DOI:
10.1039/D3LP00077J
(Review Article)
RSC Appl. Polym., 2023,
1, 132-157
Recent progress in the development of conductive hydrogels and the application in 3D printed wearable sensors
Received
9th June 2023
, Accepted 28th July 2023
First published on 22nd August 2023
Abstract
Compared with traditional device-type sensors, flexible wearable sensors have attracted fast-growing attention owing to the excellent stretchability and strain sensitivity. Conductive hydrogels, a kind of hydrophilic polymer material with a three-dimensional (3D) network structure, are excellent candidate materials for wearable sensors because of their conductivity, sensitivity, stretchability, biocompatibility, and high adjustability. The structural modifiability also endows conductive hydrogels with more functionality such as self-healing and adhesiveness. In the process of preparing wearable sensors, the traditional templating-based methods are not suitable for customer-tailored applications. And more importantly, dimensional accuracy cannot be guaranteed for the traditional methods, which limits the applications. A 3D printing technique, which constructs 3D structures by discrete-cumulative methods, can control the shape and accuracy based on a computer model. Compared with templating-based methods, 3D printing technology has more advantages in constructing wearable sensors. In recent years, there have been increasing research efforts being paid to integrating 3D printing technology with conductive hydrogels, while there is less effort spent on summarizing the current research advances. In this paper, we reviewed the research progress of 3D printing in wearable sensors based on conductive hydrogels, with an emphasis on the classification of conductive hydrogels, the mechanisms of wearable sensors and applications of 3D printing methods. Finally, the remaining problems and opportunities were discussed.
1. Introduction
Hydrogels, as a kind of polymer material with three-dimensional (3D) network structures, are generally formed by chemically or physically crosslinking the polymer chain segments. Owing to the abundant hydrophilic groups, hydrogels exhibit the feature of swelling but not of being dissolved in aqueous solution. Actually, the proportion of their internal water can often reach a value above 90%.1,2 In addition, the polymer structures can be decorated by different functional groups, which would endow hydrogels with strong functional adjustability such as stretchability, self-adhesiveness, self-healing, conductivity, antibacterial, printability, biocompatibility, and degradability, etc.3–7 Generally, the polymerization and crosslinking process of hydrogels involves the formation of various types of chemical bond, which can be mainly divided into covalent bonds and non-covalent bonds.8,9 For covalent bonds, the features of robustness and stability provide strong mechanical properties for hydrogels, while for non-covalent bonds, including hydrogen bonds, coordination bonds, electrostatic interactions, hydrophobic interactions, etc., they are mainly reversible dynamic bonds, which enable great freedom for the design of the behaviors of hydrogels. For instance, when stimulated by external factors such as temperature, pH, pressure, and strain, the bonds will get broken, and after removal of the stimulus, the broken bonds can be re-bonded and restored to the initial state, based on which a specific response to the external signal can be designed by adjusting the polymer structures.10–12 Due to these excellent properties, hydrogels have been widely used in many fields such as biomedicine, drug delivery, environmental engineering and biosensing,13,14 and increasing research attention has been attracted in recent years.
For applications in sensing areas, hydrogels are generally required to be electrically conductive to achieve the conversion of external stimuli to electrical signals. However, most of the original hydrogels have poor electrical conductivity even with abundant water inside, which impedes the applications in wearable sensors. To make full use of the application potential, it is significant to develop conductive hydrogels. Conductive hydrogels, which can be divided into electro-type and ion-type, are realized through the addition of conductive materials.15,16 Combined with the excellent flexibility, conductive hydrogels have great advantages in applications of wearable sensors. Normally, the traditional sensors often employ rigid metal or semiconductor materials, leading to bulky designs and limited compatibility with the human body. In contrast, flexible wearable sensors based on conductive hydrogels offer superior compatibility and conformability.17,18
Wearable sensors have revolutionized the way we monitor and track the various aspects of our lives. Compact, portable devices are equipped with sensors that can gather real-time data on our physical activities, health parameters, and even emotional states. From monitoring heart rate and sleep patterns to tracking fitness levels and managing chronic diseases, wearable sensors have found widespread applications in healthcare, sports, and personal well-being. In recent years, there has been an exponential growth in the development and adoption of wearable sensor technology. There are several transduction mechanisms employed in wearable sensors, including piezoresistive, capacitive, triboelectric, and piezoelectric mechanisms.19–22 As an excellent candidate material for the preparation of wearable sensors, conductive hydrogels are often combined with these transduction principles to prepare high-performance wearable sensors which can accurately and efficiently monitor human health parameters in real-time.
With the rapid development of sensing theory and great improvement of the sensing performance of conductive hydrogels, higher requirements are also put forward for the production technology. The traditional templating-based methods rely on moulds, which are only suitable for mass manufacturing and cannot meet the requirements of personalized demands. Meanwhile, the templating-based methods would consume a lot of raw materials, and produce large amounts of production waste.23 3D printing technology, also known as additive manufacturing, is a computer-aided manufacturing technology that fabricates the objects by stacking layers on layers.24 There are around ten different types of 3D printing technique, because of which great freedom in material selection can be realized. As a result, much effort has been spent by material users to develop 3D printable functional materials for application in different scenarios. Besides, an extremely high resolution can be achieved with the development of 3D printing machines. The computer-aided feature also frees the designers’ mind to design the structures. Moreover, 3D printing technology is convenient to operate with a low production cost, greatly improving the production efficiency.25,26 In recent years, great advances have been witnessed to integrate 3D printing technology with conductive hydrogels for the preparation of wearable sensors, providing more possibilities for the improvement of sensing ability. Fig. 1 illustrates different types of conductive hydrogel and their properties. It also demonstrates the various transduction mechanisms of wearable sensors and the types of 3D printing technique applicable to conductive hydrogels along with their working principles.
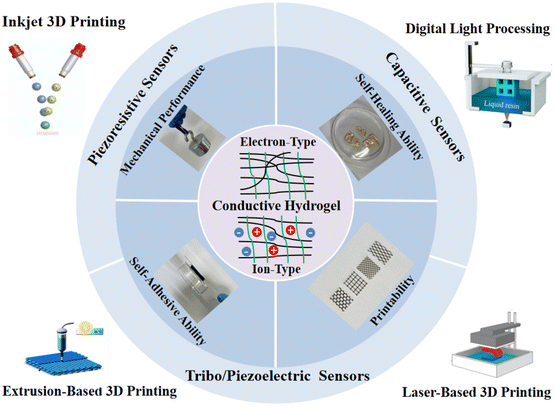 |
| Fig. 1 Classification and properties of conductive hydrogels and the related 3D printing techniques. | |
At present, many breakthroughs have been made regarding the theories and applications of conductive hydrogels and 3D printing technology in wearable sensors. However, the research into combining these two is still at the beginning stage, even though it is significant. It is important to summarize the current research status to provide more guidance for future development. This review mainly describes the classification and properties of conductive hydrogels, sensing mechanisms and 3D printing technology applicable to manufacture conductive hydrogels-based sensors in detail. The challenges and future developments in this field are also discussed. Hopefully, this work would stimulate more research interest in this area.
2. Classification and properties of the conductive hydrogels
2.1 Classification of the conductive hydrogels
When placed in ionic aqueous solution, ion channels will be formed in the hydrogels, generating the ionic conductivity inside.27 However, for sensors applied in vitro, the conductivity generated by external ionic solutions is limited. Considering the intrinsic insulation feature, it is of great significance to modify hydrogels to improve the conductivity. According to the conduction mechanisms, modified hydrogels can be divided into electron-type and ion-type.28,30 For the electron-type, electronic conductivity is normally achieved by filling or coating the hydrogels with conductive materials. In addition, the hydrogels constructed on the basis of polymers containing a π system also show electronic conductivity.29 For the ion-type, it is necessary to ensure there is a sufficient number of free ions inside to realize the enhanced conductivity by forming ion channels.
2.1.1 Electron-type.
Electron-type hydrogels achieve electrical conductivity through freely moving electrons in metals as well as delocalized π electrons in other materials. Since most of the hydrogels are insulated, the realization of electronic conductivity often requires the addition of a conductive medium. Currently, carbon nanocomposites, metal nanofillers and conductive polymers are most commonly used.
Carbon nanocomposite-based conductive hydrogels.
Carbon nanomaterials have excellent electrical conductivity due to the formation of delocalized π bonds, which can form freely moving electrons. Among the different types of carbon nanomaterial, carbon nanotubes (CNTs) and graphene have been most widely used as conductive fillers for the preparation of conductive hydrogels because of the large surface area and good mechanical properties.31
Carbon nanotubes are hollow cylinders made from graphite sheets, with a diameter on the nanoscale and a length on the nano- or micron scale.32 Due to the special structure, conductive hydrogels achieved using CNTs as conductive fillers have better mechanical properties, exhibiting obvious advantages in the application of wearable sensors.33 However, the direct addition of carbon nanotubes would easily induce aggregation for hydrogels containing a large amount of water, affecting the mechanical uniformity and electrical conductivity.34 The two main methods to relieve aggregation are to obtain a hydrophilic surface by surface modification of the carbon nanotubes or to establish valence bonds between the carbon nanotubes and hydrogel matrix by a hydrophilic dispersant.35–37 For the former, although the dispersion of carbon nanotubes in the hydrogel can be achieved, the insufficient degree of modification would lead to a loose interaction between the packed particles and the hydrogel matrix, resulting in the easy separation of the conductive fillers. Moreover, the modification will affect the conductivity of the CNTs, which limits the application of this method. As for the method of introducing the hydrophilic dispersant, the connection is established under both hydrophilic and hydrophobic interactions, effectively avoiding the damage to the structure of the conductive material itself. Compared with the surface modification method, the interaction induced by introducing the hydrophilic dispersant is much stronger and the dispersion effect is better. For example, Lu et al.38 introduced CNTs and cellulose nanofibers in the process of hydrogel polymerization. As a kind of hydrophilic material, cellulose nanofibers not only enhanced the toughness of the hydrogel, but also facilitated the dispersion of the carbon nanotubes. Fig. 2 shows the schematic diagram of CNT dispersion and the corresponding microstructural characterization, which clearly indicate the good dispersion of the nanofillers and excellent electrical conductivity of the modified hydrogel. Similarly, Chen et al.39 wrapped carbon nanotubes with alginate, a biocompatible material containing a large number of hydroxyl and carbonyl groups, through which a good dispersion of CNTs, as well as enhanced electrical conductivity (2.765 S m−1) and mechanical properties (a tensile strength of 332.9 kPa, elongation of 584.6%, Young's modulus of 91.5 kPa), have been achieved.
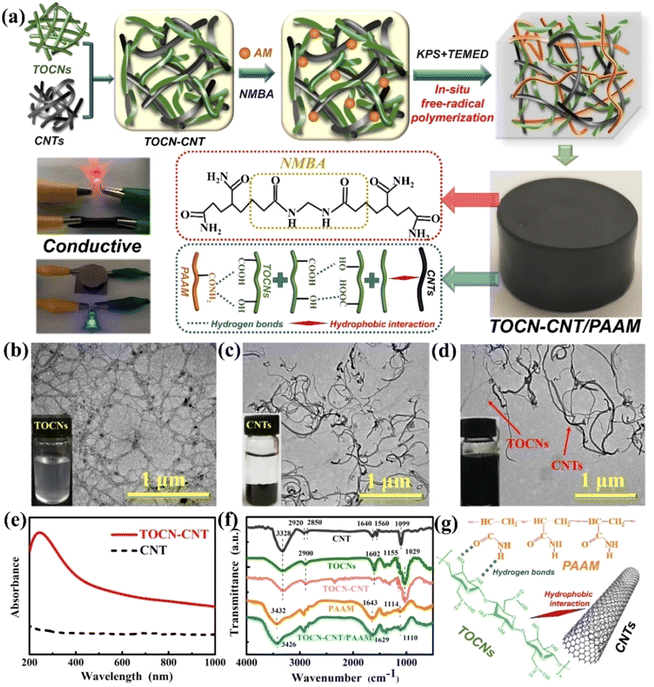 |
| Fig. 2 (a) Synthesis process of the TOCN–CNT/PAAM hydrogels. TEM figures of (b) TOCNs, (c) CNT, and (d) TOCN–CNT nanocomposites. (e) UV-vis data of CNT and TOCN–CNT. (f) FTIR data of TOCN–CNT/PAAM hydrogel and the components. (g) Illustration of the interactions among PAAM, TOCNs, and CNTs.38 | |
The addition of carbon nanotubes enhances the conductivity and mechanical properties of hydrogels.40 In addition, good 3D printability of conductive hydrogels can also be realized by adjusting the type of hydrogel monomer and the content of carbon nanotubes, which is significant for the manufacturing of wearable sensors. However, how to optimize the dispersion of CNTs and printability remains a challenge.
Graphene, as a two-dimensional, single-layer carbon atom with a hexagonal lattice structure and hybrid sp2 orbitals, is well-known for its extreme surface area and excellent properties.41–44 In addition, one of its derivatives, GO, has more functional groups, such as carboxylic, epoxide and hydroxyl, which can help it disperse better in aqueous solutions.42,44 However, compared with graphene, the electrical conductivity of GO is worse due to the lattice collapse. So for the application of graphene/GO as a conductive filler, it is necessary to consider both the dispersion ability and conductivity of the conductive fillers according to the design requirements of the matrix material. For example, Mendes et al.,45 significantly improved the conductivity of hydrogels by adding a small amount of graphene oxide sheets with an ultra-high electroactive surface area based on gelatin methacryloyl (GelMA). The addition of GO also improves the printability and shape fidelity of the hydrogels, making them more suitable for the subsequent 3D printing technology. After being further processed by chemical reactions, reduced graphene oxide (rGO)43 with better electrical conductivity can be achieved, which is preferred for use in electrochemical applications.46–48 Yang et al. prepared the Pyy–rGO–Pyy sandwiched model through a simple self-assembly reaction using the redox reaction between pyrrole and GO.49 The formation of this model effectively improves the conductivity of hydrogels and therefore exhibits excellent pressure sensitivity (gauge factor ≥ 1.98). The pressure changes can be quickly converted into readable electrical signals, which is important for the sensors applied to human health monitoring. However, the reduction reaction reduces the oxygen-containing functional groups of rGO, making it hard to disperse it in water. Moreover, when it is added to the inside of hydrogels, the problem of packing aggregation would easily happen.
Because of the formation of delocalized π bonds, the excellent mechanical properties and large specific surface area, carbon nanocomposites have certain advantages in the preparation of wearable sensors. However, when applied to hydrogel systems, the main problem of carbon nanofillers is the insolubility in water, which would lead to easy aggregation of the filling materials, affecting the performance of conductive hydrogels. Currently, the methods of surface modification of carbon materials and the introduction of hydrophilic dispersant are mainly adopted to resolve these issues. Nevertheless, the natural blackness and opacity of carbon materials greatly limit their use in light-sensing devices.
Metal nanoparticle-based conductive hydrogels.
As a good conductor, metal can be used as a conductive filler in the preparation of conductive hydrogels and the conductivity is generally much better than that of carbon conductive materials.50 In order to meet the stability requirement of nanoparticles, the commonly used metals include gold, silver and some precious metals.51–54
Silver nanowires/nanoparticles are a widely used material with excellent durability and mechanical properties, which endowed them with obvious advantages over other materials as a conductive material filled inside hydrogels.55 Shen et al.56 used polydopamine to achieve the deposition of silver nanoparticles inside the hydrogel through in situ reduction. The prepared porous hydrogels exhibit good mechanical strength (2.13–3.20 MPa) and high piezoresistive sensitivity (up to 9.34 MPa−1), which can simultaneously monitor large movements and small pressure signals of the human body. When used as wearable sensors, the good adhesion between the sensor and human skin is required to avoid the side effect from the gap between the sensor and human skin. To achieve good adhesion, Hao et al.57 introduced tannic acid (TA) and Ag nanoparticles into the gel at the same time, which could realize rapid polymerization through the dynamic catalytic system of TA–Ag, and achieve rapid dissipation of deformation energy through the dynamic interaction of hydrogen bonds between the TA and Ag as well (Fig. 3). As a result, the prepared gel presented good flexibility and tensile effect. The existence of a dynamic interaction also endowed the gel with self-healing properties. More importantly, the introduction of TA makes the hydrogel self-adhesive to a variety of surfaces without the need for external adhesives, resulting in high accuracy when used as a sensor.
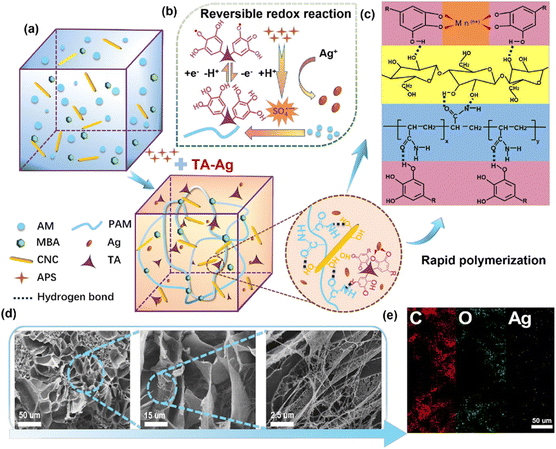 |
| Fig. 3 (a) Polymerization process of the hydrogel. (b) TA–Ag double-catalyzes APS to generate free radicals, leading to the free radical polymerization of AM monomers. (c) Internal bonding mechanism of the hydrogel. (d) Cross-sectional SEM images of freeze-dried hydrogels reveal porous interconnections and (e) related element mappings (C, O, and Ag).57 | |
In addition, the good bactericidal effect of silver can inhibit the activity of enzymes, RNA and DNA by destroying microbial cell membranes and cooperating with electron donor groups such as amine, mercaptan and hydroxyl, and ultimately, kill the bacteria.58 Therefore, conductive hydrogels containing silver fillers can also be used as a bactericidal and bacteriostatic material in some medical fields such as wound treatment.59
Generally, metal nanoparticle-based conductive hydrogels present better conductivity than carbon nanocomposite-based conductive hydrogels. However, the problem of uneven dispersion inside the hydrogels is a common issue. In order to be used in 3D printing technology for preparing wearable sensors, it is necessary to ensure the uniformity of the printing materials, which means further research on metal nanoparticle-based conductive hydrogels is needed.
Conductive polymer-based conductive hydrogels.
As a kind of organic compound, a conductive polymer has not only a conductivity comparable to an inorganic compound or semiconductor material, but also good flexibility, which enables it to be an excellent candidate for preparing wearable sensors.60 Compared with the nanocomposite-based hydrogels, the hydrogels prepared by conductive polymers do not have structural differences between the hydrogel matrix and conductive materials, so the strong interface effect commonly existing between organic and inorganic materials would be absent. As a result, the conductive polymer-based hydrogels have more advantages when considering the mechanical properties and electrical conductivity. At present, the conductive polymers commonly used for preparing conductive hydrogels include poly(3,4-ethylene-dioxythiophene) (PEDOT), polythiophene (PTh), polypyrrole (PPy) and polyaniline (PANI).61 These conductive polymers can be directly used as a conductive hydrogel matrix. However, due to the poor mechanical properties and strong rigidity of conductive polymers, the hydrogels obtained generally have certain limitations in the application of wearable sensors.62 To resolve this issue, conductive polymers are usually copolymerized with other monomers, integrating the good conductivity of the conductive polymer with the good mechanical properties of the monomers. For instance, Chen et al.63 used 2-ureido-4[1H]-pyrimidine (UPy) as a crosslinking point to form a polyaniline/poly(4-styrene sulfonic acid) (PANI/PSS) network and synthesized a hydrogel with good tensile and self-healing properties. The hydrogel could be shaped into various shapes and recovered within 30 s due to the presence of hydrogen bonds, which enable it be applicable in 3D printing technology and the preparation of wearable sensors. Fig. 4 shows the conductivity, sensing performance and self-healing ability of the as-prepared hydrogel, which illustrate the good application potential in the wearable sensors. In addition, conductive polymers can further be combined with conductive nanofillers to achieve enhanced conductivity.64
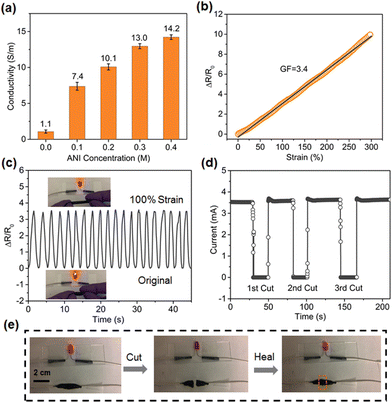 |
| Fig. 4 Testing of the electrical properties of polymer conductive hydrogels. (a) Conductivity of hydrogels with different ANI concentrations. (b) Variation of hydrogel resistance under applied strain (ΔR/R0). (c) Change in the resistance of the conductive hydrogel during the cyclic test. (d and e) Changes in LED bulb brightness before and after self-healing.63 | |
Conductive polymers produce electrical conductivity through the free movement of delocalized π electrons to form an electronic pathway.65 As polymer materials, conducting polymers have certain advantages over inorganic metals and carbon materials when combined with a hydrogel matrix. Nonetheless, most conductive polymers are rigid, and easy to break. For application in flexible sensors, it is often needed to combine them with other flexible materials, or integrate them with media that can generate non-covalent bonds to achieve better mechanical properties.
2.1.2 Ion-type.
Ionic conducting hydrogels rely on the free moving ions inside the hydrogels to form ion channels.66 Normally, ionic conductivity can be achieved by segment motion, ion hopping, or a vehicle mechanism. Compared with electron-type, ionic conductive hydrogels have higher transparency, which is more suitable for photoelectronics applications. Meanwhile, the absence of fillers also reduces the toxicity of the conductive hydrogel, which is advantageous in the application of human health monitoring.67–69
However, in order to establish the ion migration pathway, ionic hydrogels have a strong dependence on ionic aqueous solutions. The existence of aqueous solutions makes ionic conductive hydrogels more sensitive to temperature changes: when the temperature is lower than a certain degree, the hydrogel will get frozen, damaging the mechanical structure.70 On the other hand, when the temperature rises, the tendency of water to evaporate from the hydrogel increases, resulting in a loss of ionic conductivity.71
However, by taking advantage of the sensitivity of ionic conductive hydrogels to temperature, wearable sensor for testing temperature changes can be designed.72–74 For example, Pang et al.75 incorporated a PVP/TA/Fe3+cross-linked network into a P(NIPAAm-co-AM) ionic hydrogel. The prepared hydrogel has good conductivity (0.79 S m−1) and excellent transparency. By introducing the thermally sensitive material PNIPAAm into the ion-type hydrogel, a rapid volume shrinkage and electrical signal changes can be realized as the temperature rises to a certain degree (lower critical solution temperature, LCST), which indicates the capability of being applied as a wearable temperature sensor. To deal with the cryogenic freezing of hydrogels, one of the main methods is to replace the solution inside the hydrogel with some solution which has a lower ice point, so that the normal mechanical state can be maintained at a low temperature.76 For instance, Zhao et al.77 obtained ultralow-temperature freezing resistance by immersing carboxymethyl chitosan hydrogel in calcium chloride solution. The prepared hydrogel can remain non-frozen at −50 °C and has 90% transparency, ensuring the normal operation of the hydrogel-based sensor at low temperatures (Fig. 5).
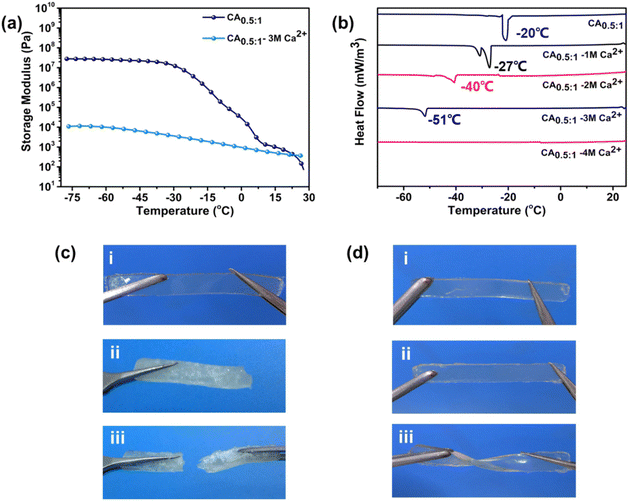 |
| Fig. 5 Antifreezing test of ionic conductive hydrogel. (a) DMA curves of CA0.5 : 1 and CA0.5 : 1-3 M Ca2+ when the temperature changes from 25 to −75 °C, (b) DSC curves of CA0.5 : 1 and CA0.5 : 1-Ca2+ when the temperature changes from 25 to −75 °C, and (c and d) images of CA0.5 : 1 and CA0.5 : 1-3 M Ca2+ bending at −50 °C, respectively.77 | |
In general, ionic conductive hydrogels are transparent in color and sensitive to temperature, and have advantages in specific applications. However, the evaporation problem of aqueous solutions is still an obstacle to their application. Although freezing at low temperature has been solved to some extent, the conductivity will inevitably decrease at low temperature.71 The inferior mechanical properties and lack of self-adhesive properties also limit their application in wearable sensors.78,79 Further research is needed to find a good solution for these problems.
Conductive hydrogels can be classified into electron-type and ion-type based on their conductive media. Each type of hydrogel offers certain advantages and should be selected according to the specific application requirements when designing conductive hydrogels. However, it is important to note that different types of conductive hydrogel also have certain limitations, which should be taken into consideration during their usage in wearable sensors. Table 1 summarizes the conductive mechanism, advantages, and disadvantages of different types of hydrogel.
Table 1 Summary of different types of conductive hydrogels
Type |
Conduction mechanism |
Advantages |
Disadvantages |
Electron-type |
Carbon nanocomposite-based conductive hydrogels |
Conductivity achieved by adding CNTs or graphene as conductive fillers to form delocalized π bonds |
(1) Large surface area; (2) excellent mechanical properties; (3) high electrical conductivity |
(1) Aggregation issues, not easy to disperse evenly in hydrogel; (2) severe interface effect |
Metal nanoparticle-based conductive hydrogels |
Achieve conductivity by adding metal nanoparticles with freely moving electrons as conductive fillers |
(1) Excellent mechanical properties and durability; (2) excellent bactericidal effect |
Conductive polymer-based hydrogels |
Conductivity realized through polymers containing π systems |
(1) The conductive material itself is flexible; (2) no organic–inorganic interface effect, which has little effect on the mechanical properties of the hydrogel |
Most conductive polymers are rigid and need to be copolymerized with flexible monomers |
Ion-type |
Achieve enhanced conductivity by forming ion channels and ensuring a sufficient number of free ions |
(1) Transparent color, suitable for photoelectronics applications; (2) sensitive to temperature |
(1) Water evaporation problem; (2) the performance is greatly affected by temperature |
2.2 Properties of the conductive hydrogels
Recent progress in the field of 3D printed conductive hydrogels has shown great potential for developing wearable sensors with enhanced properties. When applying conductive hydrogels to wearable sensors, the key properties of interest include conductivity, mechanical properties, self-adhesiveness, self-healing, and 3D printability. Conductivity serves as the foundation for hydrogel-based sensing, and its quality directly influences the sensitivity of the hydrogel. Hence, achieving excellent conductivity is crucial for hydrogel performance. We have provided a detailed explanation of conductivity in Section 2.1, and will not elaborate on it here. The mechanical properties are foundational for the applicability of hydrogels in the fabrication of wearable sensors. Among the mechanical properties, stretchability and toughness have more significant effects on the overall performance of sensors across various aspects. During the application process, failure to achieve a close contact between the hydrogel and the monitoring surface, or damage to the hydrogel itself, can lead to errors in the data generated by the wearable device. Consequently, the accuracy of the wearable sensor is compromised. Thus, the presence of self-adhesive properties enabling adherence to the detection surface, as well as self-healing capabilities for structural damage, holds great significance in enhancing the accuracy of wearable sensors. Meanwhile, for the prepared hydrogels processed by 3D printing technology for wearable sensors, it is also necessary to pay attention to the fluidity and printability. Although the current research has made great breakthroughs in these aspects, there are still challenges when the above properties are considered comprehensively.
2.2.1 Mechanical performance.
In the context of wearable sensor applications, the mechanical performance of conductive hydrogels is a crucial factor to consider. Two important mechanical properties to evaluate are toughness and stretchability, as they directly impact the functionality and durability of these materials in wearable devices.80
Toughness refers to the ability of a material to withstand mechanical stress without fracture or failure. For wearable sensors, high toughness is desirable to ensure reliable performance during daily activities. Conductive hydrogels with good toughness can maintain their structural integrity even under cyclically stretching or bending conditions. This allows for a continuous and seamless monitoring of physiological signals without compromising the sensor's electrical conductivity.81 Additionally, a tough conductive hydrogel can resist wear and tear, increasing the lifespan of the wearable device.
Alongside toughness, stretchability plays a vital role in wearable sensor applications. Wearable devices often need to transform to irregular shapes and resist various stretches to conform with the body's movements. Therefore, conductive hydrogels should possess sufficient stretchability to accommodate these deformations without losing their electrical conductivity. High stretchability enables comfortable and unrestricted wear, ensuring reliable data collection from the sensors.82,83
To enhance the mechanical properties of conductive hydrogels, one commonly employed approach is to incorporate additional constituents into the hydrogel matrix. This strategy enables the formation of diverse reversible dynamic bonds with the hydrogel matrix, thereby enhancing its stretchability and toughness.30,84 For instance, Xu et al.85 introduced sodium casein (SC) and polydopamine (PDA) into a polyacrylamide system. The dynamic crosslinking facilitated by SC and PDA within the system resulted in the development of hydrogels with exceptional stretchability and fatigue resistance. These hydrogels demonstrated a failure stress of 170 kPa and a failure strain exceeding 2100%, thus showcasing their potential as wearable sensors for the direct monitoring of substantial human body movements, as well as capturing minute physiological signals like speech and breathing.
2.2.2 Self-healing ability.
Self-healing performance refers to the ability of the structures and properties of the materials to completely recover to the initial state before the destruction.86,87 Self-healing properties of conductive hydrogels are necessary when considering the extension of operating life, especially for the sophisticated, fracture-prone structures fabricated by 3D printing technology. The self-healing properties of conductive hydrogels mainly depend on reversible covalent bonds (acylhydrazone bonds, disulfide bonds, Diels–Alder reactions and urea bonds), or reversible non-covalent bonds (hydrogen-bonding, hydrophobic interactions, guest–host interactions, metal–ligand, and polymer–ions).88 Zhang et al.89 synthesized a poly(dopamine methacrylate-co-methacrylatoethyl trimethyl ammonium chloride-co-acrylic) (PDDA) hydrogel. Depending on the internal hydrogen bonds, electrostatic action and other reversible non-covalent bond effects, good self-healing performance was achieved, so it can be restored to its original shape in two minutes without the need for external stimulation. Fig. 6 shows the characterization of the hydrogels before and after self-healing. It should be noted that there is still a 20% difference between the initial state and the self-healing state even if we extended the time to 5 min (Fig. 6(e)). How to achieve a higher self-healing efficiency in a short time should be the focus of future studies. As for the reversible covalent bonds, Jia et al.90 synthesized a new type of polyurethane (PU) hydrogel. By embedding disulfide bonds into the hydrogel polymer chains, the hydrogel can be healed quickly by utilizing the tapping and platform interaction of the disulfide bonds. A good conductivity has also been demonstrated for the healed hydrogel, which reveals its great application potential.
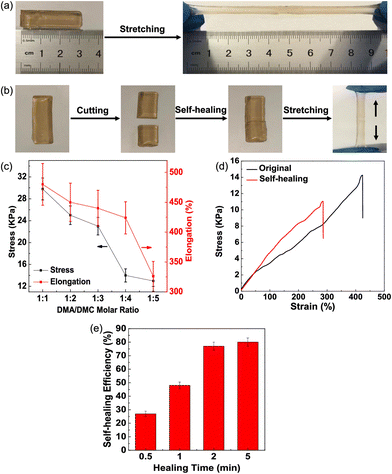 |
| Fig. 6 Self-healing performance testing. (a) Stretchability of PDDA hydrogel. (b) Self-healing behavior of PDDA hydrogel after shearing. (c) Stress and strain of PDDA hydrogels. (d) Stress–strain curves before and after self-healing of PDDA hydrogel. (e) Self-healing efficiency at different times.89 | |
For wearable sensors, changes in electrical signals such as resistance and capacitance are generally generated by structural changes. Therefore, the structure of sensors is very important for the accuracy of detection data. The self-healing performance can ensure the rapid repair of a damaged structure and the great extension of operating life. At present, many techniques based on reversible covalent bonding and non-covalent bonding have been proposed for preparing conductive hydrogels with self-healing properties. Covalent bonds, which are more rigid than non-covalent bonds, will cause certain damage to the flexibility.91 Considering the flexibility requirement of conductive hydrogels, comprehensive consideration should be taken when choosing the right solutions.
2.2.3 Self-adhesive ability.
In order to realize real-time health monitoring of the human body, good contact between sensors and human skin tissue is necessary. However, such a function is difficult to achieve with traditional sensors made of metal and semiconductor materials. Because of the excellent flexibility, wearable sensors have greater advantages over traditional sensors to achieve a good fit with the body.92,93 However, these flexible wearable sensors often require extra tape or adhesives to realize the adhesiveness to human skin. On the one hand, this will decrease the accuracy of monitored signals; on the other hand, some adhesives are toxic, which are not suitable for long-term contact with the human body.94 Therefore, self-adhesive ability is highly expected for wearable sensors. The self-adhesive property of conductive hydrogels can be realized through the chemical or physical interactions between the hydrogels and the substrate surfaces, which mainly include irreversible permanent covalent bonds and reversible weak bonds.95 Tannic acid, as a kind of polyphenol compound, contains many functional groups such as carbonyl groups, phenolic functional groups and hydroxyl groups, which can interact with various organic and inorganic substrate surfaces through hydrogen bonding, electrostatic interaction, coordination bonds and hydrophobic interaction.91,96 Therefore, tannic acid is often used to prepare self-adhesive conductive hydrogels. For example, Fan et al.97 prepared a dual cross-linked (DC) hydrogel with excellent self-adhesive ability (60–80 kPa) by immersing the prepared polyvinyl alcohol (PVA) aerogel in tannic acid. Fig. 7 specifically describes the hydrogel (TEDI) prepared by the dynamic reversible bonding of tannic acid, which exhibits a strong self-adhesive force as high as 50 kPa in adhesion to the skin.
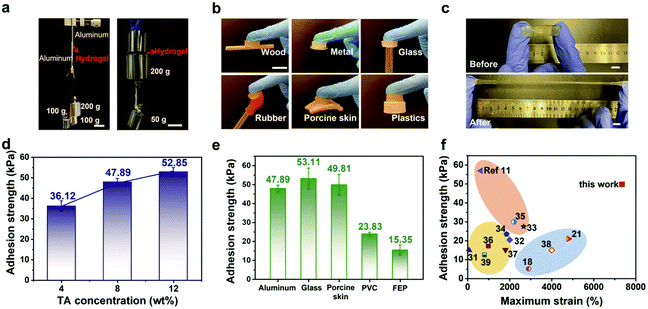 |
| Fig. 7 Self-adhesive test of TEDI hydrogel. (a) Schematic diagram of the self-adhesive nature of TEDI that can lift weights of 200 and 400 g. (b) Schematic diagram of the adhesion ability of TEDI hydrogel to different substrates. (c) Schematic diagram of TEDI hydrogel adhesion to nitrile gloves. (d) Adhesion strength of TEDI hydrogel to Al substrate with different TA concentrations. (e) Bonding strength test results of TEDI hydrogels with different substrates. (f) Comparison of this work with previously reported self-adhesive hydrogels at maximum strain and adhesion strength.91 | |
2.2.4 Printability.
Research studies have shown that the sensitivity of wearable sensors is related to the precision of their structure. 3D printing technology, which can achieve the manufacture of complex structures at the micron-to-nano level through computer digital control, is widely used in the preparation of high-performance sensors.98 Therefore, to achieve good integration between 3D printing technology and conductive hydrogel-based wearable sensors, the printability must be considered. According to the mechanism of 3D printing, the printing materials should usually have good fluidity. On the other hand, to ensure the precision of the structure, materials in a liquid state also need to be cured quickly after printing.99 Hydrogel materials, which are transformed from a liquid to a solid structure with a three-dimensional network through polymerization crosslinking, can easily meet the material properties required by 3D printing technology. In order to achieve good 3D printability of hydrogels, the current research mainly focuses on the following three aspects:100–102 (1) hydrogel materials have obvious pseudoplasticity; (2) hydrogel materials have temperature reversibility; and (3) hydrogel materials can be cured in a very short time. When the hydrogel meets one of the above three points, it can achieve good fluidity in the printing process and fast curing after extrusion. For example, by taking advantage of the property that the viscosity of hydrogels decreases with the increase of shear stress, Deng et al.103 increased the pressure during injection to improve the fluidity of the hydrogel to realize the good printability of the hydrogel. After extrusion, the viscosity of the hydrogel gradually increased until it reached the solid state as the shear stress disappeared. Wei et al.104 mixed the crosslinking agent and monomer in the process of conveying printing materials, realizing partial curing in the printing process to ensure sufficient fluidity and mechanical properties, and finally complete curing was achieved under the condition of UV light at 60 °C.
3. Different wearable sensors based on conductive hydrogels
With the growing emphasis on health and advancements in technology, traditional sensing materials are unable to meet the requirements of long-term and accurate sensing. In recent years, flexible wearable sensors have gained significant attention owing to their exceptional stretchability. Hydrogel, as a hydrophilic flexible material that closely resembles human tissue structure, is an excellent candidate for developing wearable sensors.105 Currently, wearable sensors can be categorized into four distinct categories based on their respective transduction mechanisms: (1) piezoresistive sensors; (2) capacitive sensors; (3) triboelectric sensors; and (4) piezoelectric sensors. Fig. 8 briefly illustrates the working principles of these sensors, while the specific sensing mechanisms and the applications of conducting hydrogels in these sensors are discussed in details in the following part.
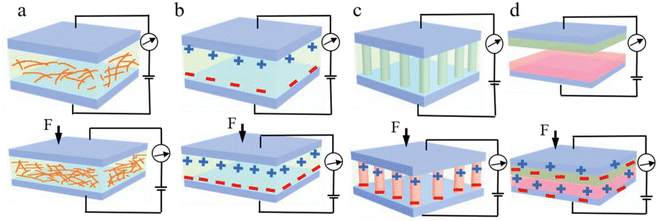 |
| Fig. 8 Schematic illustrations of the four transduction mechanisms. (a) Piezoresistive. (b) Capacitive. (c) Piezoelectric. (d) Triboelectric.106 | |
3.1 Piezoresistive wearable sensors
Piezoresistive sensors refer to the sensors that can detect external pressure signals by converting the pressure to an electrical signal.107 Piezoresistive has become the most widely used sensing mechanism because of the simple preparation process and high pressure sensitivity for the associated sensors.108 In recent years, more and more conductive hydrogel sensors based on piezoresistive sensing mechanisms have been fabricated. The following summarizes the current research, focusing on the transduction mechanism and sensor performance.
According to the definition of resistance R = ρ × (L/A) (R, ρ, L, A represent resistance, resistivity of the conductive material, length of the conductive material, and cross-sectional area of the conductive material, respectively), it can be known that the resistance of conductive materials increases with the increase of resistivity and length, while it decreases with the increase of cross-sectional area.106,109,110 When integrated with conductive hydrogels which achieve conductivity through the addition of either conductive nanocomposites, conductive polymers, or conductive solution ions, high-performance hydrogel-based piezoresistive sensors can be facilely achieved.
For the nanocomposite type, the internal structures of the hydrogels will be deformed under the action of pressure, and the internal conductive nanocomposites will probably come into contact with each other, forming a new electron transmission path, resulting in a change of resistance. In general, with the increase of pressure, the probability of contact between conductive media increases, which causes the decrease of the resistance.111–113 Sang-Ha Hwang et al.114 prepared GO conducting hydrogels with different porosities, tailoring the microstructure of the hydrogels by controlling the proportion composition of the GO and the hydrogel matrix. Fig. 9(a) shows the change of electrical resistance of hydrogels with different GO concentrations at different strain rates. The conductive pathways are formed through the crosslinking of graphene. When 5 wt% ethylenediamine (EDA) was added to GO aqueous solution, the prepared hydrogel presented good piezoresistive sensitivity and a linear signal at 6.8% strain. They also modified the hydrogel with nanoplatinum, which increased the linear range of piezoresistivity to 52.8% due to the interaction between the platinum and graphene. The changes of compressive stress and resistance of GO hydrogels modified with different substances under compressive strain are shown in Fig. 9(b–d), which demonstrated the application potential of the well-defined porous functional nanomaterials as highly stable, sensitive piezoresistive sensors.
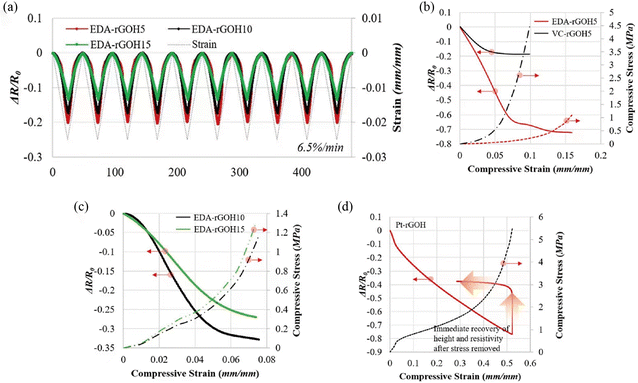 |
| Fig. 9 Piezoresistive properties of graphene oxide hydrogel (GOH) under different modification conditions. (a) Cyclic compressive strain-induced resistance changes of different concentrations of GOH at strain frequencies of 6.5% min−1. Piezoresistive behavior and s–s curve of (b) EDA-rGOH5 and VC-rGOH5, (c) EDA-rGOH10 and EDA-rGOH15, and (d) Pt-rGOH under static compressive strain with a strain rate of 6.5 cm min−1.114 | |
Regarding the conductive polymer and ionic conductive hydrogels, changes in resistance values are primarily caused by alterations to the internal pore structure of the hydrogels due to external pressure.111 When a conductive polymer hydrogel is compressed, contact between the pores can occur, which modifies the electron conduction pathway, leading to changes in electrical resistance. Similarly, for ionic hydrogels which rely on the free movement of ions, transport pathways may also change under the action of pressure due to the evolution of pores. For example, Shen et al.56 prepared a double-crosslinked ionic hydrogel with a uniformly distributed porous structure using lignocellulose. Sensitivity tests, generally represented by the slope of the ΔR/R0–ε curve with the value calculated as GF = (ΔR/R0)/ε, indicated that the prepared hydrogels demonstrated excellent sensitivity (up to 9.34). They were capable of distinguishing between light pressure and hard taps in a broad range.
It is evident that the microporous structure plays a crucial role in enhancing the sensitivity of piezoresistive wearable sensors. The existence of micropores can realize a large structural change of a hydrogel under a small pressure, resulting in a significant change in the resistance value. In this regard, 3D printing technology offers a significant advantage in the design and construction of micropores. Therefore, when preparing piezoresistive wearable sensors, attention should also be paid to the rheological properties of conductive hydrogels to make them suitable for 3D printing.
3.2 Capacitive wearable sensors
Capacitive sensors usually consist of two parallel conducting electrodes and a dielectric layer in between. Capacitance is defined as C = εA/d, where ε, A, d represent the dielectric permittivity of the dielectric layer, the overlapping area between two parallel electrodes, and the distance between two electrodes, respectively.115,116 According to the definition, the capacitance C is determined by three variables. However, when designing the sensors, the most commonly used variables are the distance d between the two electrodes and the dielectric permittivity ε. Specifically, when an external pressure is applied to the sensor, the distance between the electrodes will get reduced, leading to an observable electrical signal indicating increased capacitance. Additionally, the dielectric permittivity of the specific dielectric layer would also change in response to pressure, producing a change in capacitance.117
It can be observed that the sensing performance of capacitive sensors is highly dependent on the deformation capacity of the dielectric layer and the conductivity of the two electrodes.118 To ensure the good deformability of the dielectric layer, materials possessing excellent stretchable properties are typically employed in developing capacitive sensors. As for the material selection of the two electrodes, nevertheless, traditional electrodes usually adopt rigid materials such as metals and semiconductors. When subjected to external forces, the significant difference in Young's modulus between the dielectric layer and the electrode often results in an interface disconnection, altering the structure of the capacitive sensor and affecting its sensing and detection values.119
As a flexible material, conductive hydrogels can attain electrical conductivity by either adding a conductive medium, using a conductive polymer or adopting an ionic solution offering the conductive pathways, thus satisfying the requirements of electrode materials for capacitive sensors. This will not only ensure the conductivity of the electrode itself but also allow for excellent structural combination with the dielectric layer, reducing the effect of the interface on the mechanical properties. Moreover, since a hydrogel is a polymer material containing diverse functional groups, it can be easily modified to achieve desirable self-adhesive, self-healing, and other properties according to the design requirements. As a result, constructing capacitive sensors using conductive hydrogels facilitates the wearable performance and provides the possibility for the long-term and convenient monitoring of human health.
For example, Xu et al.120 prepared a capacitive pressure sensor with super stretchability and high sensitivity by taking an ionic hydrogel as the matrix and adding silver nanofibers inside. The ionic hydrogel of the sensor interacts with the silver nanofibers at the interface, forming the nanometer-thick Debye–Helmholtz electrical-double layer, where electrons in the metal and ions in the hydrogel (opposite polarity to the electrons) accumulate within a few nanometers. This structure results in a large interface capacitance, improving the sensitivity of the capacitive sensors by three orders of magnitude. Fig. 10 shows the specific sensing applications and effects of the capacitive hydrogel. The sensor can also achieve fast response time, with a releasing-response time of 320 ms and a stretching-response time of 130 ms, which endows the ability to detect most physiological parameters (typically at the level of seconds). Moreover, by adding cationic cellulose nanocrystals to the monomer solution of acrylic acid, Lai et al.121 successfully produced a hydrogel ink with good fluidity and 3D printability, based on which the capacitive sensors with high sensing performance were designed. After printing, the model can be cured by photopolymerization and immersion in AlCl3 solution to obtain a tough and ionic conductivity hydrogel material. This method can well combine hydrogel materials with 3D printing, which has a good advantage for designing the structure of a capacitive wearable sensor with enhanced performance.
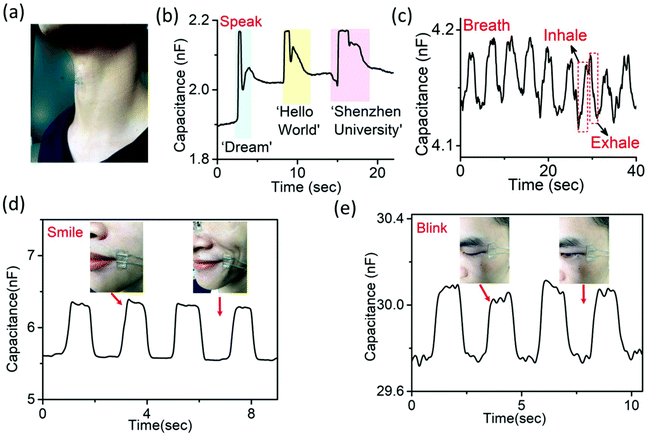 |
| Fig. 10 Applications of capacitive pressure sensors. (a) Schematic diagram of hydrogel sticking near the throat to monitor breathing, speech, etc. (b) Response curves when the volunteer spoke “Dream”, “Hello World” and “Shenzhen University”, respectively. (c) Capacitive response when the volunteer did breathing actions. (d) Capacitive response of the sensor attached to near the mouth when the volunteer was laughing. (e) Capacitive response of the sensor attached to the canthus when the volunteer was closing and opening the eyes.120 | |
Piezoresistive sensors rely on resistance change to monitor external force. However, environmental temperature and humidity have a significant influence on the material's resistance, leading to difficulty in achieving a single-variable influence during monitoring.119 For capacitive sensors, the sensing ability is realized through the change of the distance d between the electrodes and the change of dielectric permittivity ε. For the two variables, the change of distance d is the main influencing factor, and the dielectric permittivity is less affected by the external environment than the resistance.122 Thus, capacitive sensors are less susceptible to the effects of temperature and humidity, making them more stable in the monitoring process and yielding more accurate test results. Also, capacitive sensors have minimal hysteresis, which enables real-time monitoring and are better suited for long-term data observation.115,123
3.3 Tribo/piezoelectric wearable sensors
It is a common sensing mechanism for wearable sensors to realize the conversion of pressure/strain to electrical signals by using changes in resistance and capacitance. After being developed for decades, these two types of sensor can meet the needs of human health monitoring in terms of sensitivity, detection range and linearity through careful designs. Nonetheless, these sensors share a common limitation – the need for an external power supply to achieve the conversion of the electrical signals. As a result, the development of wearable devices without a dependence on electricity generation is highly desirable.124 The dependence on an external power supply not only increases the complexity of the device but also poses a significant challenge in designing the flexible sensing part integrated with the power supply equipment. To address the need for a self-power supply, recent research has shifted towards triboelectric and piezoelectric sensors.125 The self-powering mechanism of these sensors depends mainly on the creation of a nanogenerator,126 which converts small changes in mechanical energy and thermal energy into electrical energy.127,128 In the following sections, we will introduce the working mechanisms of the triboelectric nanogenerator (TENG) and piezoelectric nanogenerator (PENG), and their applications in sensing.
The triboelectric nanogenerator relies on the coupling between triboelectric and electrostatic induction to achieve self-power.129 Friction is generated between two materials with large electronegativity, leading to an equal charge of opposite electric property. The friction material is then connected to two electrodes, forming an electric potential through electrostatic induction to realize a self-power supply.130,131 Triboelectric nanogenerators can be categorized into four main types based on their electrode and operation mode: vertical contact–separation mode, contact-sliding mode, single-electrode mode, and freestanding triboelectric-layer mode.132 In the applications of sensors, the most commonly used mode is the vertical contact–separation mode, in which two electrodes adhere to the back of the two friction materials.126,133 When the two friction materials come into contact, the surface is charged, and the opposite electric charges repel each other, creating a gap between the two friction materials. Through electrostatic interaction, the two electrodes form an induced potential difference. When the external force is applied to the sensor's surface, the gap between the two friction materials changes, causing the induced potential difference to change, ultimately resulting in force sensing.
Since most materials can be charged by friction, there are many materials to choose from when designing triboelectric sensors, such as conducting polymers, metal oxide semiconductors, etc.134 Moreover, in recent years, there have been research studies on triboelectric sensors based on conductive hydrogels.135–138 For example, Luo et al.139 developed a wearable sensor based on the principles of triboelectric nanogenerators using PVA/P(AM-co-AA)–Fe3+. This sensor demonstrated excellent self-power supply with a maximum open-circuit voltage of 238 V and a short circuit current of 1.2 μA. Fig. 11 illustrates the specific performance of the hydrogel in terms of its triboelectric ability. Furthermore, this design effectively combines the superior self-adhesive ability and mechanical properties of hydrogels, which holds great potential for sensing applications.
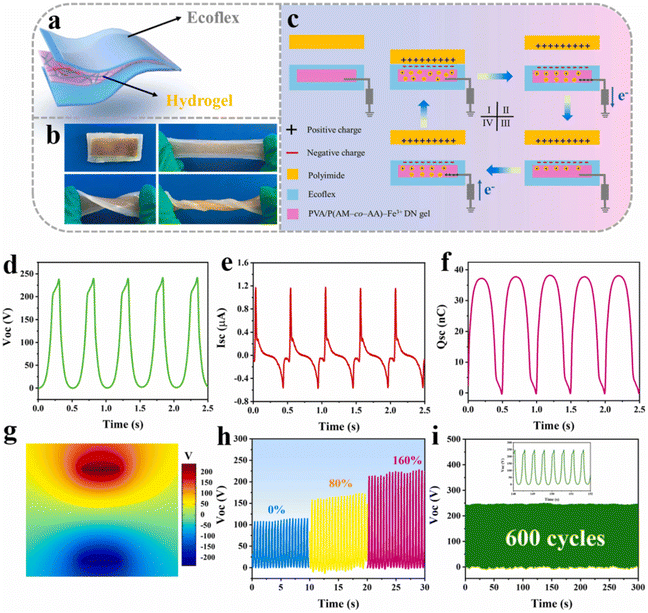 |
| Fig. 11 (a) Structure of PP-TENG. (b) Images of PP-TENG under the different states. (c) Illustration of the power generation mechanism. (d) VOC, (e) ISC, and (f) QSC of the PP-TENG. (g) Finite element analysis of PP-TENG output voltage. (h) Open-circuit voltage of the stretchable PP-TENG at different strains. (i) Stability of the PP-TENG under 600 cycles.139 | |
Piezoelectric nanogenerators are based on the piezoelectric characteristics of piezoelectric materials. Under the condition of pressure, a polarized charge is generated inside the material.140,141 When the external pressure increases, the density of the polarized charge also increases accordingly. By connecting an external load, a current is formed between the electrodes. The resulting current change is directly related to the applied pressure, thus enabling sensing functionality. However, the selection of suitable piezoelectric materials is limited primarily to ceramics and crystals,142 which constrains the use of piezoelectric sensors. Currently, there is little research on the combination of conductive hydrogels with piezoelectric sensors, which reveals that further exploration is needed in this field.
In general, wearable sensors play a crucial role in applications in biomedical areas, particularly in the realm of human health monitoring. Based on the transduction mechanisms, they can be categorized into four types. Firstly, there are piezoresistive sensors that employ conductive hydrogels as sensing layers. When subjected to external forces or pressure, the hydrogel's resistance changes. These sensors can be embedded in elastic bands to monitor muscle activity, respiratory rhythms, and more. Capacitive sensors utilize the variations in the capacitance of conductive hydrogels to detect changes in external physical quantities. They find applications in areas such as heart rate monitoring and gesture recognition, where they can be integrated into wristbands or gloves for real-time monitoring of heart rate and device control. Furthermore, triboelectric/piezoelectric sensors leverage the triboelectric effect and piezoelectric effect of hydrogels. They can spontaneously generate charges and monitor pressure by measuring charge fluctuations. For instance, when embedded in shoe soles, they can convert the motion of walking or running into electrical energy to power mobile devices. The triboelectric/piezoelectric principle offers the advantage of self-generating charge without the need for an external power source, presenting significant potential for future applications. Consequently, further in-depth research is expected for wearable sensors based on conductive hydrogel materials utilizing the triboelectric/piezoelectric sensing mechanism.
4. 3D printing of conductive hydrogel-based wearable sensors
In recent years, wearable sensors have attracted considerable attention in various application fields. Notably, research on conductive hydrogel-based wearable sensors has gained momentum, with related transduction theories becoming increasingly comprehensive.143 Consequently, the application range of flexible sensors has further expanded. However, the rapid development of wearable sensors poses several challenges to sensor fabrication technology. Traditional methods such as templating-based techniques suffer from high production costs and a lack of customizability. Furthermore, these techniques generate significant waste during processing, which is not environmentally friendly. The main drawback of traditional production methods is their limited accuracy, which fails to meet current sensing requirements as per design standards.144–146 3D printing, also known as additive manufacturing, is a computer-aided process that builds models layer by layer.147 This technology could not only reduce material waste, but also achieve high precision in constructing models through computer control.148 Considering the advantages of conductive hydrogels and 3D printing in preparing wearable sensors, an increasing number of researchers focus on combining these two to produce sensor devices with enhanced sensing performance.149 However, since hydrogels contain significant amounts of water, a more delicate printing environment is necessary when using them as printing material. This review focuses primarily on the available 3D printing technology that employs conductive hydrogels as printing material. Based on different working principles, 3D printing technology using hydrogels can be classified into laser-based, extrusion-based, and inkjet printing.150,151 The following section will discuss the working principle of each 3D printing technique in the application of conductive hydrogel-derived wearable sensors, as well as their respective advantages and disadvantages.
4.1 Laser-based 3D printing
Laser-based 3D printing refers to the printing technology that uses laser beams to trigger printing ink polymerization (excluding the laser-based technology that uses the energy of a laser beam to melt solid printing materials) to realize the transformation from liquid state to solid state, so as to complete the construction of printing models.152 The most representative method is stereolithography technology, which is the earliest printing method put into industrial application. Based on stereolithography technology, the subsequent development of laser-based 3D printing technology mainly focuses on changing the light source and imaging system.153 Therefore, stereolithography is introduced here as a representative of this type of printing method.
Stereolithography, or SLA technology, digitally controls the movement of a light beam placed over the tank containing a liquid polymer precursor, which transforms from liquid to solid state in two dimensions by photoinduced polymerization, and the whole model is completed layer by layer.154,155 The traditional method of photocuring hydrogels is to place a precursor solution containing a photoinitiator in a chamber equipped with an ultraviolet lamp. Due to the similarity in principle between SLA technology and traditional photocuring, more and more attention has been paid to the differences between hydrogels obtained by the two methods. For instance, Burke et al.156 used polyethylene dimethacrylate (PEGDMA) as the research object to compare and discuss the performance of hydrogels made by the two methods. Results have shown that the hydrogels are basically similar in thermal and chemical properties. Moreover, as the precursor solution of SLA technology is exposed to more ultraviolet light than traditional methods, it is superior to traditional methods in tensile strength and hydrophilicity. This study revealed the superiority of SLA in hydrogel preparation, and also indirectly demonstrated the feasibility of combining a conductive hydrogel with SLA technology. One of the advantages of SLA technology is that it can achieve a high resolution. Taking advantage of this feature, Wu et al.157 successfully achieved the printing of micrometer-scale hexagonal structures by combining the micro-scale SLA technology implemented using a digital micromirror projection array with GelMA–PANI conductive hydrogel (Fig. 12). Odent et al.158 synthesized a class of highly adjustable ionic conductive hydrogel materials with good 3D printability by using the reversible dynamic interaction between ions, and successfully employed SLA technology to prepare models of various complex structures, such as the Eiffel Tower and cubic lattices, offering promise for use in the sensing and control of autonomous soft robots.
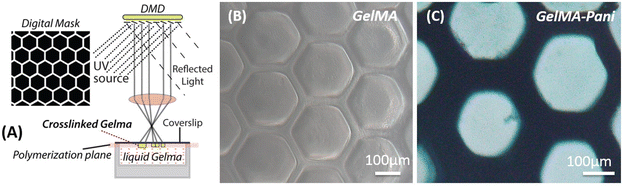 |
| Fig. 12 SLA 3D printing of micrometer-scale hexagon structures. (A) Schematic of microstereolithography: using a computer-aided design (CAD) based hexagon pattern to digitally adjust UV light, for the selective polymerization of hydrogels. (B and C) Brightfield images of GelMA and GelMA–PANI with hexagonal geometry.157 | |
Odent et al.159 reported an engineering strategy for ionic electronic touch sensors based on high-resolution SLA 3D printing, which was used to design a 3D-printed stacked ionic module composed of two-compartment systems with different ion types, charge densities and cross-linking densities. The ionic electronic touch sensor exhibited sensitivity for touch-pressure monitoring and locatable recognition during application, providing a technical reference for designing other pressure sensors with a high sensitivity and wide detection range. Most hydrogels prepared by extrusion-based 3D printing are limited by printing efficiency and resolution. Wu et al.160 proposed a simple strategy to rapidly manufacture hydrogels using SLA 3D printing technology. Owing to the advantages of SLA 3D printing, the hydrogels with complex structures suitable for human body parts could be customized for flexible sensors. The printed finger-cot, knuckle, and manipulator were shown in Fig. 13(a)–(c). Sensors based on three different structures were used for real-time monitoring of finger bending, which could clearly distinguish different bending angles. Therefore, SLA 3D printing technology can be used to print customized flexible sensors suitable for the human body.
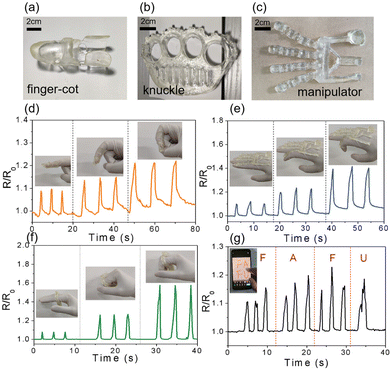 |
| Fig. 13 High-fidelity hydrogel-based flexible sensors via 3D printing: (a) finger-cot, (b) knuckle, and (c) manipulator. The relative resistance change of (d) finger-cot, (f) knuckle, and (e) manipulator flexible sensors under different angles of finger bending. (g) Handwriting function and relative resistance change of the finger-cot sensor.160 | |
SLA technology is mainly affected by printing materials and printing parameters. The most basic requirement for a printed material is that it can be cured under light.161 The conductive fillers added would influence the curability and laser absorption of the matrix resin, which should receive great attention when preparing the conductive hydrogels. Meanwhile, studies show that the solid model formed by photopolymerization will shrink in volume, which will affect the precise structure of the printed model, and even destroy the structure of the whole model in serious cases.162 The setting parameters of the beam are closely related to the printing process: the size of the beam directly determines the precision of the printing structure, and the moving speed of the beam is related to the printing speed. To enhance the printing accuracy of laser-based 3D printing technology, a technique called two-photon polymerization has been proposed. Two-photon polymerization (TPP) is an advanced 3D printing technology that utilizes lasers to achieve high-resolution manufacturing of micro- and nano-scale objects. The key to two-photon polymerization lies in the two-photon absorption effect, where the energies of two photons are combined when they are simultaneously absorbed, resulting in higher energy levels sufficient to excite electrons. By controlling the position and intensity of the light beam, the two-photon technique enables the printing of three-dimensional structures with higher precision. However, TPP technology also has some limitations, such as higher equipment costs and relatively slower printing speeds.147,163
4.2 Digital light processing
In addition to the laser-based 3D printing technology mentioned earlier, there is another photopolymerization-based technique called digital light processing (DLP) frequently used in the manufacturing of hydrogels. DLP is a rapid prototyping technique based on the principle of photopolymerization. It utilizes a digital projector to convert the light emitted from a light source into a two-dimensional image through a micromirror array, and then controls the brightness and duration of the light source to solidify the photopolymer material, thereby achieving the layer-by-layer stacking of a three-dimensional object.164,165 Compared with traditional SLA technology, DLP technology has some differences. Firstly, DLP uses a digital projector for light source control, while SLA uses a laser beam. Secondly, DLP can solidify the entire layer simultaneously, whereas SLA requires point-by-point scanning and solidification. This enables DLP to have a faster printing speed.166,167 Therefore, DLP printers typically have a higher resolution and can achieve finer printing. Due to its similar basic principles to SLA technology, this technology can also be well combined with photocurable hydrogels.168,169 By combining a conductive hydrogel with DLP 3D printing technology, it is possible to achieve the rapid fabrication of complex-shaped conductive structures, providing new possibilities for the preparation of flexible sensors, biosensors and other applications. For example, Guo et al.170 utilized DLP technology to fabricate a biodegradable poly(ACMO)/Pt hydrogel sensor with electronic conductivity. With the high resolution of DLP technology, this sensor exhibited an ultra-high gauge factor (GF) ranging from 1.5 to 7.2 within a strain range of 10–100%, while maintaining good cyclic stability, making it suitable for monitoring a variety of human activities.
4.3 Extrusion-based 3D printing
According to the working principle, extrusion-based 3D printing can be divided into fused deposition modeling (FDM) and direct ink writing (DIW).171,172 FDM is the process of melting wire through a high-temperature nozzle, so that it has fluidity to achieve printability.173 Direct writing is dominated by an external mechanical energy, using the shear-thinning and thixotropic properties of printing materials to achieve printability of materials.174 It can be seen from the working principle that the high temperature heating environment of FDM is not suitable for hydrogel printing. As a kind of polymer material, most hydrogels have pseudoplasticity, which well meets the material requirements of DIW technology. Therefore, DIW technology is mainly introduced in the following.
The direct ink writing 3D printing method uses external pressure to extrude the printing ink from the nozzle, and realizes the printing of each layer through the movement of the nozzle controlled by the computer.175,176 According to the working mechanism, the main requirements of direct ink writing printing materials are good rheological properties and thixotropic properties.177,178 In order to realize the rapid transition between the fluidity in the printing process and the timely curing after printing, there are generally two kinds of processing for DIW materials. One is based on the efficient thixotropic conversion of the material itself; that is, when under external pressure, the ink's viscosity is reduced and fluidity is enhanced, because of which the ink can be successfully extruded from the nozzle. After the pressure is removed, the ink can quickly restore to the original low flow state, achieving printing molding.179,180 The other is that the printing ink itself has good fluidity. After printing, in order to enhance the robustness of the model and prevent deformation caused by flow, further curing crosslinking will be carried out in different ways, such as photocuring, thermal-curing and pH response.181,182 Compared with the first one, the thixotropy requirement of the second method is not so high. However, initiators related to crosslinking conditions need to be added.
For example, Highley et al.183 prepared a supramolecular hydrogel with self-repairing properties based on modified hyaluronic acid (HA). The hydrogel has good shear thinning properties, which is mainly induced by the existence of reversible guest–host non-covalent bonds. The guest–host crosslinking can break quickly to enhance fluidity when subjected to external pressure, and can be quickly rebuilt after pressure removal, which makes the hydrogel very suitable for direct writing 3D printing technology. This type belongs to the first method described above. For the second method, Jiang et al.184 used polyvinyl alcohol (PVA)/κ-carrageenan glue hydrogel with good fluidity as DIW printing ink, and induced PVA to form a cross-linked network by freeze–thaw after printing, thus achieving the construction of the printing model and the improvement of the mechanical properties. Fig. 14 shows the specific printing and freeze–thaw processes and the formation of non-covalent bonds within the hydrogel during the process. For another example, Zhang et al.187 prepared an ionic conductive hydrogel material with good fluidity using polyvinyl alcohol (PVA) and polyacrylamide (PAM) as the hydrogel matrix. When the hydrogel is used as printing ink, good fluidity can cause it to be extruded smoothly at room temperature. Then, under the irradiation of ultraviolet light, the cross-linked curing of acrylamide is completed. After curing, the hydrogel material with good conductivity can be obtained by placing it in borax solution, which can be further used for the preparation of wearable sensors.
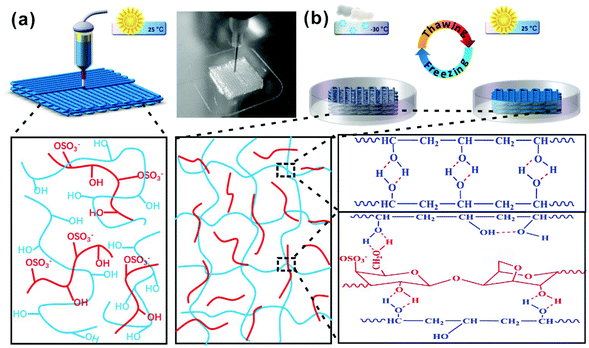 |
| Fig. 14 Hydrogel printing and post-treatment diagram and internal structure changes of hydrogel. (a) DIW 3D printed hydrogel at room temperature. (b) Mechanical properties of hydrogels were enhanced by the freeze–thaw method due to the crystallinity of PVA.184 | |
Although the structural design of hydrogels can be expanded by 3D printing technology, thereby improving the performance of hydrogel-based sensors, how to achieve printability, mechanical toughness, and self-healing capabilities simultaneously remains a big challenge. Lai et al.185 developed a thermal treatment method to uniformly disperse CNCs in DESs for a 3D printable and self-healing hydrogel. The synthesized hydrogel based on DIW 3D printing had high mechanical toughness and self-healing ability. In addition, the sensitivity of the wearable sensor could be improved by printing the hydrogel into a horseshoe structure. The sustainable synthesis process of 3D printable materials is also a major challenge. Vo et al.186 proposed a green, low-cost, and energy-saving strategy to manufacture conductive ink for the 3D printing of wearable devices based on green and low-cost Carbopol and DES. Combined with DIW 3D printing, the ink could manufacture several structures, including auxetic structures, woodpile shapes, and letters. The 3D-printed auxetic sensor had high stretchability (300% strain), high sensitivity (gauge factor (GF) of 3.1), sufficient transparency, and good moisture resistance.
In general, extrusion-based printing can be more advantageous than laser-based printing in selecting materials. However, for printing models with high precision, the extrusion-based method still has a certain technical bottleneck, which needs to be further studied and broken through.
4.4 Inkjet 3D printing
Inkjet 3D printing uses a material with low viscosity as the ink. With the movement of the nozzle, the droplets obtained by external extrusion fall onto the specified position to form the desired print model. Depending on how the droplets are formed, the inkjet 3D printing can be divided into drop-on-demand mode (DOD) and continuous inkjet mode (CIJ).188,189 DOD means that when the nozzle moves to the specified position, the external force is applied to produce a pulse to form a droplet.190 The common forms of external forces mainly include piezoelectricity and heating,191,192 as shown in Fig. 15. CIJ generates droplets continuously during the printing process and the unused droplets are deflected under the action of an applied electric field and enter the recycling device to realize the recycling of subsequent materials.193 Although this method can realize the recycling of materials to a certain extent, the material consumption is larger when compared with the DOD mode, and the need to control the deflection electric field also increases the difficulty of this method. Nonetheless, because of the continuous production of ink, CIJ's printing speed is much faster than the DOD mode.194
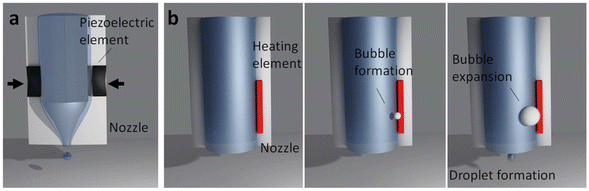 |
| Fig. 15 Drop formation in piezoelectric (a) and thermal (b) inkjet systems.192 | |
Reactive inkjet 3D printing is a technology that sprays one or more reactants onto a substrate containing another reactant and uses physical or chemical reactions between them to form products in situ.195,196 Traditional reactive inkjet 3D printing techniques can be divided into single-reactive inkjet printing and full-reactive inkjet printing, as shown in Fig. 16(a) and (b). Due to the fact that hydrogels often require additional crosslinkers and initiators to complete the transition from liquid to solid state, reactive inkjet printing technology is the most commonly used method when using inkjet printing to manufacture hydrogels. For example, Mei Ying Teo et al.197 took alginate hydrogels as the research object and adopted micro-reactive inkjet printing (MPIJP) technology to generate the desired hydrogels; that is, the reaction occurred through the collision of precursor microdroplets and crosslinking agent microdroplets in the air, which were then deposited onto the printing panel, as shown in Fig. 16(c). Compared with traditional reactive inkjet 3D printing, this method is more convenient and it is easier to achieve a more detailed structure printing. However, the development of suitable crosslinkers and initiators to generate the conductive hydrogel-based structures needs more research effort. In addition, Huang et al.198 reported a simple and novel method to print patterns on the surface of hydrogels using thermal inkjet printheads and aqueous solutions containing calcium ions as inks. The pressure capacitance sensor based on the hydrogel had high compression strength, stable capacitance change and wide pressure applications.
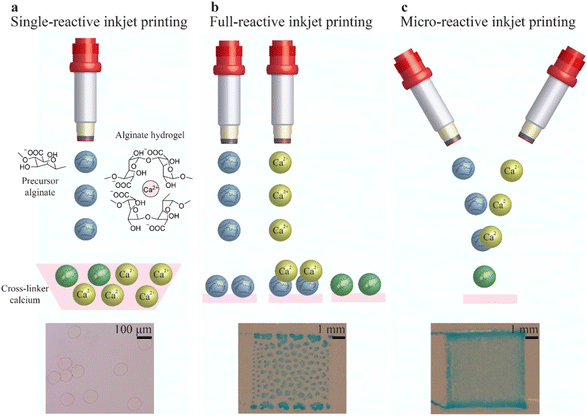 |
| Fig. 16 Illustration of three different reactive inkjet 3D printing strategies. (a) Single-, (b) full-, and (c) microreactive inkjet printing.197 | |
Because the driving pressure of inkjet 3D printing tends to be low, it is only suitable for printing materials with low viscosity. Therefore, regarding applications in conductive hydrogels, inkjet 3D printing has fewer demonstrations than the previous two methods. Further research is needed to address the material selection limitations of this technology.
In summary, 3D printing of conductive hydrogels offers several beneficial features for wearable sensor applications. Firstly, DIW printing technology enables the creation of conductive pathways by directly incorporating conductive materials such as silver or carbon nanotubes into the hydrogel, through controlling the position and speed of the print head. During the 3D printing process, only the flowability and viscosity of the conductive hydrogel need to be considered to construct the structures for wearable sensors. The design flexibility allows hydrogel-based wearable sensors to interact tightly and comfortably with the human body. Secondly, photopolymerization-based printing technology utilizes ultraviolet light to cure the hydrogel precursor, offering higher printing resolution and intricate details by adjusting the beam parameters. This makes it suitable for manufacturing complex sensor structures such as microelectrodes and fine wires. Lastly, inkjet-based printing technology precisely jets conductive hydrogel material to the desired locations. It benefits from high-speed printing and large-scale production capabilities and can be employed to fabricate intricate conductive patterns and sensor arrays. Due to its high level of automation and versatility, inkjet printing enables rapid prototyping and customized production. Particularly noteworthy is the excellent compatibility between reactive inkjet 3D printing and the crosslinking mechanism of hydrogels, allowing for a broad range of applications by integrating conductive hydrogels with 3D printing technology.
Table 2 provides a summary of the three 3D printing technologies applicable to the conductive hydrogels mentioned earlier. However, it is important to note that there are certain limitations when combining 3D printing technology with conductive hydrogels due to the unique structure of hydrogels. As a result, the application of 3D printing in manufacturing conductive hydrogel-based wearable sensors is still at the beginning stage. Here, two research directions are proposed for the integration of these two elements. (1) Enhancing the properties of conductive hydrogels: given the specific structure of hydrogels, they are only suitable for mild 3D printing processes. Therefore, optimization efforts can be made to improve the mechanical strength of hydrogels. Additionally, the fluidity and curing process of hydrogels have consistently been the primary factors influencing the printability of conductive hydrogels. Thus, special attention should be received. (2) Increasing the diversity of 3D printing technology: taking inspiration from reactive inkjet 3D printing, the development of a new 3D printing process can prioritize the polymerization and cross-linking process of hydrogels. This can serve as a starting point for innovative advancements in 3D printing technology.
Table 2 Summary of the 3D printing technologies applicable to conductive hydrogel-derived wearable sensors
Printing method |
Working principle |
Advantages |
Disadvantages |
Suitable materials |
Laser-based 3D printing |
Uses a laser beam to trigger ink polymerization, transforming it from a liquid to solid state layer by layer |
High-resolution printing capability |
Volume shrinkage of printed models |
Photopolymerizable materials |
Extrusion-based 3D printing |
Includes DIW and FDM, of which only DIW is suitable for conductive hydrogels, mainly by applying pressure to squeeze the fluid hydrogel out of the needle |
Simple operation, wide range of application |
Low structural resolution |
DIW: hydrogels with good rheological properties and thixotropic behavior |
Inkjet 3D printing |
Utilizes low-viscosity material as ink and forms droplets onto the desired position |
CIJ offers faster printing speed |
Material consumption is larger in CIJ |
Hydrogel material with high fluidity and low viscosity |
5. Conclusion and perspective
This review mainly discussed the classification and properties of conductive hydrogels, sensing mechanisms and 3D printing technology suitable for conductive hydrogel-based sensors. Conductive hydrogels can be divided into electron-type and ion-type according to the transduction mechanisms. The electron-type relies on freely moving electrons to provide electrical conductivity, which can be specifically divided into carbon nanocomposite-based, metal nanoparticle-based and conductive polymer-based. The ion-type relies on sufficient free ions to form an ionic pathway. Compared with the electron-type, the ion-type has better transparency, which is more suitable for photoelectronics. Meanwhile, ionic hydrogels have more advantages in mechanical properties owing to the filler-free feature. In order to ensure long-term stable monitoring, the mechanical stability and self-adhesive and self-healing performance of conductive hydrogels should be considered. Printability is also important when combined with 3D printing technology to achieve more detailed structures. We have discussed four hydrogel sensing mechanisms, specifically, piezoresistive, capacitive, triboelectric and piezoelectric. Compared with the latter two, the piezoresistive and capacitive types are more widely used in hydrogels. However, the piezoelectric and triboelectric types have more advantages in the preparation of portable small sensors because they can generate electricity spontaneously, which would avoid the use of the external power supply devices. Finally, 3D printing technologies suitable for manufacturing conductive hydrogels have been introduced.
In recent years, there has been extensive research on the fabrication of wearable sensors using 3D printing technology with conductive hydrogel materials. This combination offers numerous advantages, such as precise material customization and the manufacture of complex structures, while reducing production costs and simplifying the operational process. However, there are still several challenges that need to be addressed in this field, and research avenues can be proposed to further drive its development. Firstly, wearable sensors fabricated using 3D printing technology with conductive hydrogel materials currently face limitations in terms of material performance. Although researchers have made progress in areas such as stretchability, self-adhesion, self-healing, conductivity, and printability, achieving the comprehensive development of these multiple properties remains a challenge. For example, the addition of nanofillers to hydrogels leads to interface effects between the fillers and the hydrogel matrix, causing a loss of mechanical strength, which means that the electrical conductivity and mechanical properties of the hydrogels cannot be optimized simultaneously. Secondly, there are also limitations associated with 3D printing technology for the fabrication of conductive hydrogel materials. Due to the characteristics of hydrogels, the choice of available 3D printing technologies is limited. Current 3D printing techniques available include laser-based printing, which requires hydrogel materials to exhibit photocurable properties; extrusion-based printing, which requires hydrogels to possess high shear and thixotropic properties; and inkjet-based printing, which is only suitable for low-viscosity hydrogels. These material requirements restrict the application of 3D printing technology in hydrogels. Furthermore, there are other issues in current research. For example, there is a need to further enhance the sensitivity and stability of conductive hydrogel materials to meet the performance requirements in practical applications. Optimizing the interface between conductive hydrogels and human skin is necessary to improve sensor comfort and wearability. Additionally, addressing the lifespan and stability issues of conductive hydrogel materials is crucial to ensure long-term stable monitoring.
To address these challenges, several research directions can be proposed. (1) Material improvement: further development of different types of conductive hydrogel and exploring novel nanofillers and conductive polymers to overcome the trade-offs in material properties and achieve synergistic optimization of multiple characteristics. (2) Interface optimization: study the interactions between the interface of conductive hydrogels and the human skin, and seek approaches to improve interface compatibility and enhance sensor comfort, such as surface modifications and enhanced biocompatibility. (3) Sensor performance enhancement: improve the sensitivity and stability of conductive hydrogel materials by optimizing material structures, interface designs, and signal processing algorithms to enhance the performance metrics of sensors. (4) Lifespan and stability: investigate the lifespan and stability issues of conductive hydrogel materials, and explore new fabrication processes and material combinations to address these challenges.
In summary, the combination of conductive hydrogels and 3D printing technology holds great potential for the development of high-performance wearable sensors. However, there are still remaining challenges in both material development and technological advancements. With increasing efforts and focus, significant progress can be expected in the future.
Data availability
The data that support the findings of this study are available from the corresponding authors upon reasonable request.
Conflicts of interest
The authors declare that there are no competing interests.
Acknowledgements
This research was funded by the University-Industry Collaborative Education Program (no. 202102009004), and the Guangdong Basic and Applied Basic Research Foundation (no. 2021A1515111067, 2023A1515010735). The authors are grateful for the valuable comments from the anonymous referees.
References
- F. Acciaretti,
et al., Fabrication Strategies Towards Hydrogels for Biomedical Application: Chemical and Mechanical Insights, Chem. – Asian J., 2022, 17(22), e202200797 CrossRef CAS PubMed.
- U. S. K. Madduma-Bandarage and S. V. Madihally, Synthetic hydrogels: Synthesis, novel trends, and applications, J. Appl. Polym. Sci., 2021, 138(19), 50376 CrossRef CAS.
- K. Wang,
et al., Functional Hydrogels and Their Application in Drug Delivery, Biosensors, and Tissue Engineering, Int. J. Polym. Sci., 2019, 2019, 3160732 Search PubMed.
- J. J. Duan and L. N. Zhang, Robust and smart hydrogels based on natural polymers, Chin. J. Polym. Sci., 2017, 35(10), 1165–1180 CrossRef CAS.
- C. W. Peak,
et al., A review on tough and sticky hydrogels, Colloid Polym. Sci., 2013, 291(9), 2031–2047 CrossRef CAS.
- S. Talebian,
et al., Self-Healing Hydrogels: The Next Paradigm Shift in Tissue Engineering?, Adv. Sci., 2019, 6(16), 1801664 CrossRef PubMed.
- X. M. Zhang,
et al., The fabrication of antibacterial hydrogels for wound healing, Eur. Polym. J., 2021, 146, 110268 CrossRef CAS.
- X. Xu,
et al., Bioinspired double network hydrogels: from covalent double network hydrogels via hybrid double network hydrogels to physical double network hydrogels, Mater. Horiz., 2021, 8(4), 1173–1188 RSC.
- J. Zhang,
et al., Recent advances in magnetic hydrogels, Polym. Int., 2016, 65(12), 1365–1372 CrossRef CAS.
- R. Yang,
et al., Facile fabrication of carbon nanocolloids reinforced hydrogels for the application of high-performance strain sensors, Sens. Actuators, A, 2022, 359, 114507 CrossRef.
- M. Vazquez-Gonzalez and I. Willner, Stimuli-Responsive Biomolecule-Based Hydrogels and Their Applications, Angew. Chem., Int. Ed., 2020, 59(36), 15342–15377 CrossRef CAS PubMed.
- N. Nadeem,
et al., Thermosensitive hydrogels: from bench to market, Curr. Sci., 2018, 114(11), 2256–2266 CrossRef CAS.
- D. Zhong,
et al., Review of the Application of Intelligent Hydrogels in Drug Delivery Systems, Mater. Rev., 2012, 26(11), 83–88 CAS.
- Y. Gong,
et al., Preparation of Polymer Conductive Hydrogel and Its Application in Flexible Wearable Electronic Devices, Prog. Chem., 2022, 34(3), 616–629 CAS.
- S. Wang,
et al., Research progress in preparation and application of conductive hydrogels, Chem. Ind. Eng. Prog., 2021, 40(5), 2646–2664 CAS.
- Q. Rong,
et al., Conductive Hydrogels as Smart Materials for Flexible Electronic Devices, Chem. – Eur. J., 2018, 24(64), 16930–16943 CrossRef CAS PubMed.
- Z. Wang,
et al., Stretchable and tough conductive hydrogels for flexible pressure and strain sensors, J. Mater. Chem. B, 2020, 8(16), 3437–3459 RSC.
- Z. Chen,
et al., Multifunctional conductive hydrogels and their applications as smart wearable devices, J. Mater. Chem. B, 2021, 9(11), 2561–2583 RSC.
- J. Erfkamp,
et al., Enzyme-Functionalized Piezoresistive Hydrogel Biosensors for the Detection of Urea, Sensors, 2019, 19(13), 2858 CrossRef CAS PubMed.
- L. Chen,
et al., Flexible Capacitive Hydrogel Tactile Sensor With Adjustable Measurement Range Using Liquid Crystal and Carbon Nanotubes Composites, IEEE Trans. Electron Devices, 2017, 64(5), 1968–1972 CAS.
- W. Xu,
et al., Environmentally Friendly Hydrogel-Based Triboelectric Nanogenerators for Versatile Energy Harvesting and Self-Powered Sensors, Adv. Energy Mater., 2017, 7(1), 1601529 CrossRef.
- Z. Hu,
et al., Enhancing Strain-Sensing Properties of the Conductive Hydrogel by Introducing PVDF-TrFE, ACS Appl. Mater. Interfaces, 2022, 14(40), 45853–45868 CrossRef CAS PubMed.
- Z. Chen,
et al., 3D Printing of Multifunctional Hydrogels, Adv. Funct. Mater., 2019, 29(20), 1900971 CrossRef.
- J. Ai and P. Du, Discussion on 3D print model and technology, Appl. Mech. Mater., 2014, 543–547, 130–133 Search PubMed.
- X. Luo,
et al., Nanomaterials Reinforced Polymer Filament for Fused Deposition Modeling: A State-of-the-Art Review, Polymers, 2023, 15(14), 2980 CrossRef CAS PubMed.
- X. Xu,
et al., Research on 3D printing service model for innovation, entrepreneurship, and universal applications, J. Eng., 2020, 2020(13), 466–470 CrossRef.
- L. Jiang,
et al., Three-Dimensional
Printing and Injectable Conductive Hydrogels for Tissue Engineering Application, Tissue Eng., Part B, 2019, 25(5), 398–411 CrossRef CAS PubMed.
- T. Distler and A. R. Boccaccini, 3D printing of electrically conductive hydrogels for tissue engineering and biosensors – A review, Acta Biomater., 2020, 101, 1–13 CrossRef CAS PubMed.
- J. Xu,
et al., Design Strategies of Conductive Hydrogel for Biomedical Applications, Molecules, 2020, 25(22), 5296 CrossRef CAS PubMed.
- K. Liu,
et al., Conductive Hydrogels—A Novel Material: Recent Advances and Future Perspectives, J. Agric. Food Chem., 2020, 68(28), 7269–7280 CrossRef CAS PubMed.
- X. Liu,
et al., Functionalized Carbon Nanotube and Graphene Oxide Embedded Electrically Conductive Hydrogel Synergistically Stimulates Nerve Cell Differentiation, ACS Appl. Mater. Interfaces, 2017, 9(17), 14677–14690 CrossRef CAS PubMed.
- B. Pournemati,
et al., Injectable conductive nanocomposite hydrogels for cardiac tissue engineering: Focusing on carbon and metal-based nanostructures, Eur. Polym. J., 2022, 174, 111336 CrossRef CAS.
- J. Jiang,
et al., Mussel-Inspired Dopamine and Carbon Nanotube Leading to a Biocompatible Self-Rolling Conductive Hydrogel Film, Materials, 2017, 10(8), 964 CrossRef PubMed.
- E. P. Gilshteyn,
et al., A One-Step Method of Hydrogel Modification by Single-Walled Carbon Nanotubes for Highly Stretchable and Transparent Electronics, ACS Appl. Mater. Interfaces, 2018, 10(33), 28069–28075 CrossRef CAS PubMed.
- Z. Qin,
et al., Carbon Nanotubes/Hydrophobically Associated Hydrogels as Ultrastretchable, Highly Sensitive, Stable Strain, and Pressure Sensors, ACS Appl. Mater. Interfaces, 2020, 12(4), 4944–4953 CrossRef CAS PubMed.
- Y. Wu,
et al., Using TEMPO-oxidized-nanocellulose stabilized carbon nanotubes to make pigskin hydrogel conductive as flexible sensor and supercapacitor electrode: Inspired from a Chinese cuisine, Compos. Sci. Technol., 2020, 196, 108226 CrossRef CAS.
- H. Yu,
et al., Mechanically and Electrically Enhanced CNT–Collagen Hydrogels As Potential Scaffolds for Engineered Cardiac Constructs, ACS Biomater. Sci. Eng., 2017, 3(11), 3017–3021 CrossRef CAS PubMed.
- Y. Lu,
et al., Self-Recovery, Fatigue-Resistant, and Multifunctional Sensor Assembled by a Nanocellulose/Carbon Nanotube Nanocomplex-Mediated Hydrogel, ACS Appl. Mater. Interfaces, 2021, 13(42), 50281–50297 CrossRef CAS PubMed.
- G. Chen,
et al., Tough, conductive hydrogels with double-network based on hydrophilic polymer assistant well-dispersed carbon nanotube for innovative force sensor, Sci. China: Technol. Sci., 2022, 65(5), 1160–1168 CrossRef CAS.
- S. Han,
et al., Dual Conductive Network Hydrogel for a Highly Conductive, Self-Healing, Anti-Freezing, and Non-Drying Strain Sensor, ACS Appl. Polym. Mater., 2020, 2(2), 996–1005 CrossRef CAS.
- X. Wu,
et al., Three-dimensional printing of graphene-based materials and the application in energy storage, Mater. Today Adv., 2021, 11, 100157 CrossRef CAS.
- K. Zhihui and D. Min, Application of Graphene Oxide-Based Hydrogels in Bone Tissue Engineering, ACS Biomater. Sci. Eng., 2022, 8(7), 2849–2857 CrossRef PubMed.
- X. Wu,
et al., Joining of graphene flakes by low energy N ion beam irradiation, Appl. Phys. Lett., 2017, 110(13), 133102 CrossRef.
- C. Li and G. Shi, Functional gels based on chemically modified graphenes, Adv. Mater., 2014, 26(24), 3992–4012 CrossRef CAS PubMed.
- A. Xavier Mendes,
et al., Enhanced Electroactivity, Mechanical Properties, and Printability through the Addition of Graphene Oxide to Photo-Cross-linkable Gelatin Methacryloyl Hydrogel, ACS Biomater. Sci. Eng., 2021, 7(6), 2279–2295 CrossRef CAS PubMed.
- C. Yang,
et al., Reduced Graphene Oxide-Containing Smart Hydrogels with Excellent Electro-Response and Mechanical Properties for Soft Actuators, ACS Appl. Mater. Interfaces, 2017, 9(18), 15758–15767 CrossRef CAS PubMed.
- R. Zhao,
et al., Highly Strong, Stretchable, and Conductive Reduced Graphene Oxide Composite Hydrogel-Based Sensors for Motoring Strain and Pressure, ACS Appl. Polym. Mater., 2021, 3(10), 5155–5161 CrossRef CAS.
- Y. Zhang,
et al., Nanocellulose/Reduced Graphene Oxide Composite Hydrogels for High-Volumetric Performance Symmetric Supercapacitors, Energy Fuels, 2022, 36(15), 8506–8514 CrossRef CAS.
- X. Yang,
et al., Sandwich-like Polypyrrole/Reduced Graphene Oxide Nanosheets Integrated Gelatin Hydrogel as Mechanically and Thermally Sensitive Skinlike Bioelectronics, ACS Sustainable Chem. Eng., 2020, 8(29), 10726–10739 CAS.
- X. Liu,
et al., Smart Textile Based on 3D Stretchable Silver Nanowires/MXene Conductive Networks for Personal Healthcare and Thermal Management, ACS Appl. Mater. Interfaces, 2021, 13(47), 56607–56619 CrossRef CAS PubMed.
- X. Jiang,
et al., Thermoresponsive
hydrogel of poly(glycidyl methacrylate-co-N-isopropylacrylamide) as a nanoreactor of gold nanoparticles, J. Polym. Sci., Part A: Polym. Chem., 2007, 45(13), 2812–2819 CrossRef CAS.
- A. Zinchenko,
et al., DNA hydrogel as a template for synthesis of ultrasmall gold nanoparticles for catalytic applications, ACS Appl. Mater. Interfaces, 2014, 6(5), 3226–3232 CrossRef CAS PubMed.
- Y. Mao,
et al., A portable visual detection method based on a target-responsive DNA hydrogel and color change of gold nanorods, Chem. Commun., 2017, 53(47), 6375–6378 RSC.
- H. Zheng,
et al., Self-Healing, Self-Adhesive Silk Fibroin Conductive Hydrogel as a Flexible Strain Sensor, ACS Appl. Mater. Interfaces, 2021, 13(33), 40013–40031 CrossRef CAS PubMed.
- K. S. Kumar,
et al., Stretchable and Sensitive Silver Nanowire-Hydrogel Strain Sensors for Proprioceptive Actuation, ACS Appl. Mater. Interfaces, 2021, 13(31), 37816–37829 CrossRef CAS PubMed.
- X. Shen,
et al., Double-Network Hierarchical-Porous Piezoresistive Nanocomposite Hydrogel Sensors Based on Compressive Cellulosic Hydrogels Deposited with Silver Nanoparticles, ACS Sustainable Chem. Eng., 2020, 8(19), 7480–7488 CrossRef CAS.
- S. Hao,
et al., Tannic Acid-Silver Dual Catalysis Induced Rapid Polymerization of Conductive Hydrogel Sensors with Excellent Stretchability, Self-Adhesion, and Strain-Sensitivity Properties, ACS Appl. Mater. Interfaces, 2020, 12(50), 56509–56521 CrossRef CAS PubMed.
- G. Shi,
et al., An Antifouling Hydrogel Containing Silver Nanoparticles for Modulating the Therapeutic Immune Response in Chronic Wound Healing, Langmuir, 2019, 35(5), 1837–1845 CrossRef CAS PubMed.
- A. L. Urzedo,
et al., Cytotoxicity and Antibacterial Activity of Alginate Hydrogel Containing Nitric Oxide Donor and Silver Nanoparticles for Topical Applications, ACS Biomater. Sci. Eng., 2020, 6(4), 2117–2134 CrossRef CAS PubMed.
- T. Nezakati,
et al., Conductive Polymers: Opportunities and Challenges in Biomedical Applications, Chem. Rev., 2018, 118(14), 6766–6843 CrossRef CAS PubMed.
- B. Guo,
et al., Properties of conductive polymer hydrogels and their application in sensors, J. Polym. Sci., Part B: Polym. Phys., 2019, 57(23), 1606–1621 CrossRef CAS.
- Y. Zhao,
et al., Conducting Polymers and Their Applications in Diabetes Management, Sensors, 2016, 16(11), 1787 CrossRef PubMed.
- J. Chen,
et al., Stretchable, Injectable, and Self-Healing Conductive Hydrogel Enabled by Multiple Hydrogen Bonding toward Wearable Electronics, Chem. Mater., 2019, 31(12), 4553–4563 CrossRef CAS.
- W. Chen,
et al., Highly Deformable Graphene/Poly(3,4-ethylenedioxythiophene): Poly(styrene Sulfonate) Hydrogel Composite Film for Stretchable Supercapacitors, ACS Appl. Energy Mater., 2022, 5(6), 7277–7286 CrossRef CAS.
- R. A. Green,
et al., Conducting polymer-hydrogels for medical electrode applications, Sci. Technol. Adv. Mater., 2010, 11(1), 014107 CrossRef PubMed.
- J. Wu,
et al., Extremely Deformable, Transparent, and High-Performance Gas Sensor Based on Ionic Conductive Hydrogel, ACS Appl. Mater. Interfaces, 2019, 11(2), 2364–2373 CrossRef CAS PubMed.
- R. Tong,
et al., Highly Stretchable and Compressible Cellulose Ionic Hydrogels for Flexible Strain Sensors, Biomacromolecules, 2019, 20(5), 2096–2104 CrossRef CAS PubMed.
- Y. Liang,
et al., Tough and Stretchable Dual Ionically Cross-Linked Hydrogel with High Conductivity and Fast Recovery Property for High-Performance Flexible Sensors, ACS Appl. Mater. Interfaces, 2020, 12(1), 1577–1587 CrossRef CAS PubMed.
- B. Ying,
et al., An Ionic Hydrogel-Based Antifreezing Triboelectric Nanogenerator, ACS Appl. Electron. Mater., 2022, 4(4), 1930–1938 CrossRef CAS.
- H. Liu,
et al., Freezing-Tolerant, Highly Sensitive Strain and Pressure Sensors Assembled from Ionic Conductive Hydrogels with Dynamic Cross-Links, ACS Appl. Mater. Interfaces, 2020, 12(22), 25334–25344 CrossRef CAS PubMed.
- B. Zhang,
et al., Hierarchical Response Network Boosts Solvent-Free Ionic Conductive Elastomers with Extreme Stretchability, Healability, and Recyclability for Ionic Sensors, ACS Appl. Mater. Interfaces, 2022, 14(6), 8404–8416 CrossRef CAS PubMed.
- Z. Wu,
et al., Ultrasensitive, Stretchable, and Fast-Response Temperature Sensors Based on Hydrogel Films for Wearable Applications, ACS Appl. Mater. Interfaces, 2021, 13(18), 21854–21864 CrossRef CAS PubMed.
- J. Wu,
et al., Ultrasensitive and Stretchable Temperature Sensors Based on Thermally Stable and Self-Healing Organohydrogels, ACS Appl. Mater. Interfaces, 2020, 12(16), 19069–19079 CrossRef CAS PubMed.
- Z. Jiang,
et al., Multistimuli-Responsive PNIPAM-Based Double Cross-Linked Conductive Hydrogel with Self-Recovery Ability for Ionic Skin and Smart Sensor, Biomacromolecules, 2022, 23(12), 5239–5252 CrossRef CAS PubMed.
- Q. Pang,
et al., Temperature-Responsive Ionic Conductive Hydrogel for Strain and Temperature Sensors, ACS Appl. Mater. Interfaces, 2022, 14(23), 26536–26547 CrossRef CAS PubMed.
- Y. Liu,
et al., Poly(vinyl alcohol) Hydrogels with Integrated Toughness, Conductivity, and Freezing Tolerance Based on Ionic Liquid/Water Binary Solvent Systems, ACS Appl. Mater. Interfaces, 2021, 13(24), 29008–29020 CrossRef CAS PubMed.
- C. Zhao,
et al., Transparent, Antifreezing, Ionic Conductive Carboxymethyl Chitosan Hydrogels as Multifunctional Sensors, ACS Appl. Polym. Mater., 2022, 4(5), 4025–4034 CrossRef CAS.
- Z. Wang,
et al., 3D Printed Ultrasensitive Graphene Hydrogel Self-Adhesive Wearable Devices, ACS Appl. Electron. Mater., 2022, 4(11), 5199–5207 CrossRef CAS.
- S. Lu,
et al., Mussel-inspired blue-light-activated cellulose-based adhesive hydrogel with fast gelation, rapid haemostasis and antibacterial property for wound healing, Chem. Eng. J., 2021, 417, 129329 CrossRef CAS.
- F. Wang,
et al., Highly strong, tough, and stretchable conductive hydrogels based on silk sericin-mediated multiple physical interactions for flexible sensors, ACS Appl. Polym. Mater., 2021, 4(1), 618–626 CrossRef.
- N. Li,
et al., Highly Stretchable, Tough, and Self-Recoverable Cationic Guar Gum-Based Hydrogels for Flexible Sensors, ACS Appl. Polym. Mater., 2022, 4(8), 5717–5727 CrossRef.
- W. Dai,
et al., Highly Stretchable, Ultra-Sensitive, and Self-Healable Multifunctional Flexible Conductive Hydrogel Sensor for Motion Detection and Information Transmission, ACS Appl. Mater. Interfaces, 2023, 15(24), 29499–29510 CrossRef CAS PubMed.
- L. Zhou,
et al., Highly sensitive pressure and strain sensors based on stretchable and recoverable ion-conductive physically cross-linked double-network hydrogels, ACS Appl. Mater. Interfaces, 2020, 12(46), 51969–51977 CrossRef CAS PubMed.
- J. Lai,
et al., Highly stretchable, fatigue-resistant, electrically conductive, and temperature-tolerant ionogels for high-performance flexible sensors, ACS Appl. Mater. Interfaces, 2019, 11(29), 26412–26420 CrossRef CAS PubMed.
- J. Xu,
et al., Ultrastretchable wearable strain and pressure sensors based on adhesive, tough, and self-healing hydrogels for human motion monitoring, ACS Appl. Mater. Interfaces, 2019, 11(28), 25613–25623 CrossRef CAS PubMed.
- J. Li,
et al., Self-Healable Gels for Use in Wearable Devices, Chem. Mater., 2017, 29(21), 8932–8952 CrossRef CAS.
- S. Li,
et al., Flexible Self-Repairing Materials for Wearable Sensing Applications: Elastomers and Hydrogels, Macromol. Rapid Commun., 2020, 41(23), e2000444 CrossRef PubMed.
- A. A. Nada,
et al., Irreversible and Self-Healing Electrically Conductive Hydrogels Made of Bio-Based Polymers, Int. J. Mol. Sci., 2022, 23(2), 842 CrossRef CAS PubMed.
- X. Zhang,
et al., Mussel-Inspired Conductive Hydrogel with Self-Healing, Adhesive, and Antibacterial Properties for Wearable Monitoring, ACS Appl. Polym. Mater., 2021, 3(11), 5798–5807 CrossRef CAS.
- Z. Jia,
et al., Polypyrrole/PU hybrid hydrogels: electrically conductive and fast self-healing for potential applications in body-monitor sensors, New J. Chem., 2021, 45(16), 7321–7331 RSC.
- J. Mo,
et al., Design of ultra-stretchable, highly adhesive and self-healable hydrogels via tannic acid-enabled dynamic interactions, Mater. Horiz., 2021, 8(12), 3409–3416 RSC.
- Q. Yu,
et al., Mussel-inspired hydrogels as tough, self-adhesive and conductive bioelectronics: a review, Soft Matter, 2021, 17(39), 8786–8804 RSC.
- T. Zhu,
et al., Recent advances in conductive hydrogels: classifications, properties, and applications, Chem. Soc. Rev., 2023, 52(2), 473–509 RSC.
- Y. Xiong,
et al., A review of the properties and applications of bioadhesive hydrogels, Polym. Chem., 2021, 12(26), 3721–3739 RSC.
- G. Bovone,
et al., Engineering Hydrogel Adhesion for Biomedical Applications via Chemical Design of the Junction, ACS Biomater. Sci. Eng., 2021, 7(9), 4048–4076 CrossRef CAS PubMed.
- H. Jafari,
et al., Tannic acid: a versatile polyphenol for design of biomedical hydrogels, J. Mater. Chem. B, 2022, 10(31), 5873–5912 RSC.
- H. Fan,
et al., Tough, Swelling-Resistant, Self-Healing, and Adhesive Dual-Cross-Linked Hydrogels Based on Polymer–Tannic Acid Multiple Hydrogen Bonds, Macromolecules, 2018, 51(5), 1696–1705 CrossRef CAS.
- R. Yang,
et al., Recent advances in the 3D printing of electrically conductive hydrogels for flexible electronics, J. Mater. Chem. C, 2022, 10(14), 5380–5399 RSC.
- S. Kumar,
et al., 3D Bioprinting of Nature-Inspired Hydrogel Inks Based on Synthetic Polymers, ACS Appl. Polym. Mater., 2021, 3(8), 3685–3701 CrossRef CAS.
- Y. Ren and J. Feng, Skin-Inspired Multifunctional Luminescent Hydrogel Containing Layered Rare-Earth Hydroxide with 3D Printability for Human Motion Sensing, ACS Appl. Mater. Interfaces, 2020, 12(6), 6797–6805 CrossRef CAS PubMed.
- S. Liu and L. Li, Ultrastretchable and Self-Healing Double-Network Hydrogel for 3D Printing and Strain Sensor, ACS Appl. Mater. Interfaces, 2017, 9(31), 26429–26437 CrossRef CAS PubMed.
- W. Zhao,
et al., Printable hydrogels based on starch and natural rubber latex with high toughness and self-healing capability, Int. J. Biol. Macromol., 2022, 218, 580–587 CrossRef CAS PubMed.
- Z. Deng,
et al., Stimuli-Responsive Conductive Nanocomposite Hydrogels with High Stretchability, Self-Healing, Adhesiveness, and 3D Printability for Human Motion Sensing, ACS Appl. Mater. Interfaces, 2019, 11(7), 6796–6808 CrossRef CAS PubMed.
- S. Wei,
et al., Scalable and Automated Fabrication of Conductive Tough-Hydrogel Microfibers with Ultrastretchability, 3D Printability, and Stress Sensitivity, ACS Appl. Mater. Interfaces, 2018, 10(13), 11204–11212 CrossRef CAS PubMed.
- J.-C. Lin,
et al., Flexible and Stretchable Electrically Conductive Polymer Materials for Physical Sensing Applications, Polym. Rev., 2022, 63(1), 67–126 CrossRef.
- J. Li,
et al., Review—Recent Progress in Flexible and Stretchable Piezoresistive Sensors and Their Applications, J. Electrochem. Soc., 2020, 167, 037561 CrossRef CAS.
- X. Gao,
et al., Flexible Stannum-Doped SrTiO3 Nanofiber Membranes for Highly Sensitive and Reliable Piezoresistive Pressure Sensors, ACS Appl. Mater. Interfaces, 2021, 13(44), 52811–52821 CrossRef CAS PubMed.
- H. Pan and T. W. Lee, Recent Progress in Development of Wearable Pressure Sensors Derived from Biological Materials, Adv. Healthc. Mater., 2021, 10(17), e2100460 CrossRef PubMed.
- C. Cui,
et al., Recent Progress in Natural Biopolymers Conductive Hydrogels for Flexible Wearable Sensors and Energy Devices: Materials, Structures, and Performance, ACS Appl. Bio Mater., 2020, 4(1), 85–121 CrossRef PubMed.
- Y. Li,
et al., Wearable and Biodegradable Sensors for Human Health Monitoring, ACS Appl. Bio Mater., 2021, 4(1), 122–139 CrossRef CAS PubMed.
- A. Sharma,
et al., Ultrasensitive flexible wearable pressure/strain sensors: Parameters, materials, mechanisms and applications, Sens. Actuators, A, 2022, 347, 113934 CrossRef CAS.
- X. Han,
et al., Green and stable piezoresistive pressure sensor based on lignin-silver hybrid nanoparticles/polyvinyl alcohol hydrogel, Int. J. Biol. Macromol., 2021, 176, 78–86 CrossRef CAS PubMed.
- H. Ahmadi,
et al., Development of Ultrasensitive Biomimetic Auditory Hair Cells Based on Piezoresistive Hydrogel Nanocomposites, ACS Appl. Mater. Interfaces, 2021, 13(37), 44904–44915 CrossRef CAS PubMed.
- S.-H. Hwang,
et al., Pt Nanoparticle-Decorated Reduced Graphene Oxide Hydrogel for High-Performance Strain Sensor: Tailoring Piezoresistive Property by Controlled Microstructure of Hydrogel, ACS Appl. Nano Mater., 2018, 1(6), 2836–2843 CrossRef CAS.
- H. Ullah,
et al., Recent Advances in Stretchable and Wearable Capacitive Electrophysiological Sensors for Long-Term Health Monitoring, Biosensors, 2022, 12(8), 630 CrossRef CAS PubMed.
- H. Wang,
et al., Flexible capacitive pressure sensors for wearable electronics, J. Mater. Chem. C, 2022, 10(5), 1594–1605 RSC.
- J. Huang,
et al., A Dual-Mode Wearable Sensor Based on Bacterial Cellulose Reinforced Hydrogels for Highly Sensitive Strain/Pressure Sensing, Adv. Electron. Mater., 2019, 6(1), 1900934 CrossRef.
- R. B. Mishra,
et al., Recent Progress on Flexible Capacitive Pressure Sensors: From Design and Materials to Applications, Adv. Mater. Technol., 2021, 6(4), 2001023 CrossRef.
- H. Nesser and G. Lubineau, Strain Sensing by Electrical Capacitive Variation: From Stretchable Materials to Electronic Interfaces, Adv. Electron. Mater., 2021, 7(10), 2100190 CrossRef CAS.
- H. Xu,
et al., An ultra-stretchable, highly sensitive and biocompatible capacitive strain sensor from an ionic nanocomposite for on-skin monitoring, Nanoscale, 2019, 11(4), 1570–1578 RSC.
- P.-C. Lai and S.-S. Yu, Cationic Cellulose Nanocrystals-Based Nanocomposite Hydrogels: Achieving 3D Printable Capacitive Sensors with High Transparency and Mechanical Strength, Polymers, 2021, 13(5), 688 CrossRef CAS PubMed.
- J. Qin,
et al., Flexible and Stretchable Capacitive Sensors with Different Microstructures, Adv. Mater., 2021, 33(34), e2008267 CrossRef PubMed.
- F. Mo,
et al., A Highly Stable and Durable Capacitive Strain Sensor Based on Dynamically Super-Tough Hydro/Organo-Gels, Adv. Funct. Mater., 2021, 31(28), 2010830 CrossRef CAS.
- H. Lei,
et al., Advances in self-powered triboelectric pressure sensors, J. Mater. Chem. A, 2021, 9(36), 20100–20130 RSC.
- H. Joo,
et al., Piezo/Triboelectric Effect Driven Self-Powered Gas Sensor for Environmental Sensor Networks, Energy Technol., 2022, 10(7), 2200113 CrossRef CAS.
- Z. Han,
et al., Combination of Piezoelectric and Triboelectric Devices for Robotic Self-Powered Sensors, Micromachines, 2021, 12(7), 813 CrossRef PubMed.
- Y. Shao,
et al., Nanogenerator-based self-powered sensors for data collection, Beilstein J. Nanotechnol., 2021, 12, 680–693 CrossRef CAS PubMed.
- P. Song,
et al., Nanogenerators for wearable bioelectronics and biodevices, J. Phys. D: Appl. Phys., 2019, 52(2), 023002 CrossRef.
- C. Wang,
et al., An advanced strategy to enhance TENG output: reducing triboelectric charge decay, Adv. Mater., 2023, 35(17), 2209895 CrossRef CAS PubMed.
- H. J. Lee,
et al., Wearable Triboelectric Strain-Insensitive Pressure Sensors Based on Hierarchical Superposition Patterns, ACS Sens., 2021, 6(6), 2411–2418 CrossRef CAS PubMed.
- F. Rahimi Sardo,
et al., Recent Progress of Triboelectric Nanogenerators for Biomedical Sensors: From Design to Application, Biosensors, 2022, 12(9), 697 CrossRef CAS PubMed.
- Q. Gao,
et al., Triboelectric mechanical sensors-Progress and prospects, Extreme Mech. Lett., 2021, 42, 101100 CrossRef.
- Y. K. Zhou,
et al., Triboelectric nanogenerator based self-powered sensor for artificial intelligence, Nano Energy, 2021, 84, 105887 CrossRef CAS.
- Q. Miao,
et al., Toward Self-Powered Inertial Sensors Enabled by Triboelectric Effect, ACS Appl. Electron. Mater., 2020, 2(10), 3072–3087 CrossRef CAS.
- L. Dong,
et al., Stretchable, Adhesive, Self-Healable, and Conductive Hydrogel-Based Deformable Triboelectric Nanogenerator for Energy Harvesting and Human Motion Sensing, ACS Appl. Mater. Interfaces, 2022, 14(7), 9126–9137 CrossRef CAS PubMed.
- F. He,
et al., Stretchable, Biocompatible, and Multifunctional Silk Fibroin-Based Hydrogels toward Wearable Strain/Pressure Sensors and Triboelectric Nanogenerators, ACS Appl. Mater. Interfaces, 2020, 12(5), 6442–6450 CrossRef CAS PubMed.
- X. Dai,
et al., Ultra-antifreeze, ultra-stretchable, transparent, and conductive hydrogel for multi-functional flexible electronics as strain sensor and triboelectric nanogenerator, Nano Res., 2022, 15(6), 5461–5468 CrossRef CAS.
- L. Wang and W. A. Daoud, Hybrid conductive hydrogels for washable human motion energy harvester and self-powered temperature-stress dual sensor, Nano Energy, 2019, 66, 104080 CrossRef CAS.
- Y. Luo,
et al., Highly sensitive strain sensor and self-powered triboelectric nanogenerator using a fully physical crosslinked double-network conductive hydrogel, Nano Energy, 2022, 104, 107955 CrossRef CAS.
- C. Zhao,
et al., Piezoelectric Sensor Measurement Principles and Research Progress on Sensitive Element Materials, Mater. Mech. Eng., 2020, 44(6), 93–98 Search PubMed.
- Z. H. Zhang,
et al., Sensitivity enhancement of piezoelectric force sensors by using multiple piezoelectric effects, AIP Adv., 2016, 6(7), 075320 CrossRef.
- D. H. Han and L. H. Kang, Piezoelectric properties of paint sensor according to piezoelectric materials, Funct. Compos. Struct., 2020, 2(2), 025002 CrossRef CAS.
- C. Liu,
et al., 3D Printing Technologies for Flexible Tactile Sensors toward Wearable Electronics and Electronic Skin, Polymers, 2018, 10(6), 629 CrossRef PubMed.
- Y. Ni,
et al., A review of 3D-printed sensors, Appl. Spectrosc. Rev., 2017, 52(7), 623–652 CrossRef CAS.
- Y. Jiang,
et al., Recent Advances in 3D Printed Sensors: Materials, Design, and Manufacturing, Adv. Mater. Technol., 2022, 8(2), 2200492 CrossRef.
- H. Cheng,
et al., Recent research progress on additive manufacturing of high-strength low-alloy steels: focusing on the processing parameters, microstructures and properties, Mater. Today Commun., 2023, 36, 106616 CrossRef CAS.
- J. Li,
et al., 3D printing of hydrogels: Rational design strategies and emerging biomedical applications, Mater. Sci. Eng., R, 2020, 140, 100543 CrossRef.
- A. Kalkal,
et al., Recent advances in 3D printing technologies for wearable (bio)sensors, Addit. Manuf., 2021, 46, 102088 CAS.
- M. Guvendiren,
et al., Designing Biomaterials for 3D Printing, ACS Biomater. Sci. Eng., 2016, 2(10), 1679–1693 CrossRef CAS PubMed.
- H. W. Tan,
et al., 3D printed electronics:
Processes, materials and future trends, Prog. Mater. Sci., 2022, 127, 100945 CrossRef CAS.
- X. N. Zhang,
et al., Recent advances in 3D printing of tough hydrogels: A review, Composites, Part B, 2022, 238, 109895 CrossRef CAS.
- R. Brighenti,
et al., Laser-based additively manufactured polymers: a review on processes and mechanical models, J. Mater. Sci., 2021, 56(2), 961–998 CrossRef CAS.
- Y. Bao, Recent Trends in Advanced Photoinitiators for Vat Photopolymerization 3D Printing, Macromol. Rapid Commun., 2022, 43(14), 2200202 CrossRef CAS PubMed.
- Z. Wang,
et al., Visible Light Photoinitiation of Cell-Adhesive Gelatin Methacryloyl Hydrogels for Stereolithography 3D Bioprinting, ACS Appl. Mater. Interfaces, 2018, 10(32), 26859–26869 CrossRef CAS PubMed.
- D. Zhang,
et al., 3D printing of glass by additive manufacturing techniques: a review, Front. Optoelectron., 2021, 14(3), 263–277 CrossRef PubMed.
- G. Burke,
et al., Effect of Stereolithography 3D Printing on the Properties of PEGDMA Hydrogels, Polymers, 2020, 12(9), 2015 CrossRef CAS PubMed.
- Y. Wu,
et al., Fabrication of conductive gelatin methacrylate-polyaniline hydrogels, Acta Biomater., 2016, 33, 122–130 CrossRef CAS PubMed.
- J. Odent,
et al., Highly Elastic, Transparent, and Conductive 3D-Printed Ionic Composite Hydrogels, Adv. Funct. Mater., 2017, 27(33), 1701807 CrossRef.
- J. Odent,
et al., 3D-Printed Stacked Ionic Assemblies for Iontronic Touch Sensors, Adv. Funct. Mater., 2022, 33, 2210485 CrossRef.
- Y. Wu,
et al., Photocurable 3D Printing of High Toughness and Self-Healing Hydrogels for Customized Wearable Flexible Sensors, Adv. Funct. Mater., 2021, 31(21), 21072 Search PubMed.
- J. Warner,
et al., Design and 3D Printing of Hydrogel Scaffolds with Fractal Geometries, ACS Biomater. Sci. Eng., 2016, 2(10), 1763–1770 CrossRef CAS PubMed.
- H. Quan,
et al., Photo-curing 3D printing technique and its challenges, Bioact. Mater., 2020, 5(1), 110–115 CrossRef PubMed.
- K. B. Fritzler and V. Y. Prinz, 3D printing methods for micro- and nanostructures, Phys.-Usp., 2019, 62(1), 54–69 CrossRef CAS.
- M. Pagac,
et al., A review of vat photopolymerization technology: Materials, applications, challenges, and future trends of 3d printing, Polymers, 2021, 13(4), 598 CrossRef CAS PubMed.
- G. Taormina,
et al., 3D printing processes for photocurable polymeric materials: technologies, materials, and future trends, J. Appl. Biomater. Funct. Mater., 2018, 16(3), 151–160 CAS.
- A. Amini,
et al., Recent developments in digital light processing 3D-printing techniques for microfluidic analytical devices, J. Chromatogr. A, 2023, 1692, 463842 CrossRef CAS PubMed.
- H. G. Hosseinabadi,
et al., Digital light processing bioprinting advances for microtissue models, ACS Biomater. Sci. Eng., 2022, 8(4), 1381–1395 CrossRef PubMed.
- M. T. Sultan,
et al., Three-Dimensional Digital Light-Processing Bioprinting Using Silk Fibroin-Based Bio-Ink: Recent Advancements in Biomedical Applications, Biomedicines, 2022, 10(12), 3224 CrossRef CAS PubMed.
- L.-L. Naroa,
et al., Digital Light 3D Printing of PEDOT-Based Photopolymerizable Inks for Biosensing, ACS Appl. Polym. Mater., 2022, 4(9), 6749–6759 CrossRef PubMed.
- B. Guo,
et al., 3D printing of electrically conductive and degradable hydrogel for epidermal strain sensor, Compos. Commun., 2023, 37, 101454 CrossRef.
- S. Naghieh and X. Chen, Printability–A key issue in extrusion-based bioprinting, J. Pharm. Anal., 2021, 11(5), 564–579 CrossRef PubMed.
- A. Patel and M. Taufik, Extrusion-Based Technology in Additive Manufacturing: A Comprehensive Review, Arabian J. Sci. Eng., 2022 Search PubMed.
- J. K. Placone and A. J. Engler, Recent Advances in Extrusion-Based 3D Printing for Biomedical Applications, Adv. Healthcare Mater., 2017, 7(8), e1701161 CrossRef PubMed.
- L. Ning and X. Chen, A brief review of extrusion-based tissue scaffold bio-printing, Biotechnol. J., 2017, 12(8), 1600671 CrossRef PubMed.
- M. Schouten,
et al., A Review of Extrusion-Based 3D Printing for the Fabrication of Electro- and Biomechanical Sensors, IEEE Sens. J., 2021, 21(11), 12900–12912 CAS.
- M. A. S. R. Saadi,
et al., Direct Ink Writing: A 3D Printing Technology for Diverse Materials, Adv. Mater., 2022, 34(28), e2108855 CrossRef CAS PubMed.
- Z. Hou,
et al., Direct Ink Writing of Materials for Electronics-Related Applications: A Mini Review, Front. Mater., 2021, 8, 647229 CrossRef.
- S. Tajik,
et al., 3D Printing of Hybrid-Hydrogel Materials for Tissue Engineering: a Critical Review, Regener. Eng. Transl. Med., 2022, 9, 29–41 CrossRef PubMed.
- M. Seong,
et al., 3D Printable Self-Adhesive and Self-Healing Ionotronic Hydrogels for Wearable Healthcare Devices, ACS Appl. Mater. Interfaces, 2023, 15(8), 11042–11052 CrossRef CAS PubMed.
- L. Zhang,
et al., 3D direct printing of mechanical and biocompatible hydrogel meta-structures, Bioact. Mater., 2022, 10, 48–55 CrossRef CAS PubMed.
- Y. Xu,
et al., Preparation of a photocurable hydrogel with adjustable mechanical properties for 3D printing, Rapid Prototyp. J., 2021, 27(4), 797–807 CrossRef.
- K. B. C. Imani,
et al., High-Resolution 3D Printing of Mechanically Tough Hydrogels Prepared by Thermo-Responsive Poloxamer Ink Platform, Macromol. Rapid Commun., 2022, 43(2), 2100579 CrossRef CAS PubMed.
- C. B. Highley,
et al., Direct 3D Printing of Shear-Thinning Hydrogels into Self-Healing Hydrogels, Adv. Mater., 2015, 27(34), 5075–5079 CrossRef CAS PubMed.
- P. Jiang,
et al., Direct ink writing with high-strength and swelling-resistant biocompatible physically crosslinked hydrogels, Biomater. Sci., 2019, 7(5), 1805–1814 RSC.
- P. C. Lai,
et al., Thermally Induced Gelation of Cellulose Nanocrystals in Deep Eutectic Solvents for 3D Printable and Self-Healable Ionogels, ACS Appl. Polym. Mater., 2022, 4(12), 9221–9230 CrossRef CAS.
- T. H. Vo,
et al., Jammed Microgels in Deep Eutectic Solvents as a Green and Low-Cost Ink for 3D Printing of Reliable Auxetic Strain Sensors, ACS Appl. Mater. Interfaces, 2023, 15(27), 33109–33118 CrossRef CAS PubMed.
- X. Zhang,
et al., 3D printable conductive ionic hydrogels with self-adhesion performance for strain sensing, J. Mater. Chem. C, 2022, 10(38), 14288–14295 RSC.
- Y. Guo,
et al., Inkjet and inkjet-based 3D printing: connecting fluid properties and printing performance, Rapid Prototyp. J., 2017, 23(3), 562–576 CrossRef.
- M. A. Shah,
et al., Classifications and Applications of Inkjet Printing Technology: A Review, IEEE Access, 2021, 9, 140079–140102 Search PubMed.
- H. Hussin,
et al., Review-Recent Progress in the Diversity of Inkjet-Printed Flexible Sensor Structures in Biomedical Engineering Applications, J. Electrochem. Soc., 2021, 168(7), 077508 CrossRef CAS.
- A. Salim and S. Lim, Review of Recent Inkjet-Printed Capacitive Tactile Sensors, Sensors, 2017, 17(11), 2593 CrossRef PubMed.
- K. Zub,
et al., Inkjet Printing and 3D Printing Strategies for Biosensing, Analytical, and Diagnostic Applications, Adv. Mater., 2022, 34(31), e2105015 CrossRef PubMed.
-
H. V. Panchawagh, et al., Silicon Micromachined Continuous Inkjet (CIJ) Printhead with Integral Deflection and Guttering, in 25th International Conference on Digital Printing Technologies, Louisville, KY, 2009.
- T. H. Phung and K.-S. Kwon, Improved Continuous Inkjet for Selective Area Coating Using High-Viscosity Insulating Inks, Adv. Eng. Mater., 2022, 24(8), 2101527 CrossRef.
- G. L. Duffy,
et al., 3D reactive inkjet printing of poly-varepsilon-lysine/gellan gum hydrogels for potential corneal constructs, Mater. Sci. Eng., C, 2021, 131, 112476 CrossRef CAS PubMed.
- J. T. Delaney,
et al., Reactive inkjet printing of calcium alginate hydrogel porogens—a new strategy to open-pore structured matrices with controlled geometry, Soft Matter, 2010, 6(5), 866–869 RSC.
- M. Y. Teo,
et al., Enabling Free-Standing 3D Hydrogel Microstructures with Microreactive Inkjet Printing, ACS Appl. Mater. Interfaces, 2020, 12(1), 1832–1839 CrossRef CAS PubMed.
- Z. Huang,
et al., Highly stretchable hydrogels for sensitive pressure sensor and programmable surface patterning by thermal bubble inkjet technology, J. Appl. Polym. Sci., 2020, 137, e49146 CrossRef.
|
This journal is © The Royal Society of Chemistry 2023 |