Surface functionalization, particle size and pharmaceutical co-contaminant dependent impact of nanoplastics on marine crustacean – Artemia salina†
Received
9th January 2024
, Accepted 9th April 2024
First published on 10th April 2024
Abstract
Despite a significant amount of research on micronanoplastics (MNPs), there is still a gap in our understanding of their function as transporters of other environmental pollutants (known as the Trojan horse effect) and the combined effects of ingestion, bioaccumulation, and toxicity to organisms. This study examined the individual effects of polystyrene nanoplastics (PSNPs) with various surface functionalizations (plain (PS), carboxylated (PS-COOH), and aminated (PS-NH2)), particle sizes (100 nm and 500 nm), and a pharmaceutical co-contaminant (metformin hydrochloride (MH), an anti-diabetic drug) on the marine crustacean – Artemia salina. The study specifically aimed to determine if MH alters the detrimental effects of PSNPs on A. salina. The potential toxicity of these emerging pollutants was assessed by examining mortality, hatching rate, morphological changes, and biochemical changes. Smaller nanoparticles had a more significant impact than larger ones, and PS-NH2 was more harmful than PS and PS-COOH. Exposure to the nanoparticle complex with MH resulted in a decrease in hatching rate, an increase in mortality, developmental abnormalities, an increase in reactive oxygen species, catalase, and lipid peroxidase, and a decrease in total protein and superoxide dismutase, indicating a synergistic effect. There were no significant differences between the complex and the individual nanoparticles. However, accumulating these particles in organisms could contaminate the food chain. These results highlight the potential environmental risks associated with the simultaneous exposure of aquatic species to plastics, particularly smaller PS, aminated PS, and pharmaceutical complex PS.
Environmental significance
When plastics are released into the environment, they disintegrate into nanoplastics, become surface-modified, or become adsorbed with co-pollutants (pharmaceuticals, heavy metals, pesticides, etc.). Aquatic organisms consume these NPs and pass them through the food chain. Several studies have been performed on pharmaceutical adsorption on NPs, however, their toxicity studies in aquatic species are limited. Therefore, the goal of this work is to assess the influence of different sized, charged, and pharmaceutical (metformin hydrochloride) adsorbed polystyrene nanoplastics on the marine model – Artemia salina. Developmental alterations, hatching rates, mortality rates, and biochemical changes were all examined.
|
1 Introduction
The unsustainable plastic production, usage, and improper disposal have resulted in increasing worldwide plastic pollution and subsequent breakdown into micro(nano)plastics (MNPs) in the environment, jeopardizing sustainability. The amount of plastics produced worldwide has increased dramatically, reaching 368 million metric tons (Mt). It is forecast to double within 20 years, resulting in the generation of 53 Mt plastic waste annually by 2030.1 Nanoplastics (NPs) are extremely small pieces of plastic that can range in size from 1 to 1000 nm and originate from the weathering and degradation of larger plastic particles and microplastics in the environment or from the production of purposefully synthesized engineered nanoparticles.2 These particles are an emerging pollutant that has gained prominence in recent years.
Many studies have shown that MNP pollution negatively affects the environment, biota, and people.3–5 MNPs significantly harm soil fauna (earthworms, nematodes,6 and microbes7 and flora (plants8,9)). Through surface runoff, MNPs reach the aquatic environment and affect the growth and reproduction of many organisms, such as Daphnia magna,10 marine lugworms,11 and oyster larvae12 – Japanese medaka.13 Toxic mechanisms by which MNPs affect the organisms include bioaccumulation, oxidative stress, inflammation, metabolic disorders, gut microbiota dysbiosis, genotoxicity, neurotoxicity, and reproductive toxicity.14 Through the food chain, they enter humans and cause various cellular and organ toxicity. Real-time analysis reported the presence of MNPs in blood clots of cardiac patients, revealing that MNP pollution increases vascular calcification and affects normal heart functioning, revealing organ toxicity. Most up-to-date studies employed amine and carboxy-modified polystyrene NPs (PSNPs) and their assorted sizes to assess toxicity.15 Because of surface oxidation, –COOH particles were more frequent in the environment than –NH2.16 However, –NH2 groups were observed to be more toxic to living organisms due to their less negative surface charge. PS-NH2 induced higher mortality in rotifers17 and developmental toxicity in larval Artemia franciscana17 and Daphnia magna.18 Micronanoplastic sizes also play a crucial role, where NPs are more toxic due to their easier cell internalization. In Caenorhabditis elegans, for instance, NPs with a 20 nm size cause greater toxicity than 100 nm size.19 Similar results were observed for juvenile tilapia in another study.20 The current status of polymer types demonstrated that other than PS, researchers are also focusing on polyvinyl chloride,21,22 polyamide,23,24 polyethylene,25,26 and many others in toxicity studies. However, PS is commonly employed in assessment to compare the extent of toxicity with previous research.
Along with these factors concerned with toxicity, nanoplastics act as vectors for transporting other co-pollutants by adsorbing them on their surfaces, including heavy metals,27,28 organic pollutants29,30 and pharmaceutical compounds.31,32 A wealth of data is available on the extent of pharmaceuticals in the environment and their subtle results on aquatic and terrestrial organisms.33–35 However, limited research has examined the pollution caused by pharmaceutical adsorbed MNPs and their toxicity. Pharmaceuticals treat a wide range of medical conditions and are biologically active compounds. When discharged into the environment by wastewater discharges, improper disposal, or release while unmetabolized in the body, they may interact with various environmental elements, including nanoplastics. Due to various parameters, notably the size, surface charge, hydrophobicity, and functional groups of pharmaceuticals and nanoplastics, adsorption events are possible.32,36–38 Aquatic organisms are frequently exposed to complex contaminants that may have synergistic or antagonistic effects, leading to severe transboundary threats to natural ecosystems, human health, and long-term sustainability.39 One drug found in remarkably high amounts in surface water and wastewater treatment plant (WWTP) effluent has been metformin hydrochloride (MH).40 This anti-diabetic medication is administered all around the world for type 2 diabetes as a primary treatment. It works by decreasing intestinal glucose absorption and inhibiting hepatic glucose production.41 After oral administration, MH is only slowly and partially absorbed, with an absolute bioavailability of 50–60%. Due to its low metabolism, 40–50% of an oral dose is eliminated in feces or urine in its unaltered form within 24 hours.42 It is widely released into aquatic systems because of its many applications, which include suppressing cancer, reducing plasma cholesterol levels, aiding in weight loss, and treating polycystic ovarian syndrome (PCOS).43 Its elevated levels can lead to vitamin B12 insufficiency,44 lactic acidosis,45etc. Metformin concentrations in the environment vary substantially depending on the area and the number of diabetic people, with a maximum concentration of 189 μg ml−1 reported in Ontario, Canada.45 While MH was shown to have the expected no-effect concentration of 1 mg L−1, its ongoing exposure and bioaccumulation characteristics terrify scientists worldwide.46 Possibility of causing endocrine disruption in aquatic organisms has also drawn attention to concerns regarding the effect on reproduction.47–49 The drug's adsorption on polystyrene nanoplastics (PSNPs) was confirmed in our previous research.50 However, an in-depth analysis of their adsorption involving PSNP characteristics, such as size and surface functionalization, was undertaken in this study. Their combined ecotoxicity analysis using a marine organism was also performed here to elucidate co-pollutants' influence on toxic behavior, i.e., whether pharmaceuticals modify the harmful effects of nanoplastics.
Artemia salina is a vital link in aquatic food webs since it is a key food source for many aquatic creatures. These crustaceans exhibit a shortened life cycle, year-round populations, ready availability, and a standardized production process from dried cysts that is also simple to handle. Due to its susceptibility to various environmental stresses, including toxins, Artemia salina is frequently employed as a model organism in ecotoxicology investigations of various environmental pollutants.51–54 If Artemia salina consumes NP-pharmaceutical complexes (NP-drug), the pharmaceuticals may be transmitted to higher trophic levels upon predation. This can result in pharmaceutical accumulation in organisms at higher levels of the food chain, potentially influencing their health and ecosystem dynamics. Toxicological effects vary depending on the type of pharmaceutical, the concentration, duration of exposure, the health and physiological state of the organisms, and the physical and chemical properties (size55 and functional groups56) of the MNPs. Potential toxicity of these complexes on organisms includes acute toxicity, developmental and reproductive effects, behavioral changes, and bioaccumulation.
Examining the impact of pharmaceuticals adsorbed on MNPs on organisms like Artemia salina can be done with the help of biochemical assays.57 These tests offer information about the cellular and molecular alterations brought on by exposure, assisting in the clarification of the underlying toxicity mechanisms. Reactive oxygen species (ROS) generation and disruption of the anti-oxidant defense mechanisms can occur due to oxidative stress brought on by exposure to contaminants.58 To evaluate oxidative stress, tests assessing ROS levels, lipid peroxidation indicators, and anti-oxidant enzyme activity assays (such as superoxide dismutase and catalase) can be utilized.59 A thorough understanding of the toxicological effects of medications adsorbed on MNPs in Artemia salina is made possible by these biochemical assays in conjunction with other physiological and behavioral endpoints.
Growing research is focused on the combined toxicity of medicines and MNPs on aquatic life. Sublethal effects, or those that do not immediately result in death but still have an impact on species, can also be driven by these complexes. Over the extended exposure times, even small amounts of pharmaceuticals adsorbed on MNPs can cause these consequences.39 For the reasons mentioned above, it was determined in this study whether NPs, in conjunction with pharmaceuticals, increase the negative effects on organisms. This was done by assessing the effects of the toxicity of NPs (polystyrene) adsorbed with pharmaceutical substances (metformin hydrochloride) on Artemia salina. Toxicology studies were conducted to assess mortality, hatching rate, and morphological and biochemical changes following treatment to gauge potential negative consequences.
2 Materials and methods
2.1 Test contaminants
Monodispersed pristine polystyrene microspheres (PS) (mean diameter: 100 nm and 500 nm), fluorescent PS (200 nm), carboxylated PS (PS-COOH: 100 nm), and aminated PS (PS-NH2: 100 nm) were acquired from Polysciences, Inc. USA. Fluorescent PS was used in the morphological assessment of test organisms experiment to visualize through a fluorescence microscope. All others were employed in adsorption and toxicity studies. 10 g L−1 stock solutions of each particle were prepared by dispersing in Milli-Q water utilizing a sonicator (VCX-750, Sonics & Materials, Inc, USA). 10 g L−1 of metformin hydrochloride (MH), obtained from Hi-Media Laboratories, India, was prepared as stock by dissolving the drug in Milli-Q water.
2.2 Adsorption study of metformin hydrochloride on nanoplastics
2.2.1 Kinetic study.
The adsorption kinetics were carried out with NPs (PS-100 nm, PS-COOH, PS-NH2, and PS-500 nm) and an initial drug concentration of 10 mg L−1 each, separately. Samples were taken at 1, 2, 4, 6, 8, 24, 36, 48, and 60 h till the adsorption achieved equilibrium. The samples were passed through a 0.1 μm Supor membrane Acrodisc (PALLS) syringe filter to remove adsorbed drug and adsorbent nanoplastic components. The residual MH concentration in the filtrate was measured using a UV-visible spectrophotometer (Hitachi U-2910 (Japan)) at the maximum absorbance wavelengths (λmax) of 233 nm. Calculating the adsorbed concentration onto NPs involved subtracting the final (Ct) concentration of MH from the initial (Ci) concentration of MH in the solution.
The quantity of drug adsorbed by NPs, Q (mg of drug per g of NPs) was calculated as follows:
| 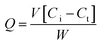 | (1) |
W = mass of the adsorbent [g].
V = total solution volume [ml].
The amount of drug adsorbed at any time t is represented as Qt, and at equilibrium, is represented as Qe. The adsorption mechanism between MH and NPs was determined by adsorption kinetic research. Either physisorption or chemisorption can be used to describe the mechanism. Physisorption is regulated by van der Waals forces and other weak interactions, whereas a chemical connection distinguishes chemisorption between the adsorbent and adsorbate molecules. Physisorption follows the pseudo-first-order kinetics model.
On the other hand, the pseudo-second-order model postulates that the adsorption rate would be determined by chemisorption, a chemical surface contact.60 Kinetic equations were employed utilizing the average values determined for Qe, Qt, and t. The variables and accompanying equations are expressed below.
Lagergren equation: pseudo Ist order61
| ln(Qe − Qt) = ln Qe − Kft | (2) |
Ho and McKay equation: pseudo IInd order62
| 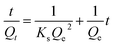 | (3) |
where,
Kf = pseudo-first-order rate constant (h
−1),
Ks (g mg
−1 h
−1) = pseudo-second-order rate constant.
Fitting experimental data into an intraparticle diffusion plot can assist in anticipating the rate-controlling stage of the sorption process,63 which is given by the Weber–Morris equation as follows.
where
Kid (mg g
−1 h
0.5) = rate constant.
C = influence of the boundary layer on adsorption.
Nanoplastics were dispersed in Milli-Q water (pH 7.4) and characterized for zeta potential using an SZ-100 nanoparticle analyzer, HORIBA Scientific, Japan. The zeta potential of individual NPs and their corresponding interacted complex with MH were measured, which reflects the surface charge.
2.2.2 Isotherm analysis.
The influence of the initial concentration of the drug on adsorption was assessed by varying the MH concentration between 1 and 20 mg L−1, following the established protocol outlined in Section 2.2.1. For each initial drug concentration, the amount of drug adsorbed (Qe) by the NPs after reaching equilibrium (determined from kinetic research) was computed, along with their corresponding residual concentrations at equilibrium (Ce). The adsorption isotherm models64 were examined using these data (Table. S1†).
2.2.3 Effect of salinity on adsorption.
As previously described, the adsorption method was carried out independently in Milli-Q water and seawater to examine the impact of salinity on the adsorption of MH on various PSNPs. The outcomes were then compared. For this, the marine water was collected from Marina Beach in Chennai (13°2′59.83′′N, 80°16′56.65′′E). Using 0.2 μm filter paper, larger colloidal particles were removed from the marine water using a filtration process that produced particle-free seawater. Its physicochemical features were preserved by not undergoing any additional filtration steps. The water was autoclaved for 15 minutes at 121 °C to eliminate any biological contaminants. The term “sea water” will henceforth be used to refer to sterilized environmental water that is free of additional pollutants. The seawater's physiochemical properties have been characterized and presented in Table. S2.† Adsorption experiments were performed to find the impact of salinity using this seawater and comparing it with Milli-Q water. This experiment used 10 mg L−1 of MH and 10 mg L−1 of each respective NP (PS-100 nm, PS-COOH, PS-NH2, and PS-500 nm), similar to the procedure described in Section 2.2.1. To find the mechanism behind the adsorption process, zeta potential and hydrodynamic sizes of all the seawater and Milli-Q water particles were analyzed using a nanoparticle analyzer (SZ100, Horiba, Japan) and compared.52,65
2.2.4 Field emission scanning electron microscope analysis.
The optimized ratio (from kinetic and isotherm analysis) of samples was prepared as per the method in Section 2.2.1 and examined under a FESEM (Thermo Fisher FEI-Quanta 250 FEG, USA) to observe the adsorption of MH on NPs (PS-100 nm, PS-500 nm, PS-COOH, and PS-NH2). NPs (10 mg L−1) and MH adsorbed NPs (0.5
:
1 ratio (ratio found from the adsorption experiment); MH – 5 mg L−1: NPs – 10 mg L−1) were shaken under optimal adsorption conditions. After that, all samples were lyophilized (ALPHA 1–2 LD plus freeze dryer, Germany) to eliminate all traces of moisture. Samples were then coated with gold and analyzed under 20 kV acceleration voltage FESEM with a 50
000× magnification. Using a backscattered electron detection system, energy dispersive X-ray spectrometry (EDX) was used to measure the compositions of the elements.
2.3 Toxicity studies
PSNPs of different sizes (PS-100 and PS-500), different functionalizations (PS-COOH and PS-NH2), and PSNPs + MH complexes (each particle mentioned mixed with MH at the optimized ratio and kept for an equilibrium time for maximum adsorption) were employed in the toxicity experiments to find their impact on Artemia salina. The A. salina cysts were obtained from Ocean Star International, Inc., USA. The above-obtained seawater was utilized for toxicity tests to simulate the aquatic habitat supporting Artemia's growth.
2.3.1 Hatchability test.
Varying concentrations of NPs, complexes, and MH (2.5, 5, and 10 μg ml−1 each) were prepared as 2 ml samples by making up using seawater and transferred to wells of 24 well plates. Twenty A. salina cysts were counted and added to each of the wells containing test contaminants and incubated at 28 ± 1 °C. Wells without contaminants were taken as controls. The ideal hatching conditions of 24 h photoperiod (continuous illumination), 29 °C temperature, and 28 ppt salinity were maintained for A. salina cysts.66 After 36 h, hatched cysts were counted using a stereomicroscope (ACCU SCOPE, 3078, Zoom Stereo, USA). The following formula was utilized to analyze the hatching percentage.67 |  | (5) |
2.3.2 Acute toxicity study.
Similar to the hatching test, NPs, complexes, and drugs (2.5, 5, and 10 μg ml−1 each) were prepared as 3 ml samples and taken in 24 well plates. Ten nauplii were added to each sample and incubated for 48 h. Survival of the organism was observed after 48 h, and mortality rate was calculated for each particle and compared with the control.58 The nauplii were not given any aeration or food during the experiment. The significance between the particles was examined using a two-way ANOVA test.
An independent action model further supported the toxicity data. The expected toxicity (CExp) of the complex was calculated by adding the individual toxicities of PSNPs and MH using the following equation.68
| 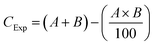 | (6) |
A = mortality (%) exerted by individual PSNPs.
B = mortality (%) exerted by MH.
From the CObs values, the ratio of inhibition (RI) was calculated using the below equation.
| 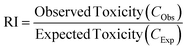 | (7) |
CObs = observed toxicity for the PSNPs + MH complex.
CExp = expected toxicity for the PSNPs + MH complex.
The calculated value of RI is used to determine the type of interaction. An interaction is deemed additive if its RI value is one. Antagonistic interactions are those in which the computed RI value is less than 1. On the other hand, an interaction is considered synergistic if the RI value is higher than 1.
2.3.3 Developmental changes.
A. salina cysts were exposed to all the test samples (each 10 μg ml−1) and kept for incubation under ideal hatching conditions without aeration or feeding. Five organisms from each treatment were chosen randomly at all stages of development and placed on a glass slide. They were then examined using a Leica DM2500 LED microscope with 40× objective lenses (Leica Microsystems, Switzerland). The DCF295 camera attached to the microscope was used to take pictures. To identify substantial developmental defects in the individuals, the morphological changes and particle accumulation in the developmental stages from cyst to 3rd instar nauplii were studied for all test samples and compared with the control groups (cyst incubated with seawater without PSNPs.)
2.4 Impact on biochemical profile
After treatment with pristine NPs, drugs, and complexes (each 10 μg ml−1), the nauplii were washed three times in deionized water to eliminate unbound NPs. Then, 2 ml of phosphate buffer (pH 7.4) was added, homogenized, and centrifuged for 10 minutes at 8000 rpm. Supernatants were collected and examined for changes in biochemical profile. Changes in the environment or harmful substances may alter the total protein content, trigger the production of reactive oxygen species, and alter the activity of certain enzymes, including superoxide dismutase, catalase, and lipid peroxidase. To observe the harmful consequences of pollutants, their levels were quantified. All activities were measured in triplicate and expressed as mean ± SD. The results were analyzed for significance using a one-way ANOVA test.
2.4.1 Total protein.
The Bradford technique was used to estimate the total protein content of the A. salina homogenate.69 190 μl of Bradford reagent was added to 10 μl of supernatant and incubated for 5 minutes in the dark, and absorbance at 595 nm was measured using a Biotek 800/T's microplate reader. Bovine serum albumin (BSA) was used as a reference standard to determine the unknown protein concentrations.
2.4.2 Estimation of reactive oxygen species.
Reactive oxygen species (ROS) produced as superoxide, hydroxyl, and peroxynitrile free radicals in response to external stressors were assessed using DCFHDA (2′,7′-dichlorofluorescin diacetate).70 In a 96-well plate, 20 μl of 5 mM DCFHDA was added to 80 μl of each supernatant sample and incubated for 30 minutes. A fluorescence spectrophotometer (FP-8300, JASCO International Co Ltd, Japan) was used to measure absorbance at an excitation wavelength of 485 nm. Differences in fluorescence intensity between test and control samples were computed and compared as ROS percentages.
2.4.3 Anti-oxidant enzyme activities (SOD and catalase).
Superoxide dismutase (SOD) activity quantification is critical in determining the anti-oxidant capabilities of a biological system and is estimated based on the reduction of nitro blue tetrazolium (NBT).71 In brief, a reaction mixture of 50 mM sodium carbonate (pH 10.2), 24 mM NBT, 1 mM hydroxylamine hydrochloride, and 0.1 mM Triton × 100 was added to 10 μL supernatant of each sample supernatant and incubated for 20 minutes. Following incubation, the absorbance was measured with a microplate reader at 560 nm (Bio-Rad xMark, 30
188). The total amount of SOD was determined using the OD values obtained, based on the concept of reduction of NBT by SOD generated in A. salina.
The CAT assay was carried out using the protocol described in an earlier study.72 The supernatant of 200 μL was treated with 800 μL of 10.8 mM H2O2 solution prepared in a 50 mM – pH 7 potassium phosphate buffer. Using a UV-vis spectrophotometer (Hitachi U-2910 Japan), the absorbance of the treated solution was measured at 240 nm for 3 min. As a reference, potassium phosphate buffer was employed. Based on the drop in absorbance value, catalase activity was calculated.
2.4.4 Lipid peroxidation assay.
Malondialdehyde (MDA) is a reliable marker of lipid peroxidation, formed during the synthesis of lipid hydroperoxides. To measure lipid peroxidation, 100 μL of each sample was mixed with 400 μL of 0.25% 2-thiobarbituric acid (TBA) and incubated at 95 °C for 60 min. After cooling, the mixtures were centrifuged at 700 rpm for 15 minutes, the supernatants were collected and their absorbance at 532–600 nm was measured. This measures TBARS (thiobarbituric acid reactive substances), indicative of lipid peroxidation. The method relies on MDA reacting with TBA to produce a pink-coloured compound (TBARS), measured at 532 nm.
2.5 Data analysis
All the experiments were repeated three times. The findings of biochemical experiments were statistically compared using one-way ANOVA. A P ≤ 0.0001 value was deemed statistically significant. A two-way ANOVA was used to compare the outcomes of hatching and mortality tests, and the P ≤ 0.001 value was considered statistically significant. All data are reported as the mean with standard deviation (SD). The slope and intercept values from the linear regression equation were used to compute the total protein content and MDA concentration in the samples for LPO analysis. The Graph Pad Prism program (version 9.4.1, San Diego, USA) was used for all statistical studies.
3 Results and discussion
3.1 Adsorption study
3.1.1 Adsorption kinetics.
According to eqn (1), the adsorbed amount of MH by NPs (Qt) was computed by tracking the concentration of MH in the filtrate at regular intervals. After three stages—the initial rapid sorption (8 hours), followed by slower sorption (16 hours later), and finally reaching equilibrium, the adsorption equilibrium was consistently achieved within 48 hours for all NPs (Fig. 1A). Because there were more adsorption sites and a bigger gradient in the adsorbate concentration in the solution, there was a greater mass transfer force initially, which accelerated adsorption. Diffusion occurs in the inner pores of NPs, and equilibrium73 is reached in 48 h. For the subsequent investigations, the adsorption period was set for 48 hours.
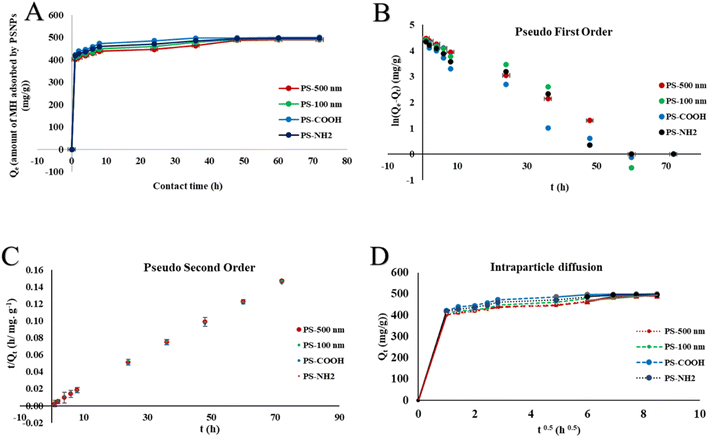 |
| Fig. 1 (A) Adsorption of MH by various NPs over time (Qevs. time), (B)–(D) kinetic models assessed from the results of the contact time experiment for adsorption of MH on PSNPs. The data points were the experiment values in triplicate, shown as mean ± SD. Microsoft Excel produced the graphs. Kinetic parameters were calculated using the slope and intercept values of the regression equation; the results are displayed in Table 1. | |
Variation in the NPs was observed in terms of the amount of MH adsorbed (Qe), with the maximum being as follows: PS-COOH > PS-NH2 > PS-100 > PS-500. Almost 50% of MH was adsorbed by PS-COOH NPs. The underlying mechanism might be a greater difference in the surface charge of PS-COOH and MH, as detected in the zeta analyzer (Fig. S1†). The greater difference in zeta potential might initiate the electrostatic attraction between NPs and drug,74 at the neutral pH, causing greater adsorption. Further, the enhanced adsorption in functionalized NPs compared to pristine NPs might be due to the complexation of carboxyl and amine functional groups in NPs75 with pollutant MH due to increased chemical reactivity. Among the different sized NPs, PS-100 and 500 nm, smaller NPs adsorbed more drug (49.07%) due to a greater surface area/volume ratio than bigger NPs76,77 (48.72%). The data obtained from the contact time experiment were applied in the kinetic models (Fig. 1B–1D), and the evaluated parameters are given in Table 1. The plots' computed equilibrium adsorption capacities (Qe) demonstrate a good agreement between the pseudo-second-order model (Table 1) values and the experimental (Qe) values (Fig. 1A). Second-order kinetics fitting indicates chemisorption's involvement in surface complexation processes.78 A thorough analysis of the data for the first-order model shows that the R2 is higher than 0.92 for every compound, indicating the possibility of physisorption as well. As a result, the kinetics describing the chemical and physical adsorption of MH on polystyrene nanoplastics are mirrored using both first- and second-order models.
Table 1 Kinetic parameters calculated for MH adsorption on various NPs
Model |
Values |
Pseudo Ist order |
K
f (h−1) |
Q
e (mg g−1) |
R
2
|
PS-500 nm |
0.067 ± 0.0002 |
92.30 ± 0.42 |
0.98 |
PS-100 nm |
0.071 ± 0.0010 |
93.27 ± 0.09 |
0.92 |
PS-COOH |
0.066 ± 0.0009 |
60.70 ± 0.15 |
0.95 |
PS-NH2 |
0.066 ± 0.0005 |
77.12 ± 0.12 |
0.95 |
Model |
Values |
Pseudo IInd order |
K
s (g mg−1 h−1) |
Q
e (mg g−1) |
R
2
|
PS-500 nm |
0.002 ± 9.80 × 10−5 |
500 ± 0.98 |
0.99 |
PS-100 nm |
0.002 ± 1.00 × 10−4 |
500 ± 0.85 |
0.99 |
PS-COOH |
0.004 ± 9.80 × 10−5 |
500 ± 0.67 |
1 |
PS-NH2 |
0.003 ± 5.00 × 10−5 |
500 ± 0.48 |
0.99 |
Model |
Values |
Intraparticle diffusion |
K
id (mg g−1 h−0.5) |
Q
e (mg g−1) |
R
2
|
PS-500 nm |
11.27 ± 0.07 |
396.99 ± 1.23 |
0.93 |
PS-100 nm |
12.93 ± 0.08 |
401.17 ± 1.05 |
0.95 |
PS-COOH |
15.62 ± 0.05 |
415.88 ± 1.58 |
0.87 |
PS-NH2 |
12.61 ± 0.04 |
412.39 ± 1.20 |
0.93 |
The intraparticle diffusion model must be assessed to determine the rate-limiting stage. The sole rate-limiting activity that occurs when the regression line crosses the origin is intraparticle diffusion.63 In this case, the plot (Fig. 1D) is multi-linear instead of passing through the origin, suggesting that multiple crucial steps were involved in the adsorption process. The Weber–Morris plot is split into three linear segments spanning the whole adsorption phase for all the complexes. The first segment is 0–1, the second is 1–6, and the third is 6–9 (the number indicates the x-axis values). These segments correspond to the three phases of surface diffusion, intraparticle diffusion, and ultimate equilibrium. The R2 value of the intraparticle diffusion plot is also considerable for all the cases of NPs.
In conclusion, liquid film diffusion led to the first rapid diffusion of MH from the bulk solution to the NP exterior surface. The second intra-particle diffusion stage proceeds more slowly due to the inside micropores' restricted diameters. Merely 17–19% of the MH has been absorbed into the NPs thus far. The plateauing plots indicate that the adsorption equilibrium was eventually reached. The second segment's intercept is correlated with the thickness of the boundary layer; a larger intercept (C in the 397–416 range) indicates a higher surface diffusion contribution during the rate-limiting phase.79 Hence, the sorption process of MH onto PSNPs in the environment encompassed surface site sorption, mass transfer, intraparticle diffusion, and physical and chemical interaction. These results aligned with those of earlier studies for different pollutant-polymer systems.78,80
3.1.2 Adsorption isotherms.
Analyzing the effect of the initial concentration of co-pollutants on the maximum adsorption capacity of polystyrene nanoplastics, it was found that all the NPs could adsorb MH to the maximum when the initial concentration is 10 mg L−1. Above this concentration, all the NPs reached a saturation point, as evident from the saturation line (Fig. 2A). Similar to the contact time experiment, the adsorption percentage was greater in the order of PS-COOH > PS-NH2 > PS-100 > PS-500. Delving deep into the adsorption capacity, among the 10 mg L−1 concentration of MH, NPs could adsorb a maximum of 50% of MH only. Hence, MH: NPs were prepared at a ratio of 0.5
:
1 for further experiments.
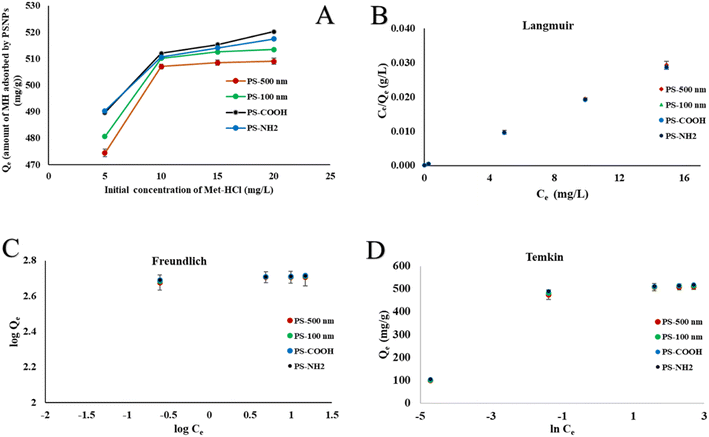 |
| Fig. 2 (A) Effect of initial concentration of MH on the adsorption process. (B)–(D) Isotherm models for the adsorption of MH on NPs. The experiment values in triplicate, shown as mean ± SD, were the data points. Isotherm parameters were calculated using the slope and intercept values of the regression equation; the outcomes are displayed in Table 2. | |
The isotherm models (Fig. 2B–2D) were used to assess the experimental results. The parameters obtained from these models are presented in Table 2. The Langmuir isotherm, a good representation of real-world systems, is predicated on the idea that all adsorbable particles have the same amount of energy and binding sites on the adsorptive surface, forming a monolayer on the surface of the adsorbent.81 A higher fitting of the Langmuir isotherm for MH adsorption on PSNPs is indicated by an R2 value of 1. At 25 °C, the maximal monolayer adsorption capacity (Qm) of PSNPs was determined to be between 500 and 526 mg g−1. The presence of heterogeneous systems can be accounted for by the Freundlich isotherm when drug molecules exhibit non-equivalent binding sites in terms of energy.82 The R2 value in the range of 0.76 to 0.77, lower than the values obtained from the other two plots, does not adequately explain this adsorption phenomenon. The n value 4.90 between the adsorbent and the adsorbate indicates strong affinity and chemisorption. The isotherm given by Temkin and Pyzhev assumes that all drug molecules have an even balance of binding sites and energy and that the adsorption heat decreases linearly with the covering of the adsorbent surface83 However, this model cannot describe the experimental data, as seen by its R2 value (0.8–0.80), which is less than the Langmuir model's. The predominant adsorption mechanism of MH on the PSNPs studied in this study is monolayer coverage, and the Langmuir model was shown to be the most accurate. For the adsorption of various contaminants by MNPs, a comparable adsorption isotherm fit was found.84
Table 2 Isotherm values calculated for MH adsorption on various NPs
Model |
Parameters |
Langmuir |
K
L (L mg−1) |
Q
m (mg g−1) |
R
2
|
PS-500 nm |
33.33 ± 0.25 |
500 ± 1.25 |
1 |
PS-100 nm |
31.66 ± 0.14 |
526.31 ± 2.24 |
1 |
PS-COOH |
21.11 ± 0.26 |
526.31 ± 2.85 |
1 |
PS-NH2 |
23.75 ± 0.21 |
526.31 ± 1.55 |
1 |
Model |
Parameters |
Freundlich |
Kf |
N
|
R
2
|
PS-500 nm |
353.99 ± 0.95 |
4.90 ± 0.009 |
0.77 |
PS-100 nm |
357.60 ± 0.80 |
4.93 ± 0.007 |
0.77 |
PS-COOH |
364.25 ± 1.35 |
5.06 ± 0.006 |
0.76 |
PS-NH2 |
363.16 ± 1.24 |
5.06 ± 0.002 |
0.76 |
Model |
Parameters |
Temkin |
b (J mol−1) |
K
T (L mg−1) |
R
2
|
PS-500 nm |
48.03 ± 0.07 |
3084.05 ± 21.24 |
0.80 |
PS-100 nm |
47.82 ± 0.05 |
3211.57 ± 10.25 |
0.80 |
PS-COOH |
48.08 ± 0.08 |
3703.38 ± 12.45 |
0.79 |
PS-NH2 |
48.32 ± 0.01 |
3778.11 ± 17.55 |
0.78 |
3.1.3 Effect of salinity on adsorption of MH on PSNPs.
Salinity can significantly influence the sorption of various pharmaceuticals onto NPs. Freshwater rivers typically have lower salinity levels, ranging from 0.5 to 1 ppt, whereas seawater has a higher salinity, ranging from 32 to 35 ppt.85 Nanoplastics and pharmaceuticals are commonly introduced into aquatic environments via inefficient wastewater treatment plants directly into rivers or ultimately into seas and oceans. Depending on the point of entry, the adsorption capacity of pharmaceuticals by nanoparticles (NPs) can vary, with the influencing factor being salinity.86 The results from the salinity experiment indicate that the sorption of MH on PSNPs demonstrates better performance in seawater than Milli-Q water (Fig. 3). This phenomenon is likely attributable to the increased presence of ionic species in seawater compared to normal water. The surface charge of NPs can be influenced by the presence of ions in the surrounding solution. At higher salinity, more ions are available to interact with the surface of NPs, potentially altering their surface charge.87 Zeta potential experiments have revealed that the surface potential of NPs becomes more negatively charged when dispersed in seawater (Table. S3†). This increase in negative charge results from attaching ionic species to the NP surface, modifying its charge. Many researchers have observed similar findings underscoring the increased negative charge of PSNPs due to abundant ionic species in seawater.88,89 This enhanced negative charge leads to a greater difference in surface potential between NPs and MH in seawater, resulting in a stronger electrostatic force of attraction and, consequently, greater adsorption of MH by NPs in seawater.87 Among the NPs tested, functionalized PSNPs exhibited greater drug adsorption than plain NPs, likely due to possible interactions with functional groups. A greater difference in surface potential was observed between PS-COOH and MH in seawater, resulting in greater adsorption. As explained in previous sections, smaller NPs (PS-100) among the plain NPs adsorbed a greater quantity due to their increased surface area.
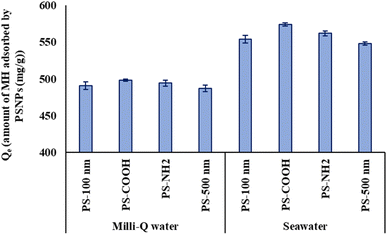 |
| Fig. 3 Effect of salinity on the adsorption process of MH on various PSNPs. | |
Adsorption may correlate with the complex's hydrodynamic sizes in different water settings. Comparing the sizes of all particles (Table S4†) dispersed in Milli-Q water and seawater, those dispersed in seawater displayed greater hydrodynamic sizes due to increased adsorption, impacted by salt ions. Consequently, greater adsorption corresponded with larger sizes observed for PS-COOH + MH particles in seawater. The obtained results indicate the involvement of various complex interactions in pharmaceutical-microplastic sorption. Similarly, increasing salinity increases the adsorption of pollutants by NPs, as observed in the sorption of chlorpromazine,86 tetracycline90 ciprofloxacin, bisphenol A,91 and PCBs92 on PSNPs.
3.1.4 FESEM analysis.
The most effective way to observe characteristics, including texture, porosity, and surface nature, as well as to magnify and examine the surface structure, is by using a FESEM. Gaining insight into the adsorption process requires knowing this information.93Fig. 4A–D show the images of PS-100, PS-COOH, PS-NH2, and PS-500, respectively, before the adsorption of MH. Particles were found to be spherical and uniform with the sizes mentioned in procurement specifications. Fig. 4E–H show the images of their corresponding particles after adsorption with MH. As can be seen from the pictures, the size of each NP increased following the adsorption process, and adsorption of all NPs was found to be monolayer. This indicates that MH has been successfully adsorbed onto MPs. Thus, the FESEM investigation confirms the Langmuir model fit of monolayer adsorption.
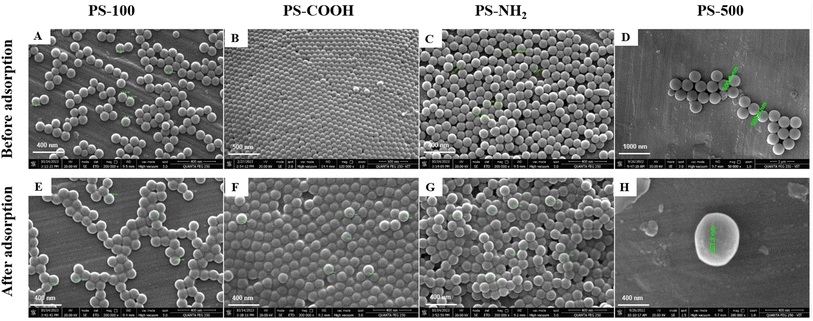 |
| Fig. 4 (A–D): FESEM images of NPs before the adsorption process. (A) PS-100, (B) PS-COOH, (C) PS-NH2, (D) PS-500. (E–H): FESEM images of NPs after adsorption of MH. (E) PS-100 + MH, (F) PS-COOH + MH, (G) PS-NH2 + MH, (H) PS-500 + MH. | |
The quantities of elements in PSNPs before and after MH adsorption were ascertained by EDX analysis. The elements in NPs are depicted both before and after the adsorption process in Fig. S2.† The oxygen and nitrogen contents in B and C represented the carboxyl and amine contents of PS-COOH and PS-NH2. Furthermore, all of the pictures following adsorption with MH showed the presence of nitrogen and chlorine from the MH medication,94 demonstrating its adsorption by NPs. The adsorption of co-pollutants by NPs was thus made clearer by elemental analysis of FESEM images.
3.2 Toxicity study
3.2.1 Effect on hatching rate.
Nanoplastics are predominant in marine ecosystems and have been consumed by numerous marine organisms. Accordingly, in the current investigation, the toxic impact of NPs was evaluated using the brine shrimp, Artemia, an appropriate indicator species for hazardous contamination.95Fig. 5A shows the hatching rate of A. salina cysts following treatment with different NPs and their MH complexes. Compared to the control, all particles showed a significant difference in hatching rate (P ≤ 0.001). Functionalized NPs have a higher negative impact on the hatching rate than pristine NPs, possibly due to the greater surface complexation with cell membranes of A. salina. Less negatively charged amine PS (10 μg ml−1) interacts more effectively with cell membranes, causing a greater decrease in hatching rate (56.66 ± 0%) compared to the highly negatively charged PS-COOH (10 μg ml−1). This contact increases their accessibility to specific cells, tissues, or the nucleus, which will cause bioaccumulation and raise potential hazards.96,97 Similar results were observed for the larval stages of franciscana.17 The hatching rate showed a consistent decrease as the concentration of each particle increased. All the particles, except MH alone, dramatically reduced the hatching rate compared to the control (P ≤ 0.001). In the size comparison experiment, PS-100 (10 μg ml−1) decreased the hatching rate (66.66 ± 0.57%) more than 500 nm (10 μg ml−1) did. This might be because smaller NPs have a greater ability to penetrate biological barriers because of their tiny sizes and high surface-to-volume ratios, which might result in accumulation and harmful effects.3 Similarly, in previous studies with Dunaliella salina, smaller NPs caused more serious biological effects than larger ones.98
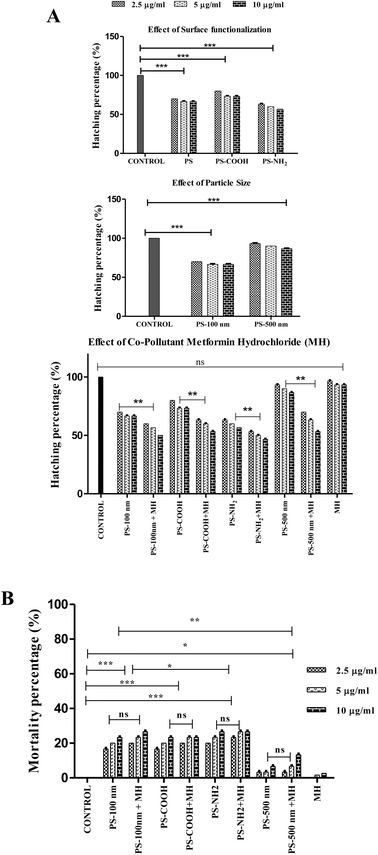 |
| Fig. 5 (A) The percentage of hatching of A. salina cysts upon exposure to different concentrations of NPs and their complexes with MH (n = 3). All values are expressed as mean ± SD. (***P ≤ 0.001). (B) The percentage of mortality of A. salina cysts upon exposure to different concentrations of NPs and their complexes with MH (n = 3). All values are expressed as mean ± SD. (***P ≤ 0.001). | |
Surprisingly, when all pristine particles and their complexes with MH were compared, the change in hatching rate was statistically insignificant (P ≥ 0.05), although a modest decrease in hatching rate by NP-MH complexes revealed their synergistic method of action. Although PS-COOH + MH particles had the least negative zeta potential, they were observed to have a lower toxic impact than the PS-NH2 + MH complex. This might be because, since the zeta potential is closer to −30 mV (Fig. S1†), their electrostatic repulsion may decrease, leading to a less stable aggregated complex. Therefore, these larger particles cause less toxicity compared to the PS-NH2 + MH complex. Previous studies have reported that the extensive release of emerging pollutant MH into wastewater treatment plants causes endocrine disruption in fish.99 Since MH is a pharmaceutical – a biologically active compound, it can interact with biological systems and alter their normal mechanism, synergistically increasing toxicity. The PS-NH2 + MH complex (10 μg ml−1), the most significant of all (46.66 ± 0.57%), is the complex that primarily lowers the hatching rate of A. salina.
Given that the procured PSNP particles were obtained in suspension form, it is imperative to consider the dynamics of these diluted particles in working concentrations. Although the size of PSNPs increased due to MH adsorption and the seawater effect, they were confined to less than 1000 nm size, as shown in Table S4.† At these sizes, PSNPs remained in suspension form throughout the experimental duration. Therefore, these suspended particles potentially disrupt the hatching process of free-floating Artemia cysts100,101 within the suspension medium.
In contrast to this study of nanoplastics, the maximum hatching rate observed by A. salina species exposed to PS microplastics (24 ± 10 μm; 100 μg ml−1) was 75%.102 The hatching rate disparity could result from using plastics of various sizes (micro and nano). In a different investigation, 48 hours were spent exposing various plastic types—PE (7.35 μm), PLA (4.41 μm), and PBS (7.64 μm)—to A. salina cysts at concentrations of 12.5 μg ml−1. The hatching rate was approximately 60% for all MPs.103 Observing all the facts, it is reasonable to infer that hatching rates depend on parameters such as polymer type, concentration of pollutants, size, etc.
3.2.2 Acute toxicity study.
The mortality observed in response to the treatment of various NPs on Artemia salina is depicted in Fig. 5B. This interaction, with increasing concentrations of pristine NPs, drugs, and complexes, resulted in an increase in mortality, which is statistically significant (p ≤ 0.001) from the control, where PS-500 nm showed their least significance (concentration: 2.5–10 μg ml; mortality%: 3.33–6.66%). Regarding functional groups, PS-NH2 treated groups showed excessive mortality (20–23.33%) at all increasing concentrations compared to others. In the same way, PS-NH2 also caused increased mortality in rotifers (Brachionus plicatilis) than PS-COOH NPs.104 PS (16.66–23.33%) and PS-NH2 (20–23.33%) treated groups were considerably less significant (P ≤ 0.05), while PS and PS-COOH (16.66–23.33%) treated groups were seen to be statistically insignificant (P ≥ 0.05). On comparing the sizes, 100 nm PS showed the highest mortality at all the concentrations, followed by 500 nm. Smaller NPs were more toxic to A. salina than bigger NPs since smaller ones can easily enter the organism's tissues, cells, or nucleus by crossing the biological barrier.105 The mortality rate was statistically insignificant between 100 nm and 500 nm (P ≤ 0.01). Interestingly, when comparing all the pristine particles and their complexes with MH, the difference in mortality was statistically insignificant (P ≥ 0.05), but a slight increase in mortality proved their synergistic mode of action. Alone, MH showed the least significant mortality (0–1.66%) compared with the control. Adsorption of environmental pharmaceuticals to NPs increased the mortality rate (PS-100 + MH: 20–26.66%; PS-COOH + MH: 20–23.33%; PS-NH2 + MH: 23.33–26.66%; PS-500 + MH: 3.33–13.33%) when compared with their respective pristine particles. Table S5† shows the independent action model (IA) modeling results to determine the nature of the interaction between MH and PSNPs. The complex concentration was positively correlated with an increase in the ratio of inhibition (RI). Except for 2.5 μg ml−1 of PS-500 + MH, which is additive, all the other combinations demonstrated a synergistic effect. Previous studies have evidenced that antibiotics, a pharmaceutical category, also influence mixture toxicity, where doxycycline increases the toxicity of microplastics to Tetraselmis chuii at concentrations in the low ppm range itself.106 Overall, PS-NH2+MH (23.33–26.66%) is comparatively the most significant complex among all, which causes maximum mortality to A. salina.
3.2.3 Morphological changes.
Artemia salina cysts were made to interact with individual NPs and their complexes with MH for continuous exposure time107 till the development of the third instar nauplii (till 72 h)108 to detect the morphological alterations, developmental abnormalities, and particle accumulation. Representative figures showing significant abnormalities are presented in Fig. 6. Artemia's changes in life stages on exposure to all the particles are elaborately displayed and discussed in Fig. S3.† There were no developmental anomalies or accumulations in the control groups. Similarly, in MH-treated groups, no harmful effects were identified. When the different surface functionalized treated groups were compared, PS-NH2 had a higher intake of particles with developmental abnormalities in the instar 1 and 2 stages than PS and PS-COOH. In terms of size, PS-100 nm was shown to have a higher intake than PS-500 nm, with just a modest variation in the body structure. In terms of MH adsorbed NPs, PS-MH delayed hatching at 16 h, followed by intake, developmental deformity at the first instar stage, and accumulation in the gut region, whereas PS-NH2-MH exhibited a highly significant difference among all others, with the cyst being completely spherical and showing delayed hatching, followed by delayed developmental growth as seen in the second and third instar stages. When it comes to the mechanism of toxicity of these particles, their accumulation in Artemia's alimentary tract has been recognized as one of the key reasons for mortality and toxicity in the earlier study report. The formation of NP aggregates in the gut inhibits excretion and increases toxicity.17,67 In a study of sea urchin (Paracentrotus lividus) embryos, PS-NH2 produced severe developmental defects.109 As evidence for pharmaceutical-assisted toxicity, ibuprofen exhibited synergistic toxicity with NPs by inhibiting growth in Chlorella pyrenoidosa.110
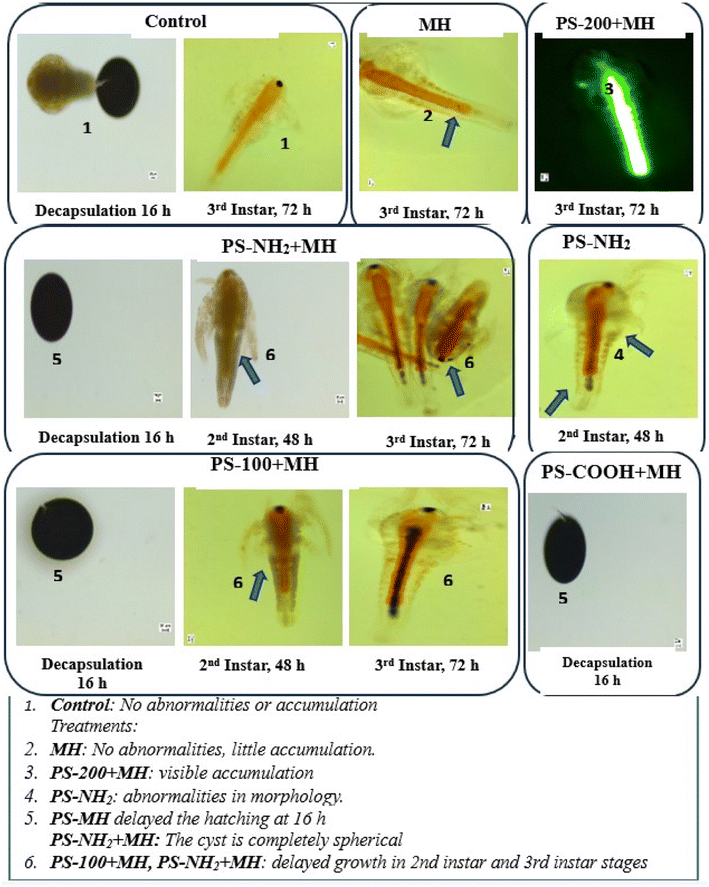 |
| Fig. 6 Representative figures showing major developmental changes of A. salina on exposure to different NPs and MH complexes. | |
3.3 Biochemical analysis
3.3.1 Total protein content.
The metabolic alterations in the test animals must be estimated and quantified to establish the influence of NPs and their complexes with MH on aquatic animals. Significant tissue total protein content changes indicate normal cellular and metabolic functioning disruptions. Fig. S2† depicts the changes in the total protein content of A. salina after exposure to several NPs and their complexes. All the NPs had a significant (P ≤ 0.0001) decrease in protein content compared to the control due to their interaction with the cell membrane, altered cellular response and metabolism. PS-NH2 treated groups showed substantially decreased protein content (28.54 ± 0.87) on comparing the functionalization. When compared to 500 nm PS, 100 nm PS enters cells more easily and lowers protein (31.85 ± 1.05) (P ≤ 0.001). Pharmaceutical MH-adsorbed NPs showed the least significant (P ≤ 0.001) difference in protein content; however, their prolonged accumulation will have a major effect on protein content and the overall health of organisms.
3.3.2 Reactive oxygen species.
An important factor in the toxicity caused by nanomaterials is ROS generation.111 ROS are highly toxic and capable of harming cells and biomolecules, resulting in oxidative stress.112 Common toxic mechanisms in microbial, algal, plant, animal, and mammalian/human cells include significant reductions in cell viability, changes in membrane integrity, reductions in cell function, and apoptosis as a result of the production of ROS.113 The physicochemical properties of nanomaterials, including the surface charge, particle size, and chemical composition have an impact on the ROS response.114 The percentage of ROS produced in A. salina after treatment with various NPs and their MH complexes is shown in Fig. 7A. Compared to the control, all of the particles demonstrated a significant amount of ROS production (P ≤ 0.0001). When comparing the functional groups, PS-NH2-treated animals demonstrated a higher ROS production (147.16 ± 0.87%) since less negatively charged amine PS interacts with cell membranes more efficiently than more negatively charged PS-COOH. This contact makes them more accessible to particular cells, tissues, or the nucleus, increasing the likelihood of a little higher oxidative stress and ROS generation (P ≤ 0.001). In an environmental study of mussels (Mytilus galloprovincialis), PS-NH2 increased ROS production, damaged lysosomes, and reduced shell length.115,116 In a study of human cell lines, PS-NH2 accumulated more in hepatocellular carcinoma (HepG2) cells than PS-COOH and PS, leading to more oxidative damage.117 In the experiment comparing sizes, PS-100 nm slightly boosted the ROS production (136.34 ± 1.05%) (P ≤ 0.001) more than 500 nm did. This may be due to the enhanced capacity of smaller NPs to get through biological barriers, which could lead to increased oxidative stress.97
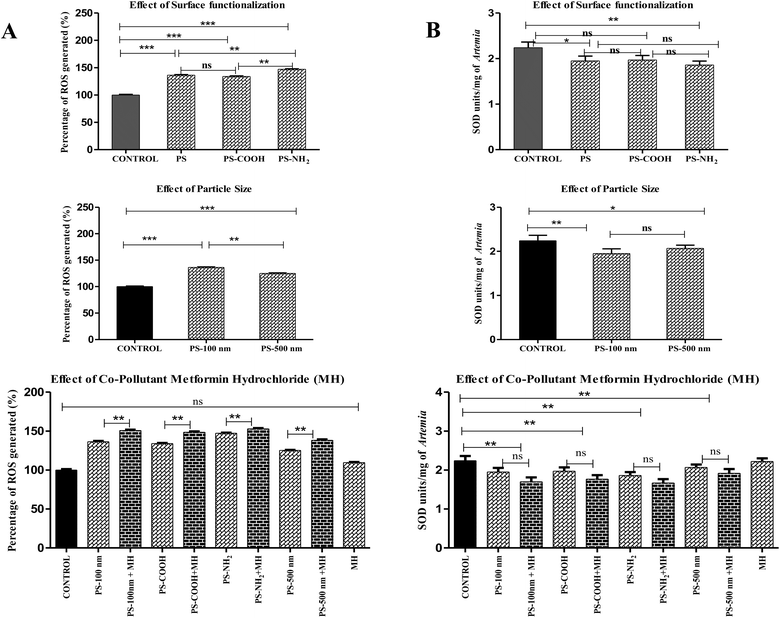 |
| Fig. 7 (A) The percentage of ROS generated. (***P ≤ 0.0001). (B) SOD units of A. salina upon exposure to various contaminants. (***P ≤ 0.01). | |
Similarly, smaller NPs induced greater ROS production than larger ones in Scenedesmus obliquus.55 Regarding the effect of MH, a small rise in ROS demonstrated their synergistic mode of action, but the difference in ROS generation between all pure particles and their complexes with MH was least significant (P ≤ 0.001). Since ROS production in the MH-treated groups was comparable to that in the control group, it was insignificant. The uptake of environmental medications by NPs enhanced their influence on oxidative stress compared to their corresponding pure particles. One of the research projects also proved this concept when PS complexed with roxithromycin increased oxidative damage in red tilapia (Oreochromis niloticus).59 Among the complexes, amine-functionalized PS adsorbed with MH generates more ROS (152.93 ± 1%) than other complexes.
3.3.3 SOD activity.
Fig. 7B displays the superoxide dismutase (SOD) units produced in Artemia following treatment with NPs and complexes. Several enzymatic defense mechanisms, including SOD, regulate the quantity and activity of toxic ROS in cells. SOD contributes to the transformation of the superoxide radical into hydrogen peroxide and molecular oxygen. This investigation discovered that species treated with NPs and complexes produced more ROS on average than control species. The anti-oxidant defense mechanisms like SOD and CAT may then be activated to protect the cells from oxidative damage. In this regard, SOD activity is expected to increase in treatment groups as a defense mechanism. However, the greater levels of ROS in the cells caused SOD to degrade,58 which is what caused the decrease in SOD activity for all of the samples compared to control (P ≤ 0.05). Upon decrease of enzymatic activity, oxidative damage might be autocatalyzed.118 Due to the association with increased ROS from the previous heading, animals treated with amine PS showed lower SOD units (1.86 ± 0.08) than animals treated with PS and PS-COOH. This is backed up by the evidence from the proton sponge hypothesis, where positive NPs initiate a chain of reactions causing cellular destruction.119 Comparatively, to 500 nm, SOD units in animals treated with 100 nm decreased (1.95 ± 0.10) as well. Parallel results were observed for PSNPs in Microcystis aeruginosa.120 SOD units were observed to be modestly decreased in MH complexes when comparing individual particles and their MH complexes, demonstrating synergistic toxicity similar to ROS production. Analogous to this, the toxicity of roxithromycin with PSNPs was examined for SOD activity in Daphnia magna, where an integrated biomarker response version 2 was applied to examine the combined response of PS and roxithromycin. It showed that PSNPs when co-exposed with roxithromycin altered the oxidative stress in Daphnia magna.59 Likewise, venlafaxine complexed with PVC-MPs increased SOD activity in Misgurnus anguillicaudatus.121
3.3.4 Catalase activity.
The anti-oxidant defense against ROS was mostly mediated by the catalase (CAT) enzyme. By lowering H2O2, the primary cellular precursor of the most harmful ROS, CAT is known to protect cells by reducing toxicity.122 Further, the disproportionation of superoxide leads to the production of oxygen and H2O2, which activates the CAT enzyme.58 The amount of catalase present in Artemia species after treatment with NPs and complexes is shown in Fig. 8A. In PS-NH2 treated groups compared to PS and PS-COOH, high ROS and low SOD levels resulted in enhanced catalase synthesis (0.053 ± 0.0008), however when compared to the control, all of the particles showed a substantial amount of catalase generation (P ≤ 0.0001). A similar increase in catalase activity was seen in PS 100 nm (0.05 ± 0.001) to that in PS 500 nm. For each NP, MH interaction raised the CAT enzyme synergistically, with the lowest significance value (P ≤ 0.01). Another pharmaceutical, sulfamethazine, also showed a negative impact on the anti-oxidant mechanism of marine medaka (Oryzias melastigma) when it coexists with PSNPs.123
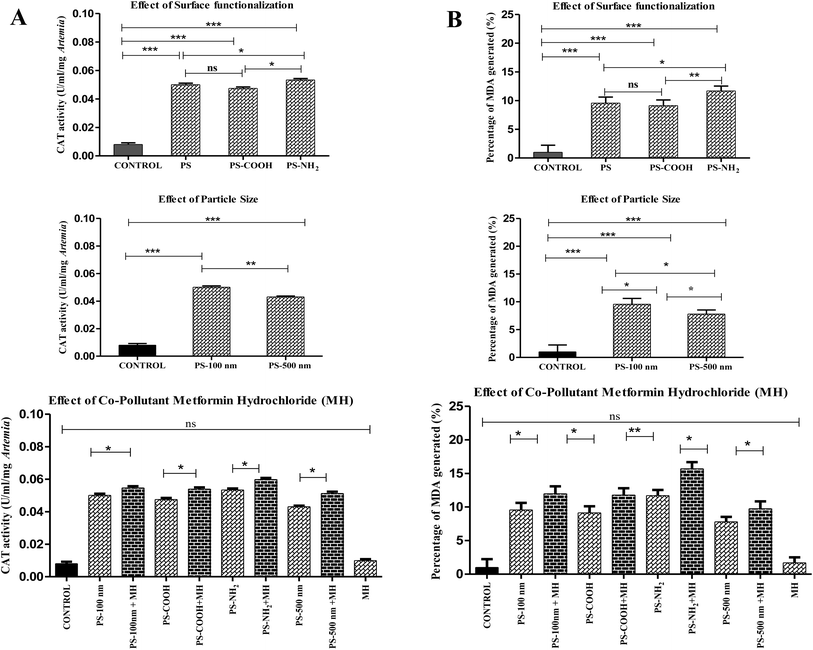 |
| Fig. 8 (A) Catalase activity of A. salina. (***P ≤ 0.0001). (B) Percentage of MDA generated in response to contaminants. (***P ≤ 0.0001). All values are expressed as mean ± SD. | |
3.3.5 LPO activity.
Lipid peroxidation (LPO) has been demonstrated to cause disruptions in the structural integrity of membranes and functional protein and DNA loss or alteration, which results in the synthesis of products like malondialdehyde (MDA). Assessing MDA levels will explore the LPO activity in response to contaminants.124 As explained in previous assays, amine PS had produced an increased amount of MDA (11.68 ± 0.87) compared to PS-COOH and PS (Fig. 8B) due to their surface charge and greater interaction with lipid membranes. LPO activity was observed to be decreased in the order of PS-100 nm > 500 nm, as smaller NPs interact with the lipid membranes more effectively. With the lowest significance value (P ≤ 0.01), MH interaction uplifted the LPO activity synergistically for each NP. Similarly, in the previous studies, sertraline complexed PSNPs increased the LPO activity in Tegillarca granosa,77 florfenicol complexed MPs increased LPO activity in Corbicula fluminea,125 and cefalexin increased LPO activity in Pomatoschistus microps.126
4 Conclusion
In conclusion, the comprehensive investigation into the adsorption study, toxicity, and biochemical effects of polystyrene nanoplastics (PSNPs) and their complexes with the environmental pharmaceutical metformin hydrochloride (MH) on the marine crustacean Artemia salina reveals a complex interplay of factors contributing to ecological harm. Adsorption kinetics and isotherm models confirmed physical and chemical adsorption mechanisms and a monolayer coverage of MH onto PSNPs, which were validated by zeta potential measurement, FESEM, and elemental analysis of particles following pre- and post-adsorption. Toxicological findings highlighted the impact of these particles on various physiological and biochemical parameters. One notable adverse effect observed was a significant reduction in the hatching rate of A. salina cysts following exposure to PSNPs and their MH complexes. This decline in the hatching rate indicates impaired reproductive ability, a crucial factor for population maintenance and ecosystem stability.
Furthermore, acute toxicity studies revealed a dose-dependent increase in mortality rates in A. salina exposed to PSNPs and their complexes. Morphological analyses revealed developmental abnormalities and particle accumulation in A. salina exposed to PSNPs and their complexes with MH. These observations suggest disruptions in normal growth and development processes, potentially leading to long-term detrimental effects on population dynamics and ecosystem functioning. Biochemical analyses further elucidated the underlying mechanisms of toxicity, with significant alterations in total protein content, reactive oxygen species (ROS) production, anti-oxidant enzyme activities, and lipid peroxidation (LPO) levels. PSNPs and PSNPs + MH complexes induced oxidative stress, characterized by increased ROS production and decreased antioxidant enzyme activities. Notably, amine-functionalized PSNPs exhibited the highest toxicity, causing excessive mortality, along with a delay in hatching rate and altered morphology and biochemical parameters. This is possibly due to the less negative surface potential of PS-NH2, leading to enhanced interactions with cell membranes. The smaller size of nanoparticles, particularly PS-100 nm, had greater toxic effects, likely due to increased penetration into biological tissues. The presence of pharmaceutical co-pollutant MH over the surface of PSNPs further influences their toxicity synergistically, which might lead to bioaccumulation of these particles in organisms. Overall, the study highlights the complex and multifaceted nature of the negative effects of PSNPs and their complexes with pharmaceuticals on aquatic organisms. These findings highlight the urgent need for effective mitigation strategies to minimize the environmental impact of nanoplastic pollution and mitigate its adverse effects on ecosystem health and biodiversity.
Abbreviation
• MH | Metformin hydrochloride |
• MNPs | Micronanoplastics |
• NPs | Nanoplastics |
• PSNPs | Polystyrene nanoplastics |
• PS | Plain polystyrene nanoplastics |
• PS-COOH | Carboxylated polystyrene nanoplastics |
• PS-NH2 | Aminated polystyrene nanoplastics |
• PS-100 nm | Plain polystyrene nanoplastics (100 nm size) |
• PS-200 nm | Plain polystyrene nanoplastics (200 nm size) |
• PS-500 nm | Plain polystyrene nanoplastics (500 nm size) |
• Plain | Represents non-functionalized PSNPs |
• Pristine | Represents PSNPs not adsorbed with MH |
•NPs-MH | Polystyrene nanoplastics adsorbed with metformin hydrochloride |
• ROS | Reactive oxygen species |
• SOD | Superoxide dismutase |
• LPO | Lipid peroxidation |
• MDA | Malondialdehyde |
• CAT | Catalase |
Author contributions
Durgalakshmi Rajendran: investigation, methodology, data curation, writing – original draft, writing – review & editing; Mahalakshmi Kamalakannan: investigation. George Priya Doss: writing –review & editing. Natarajan Chandrasekaran: conceptualization, supervision, resources, project administration, funding acquisition, writing – review & editing.
Conflicts of interest
The authors declare no conflict of interest.
Acknowledgements
The authors express their gratitude to the Indian Council of Medical Research (ICMR) for providing financial support through the Research Grant-F. No. 36/2/2020/Toxi/BMS.
References
- T. R. Walker and L. Fequet, TrAC, Trends Anal. Chem., 2023, 160, 116984 CrossRef CAS.
- N. B. Hartmann, T. Hüffer, R. C. Thompson, M. Hassellöv, A. Verschoor, A. E. Daugaard, S. Rist, T. Karlsson, N. Brennholt, M. Cole, M. P. Herrling, M. C. Hess, N. P. Ivleva, A. L. Lusher and M. Wagner, Environ. Sci. Technol., 2019, 53, 1039–1047 CrossRef CAS PubMed.
- B. Jiang, A. E. Kauffman, L. Li, W. McFee, B. Cai, J. Weinstein, J. R. Lead, S. Chatterjee, G. I. Scott and S. Xiao, Environ. Health Prev. Med., 2020, 25, 29 CrossRef PubMed.
- M. F. Cárdenas-Alcaide, J. A. Godínez-Alemán, R. B. González-González, H. M. N. Iqbal and R. Parra-Saldívar, Green Anal. Chem., 2022, 3, 100031 CrossRef.
- A. Amobonye, P. Bhagwat, S. Raveendran, S. Singh and S. Pillai, Front. Microbiol., 2021, 12, 768297 CrossRef.
- Q. Wang, C. A. Adams, F. Wang, Y. Sun and S. Zhang, Crit. Rev. Environ. Sci. Technol., 2022, 52, 3211–3243 CrossRef.
- J. Nath, J. De, S. Sur and P. Banerjee, Pathogens, 2023, 12(7), 888 CrossRef CAS PubMed.
- K. Y. Khan, G. Li, D. Du, B. Ali, S. Zhang, M. Zhong, P. J. Stoffella, B. Iqbal, X. Cui, L. Fu and Y. Guo, Environ. Pollut., 2023, 316, 120522 CrossRef CAS PubMed.
- L. Yin, X. Wen, D. Huang, C. Du, R. Deng, Z. Zhou, J. Tao, R. Li, W. Zhou, Z. Wang and H. Chen, Environ. Pollut., 2021, 290, 117999 CrossRef CAS PubMed.
- S. Rist, A. Baun and N. B. Hartmann, Environ. Pollut., 2017, 228, 398–407 CrossRef CAS PubMed.
- E. Besseling, E. M. Foekema, M. J. van den Heuvel-Greve and A. A. Koelmans, Environ. Sci. Technol., 2017, 51, 8795–8804 CrossRef CAS PubMed.
- R. Sussarellu, M. Suquet, Y. Thomas, C. Lambert, C. Fabioux, M. E. J. Pernet, N. Le Goïc, V. Quillien, C. Mingant, Y. Epelboin, C. Corporeau, J. Guyomarch, J. Robbens, I. Paul-Pont, P. Soudant and A. Huvet, Proc. Natl. Acad. Sci. U. S. A., 2016, 113, 2430–2435 CrossRef CAS PubMed.
- L. Hu, M. Chernick, A. M. Lewis, P. L. Ferguson and D. E. Hinton, PLoS One, 2020, 15, e0229962 CrossRef CAS PubMed.
- W. Wang, J. Ge, X. Yu and H. Li, Sci. Total Environ., 2020, 708, 134841 CrossRef CAS PubMed.
- I. Hansjosten, M. Takamiya, J. Rapp, L. Reiner, S. Fritsch-Decker, D. Mattern, S. Andraschko, C. Anders, G. Pace, T. Dickmeis, R. Peravali, S. Rastegar, U. Strähle, I. L. Hsiao, D. Gilliland, I. Ojea-Jimenez, S. V. Y. Ambrose, M. F. A. Belinga-Desaunay-Nault, A. O. Khan, I. Lynch, E. Valsami-Jones, S. Diabaté and C. Weiss, Environ. Sci.: Nano, 2022, 9, 375–392 RSC.
- L. Luan, X. Wang, H. Zheng, L. Liu, X. Luo and F. Li, Mar. Pollut. Bull., 2019, 139, 346–354 CrossRef CAS.
- E. Bergami, E. Bocci, M. L. Vannuccini, M. Monopoli, A. Salvati, K. A. Dawson and I. Corsi, Ecotoxicol. Environ. Saf., 2016, 123, 18–25 CrossRef CAS PubMed.
- W. Lin, R. Jiang, S. Hu, X. Xiao, J. Wu, S. Wei, Y. Xiong and G. Ouyang, Ecotoxicol. Environ. Saf., 2019, 180, 509–516 CrossRef CAS PubMed.
- H. Liu, L. Tian, S. Wang and D. Wang, Sci. Total Environ., 2021, 790, 148217 CrossRef CAS PubMed.
- T. Hao, Y. Gao, Z.-C. Li, X.-X. Zhou and B. Yan, Environ. Sci. Technol., 2023, 57, 2804–2812 CrossRef CAS PubMed.
- G. Vijayaraghavan, K. V. Neethu, B. P. Aneesh, A. Suresh, K. S. Saranya, S. Bijoy Nandan and K. V. Sharma, J. Toxicol. Environ. Health Sci., 2022, 14, 131–140 CrossRef.
- C. Henkel, T. Hüffer and T. Hofmann, Environ. Sci. Technol., 2022, 56, 14507–14516 CrossRef CAS.
- L. Z. Lara, C. Bertoldi, N. M. Alves and A. N. Fernandes, Sci. Total Environ., 2021, 796, 148983 CrossRef CAS PubMed.
- C. M. Cary, T. N. Seymore, D. Singh, K. N. Vayas, M. J. Goedken, S. Adams, M. Polunas, V. R. Sunil, D. L. Laskin, P. Demokritou and P. A. Stapleton, Part. Fibre Toxicol., 2023, 20(1), 16 CrossRef CAS PubMed.
- Z. Yao, H. J. Seong and Y.-S. Jang, Ecotoxicol. Environ. Saf., 2022, 242, 113933 CrossRef CAS PubMed.
- N. Kurchaba, B. J. Cassone, C. Northam, B. F. Ardelli and C. M. R. Lemoine, Toxics, 2020, 8, 1–16 CrossRef.
- S. Liu, J. Huang, W. Zhang, L. Shi, K. Yi, H. Yu, C. Zhang, S. Li and J. Li, J. Environ. Manage., 2022, 302, 113995 CrossRef CAS.
- M. Shi, Q. Xie, Z.-L. Li, Y.-F. Pan, Z. Yuan, L. Lin, X.-R. Xu and H.-X. Li, Environ. Pollut., 2023, 322, 121158 CrossRef CAS PubMed.
- E. Costigan, A. Collins, M. D. Hatinoglu, K. Bhagat, J. MacRae, F. Perreault and O. Apul, J. Hazard. Mater. Adv., 2022, 6, 100091 CrossRef CAS.
- J. He, X. Fu, F. Ni, G. Yang, S. Deng, J. P. Chen and F. Shen, Water Res., 2022, 224, 119024 CrossRef CAS PubMed.
- L. McDougall, L. Thomson, S. Brand, A. Wagstaff, L. A. Lawton and B. Petrie, Sci. Total Environ., 2022, 808, 152071 CrossRef CAS.
- R. Upadhyay, S. Singh and G. Kaur, Environ. Monit. Assess., 2022, 194, 803 CrossRef PubMed.
- A. B. A. Boxall, EMBO Rep., 2004, 5, 1110–1116 CrossRef CAS PubMed.
- E. P. Ambrosio-Albuquerque, L. F. Cusioli, R. Bergamasco, A. A. Sinópolis Gigliolli, L. Lupepsa, B. R. Paupitz, P. A. Barbieri, L. A. Borin-Carvalho and A. L. de Brito Portela-Castro, Environ. Toxicol. Pharmacol., 2021, 83, 103588 CrossRef CAS PubMed.
- J. L. Wilkinson, A. B. A. Boxall, D. W. Kolpin, K. M. Y. Leung, R. W. S. Lai, C. Galbán-Malagón, A. D. Adell, J. Mondon, M. Metian, R. A. Marchant, A. Bouzas-Monroy, A. Cuni-Sanchez, A. Coors, P. Carriquiriborde, M. Rojo, C. Gordon, M. Cara, M. Moermond, T. Luarte, V. Petrosyan, Y. Perikhanyan, C. S. Mahon, C. J. McGurk, T. Hofmann, T. Kormoker, V. Iniguez, J. Guzman-Otazo, J. L. Tavares, F. Gildasio De Figueiredo, M. T. P. Razzolini, V. Dougnon, G. Gbaguidi, O. Traoré, J. M. Blais, L. E. Kimpe, M. Wong, D. Wong, R. Ntchantcho, J. Pizarro, G.-G. Ying, C.-E. Chen, M. Páez, J. Martínez-Lara, J.-P. Otamonga, J. Poté, S. A. Ifo, P. Wilson, S. Echeverría-Sáenz, N. Udikovic-Kolic, M. Milakovic, D. Fatta-Kassinos, L. Ioannou-Ttofa, V. Belušová, J. Vymazal, M. Cárdenas-Bustamante, B. A. Kassa, J. Garric, A. Chaumot, P. Gibba, I. Kunchulia, S. Seidensticker, G. Lyberatos, H. P. Halldórsson, M. Melling, T. Shashidhar, M. Lamba, A. Nastiti, A. Supriatin, N. Pourang, A. Abedini, O. Abdullah, S. S. Gharbia, F. Pilla, B. Chefetz, T. Topaz, K. M. Yao, B. Aubakirova, R. Beisenova, L. Olaka, J. K. Mulu, P. Chatanga, V. Ntuli, N. T. Blama, S. Sherif, A. Z. Aris, L. J. Looi, M. Niang, S. T. Traore, R. Oldenkamp, O. Ogunbanwo, M. Ashfaq, M. Iqbal, Z. Abdeen, A. O’Dea, J. M. Morales-Saldaña, M. Custodio, H. de la Cruz, I. Navarrete, F. Carvalho, A. B. Gogra, B. M. Koroma, V. Cerkvenik-Flajs, M. Gombač, M. Thwala, K. Choi, H. Kang, J. L. C. Ladu, A. Rico, P. Amerasinghe, A. Sobek, G. Horlitz, A. K. Zenker, A. C. King, J.-J. Jiang, R. Kariuki, M. Tumbo, U. Tezel, T. T. Onay, J. B. Lejju, Y. Vystavna, Y. Vergeles, H. Heinzen, A. Pérez-Parada, D. B. Sims, M. Figy, D. Good and C. Teta, Proc. Natl. Acad. Sci. U. S. A., 2022, 119(8), e2113947119 CrossRef CAS PubMed.
- C. Wu, K. Zhang, X. Huang and J. Liu, Environ. Sci. Pollut. Res., 2016, 23, 8819–8826 CrossRef CAS PubMed.
- T. Atugoda, M. Vithanage, H. Wijesekara, N. Bolan, A. K. Sarmah, M. S. Bank, S. You and Y. S. Ok, Environ. Int., 2021, 149, 106367 CrossRef CAS PubMed.
- P. M. Gopinath, V. D. Parvathi, N. Yoghalakshmi, S. M. Kumar, P. A. Athulya, A. Mukherjee and N. Chandrasekaran, Chemosphere, 2022, 303, 135227 CrossRef CAS PubMed.
- L. H. M. L. M. Santos, S. Rodríguez-Mozaz and D. Barceló, Case Stud. Chem. Environ. Eng., 2021, 3, 100079 CrossRef CAS.
- P. M. Bradley, C. A. Journey, D. T. Button, D. M. Carlisle, J. M. Clark, B. J. Mahler, N. Nakagaki, S. L. Qi, I. R. Waite and P. C. VanMetre, Environ. Sci. Technol. Lett., 2016, 3, 243–249 CrossRef CAS.
- L. Gong, S. Goswami, K. M. Giacomini, R. B. Altman and T. E. Klein, Pharmacogenet. Genomics, 2012, 22, 820–827 CrossRef CAS PubMed.
- D. M. Zake, J. Kurlovics, L. Zaharenko, V. Komasilovs, J. Klovins and E. Stalidzans, PLoS One, 2021, 16, 1–27 CrossRef PubMed.
-
T. Sheleme, in Metformin – Pharmacology and Drug Interactions, IntechOpen, 2021 Search PubMed.
- J. Kim, C. W. Ahn, S. Fang, H. S. Lee and J. S. Park, Medicine, 2019, 98, e17918 CrossRef CAS PubMed.
- C. Littlejohn, J. B. Renaud, L. Sabourin, D. R. Lapen, J. J. Pappas, B. Tuteja, D. Hughes, E. Ussery, K. K. C. Yeung and M. W. Sumarah, Environ. Toxicol. Chem., 2023, 42, 1709–1720 CrossRef CAS PubMed.
- D. J. Caldwell, V. D'Aco, T. Davidson, K. Kappler, R. J. Murray-Smith, S. F. Owen, P. F. Robinson, B. Simon-Hettich, J. O. Straub and J. Tell, Chemosphere, 2019, 216, 855–865 CrossRef CAS PubMed.
- N. J. Niemuth, R. Jordan, J. Crago, C. Blanksma, R. Johnson and R. D. Klaper, Environ. Toxicol. Chem., 2015, 34, 291–296 CrossRef CAS PubMed.
- E. Ussery, K. N. Bridges, Z. Pandelides, A. E. Kirkwood, D. Bonetta, B. J. Venables, J. Guchardi and D. Holdway, Aquat. Toxicol., 2018, 205, 58–65 CrossRef CAS.
- W. Koagouw and C. Ciocan, J. Shellfish Res., 2018, 37, 467–474 CrossRef.
- D. Rajendran, R. P. Varghese, G. P. Doss, M. Shivashankar and N. Chandrasekaran, Environ. Toxicol. Pharmacol., 2023, 104249 CrossRef CAS PubMed.
- M. Albano, G. Panarello, D. Di Paola, F. Capparucci, R. Crupi, E. Gugliandolo, N. Spanò, G. Capillo and S. Savoca, Appl. Sci., 2021, 11, 3352 CrossRef CAS.
- V. Thiagarajan, N. Seenivasan, D. Jenkins, N. Chandrasekaran and A. Mukherjee, Aquat. Toxicol., 2022, 812, 152241 CAS.
- R. Umarani, A. K. Kumaraguru and N. Nagarani, Toxicol. Environ. Chem., 2012, 94, 1547–1556 CrossRef CAS.
- S. Schiavo, M. Oliviero, J. Li and S. Manzo, Environ. Sci. Pollut. Res., 2018, 25, 4871–4880 CrossRef CAS PubMed.
- L. Natarajan, D. Soupam, S. Dey, N. Chandrasekaran, R. Kundu, S. Paul and A. Mukherjee, Toxicol Rep, 2022, 9, 1953–1961 CrossRef CAS PubMed.
- H. Zhang, H. Cheng, Y. Wang, Z. Duan, W. Cui, Y. Shi and L. Qin, Front. Mar. Sci., 2022, 8, 800782 CrossRef.
- M. G. Albendín, V. Aranda, M. D. Coello, C. González-Gómez, R. Rodríguez-Barroso, J. M. Quiroga and J. M. Arellano, Int. J. Environ. Res. Public Health, 2021, 18(20), 10773 CrossRef PubMed.
- V. Thiagarajan, N. Seenivasan, D. Jenkins, N. Chandrasekaran and A. Mukherjee, Aquat. Toxicol., 2020, 225, 105541 CrossRef CAS PubMed.
- S. Zhang, J. Ding, R. M. Razanajatovo, H. Jiang, H. Zou and W. Zhu, Sci. Total Environ., 2019, 648, 1431–1439 CrossRef CAS.
- J. Wang, G. Liu, T. Li and C. Zhou, RSC Adv., 2015, 5, 29859–29871 RSC.
-
S. Lagergren, Svenska vetenskapsakademiens handlingar, 1898, 24, pp. 1–39 Search PubMed.
- Y. S. Ho and G. McKay, Process Biochem., 1999, 34, 451–465 CrossRef CAS.
- W. J. Weber and J. C. Morris, J. Sanit. Eng. Div., 1963, 89, 31–59 CrossRef.
- L. Fu, J. Li, G. Wang, Y. Luan and W. Dai, Ecotoxicol. Environ. Saf., 2021, 217, 112207 CrossRef CAS PubMed.
- P. Mishra, S. Vinayagam, K. Duraisamy, S. R. Patil, J. Godbole, A. Mohan, A. Mukherjee and N. Chandrasekaran, Environ. Sci. Pollut. Res., 2019, 26, 1537–1547 CrossRef CAS PubMed.
- V. V Arun, N. Saharan, V. Ramasubramanian, A. M. Babitha Rani, K. R. Salin, R. Sontakke, H. Haridas and D. G. Pazhayamadom, Sci. Rep., 2017, 7, 40394 CrossRef PubMed.
- M. R. Madhav, S. E. M. David, R. S. S. Kumar, J. S. Swathy, M. Bhuvaneshwari, A. Mukherjee and N. Chandrasekaran, Environ. Toxicol. Pharmacol., 2017, 52, 227–238 CrossRef CAS PubMed.
- V. Thiagarajan, L. Natarajan, R. Seenivasan, N. Chandrasekaran and A. Mukherjee, Environ. Res., 2019, 179, 108808 CrossRef CAS PubMed.
- M. M. Bradford, Anal. Biochem., 1976, 72, 248–254 CrossRef CAS PubMed.
- H. Wang and J. A. Joseph, Free Radical Biol. Med., 1999, 27, 612–616 CrossRef CAS PubMed.
- Y. Kono, Arch. Biochem. Biophys., 1978, 186, 189–195 CrossRef CAS PubMed.
- Y. Li and H. E. Schellhorn, J. Biomol. Tech., 2007, 18, 185–187 Search PubMed.
- R. Cui, M. C. Jong, L. You, F. Mao, D. Yao, K. Y. H. Gin and Y. He, Chemosphere, 2022, 286, 131735 CrossRef CAS PubMed.
- M. Gao, L. Bai, X. Li, S. Wang and Z. Song, Environ. Pollut., 2022, 306, 119349 CrossRef CAS PubMed.
- J. Guan, K. Qi, J. Wang, W. Wang, Z. Wang, N. Lu and J. Qu, Water Res., 2020, 184, 116205 CrossRef CAS PubMed.
- L. Qin, Z. Duan, H. Cheng, Y. Wang, H. Zhang, Z. Zhu and L. Wang, Environ. Pollut., 2021, 291, 118169 CrossRef CAS PubMed.
- W. Shi, Y. Han, S. Sun, Y. Tang, W. Zhou, X. Du and G. Liu, J. Hazard. Mater., 2020, 396, 122603 CrossRef CAS PubMed.
- V. Godoy, M. A. Martín-Lara, M. Calero and G. Blázquez, Process Saf. Environ. Prot., 2020, 138, 312–323 CrossRef CAS.
- M. A. Fawzy, H. M. Al-Yasi, T. M. Galal, R. Z. Hamza, T. G. Abdelkader, E. F. Ali and S. H. A. Hassan, Sci. Rep., 2022, 12, 8583 CrossRef CAS PubMed.
- B. Xu, F. Liu, P. C. Brookes and J. Xu, Mar. Pollut. Bull., 2018, 131, 191–196 CrossRef CAS PubMed.
- I. Langmuir, J. Am. Chem. Soc., 1918, 40, 1361–1403 CrossRef CAS.
- H. M. F. Freundlich, J. Phys. Chem., 1906, 57, 1100–1107 Search PubMed.
-
J. B. Hansen, in Ammonia, Springer Berlin Heidelberg, Berlin, Heidelberg, 1995, pp. 149–190 Search PubMed.
- P. Li, X. Zou, X. Wang, M. Su, C. Chen, X. Sun and H. Zhang, J. Hazard. Mater., 2020, 385, 121601 CrossRef CAS PubMed.
- A. Mollahosseini and A. Abdelrasoul, J. Environ. Sci., 2019, 81, 181–194 CrossRef CAS PubMed.
- M. Klavins, L. Klavins, O. Stabnikova, V. Stabnikov, A. Marynin, L. Ansone-Bertina, M. Mezulis and A. Vaseashta, Microplastics, 2022, 1, 520–535 CrossRef.
- R. Trevisan, P. Ranasinghe, N. Jayasundara and R. T. Di Giulio, Toxics, 2022, 10(6), 326 CrossRef CAS PubMed.
- S. Lu, K. Zhu, W. Song, G. Song, D. Chen, T. Hayat, N. S. Alharbi, C. Chen and Y. Sun, Sci. Total Environ., 2018, 630, 951–959 CrossRef CAS PubMed.
- L. Ramirez Arenas, S. Ramseier Gentile, S. Zimmermann and S. Stoll, Water Environ. Res., 2020, 92, 1184–1194 CrossRef CAS PubMed.
- L.-J. Feng, Y. Shi, X.-Y. Li, X.-D. Sun, F. Xiao, J.-W. Sun, Y. Wang, X.-Y. Liu, S.-G. Wang and X.-Z. Yuan, Environ. Pollut., 2020, 263, 114453 CrossRef CAS PubMed.
- Y. Xiong, J. Zhao, L. Li, Y. Wang, X. Dai, F. Yu and J. Ma, Water Res., 2020, 184, 116100 CrossRef CAS PubMed.
- I. Velzeboer, C. J. A. F. Kwadijk and A. A. Koelmans, Environ. Sci. Technol., 2014, 48, 4869–4876 CrossRef CAS PubMed.
- M. Zahmatkesh Anbarani, A. Najafpoor, B. Barikbin and Z. Bonyadi, Sci. Rep., 2023, 13(1), 17989 CrossRef CAS PubMed.
-
https://pubchem.ncbi.nlm.nih.gov/compound/Metformin-HydrochloridePubChemCompoundSummaryforCID14219MetforminHydrochloride
.
- B. R. Kiran, H. Kopperi and S. Venkata Mohan, Rev. Environ. Sci. Biotechnol., 2022, 21, 169–203 CrossRef PubMed.
- L. Sun, K. Liao and D. Wang, Sci. Total Environ., 2021, 768, 144362 CrossRef CAS PubMed.
- X.-D. Sun, X.-Z. Yuan, Y. Jia, L.-J. Feng, F.-P. Zhu, S.-S. Dong, J. Liu, X. Kong, H. Tian, J.-L. Duan, Z. Ding, S.-G. Wang and B. Xing, Nat. Nanotechnol., 2020, 15, 755–760 CrossRef CAS PubMed.
- Y. Chae, D. Kim and Y.-J. An, Aquat. Toxicol., 2019, 216, 105296 CrossRef CAS PubMed.
- N. J. Niemuth, R. Jordan, J. Crago, C. Blanksma, R. Johnson and R. D. Klaper, Environ. Toxicol. Chem., 2015, 34, 291–296 CrossRef CAS PubMed.
- M. R. Camara, Aquacult. Rep., 2020, 17, 100359 Search PubMed.
- H. Tajik, M. Moradi, S. Rohani, A. Erfani and F. Jalali, Molecules, 2008, 13, 1263–1274 CrossRef CAS PubMed.
- P. A. Athulya, Z. Sunil, S. Manzo and N. Chandrasekaran, J. Environ. Manage., 2023, 348, 119367 CrossRef CAS PubMed.
- P. Charoeythornkhajhornchai, T. Kunjiek, S. Chaipayang and S. Phosri, Emerging Contam., 2023, 9(4), 100253 CrossRef CAS.
- L. Manfra, A. Rotini, E. Bergami, G. Grassi, C. Faleri and I. Corsi, Ecotoxicol. Environ. Saf., 2017, 145, 557–563 CrossRef CAS PubMed.
- M. S. L. Yee, L. W. Hii, C. K. Looi, W. M. Lim, S. F. Wong, Y. Y. Kok, B. K. Tan, C. Y. Wong and C. O. Leong, Nanomaterials, 2021, 11, 1–23 CrossRef PubMed.
- J. C. Prata, B. R. B. O. Lavorante, M. D. C. B. S. M. Montenegro and L. Guilhermino, Aquat. Toxicol., 2018, 197, 143–152 CrossRef CAS PubMed.
- M. Oliviero, S. Schiavo, S. Dumontet and S. Manzo, Sci. Total Environ., 2019, 651, 756–765 CrossRef CAS PubMed.
- E. Bustos-Obregon and Á. Vargas, Biol. Res., 2010, 43(3), 357–362 Search PubMed.
- C. Della Torre, E. Bergami, A. Salvati, C. Faleri, P. Cirino, K. A. Dawson and I. Corsi, Environ. Sci. Technol., 2014, 48, 12302–12311 CrossRef CAS PubMed.
- F. Wang, B. Wang, H. Qu, W. Zhao, L. Duan, Y. Zhang, Y. Zhou and G. Yu, Environ. Pollut., 2020, 263, 114593 CrossRef CAS PubMed.
- A. Manke, L. Wang and Y. Rojanasakul, BioMed Res. Int., 2013, 2013, 1–15 CrossRef PubMed.
- Z. Clemente, V. L. Castro, C. M. Jonsson and L. F. Fraceto, J. Nanopart. Res., 2014, 16, 2559 CrossRef.
- D. Pan, O. Vargas-Morales, B. Zern, A. C. Anselmo, V. Gupta, M. Zakrewsky, S. Mitragotri and V. Muzykantov, PLoS One, 2016, 11, e0152074 CrossRef PubMed.
- A. A. Shvedova, A. Pietroiusti, B. Fadeel and V. E. Kagan, Toxicol. Appl. Pharmacol., 2012, 261, 121–133 CrossRef CAS PubMed.
- T. Balbi, G. Camisassi, M. Montagna, R. Fabbri, S. Franzellitti, C. Carbone, K. Dawson and L. Canesi, Chemosphere, 2017, 186, 1–9 CrossRef CAS PubMed.
- M. Auguste, T. Balbi, C. Ciacci, B. Canonico, S. Papa, A. Borello, L. Vezzulli and L. Canesi, Front. Immunol., 2020, 11, 426 CrossRef CAS PubMed.
- Y. He, J. Li, J. Chen, X. Miao, G. Li, Q. He, H. Xu, H. Li and Y. Wei, Sci. Total Environ., 2020, 723, 138180 CrossRef CAS PubMed.
- J. A. Escobar, M. A. Rubio and E. A. Lissi, Free Radical Biol. Med., 1996, 20, 285–290 CrossRef CAS PubMed.
- A. E. Nel, L. Mädler, D. Velegol, T. Xia, E. M. V. Hoek, P. Somasundaran, F. Klaessig, V. Castranova and M. Thompson, Nat. Mater., 2009, 8, 543–557 CrossRef CAS PubMed.
- X. Zheng, Y. Yuan, Y. Li, X. Liu, X. Wang and Z. Fan, Environ. Sci. Pollut. Res., 2021, 28, 13394–13403 CrossRef CAS PubMed.
- H. Qu, R. Ma, B. Wang, J. Yang, L. Duan and G. Yu, J. Hazard. Mater., 2019, 370, 203–211 CrossRef CAS PubMed.
- M. Oliveira, V. L. Maria, I. Ahmad, A. Serafim, M. J. Bebianno, M. Pacheco and M. A. Santos, Environ. Pollut., 2009, 157, 959–967 CrossRef CAS PubMed.
- Y. T. Zhang, H. Chen, S. He, F. Wang, Y. Liu, M. Chen, G. Yao, Y. Huang, R. Chen, L. Xie and J. Mu, Ecotoxicol. Environ. Saf., 2021, 226, 112820 CrossRef CAS PubMed.
- S. Gaweł, M. Wardas, E. Niedworok and P. Wardas, Wiad. Lek., 2004, 57, 453–455 Search PubMed.
- L. Guilhermino, L. R. Vieira, D. Ribeiro, A. S. Tavares, V. Cardoso, A. Alves and J. M. Almeida, Sci. Total Environ., 2018, 622–623, 1131–1142 CrossRef CAS PubMed.
- E. Fonte, P. Ferreira and L. Guilhermino, Aquat. Toxicol., 2016, 180, 173–185 CrossRef CAS PubMed.
|
This journal is © The Royal Society of Chemistry 2024 |