruments Autosorb-1 equipment on samples scratched off as-prepared films. The I–V characteristics of the cells were measured using an electrochemical analyzer (CHI630A, Chenhua Instruments Co., Shanghai) under solar simulator illumination (CMH-250, Aodite Photoelectronic Technology Ltd, Beijing) at room temperature. The monochromatic incident photon-to-electron conversion efficiency (IPCE) was measured by illumination with monochromatic light, which was obtained using a series of light filters with different wavelengths. The EIS was carried out on a Zahner IM6e impedance analyzer (Germany) in the frequency range of 0.02 Hz to 100 kHz, with illumination of 100 mW cm−2.
2.5 Photocatalysis test
The photocatalytic activity of TiO2 films was tested through their degradation of methylene blue (MB) in aqueous solution. An 18 W UV fluorescent bulb with 365 nm was used as the light source. In each run, TiO2 film (all samples were cut into squares with the same sizes of about 7.0 mm × 25.6 mm) was added into 2 mL of MB solution (2 × 10−5 M). Prior to the photocatalytic reaction, the system was kept in the dark, and the concentration of MB was monitored. The concentration of MB did not change after 60 min of reaction time, which indicates that it is enough to reach the adsorption equilibrium of MB. The MB concentration was determined by measuring the maximum absorbance at around λ = 664 nm in the UV-vis absorption spectra.
3 Results and discussion
3.1 The morphology and structure of films
The evolution of film morphology is shown in Fig. 1. The non-UV irradiated gel film obtained via the dip-coating method had many cracks in the film (Fig. 1a). However, after UV irradiation, some net-like frameworks of the hybrid polymer–titania composite with large open cavities (0.2–1.5 μm) could be observed in the gel film (Fig. 1b). After further calcinations, porous TiO2 film with an anatase phase was obtained (for XRD pattern, see Fig. S1 in ESI†). The as-prepared, porous TiO2 film shows a well-connected, net-like framework, forming three-dimensional porous structures (Fig. 1c). Compared to the porous gel film, the net-like framework is slender, changing from 600 nm to 150 nm, which may be attributed to the elimination of organic parts and shrinkage of inorganic parts during the heat-treatment process. From the high-magnification SEM image of the TiO2 film (Fig. 1d), it is obvious that its net-like framework is composed of nanoparticles of around 25 nm, and there is a highly porous structure formed by the net-like frameworks with pore openings of between 200 nm to 1.5 μm. This was also supported by TEM and HRTEM images of the film (see Fig. S2 in ESI†). To determine the specific surface area of the NF film, Brunauer–Emmett–Teller (BET) nitrogen absorption–desorption analysis was performed. The BET surface area is 46 m2 g−1, and the total porous volume is 0.14 cm3 g−1. The materials present the typical type IV N2 sorption isotherms with an H3 hysteresis loop, which is usually considered to be indicative of gas adsorption in the mesopores.29 The pore size was 3.8 nm (see Fig. S3 in ESI†).
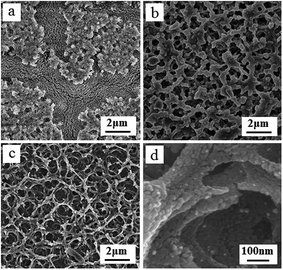 |
| Fig. 1 SEM images of (a) non-UV cured gel film, (b) UV cured gel film, (c) porous TiO2 film obtained after calcination, (d) high magnification image of the film in (c). | |
3.2 FTIR analysis of UV-cured gel film
Fig. 2 shows the FT-IR spectra of the POGTA monomer, including the unirradiated gel film and the irradiated gel film. Some peaks appeared at 1638 cm−1 and 1620 cm−1, which are assigned to C
C stretching of acrylates in the POGTA monomer.30 These peaks are observed distinctly in the spectra of the unirradiated gel film, despite the disturbance from a wide strong peak around 1650 cm−1 attributed to C
O stretching of PVP31 (Fig. 2b), while they are undistinguishable in that of the irradiated gel film. Moreover, the peaks of other groups in POGTA could be easily identified in the spectra of both unirradiated and irradiated gel films. All of the above results indicate that the monomers in the film were polymerized even under UV irradiation for a short time, which has also been concluded in other reports.30,32
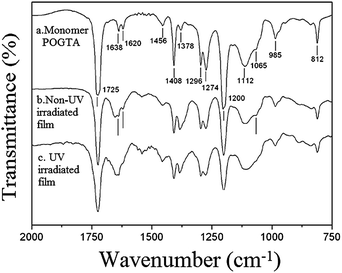 |
| Fig. 2 FTIR spectra of (a) monomer POGTA, (b) the non-UV irradiated gel film, and (c) the UV irradiated gel film. | |
3.3 The influence of precursor formulation
The prepared composite film showed a good DL structure. However, the NF layer structure was highly affected by the initial precursor solution, which then further influenced the light scattering and photoelectric conversion efficiency. Accordingly, a detailed investigation of the influence of precursor formulation was taken from theory into experiment.
In the initial precursor solution, the originating hydrolysis and condensation of TTB hardly occurred because of the highly acidic condition and low water content. It could be proposed that only small oligomeric Ti-oxo clusters were mainly formed. PVP is an amphiphilic polymer that can interact with the Ti-oxo clusters by hydrogen bonding, forming a composite Ti-oxo-PVP,33 as shown in Scheme 2a. In the UV irradiation process, radicals can be produced not only on photosensitive monomer POGTA, but also on macromolecular PVP chains. If the molar ratio between POGTA and PVP is suitable, the organic monomer will be surrounded by the macromolecular chains of PVP. As a result, the UV-induced photo-graft-polymerization of POGTA and PVP becomes the dominating reaction approach,34,35 as shown in Scheme 2b. If the above two interactions take place at same time, the fresh dip-coated film is no longer homogeneous in the UV curing process. Moreover, the composites of Ti-oxo-PVP congregate, driven by the graft-polymerization of POGTA to PVP. During the congregating process, a network may be fabricated. On the other hand, due to the evaporation of solvent in the fresh film, inorganic polymerization will be accelerated and subsequently solidify the network. The areas where Ti-oxo-PVP composites are absent form cavities after evaporation of the solvent. Finally, the porous gel film is prepared by the UV-curing process. Further, by appropriate calcinations, the porous structure is maintained, and porous TiO2 film is synthesized.
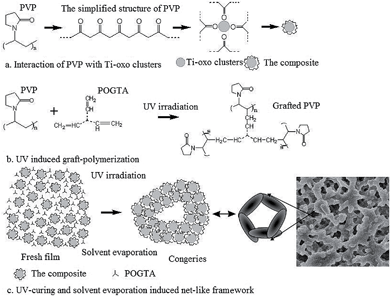 |
| Scheme 2 The possible mechanism for the synthesis of porous TiO2 films with net-like framework by the UV-curing method. | |
A detailed experimental study was conducted on the precursor solutions. The molar ratio of solvent (EtOH–DMF = 2, 1 and 0.5) was changed based on the typical composition of the precursor sol; different morphologies of TiO2 films, i.e., TF-0, TF-1 and TF-2, were prepared, whose SEM images are shown in Fig. 3. Along with the increasing molar ratio of DMF, the apparent porosity of the as-prepared TiO2 films clearly decreases. TF-0 is a porous film with a net-like framework, just as shown in Fig. 1d. TF-1 preserves the net-like framework, but has a larger size. For TF-2, the morphology of the net-like framework disappeared and was replaced by sparse round holes. Fig. 3d indicates that TF-2 is composed of TiO2 nanoparticles. It is obvious that the apparent porosity of the as-prepared TiO2 films sharply decreased as the molar ratio of DMF increased. In order to understand the above phenomenon, the thermal dynamic property of the binary mixed solution EtOH–DMF was investigated. It was found that excess functions of the solution, such as excess intermolecular free length and excess free energy, achieved minima when the molar fraction of DMF was 0.35, indicating the strong interaction between DMF and ethanol molecules, leading to the formation of a DMF–ethanol complex.36 Therefore, the volatility and saturated vapor pressure of the mixed solution is minimized as the molar ratio of solvent EtOH–DMF = 2 (xDMF ≈ 0.35), maintaining the wetness of the fresh dip-coated film for the longest time. Moreover, the wetness of the fresh film benefits UV-induced polymerization of monomers and leads to matter transfer in the fresh film, which is the reason for the preparation of the porous TiO2 film. Consequently, as EtOH–DMF = 2, the prepared TiO2 film, TF-0, exhibits the highest porosity, and as the DMF molar ratio increases, the apparent porosity of the as-prepared TiO2 films reduces.
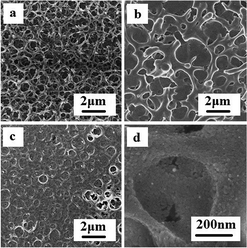 |
| Fig. 3 SEM images of (a) TF-0, (b) TF-1 and (c) TF-2 films when the molar ratio of solvent EtOH–DMF = 2, 1 and 0.5, respectively; high magnification of TF-2 (d). | |
Changing the molar ratio of nitric acid (HNO3/TTB = 0.3, 0.5, 0.7 and 0.8) based on the typical composition of the precursor sol yielded different morphologies of TiO2 films, i.e. TF-3, TF-0, TF-4 and TF-5, whose SEM images are shown in Fig. 4. Considering that inorganic polymerization can be readily controlled by the acidity of precursor sol, restraining the inorganic polymerization rate to the equivalent of the UV-induced organic polymerization rate efficiently utilizes the structure-directing agent synthesized in situ. The molar ratio (HNO3/TTB = 0.5) may be the optimum. A porous film, TF-0, with a net-like framework was fabricated. When the acidity was reduced to HNO3/TTB = 0.3, indiscriminate condensation of inorganic precursors took place, hindering the template effect of the organic polymer synthesized in the UV irradiation process. Ultimately, film TF-3, bearing only sparse holes, was formed. Improving the acidity to HNO3/TTB = 0.7 increased the UV-induced organic polymerization rate, thus increasing the size of the fabricated net-like framework in TF-4. However, when the acidity increased to HNO3/TTB = 0.8, possibly due to the high density of inorganic colloids, the interaction between the inorganic colloid and the structure-directing agent was weakened. Therefore, the net-like framework morphology disappeared, being replaced as the nearly-dense film TF-5 was prepared. The high-magnification SEM images of the as-prepared TiO2 films indicated that all the films were composed of TiO2 nanoparticles.
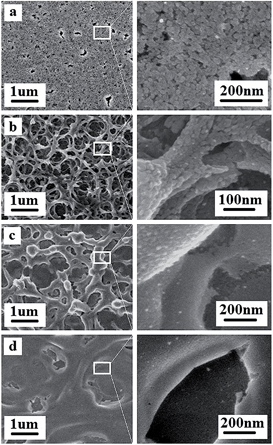 |
| Fig. 4 SEM images of (a) TF-3, (b) TF-0, (c) TF-4 and (d) TF-5 films when the molar ratio HNO3/TTB = 0.3, 0.5, 0.7 and 0.8, respectively. | |
Changing the amount of PVP (0 wt%, 1 wt%, 2 wt%, 4 wt%, 5 wt% and 6 wt%) based on the typical composition of the precursor sol yielded different morphologies of TiO2 films, i.e. TF-6, TF-7, TF-0, TF-8, TF-9 and TF-10, as shown in SEM images in Fig. 5. Apparently, when no PVP was added, only small islands grew on the dense film. When the amount of PVP added was up to 1 wt%, the small islands connected with each other to form a tree-like structure. Increasing the added PVP to 2 wt% resulted in a net-like framework. Some cracks appeared, in addition to the net-like framework, when it was increased to 4 wt%. However, when PVP addition achieved 5 wt%, the net-like framework covering the whole region of the film began to disappear, while a lot of local features with net-like frameworks still remained. When the PVP addition was increased to 6 wt%, the net-like framework disappeared completely, resulting in a nearly dense film. Therefore, PVP addition is a key parameter controlling the formation or reduction of the net-like framework.
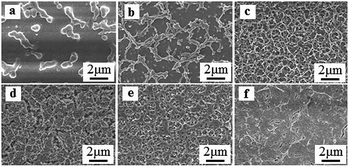 |
| Fig. 5 SEM images of (a) TF-6, (b) TF-7, (c) TF-0, (d) TF-8, (e) TF-9 and (f) TF-10 films when PVP added was 0 wt%, 1 wt%, 2 wt%, 4 wt%, 5 wt% and 6 wt%, respectively. | |
Changing the molar ratio of organic monomer (POGTA/TTB = 0.3, 0.6, 0.9, 1.0 and 1.1) based on the typical composition of the precursor sol yielded different morphologies of TiO2 films, i.e. TF-11, TF-0, TF-12, TF-13 and TF-14, whose SEM images are shown in Fig. 6. UV-induced organic monomer polymerization is the main mechanism for the preparation of porous TiO2 film. Therefore, when no organic monomer was added, only dense film composed of nanoparticles was synthesized (see ESI, Fig. S4†). POGTA/TTB = 0.9 may be the optimized molar ratio. Possibly due to the appropriate size of structure-directing agents formed during the UV irradiation process using this ratio, the porous film with largest apparent porosity and the highest amount of net-like frameworks was formed. When the monomer amount was reduced, no net-like framework was formed, and the porosity of prepared films (TF-11 and TF-0) decreased. However, upon improving the amount of monomer to POGTA/TTB = 1.0 and 1.1, as sufficient templates were synthesized, the porosity of films TF-13 and TF-14 also decreased compared to TF-12. The obtained DL-films with different NF layer morphology, i.e., DL-TF-11, DL-TF-0, DL-TF-12, DL-TF-13 and DL-TF-14, were used as the composite electrodes to investigate their photocatalytic properties.
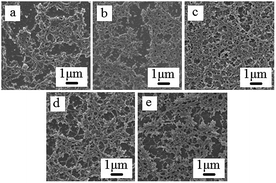 |
| Fig. 6 SEM images of: (a) TF-11, (b) TF-0, (c) TF-12, (d) TF-13 and (e) TF-14 films when the molar ratio POGTA/TTB = 0.3, 0.6, 0.9, 1.0 and 1.1, respectively. | |
3.4 Photovoltaic behavior of DSSCs
As shown in Fig. 7a, the TiO2 films with a net-like framework were used as the scattering layer to form a novel DL-film which was used as the photoanode of DSSCs. The overlayer seemed to be firmly attached on the P25 layer, whose thickness is about 3 μm (Fig. 7b). The photocatalytic properties of the prepared photoanodes are summarized in Table 1. The sample DL-TF-12 was found to display the best photovoltaic performance, with a short-circuit photocurrent density (Jsc) of 14.28 mA cm−2, open-circuit voltage (Voc) of 0.68 V, and conversion efficiency (η) of 6.57%. However, the BET surface area of 35.7 m2 g−1 for this sample was the lowest value among all the samples. The improvement of photoelectron utilization is attributed to the network layer with plentiful branches of the material, which can effectively improve light scattering. Hence, more solar energy is constrained in the solar cells, increasing light intensity and improving the photocurrent. The DL-TF-12 network layer used for the following research had a thickness of 3 μm.
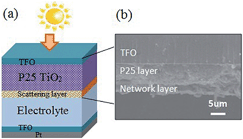 |
| Fig. 7 (a) Schematic diagram of the dye-sensitized solar cell: the photoanode was prepared using a novel bilayer-structured film that uses the TiO2 films with a net-like framework as the scattering layer on top of the P25 layer. (b) The cross-section SEM image of the as-prepared, bilayer-structured film. | |
Table 1 Photovoltaic properties of the DSSCs composed of bilayer film photo electrodes with different net-like framework layersa
Electrodeb |
Voc (V) |
Isc (mA cm−2) |
FF (%) |
η (%) |
Photovoltaic parameters of different electrodes. Measurement condition: simulated sunlight (AM1.5, 100 mW cm−2). The sensitizer was N3 (ruthenium dye). The cell active area was 0.20 cm2 and the light intensity was 100 mW cm−2. The values shown are from cells, based on the averages of about ten samples. The P25 layer and network layer thickness were 10 μm and 3 μm, respectively. |
DL-TF-11 |
0.67 |
13.21 |
70.84 |
6.27 |
DL-TF-0 |
0.67 |
13.92 |
68.09 |
6.35 |
DL-TF-12 |
0.68 |
14.28 |
67.66 |
6.57 |
DL-TF-13 |
0.67 |
13.52 |
71.20 |
6.45 |
DL-TF-14 |
0.67 |
13.22 |
71.35 |
6.32 |
The effect of the thickness of the P25 layer was also investigated. The photovoltaic performance of DL-films with different thicknesses of P25 layer (7 μm, 8 μm, 9 μm and 10 μm), i.e. DL-P-7, DL-P-8, DL-P-9 and DL-P-10, are summarized in Table 2, and a pure P25 single-layer film (SL-film) was tested as control. The cells derived from all the DL-films demonstrated considerably improved conversion efficiency (η) compared to that of the SL-film. With increasing the thickness of the P25 layer of DL-films, conversion efficiency was firstly increased then decreased, and the 8 μm thick P25 layer showed the best conversion efficiency (6.95%), which increased by 12.6% compared to the SL-film. However, the saturated dye adsorption capacity of DL-film (1.27 × 10−7mol cm−2) was lower than that of P25 (1.36 × 10−7mol cm−2); the dye adsorption capacity was reduced by seven percent, which may be attributable to the considerably lower surface areas of the DL-film. It means the DL-film has a higher utilization of the adsorbed photoelectrons. Accompanied by the branches of the network layer, light scattering was enhanced, and cell performance was improved as well. This can be proven by further optical measurements. Sample DL-P-8 was used to compare with the P25-only layer owing to its outstanding photovoltaic performance.
Table 2 Photovoltaic properties of the DSSCs composed of bilayer photo electrodes with different thicknesses of the P25 layera
Electrodeb |
Voc (V) |
Isc (mA cm−2) |
FF (%) |
η (%) |
Photovoltaic parameters of different electrodes. Measurement conditions: simulated sunlight (AM1.5, 100 mW cm−2). The sensitizer was N3 (ruthenium dye). The cell active area was 0.20 cm2, and the light intensity was 100 mW cm−2. The values shown are from cells, based on averages of about ten samples. The net-like framework layer was prepared by POGTA/TTB = 0.9, and the thickness was 3 μm. |
SL-P25 |
0.69 |
15.58 |
57.39 |
6.17 |
DL-P-7 |
0.71 |
16.41 |
59.05 |
6.88 |
DL-P-8 |
0.69 |
17.07 |
59.01 |
6.95 |
DL-P-9 |
0.69 |
16.06 |
59.83 |
6.63 |
DL-P-10 |
0.68 |
14.18 |
68.24 |
6.58 |
3.5 UV-vis
The reflectivity of each film with and without dye loading was studied to investigate the scattering efficiency of the DL-film and SL-film P25, Fig. 8a. Compared to the SL-film electrode, the DL-film with the network layer brings an increasing reflectance over the entire wavelength range. Before the dye adsorption, both DL-film and SL-film had high reflectivity. Because the pore size of the network layer is around the wavelength of visible light, the DL-film showed better light-scattering ability compared to the SL-film. After dye adsorption, the incident light was absorbed by the dye molecules; the reflectance of the DL-film decreased drastically to ca. 33% in the short wavelengths ranging from 400 to 550 nm (Fig. 8b). However, the dye-absorbed DL-film retained a substantially higher reflectance than the dye-absorbed P25 film in the wavelength region over 450 nm, which further supports our argument that the better light scattering property of the network layer structure results in higher conversion efficiency of the DL-film compared to P25 SL-film.
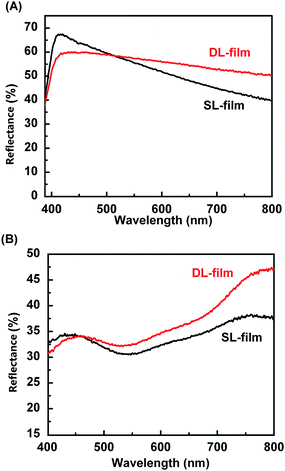 |
| Fig. 8 Diffused reflection patterns of the single-layer film (SL-film: only P25 layer) and double layer film (DL-film: TiO2 films with net-like framework on top of the P25 layer, sample DL-P-8) (A) before dye absorbed; (B) after dye absorbed. | |
3.6 Incident photon-to-current conversion efficiency (IPCE) performance
The IPCE spectra for the DSSC cells provide further evidence on the scattering effect of the bilayer structures (Fig. 9). Compared to SL-film, the IPCE response over the entire range of light was enhanced. The peak value of the IPCE was increased by 30% for IPCE630 with DL-film electrode. The enhanced IPCE provides more evidence of the superior optical characteristic of the bilayer structures of DL-film. The DL-film electrode showed a higher incident photon-to-current conversion efficiency. An enhancement in light conversion efficiency from 580 nm until far in the absorption tail (up to 750 nm) can be observed, which may be attributed to the superior light scattering properties of the network layer on top of the DL-film, especially in the long-wavelength region. This result is highly consistent with the UV-vis diffuse reflectance spectrum.
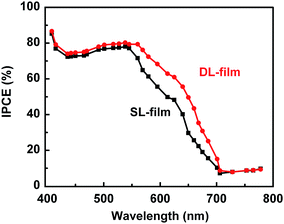 |
| Fig. 9 The normalized IPCE spectra of DSSCs for the single-layer film (SL-film: only P25 layer) and double-layer film (DL-film: TiO2 films with net-like framework on top of the P25 layer, sample DL-P-8). | |
3.7 Electrochemical impedance spectra (EIS) measurement of different electrodes
EIS is a useful method to clarify the electronic and ionic transport processes in DSSCs. To further confirm the effect of the network layer in enhancing the light scattering in DSSCs, the SL-film and DL-film were characterized by EIS, measured under the illumination of one sun at open-circuit potential. As shown in Fig. 10, the Nyquist plots consist of two semicircles. The biggest semicircle at the medium frequencies is attributed to photo injected electrons in the TiO2 which should be considered in the device design. As shown, the semicircle at the intermediate frequency region decreased for the DL-film electrode, indicating the fast redox activity of the electrolyte in the working electrode interface.
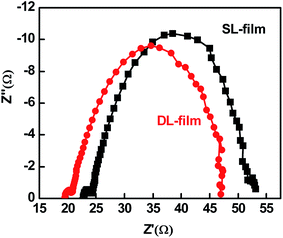 |
| Fig. 10 Nyquist of the electrochemical impedance spectra of electrodes prepared by single-layer film (SL-film: only P25 layer) and double-layer film (DL-film: TiO2 films with net-like framework on top of the P25 layer, sample DL-P-8). | |
4 Conclusions
A DL-film with the NF layer on top of the P25 layer was prepared and applied as a photoanode of dye-sensitized solar cells (DSSCs). The NF layer, with hierarchically meso-macroporous structures, was prepared via a UV light-induced in situ polymerization of hybrid organic–inorganic films containing propoxylated glyceryltriacrylate monomers and titania precursors. A tentative mechanism was proposed to elucidate the preparation of porous TiO2 film by UV-induced in situ polymerization. The corresponding results of DSSCs made with DL-films were compared to those with SL-films. It was observed that DSSCs prepared with DL-films showed higher conversion efficiency than those with SL-films. Furthermore, the highest efficiency (6.95%) is realized for DSSCs made using DL-film with a 3 μm NF layer (prepared by POGTA/TTB = 0.9) and 8 μm P25 layer. Nevertheless the saturated dye adsorption capacity of DL-film (1.27 × 10−7 mol cm−2) was lower than that of P25 (1.36 × 10−7mol cm−2), which means the DL-film has a higher utilization of the adsorbed photoelectron. Promoted by the branches of the network layer, light scattering was enhanced, improving cell performance.
Acknowledgements
The authors are grateful for financial support from the National Natural Science Foundation of China (nos. 21031005, 21203201, 51302266, 91122014, 51172235, 21325105, 21201167), National Science Fund for Distinguished Young Scholars (no. 21325105), the Foundation for State Key Laboratory of Biochemical Engineering (no. 2012KF-08), Scientific Research Foundation for the Returned Overseas Chinese Scholars, State Education Ministry.
Notes and references
- B. O'regan and M. Grfitzeli, Nature, 1991, 353, 737–740 CrossRef.
- M. Grätzel, Nature, 2001, 414, 338–344 CrossRef PubMed.
- M. Yang, D. Kim, H. Jha, K. Lee, J. Paul and P. Schmuki, Chem. Commun., 2011, 47, 2032–2034 RSC.
- P. M. Sommeling, B. C. O'regan, R. R. Haswell, H. J. P. Smit, N. J. Bakker, J. J. T. Smits and J. A. M. Van Roosmalen, J. Phys. Chem. B, 2006, 110, 19191–19197 CrossRef CAS PubMed.
- S. H. Ko, D. Lee, H. W. Kang, K. H. Nam, J. Y. Yeo, S. J. Hong and H. J. Sung, Nano Lett., 2011, 11, 666–671 CrossRef CAS PubMed.
- H. J. Koo, Y. J. Kim, Y. H. Lee, W. I. Lee, K. Kim and N. G. Park, Adv. Mater., 2008, 20, 195–199 CrossRef CAS.
- Y. Qiu, W. Chen and S. Yang, Angew. Chem., Int. Ed., 2010, 122, 3757–3761 CrossRef.
- N. Yang, J. Zhai, D. Wang, Y. Chen and L. Jiang, ACS Nano, 2010, 4, 887–894 CrossRef CAS PubMed.
- X. Wu, G. Q. M. Lu and L. Wang, Energy Environ. Sci., 2011, 4, 3565–3572 CAS.
- N. Yang, Q. Yuan, J. Zhai, T. Wei, D. Wang and L. Jiang, ChemSusChem, 2012, 5, 572–576 CrossRef CAS PubMed.
- J. G. Nam, E. S. Lee, W. C. Jung, Y. J. Park, B. H. Sohn, S. C. Park, J. S. Kim and J. Y. Bae, Mater. Chem. Phys., 2009, 116, 46–51 CrossRef CAS PubMed.
- M. Zukalova, A. Zukal, L. Kavan, M. K. Nazeeruddin, P. Liska and M. Grätzel, Nano Lett., 2005, 5, 1789–1792 CrossRef CAS PubMed.
- K. Zhu, N. R. Neale, A. Miedaner and A. J. Frank, Nano Lett., 2007, 7, 69–74 CrossRef CAS PubMed.
- I. G. Yu, Y. J. Kim, H. J. Kim, C. Lee and W. I. Lee, J. Mater. Chem., 2011, 21, 532–538 RSC.
- I. G. Yu, Y. J. Kim, H. J. Kim, C. Lee and W. I. Lee, J. Mater. Chem., 2011, 21, 532–538 RSC; J. Y. Liao, B. X. Lei, D. B. Kuang and C. Y. Su, Energy Environ. Sci., 2011, 4, 4079–4085 Search PubMed.
- J. Y. Liao, B. X. Lei, H. Y. Chen, D. B. Kuang and C. Y. Su, Energy Environ. Sci., 2012, 5, 5750–5757 CAS.
- Z. S. Wang, H. Kawauchi, T. Kashima and H. Arakawa, Coord. Chem. Rev., 2004, 248, 1381–1389 CrossRef CAS PubMed.
- J. Du, X. Lai, N. Yang, J. Zhai, D. Kisailus, F. Su, D. Wang and L. Jiang, ACS Nano, 2011, 5, 590–596 CrossRef CAS PubMed.
- S. Hore, P. Nitz, C. Vetter, C. Prahl, M. Niggemann and R. Kern, Chem. Commun., 2005, 15, 2011–2013 RSC.
- J. H. Yoon, S. R. Jang, R. Vittal, J. Lee and K. J. Kim, J. Photochem. Photobiol., A, 2006, 180, 184–188 CrossRef CAS PubMed.
- I. S. Cho, Z. Chen, A. J. Forman, D. R. Kim, P. M. Rao, T. F. Jaramillo and X. Zheng, Nano Lett., 2011, 11, 4978–4984 CrossRef CAS PubMed.
- W. Peng and L. Han, J. Mater. Chem., 2012, 22, 20773–20777 RSC.
- D. Grosso, G. Soler-Illia, F. Babonneau, C. Sanchez, P. A. Albouy, A. Brunet-Bruneau and A. R. Balkenende, Adv. Mater., 2001, 13, 1085–1090 CrossRef CAS.
- E. L. Crepaldi, G. J. d. A. Soler-Illia, D. Grosso, F. Cagnol, F. Ribot and C. Sanchez, J. Am. Chem. Soc., 2003, 125, 9770–9786 CrossRef CAS PubMed.
- W. Zhou and H. Fu, ChemCatChem, 2013, 5, 885–894 CrossRef CAS.
- M. Zukalova, A. Zukal, L. Kavan, M. K. Nazeeruddin, P. Liska and M. Grätzel, Nano Lett., 2005, 5, 1789–1792 CrossRef CAS PubMed.
- K. Hou, B. Z. Tian, F. Y. Li, Z. Q. Bian, D. Y. Zhao and C. H. Huang, J. Mater. Chem., 2005, 15, 2414–2420 RSC.
- C. Decker, L. Keller, K. Zahouily and S. Benfarhi, Polymer, 2005, 46, 6640–6648 CrossRef CAS PubMed.
- J. Qi, J. Chen, G. D. Li, S. X. Li, Y. Gao and Z. Y. Tang, Energy Environ. Sci., 2012, 5, 8937–8941 CAS.
- P. Yuan, H. He, F. Bergaya, D. Wu, Q. Zhou and J. Zhu, Microporous Mesoporous Mater., 2006, 88, 8–15 CrossRef CAS PubMed.
- V. Vijayabaskar, S. Bhattacharya, V. K. Tikku and A. K. Bhowmick, Radiat. Phys. Chem., 2004, 71, 1045–1058 CrossRef CAS PubMed.
- C. Leger, Q. T. Nguyen, J. Neel and C. Streicher, Macromolecules, 1995, 28, 143–151 CrossRef CAS.
- A. Ali, A. K. Nain and M. Kamil, Thermochim. Acta, 1996, 274, 209–221 CrossRef CAS.
- J. M. Song, J. H. Zhu and S. H. Yu, J. Phys. Chem. B, 2006, 110, 23790–23795 CrossRef CAS PubMed.
- H. J. Chun, S. M. Cho, Y. M. Lee, H. K. Lee, T. S. Suh and K. S. Shinn, J. Appl. Polym. Sci., 1999, 72, 251–256 CrossRef CAS.
- P. S. Majumder and A. K. Bhowmick, J. Appl. Polym. Sci., 2000, 77, 323–337 CrossRef CAS.
Footnotes |
† Electronic supplementary information (ESI) available. See DOI: 10.1039/c4ra05174b |
‡ These authors contributed equally. |
|
This journal is © The Royal Society of Chemistry 2014 |
Click here to see how this site uses Cookies. View our privacy policy here.