DOI:
10.1039/D4VA00085D
(Critical Review)
Environ. Sci.: Adv., 2024,
3, 1030-1038
Glyphosate: a review on its widespread prevalence and occurrence across various systems
Received
19th March 2024
, Accepted 24th May 2024
First published on 25th May 2024
Abstract
Glyphosate (GLY), a versatile herbicide with several applications, has become quite popular for controlling weed growth in residential, commercial, and agricultural settings. Its widespread acceptance has been facilitated by its effectiveness and low cost. However, overuse and improper application of GLY have become an urgent concern, raising questions about potential harm to human health and environmental sustainability. Studies have revealed that GLY exhibits toxic properties that can lead to detrimental consequences for human well-being. These include the potential to induce cancer, contribute to birth defects, and disrupt reproductive functions. Moreover, when exposed to non-target organisms, GLY has been found to inflict adverse impacts on various forms of aquatic life, insects, and essential soil microorganisms. Because of its great solubility and low quantities in soil and water, GLY detection is a difficult process. In response to the concerns surrounding GLY, several detection techniques have been devised, encompassing chromatography, immunoassays, and mass spectrometry. These methods play a crucial role in investigating the ramifications associated with GLY application in agriculture and the environment. The study also emphasizes the need for continued research to fully understand the long-term effects of GLY exposure on human health and the environment.
Environmental significance
The occurrence and chemistry of glyphosate in the environment, its impact on living beings, and its detection in the environmental system are central themes of this review. GLY, a herbicide, has become a matter of environmental and public health concern in recent years. Although it is effective in weed control, its excessive use has negative effects on ecosystems, biodiversity, and precious soil. The overuse of GLY has prompted the search for alternatives, which is a topic of discussion in both the scientific community and civil society. Numerous chromatographic and immunoassay-based detection techniques, as well as precision-era sensitive detection techniques, have been developed and utilized for monitoring and regulating GLY.
|
1. Introduction
In the modern world of agriculture, herbicides and pesticides have emerged as powerful tools, enabling farmers to combat pests and weeds masterfully, and thereby ultimately boosting production to new heights. However, the widespread use of these chemicals has also raised concerns about their impact on human health and the delicate balance of our environment. One such herbicide glyphosate (GLY) or N-phosphomethyl glycine is used most widely due to its effectiveness and versatility.1 Dr John Franz, a chemist at Monsanto, made a ground-breaking discovery on the herbicidal properties of GLY in 1970. This important finding paved the way for the much-praised Roundup's commercial debut in 1974.2 It was approved and registered in the USA in 1974 and underwent re-registration in 1983. Subsequently, it had to be registered separately in each country under varying conditions in Europe. The European Union (EU) introduced a harmonized registration system in 1991, requiring the re-registration of “old” active ingredients. GLY went through evaluation, with Germany as the Rapporteur Member State, and was included in Annex I of Council Directive 91/414/EEC. Its authorization was renewed under subsequent regulations in 2011 and 2012.3 Regulatory agencies in the US further divided pesticide chemicals into two groups: active components and inert ingredients. A pest or weed is a target organism; active components are compounds that directly inhibit the growth of a pest. Ingredients are termed inert and are added to the pesticide formulation to enhance its overall performance but do not have any direct pesticidal activity.4 GLY is an active ingredient of nearly 750 formulations, e.g. isopropyl amine (IPA), diammonium, mono ammonium, potassium, trimethyl sulfonium, and sesquisodium salts.4 Polyoxyethylene amine (POEA) is one such formulation that is usually used to increase the uptake and transfer of active ingredients in the plant body. The most prevalent POEA-based weed control product, Roundup, is used throughout the world and is believed to be more hazardous than pure GLY.4,5 To minimize weeds and other undesirable plant growth, glyphosate-based herbicides (GBHs) are now widely used in forestry, agriculture, and other sectors such as verges, railway sidings, and land preparation for grass seeding.6 The compound enters through different modes, such as leaves, roots, stems, and trunks, resulting in the inhibition of the shikimate pathway. It is generally considered to have low toxicity for animals because it functions by inhibiting an enzyme pathway that is specific to plants and not found in animals. In both agricultural and non-agricultural environments, GLY is a favoured weed killer due to its selective toxicity.6 There are almost hundreds of commercialized GBHs used in more than 100 countries, and nearly 600–750 thousand tonnes are used annually.7,8 Crops using GLY more frequently are soybeans, field corn, pasture, wheat, and hay.2,9 About an average of 1 kg hectare−1 of GLY is used to control specific weed species by minimizing their impact on the main crops.2 However, the quantity is not fixed, and it may vary depending on different geographical locations, crops, and government policies. Further, more details are available in previous papers.2,9
Detecting GLY can be quite challenging despite its advantages due to its presence in low concentrations and susceptibility to various toxic effects. However, different analytical methods are used for GLY detection, including chromatography-based methods, immunoassays, and molecular methods. These methods offer varying degrees of sensitivity, specificity, and practicality depending on the application and the matrix of interest.1 Accurate detection of GLY is crucial for several reasons, including monitoring for potential exposure in occupational and environmental settings, ensuring compliance with regulatory limits, and assessing the safety and quality of food and water supplies. Moreover, detecting GLY can help to inform decisions about the appropriate use and management of this herbicide, including efforts to minimize its potential impacts on human health and the environment. Thus, continued efforts to develop and refine GLY detection methods are likely to remain an important area of research and development in the years to come.
This review extensively discusses the detrimental impacts of GLY on both human health and the environment, along with the challenges associated with its detection across multiple parameters. Emphasizing the necessity for ongoing research and the exploration of innovative approaches to mitigate potential risks, this review underscores the importance of GLY monitoring.
2. Insight into the chemical and physical features of GLY
The GLY herbicide is a relatively tiny and polar chemical compound with a molecular weight of 169.05 g mol−1.10 GLY is sparingly soluble in organic solvents, such as ethanol and acetone, but it is highly soluble in water. Its solubility is inversely pH dependent, demonstrating higher solubility at a lower pH value.11 The chemical and physical features of GLY, as summarized in Table 1, provide insights into its behaviour and fate in multiple ecosystems.
Table 1 Physio-chemical properties of GLY
Property |
Value |
Chemical name |
N-(Phosphonomethyl) glycine10 |
Molecular formula |
C3H8NO5P10 |
Molecular weight (g mole−1) |
169.07 (ref. 10) |
Solubility (water) |
11.6 g L−1 at 25 °C (ref. 12) |
Melting point |
184 °C (ref. 10) |
Boiling point |
No data available10 |
Vapour pressure |
(1.31 × 10−5 Pa, 25 °C) (ref. 13) |
Log P (octanol/water) |
−1.70 (ref. 14) |
Donor electron pairs |
6 × 10−10 |
van der Waals volume |
129.8 Å3 (ref. 10) |
van der Waals surface area |
220 Å2 (ref. 10) |
Solvent accessible surface area |
322 Å2 (ref. 10) |
Topological polar surface area |
106.9 Å2 (ref. 10) |
Half-life (soil and water) |
1.0 to 67.7 days15,16 |
Degradation to AMPA (Ochrobactrum sp.) |
Complete degradation of GLY within 4 days17 |
3. Deciphering the role of GLY in integrated pest management
GLY is a synthetic phosphonate molecule consisting of a carbon–phosphorus (C–P) link (Fig. 1), which is an important component that contributes to its herbicidal effect. Unlike other herbicides, Gly normally features a carbon–nitrogen (C–N) or carbon–oxygen (C–O) bond. Because of the strong stability of GLY and resistance to deterioration, the herbicide can persist in the environment for up to one year after application at low concentrations.17,18 An IPA salt of GLY, a surfactant, and water are the three major ingredients needed for commercial GLY manufacturing. Because of the existence of the C–P linkage, it is resistant to full decomposition. Nonetheless, biologically induced biodegradation of such chemical is conceivable via two major pathways, namely by the C–P lyase enzyme and oxidase enzyme, which breaks down the C–N bond in GLY.19 It is present in both terrestrial and aquatic habitats due to improper application and overspray because it adheres to soil particles and deposits majorly in the top layer of soil. It disturbs soil microflora by restricting microorganism growth and encouraging plant pathogen growth.17 It also inhibits the acetylcholinesterase enzyme and causes oxidative stress in non-mammalian animals, leading to organ failure.20 The herbicide is believed to be the safest candidate although its excessive use can have long-term impacts on life and the environment. Weed control is critical because weeds compete with the crop, nutrients, sunlight, and space, and also their seeds taint crop harvests and degrade grain quality.6
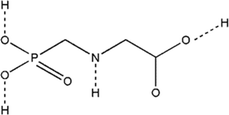 |
| Fig. 1 Chemical composition of GLY, a compound. | |
4. Understanding glyphosate's mechanism: inhibition of the shikimate pathway and its implications
Because GLY is non-selective in nature and not applicable directly to the crop, therefore, it diffuses after being sprayed on plant parts. Surfactants based on ethylene oxide (EO) are highly used to enhance the surface for GLY uptake. One of the most crucial measures is the hydrophile/lipophile balance (HLB), which links the surfactant type and mechanism of action. Low HLB surfactants often have a higher lipophilicity than high HLB surfactants, which allows them to diffuse more easily into the lipophilic cuticle.21 After entering the phloem, the GLY travels through a symplastic pathway and can persist for longer periods. Moreover, the herbicide is transported to the plant xylem through an apoplastic pathway that originates from the roots.22 It can be taken up by roots of different crop species, such as maize, beets, rapeseed, cotton, and barley, with 2–6% of the applied GLY being absorbed by roots within 6–24 h after application.11,23 The enzyme EPSPS, found in plant plastids, facilitates the transfer of the enolpyruvyl group from phosphoenolpyruvate (PEP) to 5-hydroxyl shikimate-3-phosphate, resulting in 5-enolpyruvylshikimate-3-phosphate (EPSP). Upon application to crops, it binds to the enzyme EPSPS and inhibits its functions, as shown in Fig. 2, resulting in hindered plant growth.8,23 The manufacturing of GBHs involves mixing GLY with different formulations to stabilize the active ingredient and enhance its penetration into plant parts.24
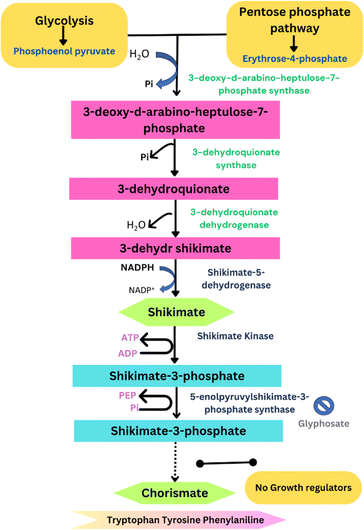 |
| Fig. 2 Inhibition of the enzyme EPSPS by the action of GLY, which results in the absence of growth hormones in plants.25 | |
5. Examining the risks and consequences to health and the environment
5.1. Land and water
After being sprayed, GLY breaks down into two stages: early or primary degradation and secondary or ultimate degradation. GLY first degrades into aminomethylphosphonic acid (AMPA) and glyoxylate, both of which have half-lives of approximately 68 days. There are several common sources of AMPA, including the degradation of organic phosphonates used in water treatment and phosphonic acids used in detergents and industrial boilers in Europe. AMPA combines with Ca2+, Mg2+, Mn2+, and Zn2+ to create metal complexes because of its phosphonate and amine functional groups. AMPA is securely adsorbed to the soil.26 The process for establishing environmental quality standards for AMPA at 450 mg L−1 is provided under the water framework directive.26 Similar to GLY, AMPA breaks down considerably more slowly in soil and water. Its adhesion to particles may be stronger, which explains why cell membrane penetration is reduced. The sediment's concentration of AMPA may vary based on how quickly it degrades compared to GLY.26
During secondary degradation, AMPA further breaks down into various compounds.5 However, the degradation rates of AMPA depend on various factors, such as soil habitat and microflora. Additionally, GLY acts as a potent chelating agent and can form chemical bonds with essential micronutrients, such as calcium, iron, magnesium, nickel, and zinc, present in soil.6 These interactions can impact the availability of these micronutrients for plants and other organisms in the soil ecosystem. It also alters nitrogen metabolism, which affects the rhizobia symbiont and ultimately leads to plant death by affecting the physiology of the host genotypes.20 Earthworms are important in soil ecology because they are used as indicator organisms. They are highly affected by exposure to pure GLY. A previous study stated that 26.3 mg of GLY for one kg of soil containing compost worms can lose their biomass by 14.8 to 25.9%.4 Studies have shown that low dosages of GLY can considerably reduce root mycorrhization with a 40% reduction in arbuscular production noted particularly. Arbuscular mycorrhizal fungal (AMF) spore quantity in the soil is significantly regulated by arbuscules.27
Recent studies have suggested that GLY may affect other aquatic creatures less adversely than fish and amphibians. However, it is still unclear how exposure to GLY can affect the ecological systems of these creatures, underscoring the need for more study in this area.28 In the EU, the drinking water directive has set a maximum allowed concentration (MAC) of 0.1 μg L−1 for pesticides in drinking water.29 In the USA, the environmental protection agency (EPA) allows a MAC of 700 μg L−1 for GLY in drinking water.30 To reduce long-term health effects, regular GLY content assessments and effective water treatment methods are essential. The government and the general public must work together to ensure that the right steps are taken to secure water supplies and defend against pollution of human health. Fig. 3 shows the toxic effects of GLY across different parameters.
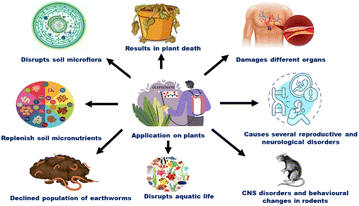 |
| Fig. 3 Illustration of different risks associated with limitless use of GLY altering environment as well as human health. | |
5.2. Plants
The maximum residue limits (MRLs) for GLY and AMPA in agricultural goods exhibit considerable variation based on the commodity and regulatory body. The range extends from 0.1 to 40 mg kg−1 in plant products for human consumption to 530 mg kg−1 in cattle feed and grass.5 The significance of the plant microbiome has long been linked to the plant's health and growth. If GLY disrupts the microbiome in a plant's rhizosphere, phyllosphere, or endosphere, it may decrease competition for attachment sites and decrease the amount of antibiotics produced to fight against infections.5
Additionally, disruptions in the production of aromatic amino acids reduce a plant's mechanical and chemical defence, making it easy for plant pathogens to enter through roots. These pathogens increase the exudation of carbohydrates and amino acids, resulting in reduced plant growth.5,22
This also results in a dose-dependent reduction in CO2 fixation and biomass production.31 GLY acts as a competitive inhibitor of PEP, resulting in end-product inhibition. This prevents the formation of growth regulators and changes the structure of the carbon intermediate.32 The harmful effects of GLY on plants can affect the synthesis of chlorophyll and Rubisco activity, which decreases biomass production and CO2 fixation. Its insidious effects even extend to the C2 cycle, thwarting aniline production and leaving plants struggling to survive (Fig. 4).20
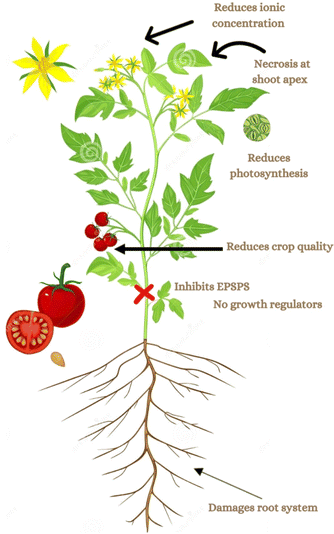 |
| Fig. 4 Illustration of the effects of GLY on several plant components. | |
5.3. Human health
GLY has been a subject of controversy and concern owing to its possible implications on human health. Some studies suggest that exposure to GLY may be linked to an increased risk of cancer, while others suggest no significant association. Additionally, this may disrupt the gut microbiome, potentially contributing to various health issues, such as obesity, diabetes, and autoimmune disorders.33 To further understand the potentially harmful effects of GLY on human health, researchers have investigated its impact on DNA methylation processes. A study conducted by Kwiatkowska et al. examined the effects of GLY and GBHs on the DNA methylation process, which is a crucial epigenetic mechanism regulating gene expression in living organisms. The study found that exposure to GLY resulted in DNA damage, including the formation of alkali-labile sites and repaired DNA lesions, which may lead to generic instability.34
Research has shown that exposure to GLY can lead to reduced sperm motility and DNA fragmentation, which can significantly reduce fertilization rates.35 Additionally, the utilization of GBH develops aseptic meningitis with measurable GLY concentrations in cerebrospinal fluid,33 and RoundUp can develop vasculitic neuropathy.33 Therefore, long-term exposure to GLY may encourage the development of neurological diseases. Table 2 presents the half maximal effective concentration (EC50) values for the different species. Furthermore, high concentrations of GLY in wheat may lead to exposure to harmful microbes, resulting in celiac disease.36 Given the possible negative effects of GLY and GBHs on human health and the environment, it is essential to take caution when using them and to give priority to the creation and use of sustainable and secure substitutes.
Table 2 EC50 values for GLY and its salts in different species
Salt |
EC50 value |
mgAE per L is a specific unit proposed to measure GLY.
|
Toxicity to aquatic species
|
IPA salt of GLY |
4.2 mgAE per La (ref. 43) |
Roundup Quick™ (without POEA) |
48.9 mgAE per La (ref. 43) |
Roundup Max™ (with POEA) |
38.1 mgAE per La (ref. 43) |
![[thin space (1/6-em)]](https://www.rsc.org/images/entities/char_2009.gif) |
Toxicity to bacterial growth
|
IPA salt of GLY |
506 mgAE per La (ref. 43) |
Roundup Quick™ (without POEA) |
706 mgAE per La (ref. 43) |
Roundup Max™ (with POEA) |
548 mgAE per La (ref. 43) |
![[thin space (1/6-em)]](https://www.rsc.org/images/entities/char_2009.gif) |
Toxicity to human cells
|
GLY |
41.13 mgAE per La (ref. 44) |
Fipronil |
37.59 mgAE per La (ref. 44) |
Imidacloprid |
663.66 mgAE per La (ref. 44) |
5.4. Animals and insects
The widespread usage of GLY and GBHs has been found to have harmful effects on animals and insects, including rodents, fish, and honey bees.37 Studies have shown that GLY residues are toxic to the nervous system of several species of animals and can lead to behavioural changes, disturbances in blood flow across the brain, decreased neurotransmission, and impaired synaptic function.37 Similar exposure to GLY can alter the behaviour of rodents, resulting in changes in anxiety levels, depressive-like symptoms, and learning difficulties.30,37,38 By increasing immunotoxicity or overexpression in cells, GLY toxicity in fish might cause changes in the population of fish. It can also weaken immunity, exacerbate inflammation, and diminish antioxidative capacity, all of which can kill fish.39 GLY exposure has been found to have acute toxicity on common carp and can reduce the number of phagocytes and decrease phagocytic potential in catfish.40,41 GLY usage also has an impact on insect pollinators, such as honeybees. The gut microbiota of honeybees is at risk when exposed to GLY, leading to a decrease in beneficial bacterial composition and an increase in opportunistic infections, such as Serratia, which promote mortality.42
6. Exploring analytical techniques for accurate GLY detection
Accurate detection methods are essential for monitoring GLY levels in soil, water, and food products to ensure regulatory compliance and assess potential health risks. Exploring analytical techniques for precise GLY detection involves a multidisciplinary approach, combining advancements in chemistry, biochemistry, and instrumentation.
6.1. Chromatography
The most widely used methods for detecting GLY and AMPA have been gas chromatography (GC) and high-performance liquid chromatography (HPLC), both of which require the derivatization step. However, the direct determination by introducing a detection system having inductively coupled plasma with a triple quadrupole mass spectrometer (ICP-MS/MS) for HPLC may be more advantageous, as it omits the derivatization step.45 Instead of identifying compounds, the ICP-MS/MS has been used to identify heteroatoms, such as the phosphorus contained in the AMPA and GLY molecules. Additionally, using the MS/MS mode, interferences are removed from the matrix, which raises the sensitivity of the method.45 A comparative study was performed between HPLC and HPLC-ICP-MS/MS using different water samples, which showed that the HPLC-ICP-MS/MS method presented less analysis time and no derivatization step. Furthermore, the method's reliability is enhanced by the detector's specificity and the reduced number of sample preparation steps. Phosphorous (P) atom detection provides enhanced specificity and sensitivity with LOD 8.2 μg L−1 compared to LOD of HPLC 300 μg L−1. Only GC also helps in the detection of GLY in different samples, but when it is coupled with tandem mass spectrometry and isotope labelled standards, it provides more selectivity and sensitivity with low LOD (0.05 μg L−1), thereby overcoming any possible matrix effects.46 To overcome quantitative errors caused by the spectral overlap between natural and labelled GLY in the chosen MRM transitions, the isotope pattern deconvolution method has proved to be a useful substitute for the traditional isotope dilution method in the quantification of GLY.46 Using both synthetic and real samples (urine), a thorough analysis of the results revealed that the method is proficient in detecting GLY to evaluate the health risks associated with GLY exposure.
Another chromatographic analysis for different soil samples using liquid chromatography coupled (LC) to mass spectrometry (MS) with triple quadrupole was conducted by Hernández et al.47 Analysis of GLY through LC contains matrix effects (strong ionization suppression) and the presence of interfering compounds in samples to overcome this problem, and a clean-up step after collection of the soil sample was performed, followed by derivatization with fluorenyl methoxycarbonyl (FMOC). QTOF MS is a useful tool for identifying matrix interferences and/or assisting in the proper selection of ions for analytical use. Matrix interference discrimination is made possible by its high resolution and mass accuracy. Although it has a lower sensitivity than LC-MS/MS, LC-QTOF MS seems like a promising tool for analyzing GLY in soils and merits further investigation soon.47
6.3. Spectrophotometric analysis
Other screening methods for GLY include colorimetric detection, which uses the Ponceau 4R-copper (P4R-Cu) complex as the chromophore probe.53 Due to P4R's unchelated reaction with Cu, GLY functions as a chelating agent for Cu and can result in a very effective, dose-dependent color recovery at 505 nm. This method's obtained LOD of 0.14 μM demonstrates its high sensitivity, affordability, and simplicity.53
Another spectrophotometric analysis, including SERS and the ninhydrin reaction-based indirect GLY detection, was developed.49 An indirect method for analyzing GLY through its amino group with ninhydrin to form a purple derivative. The SERS-active coupled with Ag nanoparticles (NPs) was developed to overcome this difficulty, as chromatography-based methods require cleanup processes and multiple derivative operation steps that make analysis difficult. The formed product was then detected using SERS bands.49
The LOD of the developed method was 1.43 × 10−8 mol L−1, which shows a strong linear relationship between GLY concentration and the product formed by the ninhydrin test using the SERS band.49
6.4. Biosensors
The analytical techniques have few disadvantages because they require a complete lab setup for herbicide, which cannot be easily accessed by the common man. Therefore, to overcome such disadvantages and continuity in the detection of GLY in different samples, various biosensors have been developed, which not only detect a sample in few minutes but are also highly sensitive and cost effective. From immunoassays to aptamers, biosensors for GLY detection have come a long way, but the common thread that ties them together is an ever-evolving sensitivity that pushes the boundaries of detection limits (Table 3). There has been rapid evolution in the development of biosensors that can detect GLY at increasingly lower concentrations. In this section, we trace the evolution of biosensors owing to their detection, beginning with the primary biosensor and highlighting the key advancements that have been made over the years.
Table 3 Detection techniques for GLY: sensitivity and limit of detection (LOD)
Sample sources |
Techniques |
Sensitivity |
LOD |
Soil and water |
HPLC (pre-column derivatisation) |
High |
20 μg kg−1 and 0.2 μg L−1 (ref. 1) |
GC (nitrogen-phosphorous detector) |
Soil and water |
GC/ion-trap MS |
Moderate |
20 μg kg−1 and 0.5 μg L−1 (ref. 1) |
Soil and groundwater |
Ion-pairing reversed phase liquid (RP-LC) coupled to inductively coupled plasma mass spectrometry with octapole (ICP/MS) |
Moderate |
6 μg kg−1 and 0.1 μg L−1 (ref. 1) |
Water |
Solid phase extraction-liquid chromatography-electrospray ionization-mass spectrometry (SPE-LC-ESI-MS) |
Low |
25–32 0.2 μg L−1 (ref. 1) |
Soil and water |
Electrochemical biosensor (graphite epoxy electrodes (GE) modified with multiwalled carbon nanotubes (MWCNTs) and horseradish peroxidase (HRP)) |
Moderate |
50 μg kg−1 and 0.0005 μg L−1 (ref. 1) |
Surface-enhanced |
Maize |
Raman scattering spectroscopy (SERS) |
High |
0.00132 μg L−1 (ref. 48) |
Natural water |
Raman scattering spectroscopy (SERS) using the ninhydrin reaction |
High |
573 115.4 μg L−1 (ref. 49) |
Drinking water |
Immunosensor (white light reflectance spectroscopy) |
High |
1 × 10−5 μg L−1 (ref. 50) |
Vegetable juice |
Enzyme-assisted electro chemiluminescent biosensor |
Moderate |
132 000 μg L−1 (ref. 51) |
Lake water |
Electrochemical luminescence sensor based on double suppression |
High |
6000 μg L−1 (ref. 52) |
For an accurate analysis of GLY, a sensitive electrochemical biosensor GE/MWCNT-HRP has been reported. HRP was immobilized on a modified electrode surface (GE/MWCNTs) for measuring GLY concentration. The structural confirmation of GLY shows both positive and negative charges.48 This structural characteristic encourages interactions between the amino acid residues on HRP and their opposing charges, which enhances the selectivity and sensitivity of the detection system while increasing the HRP/GLY molecular recognition. Studies of circular dichroism (CD) reveal that after GLY incubation, the α-helix content of HRP significantly decreased and continued to be amplified when hydrogen peroxide (H2O2) was added. Consequently, a sample clean-up procedure was unnecessary because the biosensor allowed for the sensitive detection of the herbicide in corn samples. It operates based on the amperometric response inhibition of the H2O2 reduction at GE/MWCNT-HRP electrodes in the presence of GLY. The LOD for the developed biosensor was 1.32 pM and the LOQ was 1.63 pM.48 A study presents a novel biosensor for GLY detection using electro chemiluminescent (ECL) technology. The biosensor is based on the in situ synthesis of ZnS quantum dots (QDs) on an ordered mesoporous carbon substrate using an enzyme. HRP was added to the reaction mixture to accelerate the production of ZnS QDs by reducing sodium thiosulfate with H2O2, resulting in hydrogen sulfide, which reacts with Zn2+ ions. Sensitive detection was achieved through the GLY inhibition of HRP activity. The suggested ECL QD biosensor showed outstanding sensitivity, repeatability, and selectivity.51 The developed biosensor showed a linear range and sensitivity of 0.1 nM to 10 mM for GLY. However, the sensitivity of enzymatic biosensors for certain analytes, such as GLY, can still be a challenge. To address this issue, researchers have developed a novel biosensor that includes the ultrasensitive detection of GLY in environmental and food samples.
Molecularly imprinted polymers (MIPs) with electrochemical impedance spectroscopy (EIS) are used to construct a highly sensitive sensor for the detection of GLY. The sensor can detect GLY concentrations ranging from 0.31 pg mL−1 to 50 ng mL−1, with an impressively low detection limit of 0.001 pg mL−1. This sensor has the potential to be a valuable tool for the sensitive and specific detection of GLY in various samples.54
Another study aimed to develop a new biosensor for GLY detection based on acid phosphatase inhibition. The biosensor was constructed using silver nanoparticles and electrochemically reduced graphene oxide at the active electrode surface of a screen-printed carbon electrode.55 Acid phosphatase (ACP) was immobilized through glutaraldehyde cross-linking for indirect measurement of GLY. The biosensor showed two linear ranges for GLY, with a detection limit of 0.015 μg mL−1 and a quantification limit of 0.045 μg mL−1, demonstrating high sensitivity and accuracy.55
Although enzymatic biosensors have shown promise for detecting GLY, they can suffer from sensitivity issues. However, recent studies have demonstrated the potential of using nucleic acids, specifically ssDNA coated on carbon nanotubes, as a means of detecting GLY with high precision and sensitivity. This approach, which utilizes differential pulse voltammetry, is cost-effective, efficient, and easy to handle and monitor.48 Moreover, this approach has been demonstrated to be effective in detecting trace amounts of GLY.
Compared to enzymatic biosensors, this novel approach may offer improved sensitivity and accuracy for GLY detection. Additionally, optical biosensors integrated with transducer systems have shown promise for GLY sensing.48
Therefore, aptameric detection of toxic compounds is gaining popularity due to its high sensitivity and easy detection. A recent study by Zhao et al. 2022 combined a highly selective aptamer reaction with an iron metal organic framework (FeMOF) noncatalytic 3,3′,5,5′-tetramethylbenzidine (TMB)/H2O2 trimode indicator reaction to detect GLY in wastewater. They employed the SERS method to indicate GLY, which showed positive results. This indicates that aptameric detection coupled with the SERS method has the potential to be a powerful tool for GLY detection. These methods offer advantages such as high specificity, sensitivity, low cost, and accessibility.56
7. Conclusion and future perspectives
GLY is a commonly used herbicide that has harmful effects on the environment and health when used excessively. Numerous studies have highlighted various negative effects, including disrupting ecosystems, reducing biodiversity, and potentially damaging non-target organisms. These findings emphasize the need for effective detection technologies to monitor the levels of GLY and reduce its environmental impact. The development of detection techniques has played a crucial role in assessing and understanding the extent of GLY pollution. Analytical methods such as chromatography, immunoassay and biosensors have been used to detect and quantify GLY in different environmental samples. These techniques have several advantages, such as high sensitivity, selectivity, and rapid analysis, which enable researchers and environmental authorities to precisely monitor the presence and amounts of GLY. Additionally, the development of biosensors has shown enormous potential in the detection of GLY. These biosensing technologies offer real-time, on-site monitoring capabilities with improved sensitivity and specificity, frequently including bioreceptors or nanomaterials. A useful tool for early warning systems, biosensors, enables quick response to reduce the negative environmental consequences of GLY. However, to solve the difficulties related to GLY detection, continued research and development are required. Future research should focus on enhancing detection limits, extending the range of GLY metabolites that may be detected, and enhancing the portability and cost of detection methods.
To conclude, an understanding of the environmental impact of GLY and advanced and reliably sensitive detection techniques is crucial for effective environmental monitoring and sustainable agricultural practices. By incorporating robust detection methods into the regulatory framework, we can reduce the impact of GLY on the environment and safeguard ecosystems for current and future generations. Continued research, collaboration and innovation in the detection of GLY will pave the way for a healthier and more environmentally conscious future.
Author contributions
S. Kumar: conceptualization, design, editing and supervision the present work. M. Ahuja: literature search, information collection, data compilation, writing, illustration and editing. L. Kumar and K. Kumar: conceptualize and edited the draft. V. M. Shingatgeri: conceptualization discussion was provided. All authors read and approved the final manuscript.
Conflicts of interest
There are no conflicts to declare.
Acknowledgements
We are grateful to School of Biosciences, Apeejay Stya University and Motilal Nehru College, University of Delhi for their infrastructural and academic support systems. This review is not funded by any grants.
References
- A. L. Valle, F. C. C. Mello, R. P. Alves-Balvedi, L. P. Rodrigues and L. R. Goulart, Glyphosate detection: methods, needs and challenges, Environ. Chem. Lett., 2019, 17(1), 291–317, DOI:10.1007/s10311-018-0789-5.
- C. M. Benbrook, Trends in glyphosate herbicide use in the United States and globally, Environ. Sci. Eur., 2016, 28(1), 1–15, DOI:10.1186/s12302-016-0070-0.
- A. Székács and B. Darvas, Re-registration Challenges of Glyphosate in the European Union, Front. Environ. Sci., 2018, 6, 78, DOI:10.3389/FENVS.2018.00078/BIBTEX.
- S. Pochron, L. Simon, A. Mirza, A. Littleton, F. Sahebzada and M. Yudell, Glyphosate but not Roundup® harms earthworms (Eisenia fetida), Chemosphere, 2020, 241, 125017, DOI:10.1016/j.chemosphere.2019.125017.
- A. H. C. van Bruggen, M. R. Finckh and M. He,
et al., Indirect Effects of the Herbicide Glyphosate on Plant, Animal and Human Health Through its Effects on Microbial Communities, Front. Environ. Sci., 2021, 9(October), 1–22, DOI:10.3389/fenvs.2021.763917.
- R. Kanissery, B. Gairhe, D. Kadyampakeni, O. Batuman and F. Alferez, Glyphosate: Its Environmental Persistence and Impact on Crop Health and Nutrition, Plants, 2019, 8(11), 499, DOI:10.3390/plants8110499.
- F. Maggi, D. la Cecilia, F. H. M. Tang and A. McBratney, The global environmental hazard of glyphosate use, Sci. Total Environ., 2020, 717, 137167, DOI:10.1016/j.scitotenv.2020.137167.
- D. Soares, L. Silva, S. Duarte, A. Pena and A. Pereira, Glyphosate use, toxicity and occurrence in food, Foods, 2021, 10(11), 1–22, DOI:10.3390/foods10112785.
- S. Saha, A. Fibres, S. Vijayakumar, S. Elangovan and K. Ramesh, Management strategies for quarantine and invasive weeds, Indian Farming, 2024, 74(2), 27–31 Search PubMed.
- H. A. Pereira, P. R. T. Hernandes and M. S. Netto,
et al., Adsorbents for glyphosate removal in contaminated waters: a review, Environ. Chem. Lett., 2021, 19(2), 1525–1543, DOI:10.1007/S10311-020-01108-4.
- L. E. Saunders and R. Pezeshki, Glyphosate in runoffwaters and in the root-zone: A review, Toxics, 2015, 3(4), 462–480, DOI:10.3390/toxics3040462.
- K. Pallett, Special Issue of Outlooks Focussing on Glyphosate, Outlooks Pest Manage., 2022, 29, 245–246, DOI:10.1564/v29_dec_02.
- C. P. M. Bento, X. Yang and G. Gort,
et al., Persistence of glyphosate and aminomethylphosphonic acid in loess soil under different combinations of temperature, soil moisture and light/darkness, Sci. Total Environ., 2016, 572, 301–311, DOI:10.1016/j.scitotenv.2016.07.215.
- R. C. Wester, J. Melendres, R. Sarason, J. Mcmaster and H. I. Maibach, Glyphosate Skin Binding, Absorption, Residual Tissue Distribution, and Skin Decontamination, Toxicol. Sci., 1991, 16(4), 725–732, DOI:10.1093/toxsci/16.4.725.
- S. O. Duke, Glyphosate : environmental fate and impact, Weed Sci., 2020, 68(May), 201–207, DOI:10.1017/wsc.2019.28.
- E. L. C. Souza, L. L. Foloni, J. T. Filho and E. D. Velini, Half-Life of Glyphosate on the Control of Water Hyacinths in Water Tanks, J. Water Resour. Prot., 2017, 9, 470–481, DOI:10.4236/jwarp.2017.95030.
- H. Zhan, Y. Feng, X. Fan and S. Chen, Recent advances in glyphosate biodegradation, Appl. Microbiol. Biotechnol., 2018, 102(12), 5033–5043, DOI:10.1007/s00253-018-9035-0.
- C. B. Edge, M. I. Brown, S. Heartz, D. Thompson, L. Ritter and M. Ramadoss, The persistence of glyphosate in vegetation one year after application, Forests, 2021, 12, 601 CrossRef.
- B. Hove-Jensen, D. L. Zechel and B. Jochimsen, Utilization of Glyphosate as Phosphate Source: Biochemistry and Genetics of Bacterial Carbon-Phosphorus Lyase, Microbiol. Mol. Biol. Rev., 2014, 78(1), 176, DOI:10.1128/MMBR.00040-13.
- S. Singh, V. Kumar and J. P. K. Gill,
et al., Herbicide glyphosate: Toxicity and microbial degradation, Int. J. Environ. Res. Public Health, 2020, 17(20), 1–18, DOI:10.3390/ijerph17207519.
- I. Travlos, N. Cheimona and D. Bilalis, Glyphosate efficacy of different salt formulations and adjuvant additives on various weeds, Agronomy, 2017, 7(3), 60, DOI:10.3390/agronomy7030060.
- S. O. Duke, The history and current status of glyphosate, Pest Manage. Sci., 2018, 74(5), 1027–1034, DOI:10.1002/PS.4652.
- S. O. Duke, Glyphosate : Uses Other Than in Glyphosate-Resistant Crops , Mode of Action , Degradation in Plants, and Effects on Non-target Plants and Agricultural Microbes, Rev. Environ. Contam. Toxicol., 2021, 255, 1–65, DOI:10.1007/398_2020_53.
- M. Mertens, S. Höss, G. Neumann, J. Afzal and W. Reichenbecher, Glyphosate, a chelating agent-relevant for ecological risk assessment?, Environ. Sci. Pollut. Res. Int., 2018, 25(6), 5298–5317, DOI:10.1007/S11356-017-1080-1.
- A. Zabalza, L. Orcaray, M. Fernández-Escalada, A. Zulet-González and M. Royuela, The pattern of shikimate pathway and phenylpropanoids after inhibition by glyphosate or quinate feeding in pea roots, Pestic. Biochem. Physiol., 2017, 141, 96–102, DOI:10.1016/j.pestbp.2016.12.005.
- N. Tresnakova, A. Stara and J. Velisek, Effects of glyphosate and its metabolite AMPA on aquatic organisms, Appl. Sci., 2021, 11(19), 9004, DOI:10.3390/app11199004.
- M. Helander, I. Saloniemi, M. Omacini, M. Druille, J. P. Salminen and K. Saikkonen, Glyphosate decreases mycorrhizal colonization and affects plant-soil feedback, Sci. Total Environ., 2018, 642, 285–291, DOI:10.1016/J.SCITOTENV.2018.05.377.
- K. Gandhi, S. Khan and M. Patrikar,
et al., Exposure risk and environmental impacts of glyphosate: Highlights on the toxicity of herbicide co-formulants, Environ. Challenges, 2021, 4, 100149, DOI:10.1016/j.envc.2021.100149.
- T. Dolan, P. Howsam, D. J. Parsons and M. J. Whelan, Is the EU drinking water directive standard for pesticides in drinking water consistent with the precautionary principle?, Environ. Sci. Technol., 2013, 47(10), 4999–5006, DOI:10.1021/es304955g.
-
M. I. Masood, Neural stem cells as a model for screening environmental toxicants and a pharmacologically active molecule, PhD thesis, Division of Bioorganic Chemistry, School of Pharmacy, Saarland University, Saarbrücken, Germany, 2021.
- I. P. F. S. Brito, L. Tropaldi, C. A. Carbonari and E. D. Velini, Hormetic effects of glyphosate on plants, Pest Manage. Sci., 2018, 74(5), 1064–1070, DOI:10.1002/ps.4523.
- C. Piasecki, I. R. Carvalho and J. Cechin,
et al., Oxidative stress and differential antioxidant enzyme activity in glyphosate-resistant and-sensitive hairy fleabane in response to glyphosate treatment, Bragantia, 2019, 78(3), 379–396, DOI:10.1590/1678-4499.20180289.
- C. Peillex and M. Pelletier, The impact and toxicity of glyphosate and glyphosate-based herbicides on health and immunity, J. Immunotoxicol., 2020, 17(1), 163–174, DOI:10.1080/1547691X.2020.1804492.
- M. Kwiatkowska, E. Reszka, K. Woźniak, E. Jabłońska, J. Michałowicz and B. Bukowska, DNA damage and methylation induced by glyphosate in human peripheral blood mononuclear cells (in vitro study), Food Chem. Toxicol., 2017, 105, 93–98, DOI:10.1016/j.fct.2017.03.051.
- G. Anifandis, K. Katsanaki and G. Lagodonti,
et al., The Effect of Glyphosate on Human Sperm Motility and Sperm DNA Fragmentation, Int. J. Environ. Res. Public Health, 2018, 15, 1117, DOI:10.3390/ijerph15061117.
- J. A. Barnett and D. L. Gibson, Separating the Empirical Wheat From the Pseudoscientific Chaff: A Critical Review of the Literature Surrounding Glyphosate, Dysbiosis and Wheat-Sensitivity, Front. Microbiol., 2020, 11(September), 1–8, DOI:10.3389/fmicb.2020.556729.
- C. Costas-Ferreira, R. Durán and L. R. F. Faro, Toxic Effects of Glyphosate on the Nervous System: A Systematic Review, Int. J. Mol. Sci., 2022, 23(9), 4605, DOI:10.3390/ijms23094605.
- R. Coullery, A. M. Pacchioni and S. B. Rosso, Exposure to glyphosate during pregnancy induces neurobehavioral alterations and downregulation of Wnt5a-CaMKII pathway, Reprod. Toxicol., 2020, 96(August), 390–398, DOI:10.1016/j.reprotox.2020.08.006.
- N. Tresnakova, A. Stara and J. Velisek, Effects of glyphosate and its metabolite AMPA on aquatic organisms, Appl. Sci., 2021, 11(19), 9004, DOI:10.3390/app11199004.
- J. Ma and X. Li, Alteration in the cytokine levels and histopathological damage in common carp induced by glyphosate, Chemosphere, 2015, 128(May), 293–298, DOI:10.1016/j.chemosphere.2015.02.017.
- T. Rivas-Garcia, A. Espinosa-Calderón, B. Hernández-Vázquez and R. Schwentesius-Rindermann, Overview of Environmental and Health Effects Related to Glyphosate Usage, Sustainability, 2022, 14(11), 1–14, DOI:10.3390/su14116868.
- L. Rueda-Ruzafa, F. Cruz, P. Roman and D. Cardona, Gut microbiota and neurological effects of glyphosate, Neurotoxicology, 2019, 75(August), 1–8, DOI:10.1016/j.neuro.2019.08.006.
- M. Sihtmäe, I. Blinova, K. Künnis-Beres, L. Kanarbik, M. Heinlaan and A. Kahru, Ecotoxicological effects of different glyphosate formulations, Appl. Soil Ecol., 2013, 72, 215–224, DOI:10.1016/j.apsoil.2013.07.005.
- F. M. Conte, L. V. Cestonaro and Y. V. Piton,
et al., Toxicity of pesticides widely applied on soybean cultivation: Synergistic effects of fipronil, glyphosate and imidacloprid in HepG2 cells, Toxicol. In Vitro, 2022, 84(January 2022), 105446, DOI:10.1016/j.tiv.2022.105446.
- E. M. Pimenta, F. F. da Silva and É. S. Barbosa,
et al., Quantification of glyphosate and AMPA by HPLC-ICP-MS/MS and HPLC-DAD: A comparative study, J. Braz. Chem. Soc., 2020, 31(2), 298–304, DOI:10.21577/0103-5053.20190175.
- E. Junqué, P. Fernández, I. Filippi and J. O. Grimalt, Determination of glyphosate and its derivative, aminomethylphosphonic acid, in human urine by gas chromatography coupled to tandem mass spectrometry and isotope pattern deconvolution, J. Chromatogr. Open, 2023, 4(May), 100087, DOI:10.1016/j.jcoa.2023.100087.
- M. Ibá, J. V. Sancho and F. Hernández, Improvements in the analytical methodology for the residue determination of the herbicide glyphosate in soils by liquid chromatography coupled to mass spectrometry, J. Chromatogr. A, 2013, 1292, 132–141, DOI:10.1016/j.chroma.2012.12.007.
- S. L. Cahuantzi-Muñoz, M. A. González-Fuentes and L. A. Ortiz-Frade,
et al., Electrochemical Biosensor for Sensitive Quantification of Glyphosate in Maize Kernels, Electroanalysis, 2019, 31(5), 927–935, DOI:10.1002/elan.201800759.
- X. M. lei, Y. Gao and Y. Li,
et al., Indirect glyphosate detection based on ninhydrin reaction and surface-enhanced Raman scattering spectroscopy, Spectrochim. Acta, Part A, 2018, 15733, 1–5, DOI:10.1016/j.saa.2018.01.014.
- E. Stavra, P. S. Petrou and G. Koukouvinos,
et al., Talanta Fast , sensitive and selective determination of herbicide glyphosate in water samples with a White Light Reflectance Spectroscopy immunosensor, Talanta, 2020, 214(February), 120854 CrossRef CAS PubMed.
- Q. Zhang, G. Xu and L. Gong,
et al., An enzyme-assisted electrochemiluminescent biosensor developed on order mesoporous carbons substrate for ultrasensitive glyphosate sensing, Electrochim. Acta, 2015, 186, 624–630, DOI:10.1016/j.electacta.2015.10.081.
- H. Liu, P. Chen and Z. Liu,
et al., Electrochemical luminescence sensor based on double suppression for highly sensitive detection of glyphosate, Sens. Actuators, B, 2019, 127364, DOI:10.1016/j.snb.2019.127364.
- B. C. Hsieh and Y. T. Li, Colorimetric detection of glyphosate by its competitive reaction against Ponceau 4R for copper ion, Sens. Actuators, B, 2024, 401, 135052, DOI:10.1016/j.snb.2023.135052.
- F. Zouaoui, S. Bourouina-Bacha and M. Bourouina,
et al., Electrochemical impedance spectroscopy determination of glyphosate using a molecularly imprinted chitosan, Sens. Actuators, B, 2020, 309(November 2019), 1–7, DOI:10.1016/j.snb.2020.127753.
- P. Butmee, G. Tumcharern and C. Songsiriritthigul,
et al., Enzymatic electrochemical biosensor for glyphosate detection based on acid phosphatase inhibition, Anal. Bioanal. Chem., 2021, 413(23), 5859–5869, DOI:10.1007/s00216-021-03567-2.
- Y. Zhao, Q. Chen, C. Zhang, C. Li, Z. Jiang and A. Liang, Aptamer Trimode Biosensor for Trace Glyphosate Based on FeMOF Catalytic Oxidation of Tetramethylbenzidine, Biosensors, 2022, 12(11), 920, DOI:10.3390/bios12110920.
|
This journal is © The Royal Society of Chemistry 2024 |