Resource recovery technologies as microbial risk barriers: towards safe use of excreta in agriculture based on hazard analysis and critical control point†
Received
1st November 2022
, Accepted 26th February 2023
First published on 1st March 2023
Abstract
Agricultural use of human excreta contributes to sustainable nutrient resource management. In contrast, resource recovery from human excreta is associated with the risk of infection by pathogenic microorganisms. The microbial risk associated with human excreta needs to be properly managed. Pathogen inactivation efficacy of resource recovery technologies should be evaluated so that individual resource recovery processes can be monitored as health risk barriers. To this end, we reviewed the sanitization potential of resource recovery technologies from human excreta and identified the monitoring parameters of hazard analysis and critical control point (HACCP). We describe the inactivation of surrogate microorganisms in selected technologies in terms of the physicochemical conditions of matrices, different tolerances among surrogate microorganisms, and inactivation mechanisms. The estimated storage/operating time required to achieve the target log reduction values (LRVs) is shortened in thermal processes such as thermophilic storage, drying, composting, microwaving, and thermophilic digestion. In most processes, phage and helminth eggs were found to be appropriate indicators for conservative estimation. Finally, we argue that sanitization is congruent with decomposition of readily degradable organic matter and resource recovery. The barrier efficacy of resource recovery technologies should be validated using a pathogen inactivation kinetics model so that the exposure risk to infectious pathogens is sufficiently reduced through a reasonable combination of non-treatment and non-technical health risk barriers.
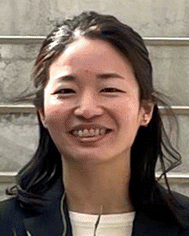 Wakana Oishi | Dr. Wakana Oishi is an assistant professor in the Department of Civil and Environmental Engineering at Tohoku University. Her research focuses on microbial risk management for the safe resource recovery from human excreta. She specializes in the modeling of inactivation of pathogens in environmental matrices and infection risk associated with recycling human excreta. |
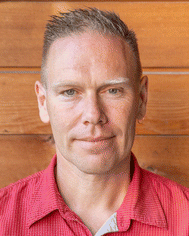 Björn Vinnerås | Dr. Björn Vinnerås is a professor in the Department of Energy and Technology at the Swedish University of Agricultural Sciences. He specializes in developing technologies to recycle plant nutrients from human excreta back to agricultural fields in an efficient and safe way, and mainly works on hygiene technology, protein production, and recovering plant nutrients from source separating sewage systems. |
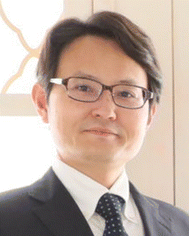 Daisuke Sano | Dr. Daisuke Sano is a professor in the Department of Civil and Environmental Engineering at Tohoku University. He specializes in health-related water virology and public health. His group's research has focused on water and public health issues, including anti-microbial resistant bacteria in a water environment, histo-blood group antigen-positive bacteria in the ecology of human noroviruses, and modeling of enteric viruses' disinfection/removal. |
Water impact
Pathogens in human excreta can be inactivated through physical, chemical, and biological processes for recovering plant nutrients and organics in human excreta. This study summarizes the sanitization potential and important factors that affect the inactivation rate of pathogens in excreta matrices, aiding the identification of appropriate operational parameters to be monitored to improve microbiological quality of the final products.
|
1. Introduction
Nutrient recycling of human excreta for agricultural production is a sustainable nutrient management approach to feed the growing global population.1 A paradigm shift that reframes sanitation as part of integrated nutrient management in farming systems was proposed by Harder et al.2 Human excreta are particularly rich in plant nutrients as an average person excretes 4.5 kg of nitrogen, 0.5 kg of phosphorus, and 1.2 kg of potassium every year.3 These nutrients excreted from the global populations amount to 41 million tones, which represents 28% of the current consumption of nitrogen, phosphorus, and potassium.4 Agricultural resources are recovered from human excreta using appropriate sanitation technologies.5 However, human excreta contain pathogens that cause infectious diseases. To protect public health, resource recovery activities should be guided by an appropriate health risk management program. Additional benefits from treating and recycling the excreta is that we decrease the environmental pollution and stop pathogens from reaching the environment.
The WHO Guidelines6 provide a comprehensive framework for health risk management of agricultural use of human excreta. The framework consists of 1) identification of risk points, 2) identification of parameters for monitoring risk points, 3) development of multiple barriers, 4) monitoring strategy for developed barriers, and 5) validation of the effectiveness of health protection measures.6 Sanitation Safety Planning (SSP) is a tool that guides local authorities and practitioners to implement the 2006 WHO guidelines.
SSP uses the methods and procedures of hazard analysis and critical control point (HACCP) in the operational monitoring and validation of a sanitation system.7 The fundamental principle of HACCP is monitoring operational parameters (hereinafter monitoring parameters) and record keeping at a critical control point (CCP), which is a vital process to reduce infection risk. The critical limits (CLs) of the monitoring parameter are defined as the standard values that must be achieved at the CCPs.8 Technological microbial risk barriers, such as storage and treatment processes, are monitored as CCPs in sanitation systems (Fig. 1). Microbial concentration is usually not used as a monitoring parameter for CCPs. Chemical and physical indicators that reflect the level of pathogens and can be quickly monitored are more suitable monitoring parameters; thus, the pathogen concentration in products from sanitation systems is indirectly estimated based on the monitoring parameters, which is the basis of HACCP. The CLs can be determined in advance using a mathematical model that is a function of chemical and physical indicators (Fig. 1).9
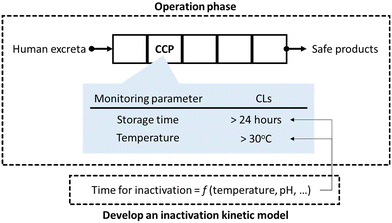 |
| Fig. 1 Implementation of the HACCP approach to a process train for resource recovery from human excreta. A process train is composed of sequential treatment units, including sedimentation, dewatering, stabilization of readily degradable organic matter, and storage. A critical control point (CCP) identified among the continuous processes is monitored with monitoring parameters so that they meet the critical limits (CLs). | |
When pathogens are inactivated in a resource recovery process, the process functions as a health risk barrier and should be monitored as CCP. The WHO recommends operational monitoring of indicators that are quickly monitored but represent the pathogen level; it recommends storage time based on the temperature and pH of fecal sludge.6 Meanwhile, for emerging resource recovery systems and practices which are not restricted by the WHO guidelines, it recommends voluntary evaluation of pathogen inactivation values and validation of the microbial quality of products from sanitation systems.6 The validation thresholds are specified by the WHO, International Organization for Standardization (ISO), and local governments.6,10–13 The ISO 30500 specifies the target log reduction values of surrogate microorganisms, and they also addresses the need for performance testing of treatment units to evaluate inactivation efficacy of surrogate microorganisms.12 Pathogen inactivation efficacy of emerging resource recovery technologies have been evaluated over the past few years; pathogen inactivation and biocidal factors have been summarized in previous reports.14–16 However, individual technologies are yet to be characterized for implementing the HACCP approach in SSP. To fully implement HACCP-based management in SSP, the following procedures are needed for technological barriers in advance:8,17 1) identification of CCPs in a sanitation system, 2) selection of monitoring parameters that affect pathogen inactivation, 3) development of a pathogen inactivation kinetics model, and 4) determination of the CLs for sufficient inactivation of pathogens.
This review aimed to identify the potential CCPs among resource recovery technologies used in a non-sewer sanitation system, and to identify the monitoring parameters at CCPs. First, we evaluated the barrier efficacy of individual technologies based on storage/operating time to reduce the concentration of the surrogates to the target log reduction values (LRVs). The inactivation rate of pathogens is influenced by physicochemical factors of matrices.17 Therefore, CLs of storage time and environmental factors were determined using regression tree which allowed to categorize the required storage time according to the environmental condition of storage. This review provides with the potential CCPs in a non-sewer sanitation system, what to be monitored at CCPs, and the concept of determining CLs which needs to be achieved at CCPs. The concept provided in this review assists engineers and practitioners implementing the HACCP-based microbial risk management which facilitates recycling of nutrients in human excreta to agriculture.
2. Methods
2.1 Target log reduction values
The target LRVs are shown in the Table 1. For viruses and phages, the target LRVs were set to 9
log10, according to the suggestion in the 2006 WHO Guidelines.6 Bacterial concentrations in feces were approximated to 9
log10 cfu g−1 feces,18 and regulated to <103 cfu g−1 feces;6,18 thus, 6
log10 inactivation is required. The concentration of helminth eggs in fecal matters is 4
log10 eggs per g feces,18 and viable egg should not be detected,6 accordingly 4
log10 inactivation is required. Pathogens originated from misplaced feces are diluted by 10
000-fold in source-separated urine,6 therefore, the target inactivation for urine is smaller than fecal matter: 5
log10 unit of viruses and phages, and 2
log10 unit of bacteria. For helminth eggs, we set a 1
log10 unit as a conservative target.
Table 1 Target inactivation values (log10(Nt/N0)) of virus/phage, helminth egg, and bacteria in urine and fecal sludge, determined according to the 2006 WHO requirements
|
In urine |
In fecal sludge |
Virus and phage |
5 |
9 |
Helminth egg |
1 |
4 |
Bacteria |
2 |
6 |
2.2 Estimation of storage duration to achieve the target log reduction values of microorganisms
This study estimated time to achieve the target LRVs based on the data reported in previous studies. We extracted time-course concentrations of microorganisms when they were provided in a table (i.e., numerical form). Data extraction from a figure was carried out using the WebPlotDigitizer version 4.2.19 Time-course concentration of microorganisms were applied to the Hom's model.20where Ni and N0 are the concentration of microorganisms at time i and 0, k is the inactivation rate constant, and m is the empirical constant. Storage duration to achieve the target LRV was derived as follows: | 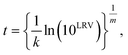 | (2) |
where k and m are the estimated parameters of Hom's model. When the k value was provided, we used it to estimate storage duration to achieve the target LRVs. The parameter estimation was performed based on the maximum likelihood approach using the statistical software R (version 3.6.1)21 (see ESI†).
2.3 Regression tree
Regression trees were created using the rpart package in the statistical software R.21 The explanatory variables employed in a regression for stored urine were pH, temperature, dilution ratio, and those employed for stored fecal sludge were pH, temperature, moisture content, uncharged ammonia concentration, and types of desiccants. The number of branches was determined based on the complexity of parameters to generate minimum error in cross-validation.
3. Sanitation technologies for agri-resource recovery
3.1 Urine
Urine contains the largest proportion of nitrogen (90%), phosphorus (50–65%), and potassium (50–80%) of the feces and urine fractions released from the body.22 In fresh urine, approximately 85% of nitrogen present in the form of urea, while urea is hydrolyzed to ammonium by urease produced by urease-forming bacteria during collection and storage.23 Simplest management is storage and direct application of the urine, however the concentration is low, about 0.6%, large volumes need to be transported, stored and applied when reused. Several treatment technologies to remove the water to produce a concentrated fertilizer has been developed during recent years. Recovered nutrients vary depending on technologies. Nearly all nutrients are recovered by nitrification/distillation, while phosphate mineral is the primary nutrients in struvite.24 The loss of nitrogen is prevented by addition of acid or alkali because it decreases urease activity and inhibits urea hydrolysis.25,26 Recovered urea (80–90% of the N in urine) is used to produce the slow-release nitrogen fertilizer which allows effective utilization of nutrients in agricultural production.26
Feces potentially contain a large number of pathogens. The fecal contamination of source separated urine was estimated to be within a range of 1.6–18.5 mg of feces per little of urine, with a mean of 9.1 ± 5.6 mg L−1, thus resulting in about a 5
log lower concentration of pathogens than in feces.6,27 The main risk is from organisms like Mycobacterium tuberculosis that can be excreted in the urine.28
3.1.1 Storage.
Urine is normally not used immediately but stored in containers or tanks. Heterotrophic bacteria grow in fresh urine consuming the nutrients and organic matter.29–31 During storage in a sealed container, urea in urine is enzymatically degraded to ammonia and carbon dioxide, resulting in increased pH to more than 8.8.32 Ammonium and carbonates, which inherently present in excreta matrices, promote inactivation of single-stranded RNA phage by degradation of viral genomic RNA via alkaline transesterification.33
Double-stranded DNA phages, including 28B31,34 and T4,35rhesus rotavirus,34 and Ascaris eggs,36 are the most tolerant surrogates in urine. The estimated storage time to achieve the target LRV is the longest for Ascaris eggs and phages under the physicochemical conditions reported in literatures (Fig. 2(a) and S1†); thus, they were found to be the critical surrogates within those environmental conditions. Pathogen inactivation in stored urine is mainly governed by ammonia and carbonate concentrations, pH, and temperature.31,33,37,38 The storage time for 1 LRV of Ascaris eggs and 5 LRV of phages were determined primarily based on storage temperature; it was approximately 25 d when the temperature was >24.5 °C irrespective of the dilution ratio (Fig. 2(b)). At temperatures >14.5 °C and without dilution, 75 d of storage was sufficient for inactivation, while six months of storage was needed for persistent phages (Fig. 2(b), node 3). This indicates that the WHO recommendation of 6 month storage at >20 °C ensures sufficient inactivation in most cases. However, when urine was diluted with water, the inactivation rate was slower, and the storage time was exceptionally long (Fig. 2(b), node 10), probably because of the lower concentration of biocidal components, including bases, indigenous microbes, and enzymes.35,39 Rotavirus and phage 28B were not inactivated in six months at 5 °C,34 therefore they are not included in the Fig. 2. The storage period should be monitored in a cold region or during winter if external heating equipment is not available. It may not practical to storage urine for such long times at a scale in dense urban environments due to storage volume requirements. Additional treatments are needed to shorten storage time. The strategy for urine storage should also consider the amount of water used for flushing, as it affects the concentration of biocidal components in urine. Additionally, if diluting flush water is decreased during collection the agricultural value increases as less water need to be managed and applied to the fields.
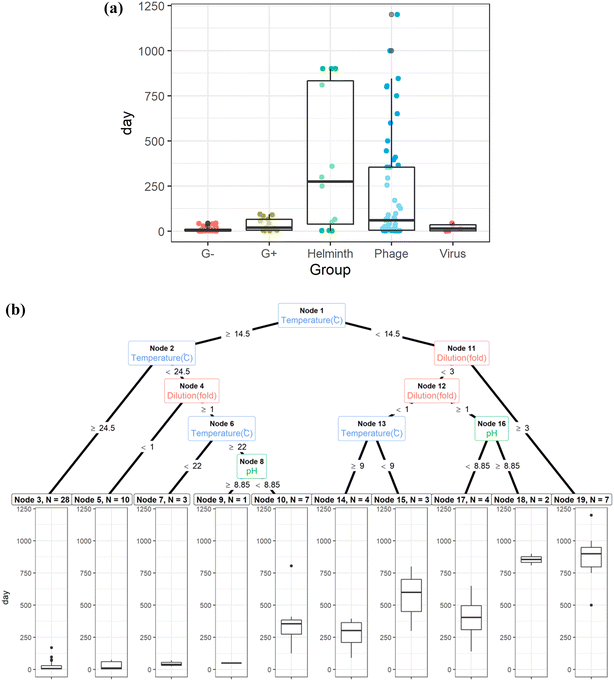 |
| Fig. 2 (a): Storage time of urine to achieve the target log reduction values for Gram-negative bacteria (G−), Gram-positive bacteria (G+), Ascaris eggs (helminth), phages, and viruses.29,31,33,34,36,38,39 (b): Regression tree to determine storage time of urine to achieve the target log reduction values for phages and Ascaris eggs.29,31,33,34,36,38,39 | |
3.1.2 Solar heating and pasteurization.
An increase in temperature enhances pathogen inactivation; therefore, thermophilic storage would help shorten the storage time. Solar heating of urine containers is a relatively simple measure to increase storage temperature. Moreover, solar heating gives the added advantage of ultraviolet radiation when a transparent container is used; the container temperature can be increased to 65 °C in a tropical climate, which promotes pathogen inactivation.40 At thermophilic temperature, bacterial inactivation occurs via damages on metabolic functions, cell membranes, and enzyme activities; while viral inactivation occurs due to the loss in ability to bind with its host cell, which probably caused by structural change in proteins.41,42 Increasing temperature can indirectly facilitate helminth eggs inactivation by increasing the membrane permeability of the lipid layer on the egg shell.43
Although thermophilic conditions are not achieved in colder regions, pathogens are inactivated faster under continuous sunshine, and the required storage time for Ascaris inactivation under sunlight is halved compared to that for storage indoors (light intensity was not reported).44 Sunlight intensity changes with the latitude, which affect the inactivation efficiency of pathogens in stored urine;45 therefore, regional difference in the sunlight intensity need to be considered. Irrespective of the presence of sunlight, the estimated times for the target LRVs were 17 d and one d for Ascaris eggs and Gram-negative bacteria, respectively, while those for bacteriophages MS2, phiX174, and 28B were 41, 185, and 275 d, respectively (Table S1†). The persistence of viruses, phages, and helminth eggs during thermophilic storage has not been reported in the literature. Phages, specifically 28B, phiX174, and T4, could be conservative indicators of urine pasteurization because the storage time required to reach the target LRV is longer than that for helminth eggs. The estimated storage time was longer than two months at temperatures >34 °C without sunlight exposure (outlier in the Fig. 2(b), node 3).
3.1.3 Nitrification and distillation.
All nutrients in hydrolyzed urine are concentrated in a small volume via the process that consists of nitrification and distillation. Nitrification is applied to reduce nitrogen loss through ammonia volatilization, and the stabilized urine is then concentrated by distillation, which enables complete nutrient recovery from source-separated urine.24 The complete nutrient recovery process requires relatively high electrical energy input and investment costs: a specific energy consumption of the distiller is 100 W h L−1 treated urine, while the energy demand for nitrification is about 50 W h L−1 urine.46
Pathogens are inactivated during a 20 day-nitrification at 20 °C and pH 6.05: bacteriophage Qβ is inactivated by 2
log10 by physical (i.e., aeration) process, and Salmonella typhimurium and Enterococcus spp. are inactivated by 1
log10 by biological (i.e., microbial activity) processes.47 However, nitrification is not a stand-alone process for sanitization because of the insufficient removal of pathogens; thus, it cannot be a CCP for monitoring. Pathogens are effectively inactivated during distillation as they are subjected to thermophilic conditions (∼80 °C).24
3.1.4 Membrane technologies.
Membrane technologies such as membrane distillation, nanofiltration, reverse osmosis, and forward osmosis are applied to concentrate source-separated urine on-site. Pathogens are retained in the feed solution as they cannot pass through the membrane filters; the fate of the pathogens could then be determined in the concentrated urine. Forward osmosis technology is advantageous in regards to operational cost and equipment investment in comparison with nanofiltration and reverse osmosis due to the lower hydraulic pressure and energy demand.48
Previous studies have simulated the fate of E. coli and MS2 in a feed solution using concentrated synthetic urine.30,49 MS2 was inactivated more slowly than E. coli, and the time for a 5
log10 inactivation was estimated as 4.8 months and 5 h for 10-folds concentrated non-hydrolyzed and hydrolyzed urine, respectively. Ammonia activity of 10-fold concentrated urine was approximately 1000 mM. Ammonia activity in urine facilitated the inactivation of MS2 better than other parameters such as osmotic pressure and pH,25 suggesting that concentrated urine should be completely sealed to avoid the loss of ammonia during storage. The fate of persistent pathogens, including helminth eggs and DNA phages/viruses, should be investigated in future studies.
3.1.5 Struvite precipitation.
Struvite (MgNH4PO4·6H2O) is a fertilizer obtained in powder-form from source-separated urine (capturing almost all the P and about 5% of the N). Struvite crystals form when the supersaturation in the solution is achieved by magnesium addition, and then they are recovered in a powder form through filtration and drying. The fate of the pathogens during struvite production has been reported in previous studies.50,51 Phage phiX174 and Ascaris eggs were not considerably inactivated during the mixing of influent urine (pH 8.8) and during filtration, while Ascaris eggs and bacteria accumulated on the cake layer during precipitation. In the subsequent 3 day drying at 5–35 °C, the Ascaris eggs and phage phiX174 in the cake were not significantly inactivated.52 A 2
log10 inactivation of Ascaris eggs was achieved in less than one day, whereas three days were needed for a 3.5
log10 inactivation of phiX174 at 36 °C and 35% relative humidity, which indicates that viruses remain infectious for a longer duration in struvite cakes.52 Initial heating prior to drying or drying at higher temperatures could prove effective in inactivating pathogens; however, the temperature should be maintained at 55 °C or less to prevent substantial loss of ammonia. Struvite production can be a CCP if pre-heating of stored urine or post-drying at thermophilic temperatures is employed.
3.1.6 Alkali dehydration.
Alkali dehydration is used to reduce the volume of source-separated urine through drying in alkaline matrices, such as lime and wood ash. Liquid urine is converted into a dry, solid fertilizer. Urease activity is inhibited under alkaline conditions (pH > 10), which prevents the loss of nitrogen due to volatilization of ammonia generated through urea hydrolysis.53
We estimated the required retention time for sanitization using the data extracted from Senecal et al.54 Bacteria and phage were inactivated post-storage in less than three days at 20 °C, whereas the inactivation of Ascaris took longer than five months. The storage time for Ascaris inactivation can be shortened to four days by heating at >42 °C. Thermophilic storage of dried urine is recommended when the prevalence of helminth infection is high.
3.1.7 Ion exchange/sorption.
Sorbents, including charcoal and mineral sorbents, are used for the absorption and ion exchange of nutrients in urine.5 Charcoal, especially activated carbon, has the potential to remove viruses, bacteria, and bacterial spores during water treatment, although the removal efficiency is not always high.55–57 To the best of our knowledge, no studies have been conducted on the fate of pathogens in the sorption and ion exchange processes. Ammonium, phosphate, and potassium ions are recovered by the use of ion exchange resins. Those adsorbed ions are desorbed in solutions containing NaOH, H2SO4, or HCl. Pathogens may be physically adsorbed on the sorbents, however, removal efficiency of viruses by adsorptions is lower than 5 LRVs.58–60 Pathogens can be inactivated in the NaOH desorption solution, while they may not be inactivated in acid desorption solutions. The fate of pathogens in generated solutions and sorbents need to be investigated.
3.2 Fecal sludge
Urine contains most nitrogen and about half of phosphorus and potassium in human excreta, while feces are rich in phosphorus and potassium, and contain most carbon excreted from the body. Fecal sludge is the fecal waste stored within onsite sanitation systems. In addition to fresh feces, it includes everything that go into toilet pit, including urine, anal cleaning materials, greywater, and municipal solid waste.61 Although the physicochemical characteristics are different from stored feces, fecal sludge also contain organic matter and nutrients; therefore, it is used as combined soil amendments and fertilizers. The concentrations of nitrogen and phosphorus are 10–100 times and 2–50 times higher than those of domestic wastewater.61 The available nutrients are affected by the type of toilet pit and treatment technologies, for example, treatment takes place in an open system is prone to lose nitrogen through volatilization and leaching.
Untreated fecal sludge has a high oxygen demand due to the presence of readily degradable organic matter. Stabilization process is recommended to avoid depletion of oxygen in farmlands. In a centralized system, fecal sludge is collected from a single pit, transported to a (semi-)centralized treatment facility, and treated before its application in farmlands.62
3.2.1 Storage.
Some containment technologies, including bucket latrines, pit latrines, and septic tanks, are not designed for resource recovery, but fecal sludge collected from them can be applied for resource recovery if it is collected and transported accordingly.63 Pathogens are affected by biocidal components inherent in human excreta matrices along with external environmental factors and are therefore inactivated during storage, which is often called self-sanitization or natural inactivation.
The estimated storage time was longest for phages and Ascaris eggs under the physicochemical conditions reported in the literature (Fig. 3(a) and S2†). Ascaris eggs can remain viable for more than one year at temperatures below 22.5 °C (Fig. 3(b)), whereas in a warmer climate with lower moisture content (<26%) and with the use of desiccants, they can be inactivated within 1.5 months or less (Table S2†).
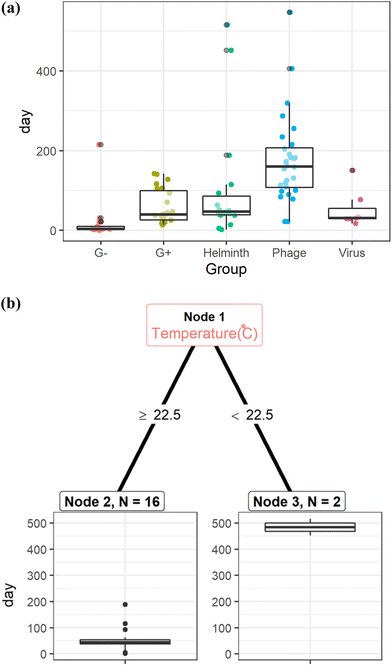 |
| Fig. 3 (a): Storage time of fecal sludge to achieve the target log reduction values for Gram-negative bacteria (G−), Gram-positive bacteria (G+), Ascaris eggs (helminth), phages, and viruses.81–83,144,145 (b): Regression tree to determine storage time of fecal sludge to achieve 4 log reduction of Ascaris eggs.81–83,144 | |
Chemical or physical treatments such as ash/lime, pasteurization, and solar drying are commonly used to shorten storage time. A pH > 9 has been recommended by WHO,7 but the target LRV for MS2 and phiX174 may not be achieved in six months at pH > 9 (Table S2†). Recent studies have suggested that the matrices pH should be maintained above ten along with the presence of ammonia for virus and bacterial inactivation.14,64 Complete sealing of the container is effective in reducing the volatilization of ammonia and the inactivation of pathogens. The pH and ammonia concentration need to be high enough for hampering the biological activity otherwise it may lead to accumulation of harmful gasses such as methane and sulfur dioxide; this further leads to oxygen deficiency. Sanitation workers are at a high risk of exposure to harmful gases and anoxic conditions when they empty a pit.65 Inhalation of harmful gases under anoxia must be prevented by providing active ventilation or should be monitored using an oximeter. Trade-offs in chemical and microbial risk should be addressed.
Although storage is a potential CCP, the sanitization efficacy is mainly affected by storage time, temperature, and the use of additives, which is different by the type of containers. The risk of exposure to pathogens is determined by the frequency of collection/emptying of containers, and the required LRVs can be changed by the type of containers and the collection schemes of containers, consequently. The biocidal factors in fecal sludge are common in container storage, while controllable biocidal factors vary depending on the structure and the type of containers. Thus, we have reviewed pathogen inactivation during storage in different types of containers in the following sections.
(1) Bucket latrine and container.
A bucket latrine consists of a pedestal or seat drop hole with a bucket or pan (25–30 L) placed in a chamber below. When the bucket is full, it is manually removed and emptied66 (Fig. S3†). The installation of bucket latrines should not be promoted because of the significant infection risk through vector breeding, human contact while emptying and washing a bucket, and overflow due to inadequate regular emptying.66 Container-based sanitation (CBS) involves the use of a sealable container placed under a pedestal and a collection and transportation system for containers; it is a promising option for resource recovery in a densely populated area.67 When urine-diverting toilets are used for households in a densely populated area, a CBS system realizes off-site resource recovery from the collected urine and feces.
The pathogen inactivation rate in a container depends on the storage time without the application of chemical/physical disinfection. Significant inactivation can occur when stored buckets are heated from being directly exposed to sunlight.66 Chemical disinfectants are used where bucket-based sanitization of human excreta matrices is needed, specifically in emergency situations such as infectious disease outbreaks in evacuation shelters. Lime-based sanitization with calcium hydroxide resulted in approximately 5
log10 inactivation of fecal coliforms, intestinal enterococci, somatic coliphage, F+-specific phages, and Bacteroides fragilis phages in 30 min at a pH > 12,68 while nematodes are not inactivated during this short treatment.69 Because of the limited storage capacity and consequent shorter storage time compare to pit latrines, significant inactivation of pathogens is not achieved. Treatment in buckets and container can be a CCP if additional onsite sanitization is combined.
(2) Single pit latrine.
A single pit latrine is a shaft dug below the ground level, which is either lined with reinforcing materials or left unlined.66 A single ventilated improved pit (VIP) latrine is associated with a ventilation pipe that vents odor and acts as a trap for flies (Fig. S4†). The potential for fly breeding is reduced by a fly mesh at the ventilation pipe, the use of a toilet cover, and frequent addition of bulking materials or ash to reduce the possibility of flies encountering fresh fecal material. Excreta, along with anal cleaning materials (water or solids), were deposited in a pit. Depending on how deep they are dug, some pits may last for more than 20 years without emptying, which allows greater inactivation of pathogens during longer storage than bucket latrines and CBS systems.70 However, in a dense area, pit emptying is periodically needed owing to the high rate of pit filling and space limitations. Improper emptying is associated with a significant risk of infection among sanitation workers.71
Pathogen survival in a pit latrine is affected by temperature, moisture content, oxygen availability, and presence of indigenous microorganisms.72 Uncharged ammonia has a biocidal effect; however, the sludge in a pit latrine has neutral pH, which is more conduction for ionized ammonia than uncharged ammonia.73 Additionally the design with good ventilation results in losses of the formed ammonia to ambient air. The temperature in a pit latrine changes when there is a change in ambient temperature.74 Because the temperature within a pit latrine is not commonly controlled, the best monitoring parameter is the storage time.
(3) Septic tank.
A septic tank is a watertight chamber made of concrete, fiberglass, polyvinyl chloride, or plastic through which blackwater and greywater flow for primary treatment in one to three chambers (Fig. S5†).62 The treatment performance is determined by maintaining an appropriate hydraulic residence time for the solid–liquid separation of wastewater. The effluent from the final chamber is further treated using a subsurface drain field or other additional processes.
The mechanisms for the reduction of pathogens in a septic tank include settling with sludge and natural inactivation. The efficacy of pathogen reduction in a septic tank varies depending on the type of pathogen and is affected by the temperature and retention time.75 Predation by heterotrophic microorganisms, interspecific competition for nutrients and reduced red-ox potential are also involved in pathogen inactivation.76,77 The inactivation efficiency of pathogens in a septic tank is usually low; therefore, high levels of pathogens remain in both the effluent and sludge. Helminth eggs are removed from the liquid phase by gravity settling because they are sufficiently large for settling to be effective, but the reduction is usually less than 0.5
log10.6
(4) Twin pits for pour flush toilet (wet pit).
The twin pits for pour flush toilet consists of two pits connected to a pour flush toilet (Fig. S6†). Unlike the double VIP and Fossa-Alterna which are described in the latter section, this toilet allows the use of water and does not require addition of organic materials. One pit is used while the fecal contents in another pit is stabilized.66 Because fecal sludge has undergone significant dewatering and degradation, further treatment in a semi-centralized treatment facility is not required. After one year of storage in a pit, significant inactivation of viruses, bacteria, and protozoa is achieved, but Ascaris eggs may still be viable.78 Fecal sludge is transformed into nutrient-rich humus that is safe to excavate after storage for more than two years.66 There is a higher risk of groundwater contamination due to more leachate compared to waterless toilets.
(5) Double alternating dry pit (dry pit).
Consisting of two pits, a double alternating dry pit allows at least 1.5 to 2 years resting time of the pit contents while another pit is used (Fig. S7†). The excreta in the pit is transformed into nutrient-rich humus. Fossa-Alterna, an alternating dry pit, requires the addition of leaves and soils following each defecation to introduce microorganisms that assist in the stabilization of the excreta. Owing to enhanced biological degradation, sludge in the pit is transformed into an earth-like material that is used as a nutrient-rich soil conditioner (mainly P as most of the N is lost during storage) after one year of storage.62
Pathogen inactivation and other important factors are similar to double dehydration vaults, which are described in “(6) Double dehydration vaults (double desiccating toilet)” in the present section. The distinctive feature of the double alternating dry pit is that urine is not stored separately, which could result in a high concentration of ammonia in the pit. The presence of indigenous microorganisms also affects pathogen inactivation in pits. Vector attraction and odor are significantly reduced by installing ventilation pipes compared with non-ventilated toilets. The constant addition of ash, lime, soil, or sawdust further reduces the moisture content, minimizes odors, and provides a physical barrier between the excreta and vectors. The addition of alkali materials, such as ash and lime, promotes pathogen inactivation and dehydration.
Viruses, bacteria, and helminth eggs survive in pits for several months under mesophilic conditions.79 WHO recommends the common practice of double alternating dry pits and double dehydration vaults in the SSP. A storage time of 1.5–2 years at ambient temperature (2–20 °C) is recommended in areas with a high prevalence of helminth infections.6 The recommended storage time can be shortened to one year in tropical climates with ambient temperatures higher than 20–35 °C.6 A prolonged storage time of 18 months is recommended for highland subtropical areas (17–20 °C).66
When subsequent solar drying is used, the storage time can further be reduced to 8–10 months.66 When the pit content was maintained at pH > 9, the storage time was shortened to six months.6 Recent studies have suggested that the pH of the matrices should be maintained above ten in the presence of ammonia for effective inactivation of virus and bacteria.14,64
(6) Double dehydration vaults (double desiccating toilet).
A double dehydration vault, which is associated with a urine-diverting dry toilet, generates dehydrated feces that can be used as a soil conditioner (Fig. S8†). Commonly used additives include ash, dry soil or sand, dry leaves, dry grass, rice husks, lime, crushed oyster shells, and shea nut shells, all of which contribute to the essential attributes of generating dehydrated fecal sludge that is bulky and dry.80
Pathogen inactivation through urea amendment has been evaluated in source-separated feces.36,81–83 Inactivation of Ascaris eggs is faster in feces amended with a mixture of ash and urea than that with a single addition of ash or urea.36 Cruz et al.81 demonstrated that greater inactivation of Ascaris eggs was achieved with a lower moisture content (27.5%). When 1–2% dirt and urea were added to feces, viable Ascaris eggs were reduced by 2
log10 in 14 d at 28 °C, whereas the eggs were viable when feces was stored without the addition of urea. A higher storage temperature further promoted the inactivation of Ascaris eggs,36,81Enterococcus spp., Salmonella spp., and phage 28B, while the inactivation rate is not linear with temperature.83
3.2.2 Alkali sanitization.
Ash and lime (limestone, slaked lime, and burnt lime) are common additives that have been applied to a variety of sludges, including sewage sludge, dehydrated feces, or humus, and finished compost. Alkali additives are used to minimize the potential for odor generation, inactivate pathogens, and reduce the material's vector attraction potential.84 Common desiccants applied to dehydration vaults and desiccation toilets including wood ash (pH: 9.4 to 11.3), rice husks (pH 10.6), and lime (pH: 10.3 to 12.4) have a basic pH.80 The application of alkali treated fecal sludge is effective to neutralize acid soil. The increase of pH of the soil amended with slaked lime alters microbial community structure and the enzymatic activity, which facilitates nitrogen mineralization in the soil.85,86 The nitrates-uptake capacity of a plant should be considered to prevent surface and groundwater contamination.
Alkaline-treatment of compost facilitates inactivation of E. coli via the damage to the outer membrane and enzyme activities, while it leads to the loss of infectivity of coliphage via the partial capsid damage and RNA exteriorization.87 In addition to the single effect of increasing the pH, alkali additives change the ratio of indigenous biocidal components, including ammonia and carbonate, which enhances the biocidal effect of ammonia inherent in excreta matrices.
The effective lime dosage varies from 1% to 35%, and it elevates the pH from 8.0 to 12.9.68,69,87–89 The estimated storage time for inactivation of phage MS2 was several hours (Table S3†), while it took more than six months for inactivation without desiccants (Table S2†); this indicated that lime treatment is a promising measure for virus inactivation. The estimated time for inactivation of Ascaris eggs was three weeks in the presence of approximately 500 mM NH3, but it took more than two years with 50 mM NH3, which suggests that alkaline pH alone has a limited effect on helminth inactivation, as shown by Senecal et al. with no reduction at all in ammonia free pH 12.4 environment.90 The tailing-off profiles resulted in exceptionally longer estimates (more than two years for Ascaris, two months for E. coli, and 26 d for MS2) (Table S3†).
It should be noted that the estimates do not include datasets obtained at lower temperatures (<20 °C) (Table S3†), although surrogates are found to survive for several years at lower temperatures.91 Future studies should evaluate the effects of alkali additives in cold regions. Lime treatment should be designed to inactivate Ascaris spp., which survives the longest under alkaline environments; when Ascaris is not an organism of concern, a pH > 10 is sufficient for the inactivation of viruses and bacteria.64 Viruses and phages are rapidly inactivated at 4−28 °C. However, the virucidal effect of lime is yet to be fully understood owing to the lack of data for phages and viruses.64 Future studies on lime treatment should characterize the inactivation of ssRNA and non-ssRNA phages and assess whether phages are representative of the inactivation of pathogenic mammalian viruses. By using burned lime, CaO, the oxidation reaction is very strong and releases heat together with increased pH. During emergency sanitation, this can be an alternative. However, the high reactivity poses a health risk to the workers mixing in the lime.
3.2.3 Ammonia/urea sanitization.
Ammonia/urea sanitization, such as Peepoo bags, is applied at the individual, household, and city levels.92 When the use of synthetic urea and ammonia solutions as additives in urine-diverting toilets is not economically feasible, stored human urine may be a potential alternative. An ammonia solution and urea pellet take advantage of the long-lasting sanitization effect in high organic-loaded matrices and the addition of fertilizer value. Urea is hydrolyzed to ammonia and carbonate by urease-producing bacteria, which exist ubiquitously in the surrounding environment. The pH of the matrices increases with the production of ammonia, and at pH greater than 9.25, which is the pKa of ammonia (25 °C), the fraction of uncharged ammonium becomes dominant in the equilibrium of ionized ammonium.
Kohn et al.64 reviewed the inactivation profiles of surrogates and proposed treatment recommendations for ammonia sanitization. The recommended storage times are six months (T < 20 °C), two months (T < 30 °C), and 15 days (T > 30 °C) with 1.5% urea addition (corresponding to 0.75% NH3) at pH > 8.8.64
Treated fecal sludge is used as a quick-release nitrogen fertilizer which has nitrogen as a form being immediately available to plant roots. Oversupply of quick-release fertilizers to farmland leads to nitrates pollution of surface and groundwater due to the lower nitrates uptake efficiency by plants, and consequent washing out of the root zone of plants.93 Direct application of treated fecal sludge should be designed to prevent contamination of drinking water source with nitrates.
3.2.4 Solar drying and pasteurization.
Solar drying is widely applicable for the stabilization of solid waste including feces, excreta, compost, and fecal sludge; it can be used for an onsite or a centralized treatment (e.g., drying bed). An unplanted or planted drying bed is used for volume reduction of fecal sludge collected from a community-level facility, such as pit latrines and septic tanks.62 Pathogen inactivation in a sludge drying bed has been summarized in a previous study.66 There was a reduction in the total number of pathogens during the drying process, but the concentration of pathogens increased with a reduction in the volume of the sludge. Although solar drying on a drying bed is effective in reducing sludge volume, sufficient degradation of organic matter and pathogen inactivation were not achieved during the 15 day drying period.62 Further treatments such as storage and composting were recommended since the increased dry matter content result in longer survival of many microorganisms mainly due to decreased heat transfer capacity in dried substrate.94
Irrespective of the presence of sunlight, the target LRVs for bacteria and helminth eggs were achieved within four days at a thermophilic temperature (50–75 °C), but it should be prolonged at a mesophilic temperature; the estimate for Ascaris inactivation is eight months at 31 °C (Table S4†). Helminth eggs are appropriate indicator microorganisms for solar drying. Future studies should include inactivation of phages and viruses at mesophilic temperatures with exposure to sunlight.
3.2.5 Composting.
Composting is primarily an aerobic (and sometimes anaerobic) microbiological decomposition process that stabilizes organic matter to odorless substances.80 Increasing the temperature to >40 °C in the thermophilic stage effectively inactivated pathogens. Even if a thermophilic temperature is not achieved, a small change in temperature in the mesophilic range facilitates the inactivation of Ascaris eggs.95 Pathogen inactivation in a composting chamber primarily relies on the heat generated by aerobic biological reactions. Thus, maintaining optimum conditions for biological reactions is important for sanitization. Alkaline additives are used to enhance pathogen inactivation; however, an excessively high pH in a composting toilet may limit the overall biological reactions occurring within the pile of excreta to sufficiently raise the temperature.80
Under optimum conditions for composting with organic waste, the temperature of the chamber reached approximately 65 °C over several days, and the thermophilic condition was maintained for several weeks. The estimated composting duration for sufficient inactivation of E. coli and Enterococcus spp. is less than two weeks, as long as the thermophilic temperature of 50–60 °C is maintained.96 Bacterial regrowth can occur when the chamber temperature is <50 °C.96 The fate of coliphages (T4, λ, Qβ and MS2) in sawdust was investigated under various matrix temperatures and moisture content.97 The estimated time for T4 inactivation was 126 d at 30 °C and 15 d at 50 °C, while that for Qβ inactivation was 15 d at 30 °C and three days at 50 °C. Helminth eggs and Salmonella can survive longer than phages under thermophilic conditions. Ascaris eggs were completely inactivated under an 8 week co-composting as long as the thermophilic temperature (50.7–58.7 °C) was maintained for 31 d, and pile turning was conducted every seven days.98 Based on the modeling study by Vinnerås et al., Salmonella is considerably more resistant to temperature compared to Ascaris.99
A composting latrine chamber was originally designed for a 6 month storage period to achieve adequate pathogen removal. However, it is now recommended to increase the storage time to 1.5–2 years,100 because composting chambers in the field were unable to reach a high enough temperature for the inactivation of resistant pathogens such as helminths.101
3.2.6 Vermicomposting.
Vermicomposting is a mesophilic (<35 °C) waste oxidation and stabilization process in which earthworms and diverse microbial communities jointly process organic waste, including source-separated human feces, fecal sludge, and human fecal slurry under aerobic conditions.102,103 Vermicomposting is available on an industrial scale for centralized treatment and on a toilet chamber scale for decentralized treatment. The earthworms function in synergy with bacterial communities within a “vermi-filter”, which treats diluted domestic wastewater sludge in a system inoculated with earthworms.104
Pathogen inactivation is achieved by the intestinal digestive action of earthworms as well as intestinal enzymes and antibacterial coelomic fluids excreted by earthworms.103 Pathogen inactivation is indirectly promoted by enhanced aeration by the burrowing activity of earthworms, which favors other microbial communities that compete for available nutrients by engaging in antagonism and phagocytic activity, as well as in the production of humic acids.103 Vermicomposting cannot be performed at thermophilic temperatures of composting. Temperature is an important factor for worm activities.
Previous studies have investigated the reduction in pathogens during vermicomposting. More than 2
log10 removal of coliforms and 1.6
log10 removal of E. coli were achieved after 60 d of vermicomposting.105,106 Viable Ascaris eggs were not reduced after 90 d of vermicomposting, whereas helminths, coliforms, and Salmonella were inactivated after 180 d.107 Inactivation of thermotolerant coliforms, Salmonella spp., Enterococcus spp., and bacteriophage phiX174 is limited to 1.5
log10 to 2
log10.108 However, further treatment (e.g., storage for longer than one year) is necessary to meet the requirements of the 2006 WHO guidelines.14,108 Vermicomposting is not a stand-alone process for sanitization because of the insufficient removal of pathogens; thus, it cannot be a CCP for monitoring.
Pathogen inactivation is affected by stocking density of worms and the pH of the matrices. Optimum stocking density of earthworms (2.0–4.0 kg m−2) should be maintained for pathogen inactivation.103 A greater stocking density beyond a certain level led to overcrowding of earthworms, which resulted in a decline in waste processing efficiency and consequently affected pathogen inactivation. In vermicomposting source-separated human feces, inactivation of Enterococcus spp. and thermotolerant coliforms is affected by pH. The compost pH is also correlated with the maturity index; thus, the maturity of vermicompost is involved in bacterial inactivation.108 Meanwhile, for coliphage inactivation, the time since last maintenance appears to be more important than pH and ammonia concentration.108 The pH of vermicompost and composting duration should be the monitoring parameters in vermicomposting.
3.2.7 Black soldier fly composting.
Black soldier fly (BSF) (Hermetia illucens) larvae grow by consuming organic waste, including animal manure and fecal sludge, which results in up to a 75% volume reduction of organic waste, together with nutrient removal such as nitrogen and phosphorus.109 The residual fecal sludge after the BSF larval feed must be composted or anaerobically digested to produce a soil conditioner.110
Adenovirus, reovirus, and enterovirus (106 TCID50 g−1) were inactivated to below the detection limit in two weeks, and more than 6 log10 inactivation of Salmonella spp. was achieved in seven weeks, whereas, Enterococcus spp. and Ascaris suum eggs were not reduced at eight weeks.111 Pathogen inactivation in BSF composting is mainly time-dependent, through natural inactivation at an ambient temperature, although adsorption onto particles and ammonia inactivation occur. Retention time should be an appropriate monitoring parameter in a BSF composting system. BSF composting is not a stand-alone process for sanitization; thus, it cannot be a CCP. Additional sanitization is needed, especially where helminth infection is a main concern.
3.2.8 Lactic acid fermentation.
Lactic acid fermentation (LAF) is a biological anaerobic process. The advantage of LAF in human excreta is its ability to prevent nitrogen loss and inhibit the production of malodorous compounds, which is attributable to suppressed protein degradation during fermentation. LAF is a fundamental process in the terra preta sanitation system, in which human excreta are converted into terra preta-like soils and subsequently used in agriculture.112
LAF does not induce a temperature increase; fermentation temperature is usually below 36 °C. Pathogen inactivation through LAF is attributed to the acidification caused by the production of lactic acid as well as the release of other compounds with suppressive effects on pathogens such as bacteriocins, glucose oxidase, and hydrogen peroxide.113 Inactivation of E. coli in a week varied from 0.1 log10 to 4.4
log10 in previous studies;114,115 this is probably due to fluctuations in the activity of lactic acid bacteria (LAB), which is affected by the type of carbon source (i.e., molasses or biochar). The pathogen inactivation rate fluctuates among experiments conducted in a narrow range of acidic pH (pH 3.7–3.9) and even within a single study.116–118 Till date, appropriate monitoring parameters for LAF have not been identified. The microbial activity of LAB is relevant to the performance of pathogen inactivation; therefore, parameters that reflect the activity of LAB can be used as monitoring parameters.
3.2.9 Anaerobic digestion.
Digestate or slurry generated through anaerobic digestion is used as fertilizer or soil conditioner. The determinant factors for pathogen inactivation are the operating temperature (i.e., thermophilic digestion at 50–60 °C or mesophilic digestion at 30–38 °C), hydraulic retention time, pH, and volatile fatty acids.66 Without a thermophilic process, the hydraulic retention time should be monitored. Fully mixed systems give short minimal retention time; therefore, these systems require additional sanitation steps even at thermophilic temperature.
At a moderate temperature (28 °C), more than one year of storage is needed for 1
log10 inactivation of Campylobacter jejuni, whereas E. coli and Salmonella are inactivated at 77 and 35 d, respectively.119,120 At mesophilic temperatures (35−37 °C), the estimated storage time was the longest for DNA phages (phiX174 and Bacteroides fragilis phage B40-8), whereas the estimates for viruses (murine norovirus 1 and human adenovirus) were less than three months (Fig. 4 and Table S5†). Ascaris eggs are not inactivated at temperatures below 36 °C without uncharged ammonium.95,121 At a thermophilic temperature, the estimated storage time was significantly shortened to <1 d for bacteria and Ascaris eggs and 30 d for murine norovirus 1 (Fig. 4 and Table S5†). Plug flow or batch reactors reaching a considerably lower red-ox potential in the material have better reduction even at ambient treatment temperature compared to fully mixed systems.77 When a longer retention time is not feasible due to the size constraint of the reactor, anaerobic digestion cannot be a CCP. A promising post-treatment is pasteurization. In Sweden, heating at >70 °C for 1 h is required for digestate produced from biogas plants.122 Conservative treatment conditions should be designed to inactivate double-stranded DNA phages.
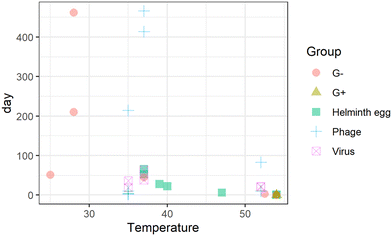 |
| Fig. 4 Estimated retention time to achieve the target inactivation units of Gram-negative bacteria (G−), Gram-positive bacteria (G+), Ascaris eggs (helminth), phages, and viruses in anaerobic digestion under various temperatures.95,119,146–150 | |
3.2.10 Drying.
The latrine dehydration and pasteurization (LaDePa) pelletizer is a sludge-drying and pasteurization technology capable of producing a dry, pelletized soil amender in a pit latrine at a rate of approximately 1000 kg per hour.62 The entire process of sludge drying and pasteurization was completed in 16 min. When heated above 100 °C, pathogens are completely inactivated during pasteurization. After processing at >200 °C, Ascaris eggs (135 eggs per g-TS) were completely inactivated.123
3.2.11 Microwaving.
Microwave-based technology has been developed for slums and emergence situations where latrines are rapidly filled up, creating the need for efficient treatment of fecal sludge. The sanitization performance was evaluated over a relatively short operating times; for 100 g of fecal sludge, 3 min at 465 W (23 W h) compared to 1 min at 1550 W (26 W h).124,125
Pathogen inactivation occurs owing to the nonthermal (electromagnetic radiation) and thermal effects of electromagnetic energy.126 Microwave-based technologies can significantly inactivate bacteria and helminth eggs within a few minutes.124,126 The mechanisms of bacterial inactivation has been well summarized by Afolabi and Sohail.127 Both the thermal and athermal effects triggered by ionic conduction/migration are involved in sanitization of fecal sludge. Inactivation of bacteria is initiated from the rupture of cell membranes which has a strong affinity for microwave absorption, and subsequently leads to rupturing of the cell walls and release of intracellular materials. The bacterial inactivation level is determined by the input microwave power level, amount of fecal sludge treated, and operating time.124 Operating conditions should be designed to increase the amount of sludge input. A holding time of at least 3 min is recommended at the lethal temperature of the pathogens.124
4. Discussion and outlook
4.1 Potential critical control points
This study reviewed the sanitization potential of resource recovery technologies by comparing the storage time required to achieve certain LRVs. The potential CCPs and monitoring parameter candidates are summarized in the Table 2. We identified appropriate monitoring parameters for the respective technologies based on simplicity and feasibility of real-time monitoring, and on the contribution to pathogen inactivation.
Table 2 Potential of sanitization, feasible monitoring parameters, and indicator microorganisms in a technology for resource recovery from (A) urine and (B) fecal sludge
(A) |
Technologies for urine |
Sanitization potential |
Indicator microorganisms |
Monitoring parameters |
Controllable parameters |
Storage |
Moderate, depending on storage temperature and dilution rate |
Phages and helminth eggs |
Storage time, which can be reduced at >24.5 °C; temperature; dilution ratio; NH3 concentration |
Dilution ratio, storage time, temperature when heating devices are available |
Solar heating and pasteurization |
High, depending on storage temperature |
Phages |
Storage time and temperature. Bacterial inactivation can be achieved in 1 day at >45 °C |
Dilution ratio, storage time, temperature when heating devices are available |
Nitrification and distillation |
Low in nitrification, but high in distillation |
|
|
|
Membrane technologies |
High for hydrolyzed urine, but low when urea-hydrolysis is inhibited |
Phages |
Storage time and NH3 concentration for a ssRNA phage. Storage for 1 day causes enough inactivation of Gram-negative bacteria and a ssRNA phage |
Storage time |
Struvite precipitation |
Low. Initial heating prior to drying or drying at higher temperature could be effective |
Phages. DNA viruses/phages should be investigated in future studies |
Drying temperature |
Storage time, moisture content and drying temperature (<55 °C) |
Alkali dehydration |
High, but moderate for helminth eggs |
Helminth eggs |
Temperature and storage time |
Storage time and drying temperature. Post-storage for 4 d at >42 °C is recommended for helminth inactivation |
Ion exchange/sorption |
No studies |
|
|
|
(B) |
Technologies for fecal sludge |
Sanitization potential |
Indicator microorganisms |
Monitoring parameters |
Controllable parameters |
Storage |
Moderate, depending on storage temperature, NH3 concentration and use of desiccants |
Phages and helminth eggs |
Storage time; temperature (a critical value is 22.5 °C for helminth inactivation); pH (>10); and NH3 concentration |
For bucket latrines and container, storage time, temperature, pH, ammonia, moisture content; for single pit latrine and double pit latrine, storage time, pH, ammonia, moisture content; for septic tank, storage time; for twin pits for pour flush toilet, storage time; for double alternating dry pit, storage time, pH, ammonia, moisture content; for double dehydration vaults, storage time, pH, ammonia, moisture content |
Alkali sanitization |
High. Bacteria regrowth should be mitigated by overdosing |
Helminth eggs and phages |
Storage time (several hours to 3 d, 3 weeks for helminth eggs), pH (>10); and NH3 concentration |
Storage time and pH |
Ammonia/urea sanitization |
Moderate, depending on storage temperature |
Helminth eggs and non-ssRNA viruses |
Storage time, 6 months (T < 20 °C), 2 months (T < 30 °C), and 0.5 month (T > 30 °C); temperature; NH3 concentration; pH (>8.8) |
Storage time and NH3 concentration |
Solar drying and pasteurization |
High, depending on storage temperature |
Helminth eggs |
Temperature and storage time (5 d at >40 °C) |
Storage time and temperature |
Composting |
Moderate, depending on operating temperature |
Helminth eggs and Salmonella |
Operating time, temperature, C/N ratio and aeration. 8 weeks composting with 1 month at thermophilic condition (50.7–58.7 °C). Post-storage for 1.5–2 years is recommended for incomplete composting |
Retention time, C/N ratio and moisture content |
Vermicomposting |
Low. Need post-treatment |
Helminth eggs and phages |
Stocking density, pH, composting duration |
Stocking density, composting duration |
Black soldier fly composting |
Low. Need post-treatment |
Helminth eggs |
Retention time |
|
Lactic acid fermentation |
Uncertain |
|
|
|
Anaerobic digestion |
High in thermophilic digestion, moderate in mesophilic digestion |
Phages |
Temperature and retention time (30 d in thermophilic digestion at >52 °C) |
Retention time |
Drying |
High |
|
Heating time, temperature |
Heating time, temperature |
Microwaving |
High |
|
Microwave power level, the amount of fecal sludge treated and operating time |
Microwave power level, the amount of fecal sludge treated and operating time |
Regression tree models allowed to identify monitoring parameters and to estimate the storage time according to storage conditions. The requisite storage time for pathogen inactivation in urine and fecal sludge was determined mainly by temperature, and the CLs of temperature were 14.5 °C and 22.5 °C, respectively (Fig. 2(b) and 3(b)). Sanitization is achieved faster in thermal processes such as solar heating, pasteurization, drying, composting, microwaving, and thermophilic digestion (Table 2). Temperature can be a promising monitoring parameter; thus, appropriate CLs of temperature should be provided for each process. Persistence and important biocidal factors varied among the types of pathogens; therefore, the monitoring parameter and its CL should be determined using an appropriate surrogate for the pathogen that causes local endemic infectious diseases. Future studies should investigate the inactivation of phages, mammalian viruses, and helminth eggs in a wide range of temperatures and environmental conditions (Table 3).
Table 3 Knowledge gaps and research priorities in the fate of pathogens
Technologies |
Types of microorganism and conditions to be examined |
For urine |
Solar heating and pasteurization |
Non-ssRNA phages and mammalian viruses, in mesophilic and thermophilic temperatures, with exposure to sunlight |
Membrane technology |
Helminth eggs, non-ssRNA phages and mammalian viruses, in various temperature conditions |
Ion exchange/sorption |
Various types of microorganisms in various temperature conditions |
For fecal sludge |
Alkali sanitization |
Various types of phages and mammalian viruses, in various temperature conditions |
Solar drying and pasteurization |
Various types of phages and mammalian viruses, in a mesophilic temperature, with exposure to sunlight |
Lactic acid fermentation |
Helminth eggs, various types of phages and mammalian viruses in a mesophilic temperature |
Sufficient inactivation does not always occur in a single treatment process; however, it is achieved by combination of treatment processes. Thermophilic conditions are not always present in the composting reactors. Pre-stabilization by LAF before composting realizes sanitization and stabilization in one month. The use of easily soluble carbohydrates (e.g., molasses) contributes to maintaining a thermophilic condition (>55 °C) without any turning or mixing for 9 d, which leads to the elimination of most of the pathogens.118 When a thermophilic condition is not achieved during the composting reaction, a 3 months storage of the finished compost79 or secondary composting at >50 °C for at least one week7 is recommended. The addition of lime and urea, or the application of direct sunlight can be used as post-treatment. Solar drying and heat treatment are promising measures for the inactivation of helminth eggs: a 4
log10 inactivation of Ascaris eggs is achieved within a day by raising the matrix temperature to 50 °C (ref. 128) and 75 °C.129 Each process is monitored as CCPs if CLs at each process are determined so that target LRVs is achieved in a whole process train.
4.2 Needs of the inactivation kinetics model
The efficacy of pathogen inactivation is affected by the surrounding environmental conditions, including climatic factors and physicochemical properties of the matrices. Therefore, the storage times required to achieve a certain LRV, inactivation rate, and decimal reduction time are not comparable when the properties of the matrices and operating conditions vary within and among studies. Arriving at general conclusions regarding the sanitization potential of an individual technology and comparing it across the technologies is difficult because the experimental conditions are not comparable within or among studies.
The target LRVs were set based on the risk assessment in the 2006 WHO guidelines. The WHO determines the target LRVs aiming at 10−6 disability adjusted life years (DALYs), but it can be changed under a different exposure scenario, and the estimated storage time is changed accordingly.130 In addition, the target LRVs were reduced when we aim at 10−4 DALYs, which is a cost-effective target proposed by Mara.131 With the relaxed requirements for LRVs, the technologies we evaluated as “low” can be a stand-alone process for sanitization. Therefore, evaluations in the Table 2 are reliable for the predefined specific target LRVs in this study.
An inactivation kinetics model that represents the LRV as a function of storage time and chemical or physical indicators is used to estimate LRVs at a certain storage time and matrix conditions.9 The inactivation kinetics model is also used to evaluate the sanitization potential across resource-recovery technologies under specific conditions.9 When the inactivation profile is defined as a function of treatment/storage time, estimates of storage time are affected by the inactivation profiles, that is, straight decay, tailing, shouldering, or combinations. A tailing-off profile derives estimates of a much longer storage duration compared to a first-order decay and shouldering profile. The shape of the kinetic curve and mechanisms behind the profile should be identified, especially when pathogens remain infective for more than several months. Daily fluctuations in the properties of the matrices affect the inactivation kinetics parameters and can significantly affect the prediction of microorganisms that survive for more than several months. Future studies should develop an inactivation kinetics model that considers the temporal changes in environmental conditions over a longer storage period.
4.3 Appropriate surrogate microorganisms
We focused on the inactivation of viruses, phages, bacteria, and helminth eggs, as they have often been used in disinfection experiments. Helminth eggs, which survived the longest among the reviewed microorganisms, were effectively inactivated in treatments that created a thermal environment, including composting, thermophilic anaerobic digestion, drying, and pasteurization. Bacterial spores such as Bacillus cereus and Clostridium perfringens are extremely resistant to thermal treatment;132 however, we did not consider them because they are not pathogens. ISO 30500 provides restrictions on the number of viable spores in products from sanitation systems.12
The target LRVs were highest for phages and viruses because enteric viruses are excreted in feces in relatively high numbers and survive in soil and aquatic environments for a long period.133 Among the reviewed technologies, phages, specifically double-stranded DNA phages, are conservative surrogates for determining CLs in a CCP. Nevertheless, the number of reports on virus inactivation is still smaller than that on bacteria and helminth eggs. Future studies are needed to investigate the inactivation of viruses or phages, and to assess whether they are conservative indicators compared to helminths and bacteria.
Bacteriophages are often used as surrogates for mammalian viruses because they are considerably easier to cultivate. Variability in tolerance among viruses and bacteriophages can be explained by the form of the viral genome (i.e., single-stranded RNA, double-stranded RNA, single-stranded DNA, and double-stranded DNA) and sensitivity to indigenous microbial activity.35,39 Furthermore, tolerance to disinfection varies among environmental and laboratory strains in a single serotype.134–136 Virus adaptation to disinfection can be explained by the dynamic alternation of dominant sequences in a viral population, which is induced by virus evolution against selection constraints.137 Virus inactivation in a sanitization process (e.g., raising temperature, pH, and ammonia concentration) should be investigated with an insight into virus population genetics; the expected maximum tolerance of viruses should be considered when determining sanitization conditions. As only few viruses are zoonotic, a consideration about if reduction of viruses is required in a circular system for non-food use is necessary.
4.4 Designing a multi-barrier to attain sanitization and stabilization
The sanitization potential of a single technology is determined by the biocidal factors inherent to the technology, including temperature, pH, ammonia concentration, moisture content, and indigenous microbial activity (Table 4). Individual technologies were compared to the inherent biocidal effect of these factors (Table 4). It should be noted that susceptibility to biocidal factors differs among microorganisms. For example, Salmonella spp. were rapidly inactivated at pH 12 in ammonia- and carbonate-free buffer solutions, whereas Ascaris eggs and spores of Clostridium perfringens were not inactivated.121,138 Temperature, pH, ammonia concentration, and moisture content were used as monitoring parameters. Continuous monitoring of microbial and enzymatic activities is not considered practical because culture methods usually require an overnight incubation period. Indicators that represent the performance of biological treatments (e.g., C/N ratio and odor) could be alternative monitoring parameters.
Table 4 Biocidal factors (+) inherent in the individual technologies, regarding temperature, pH, uncharged ammonia concentration, moisture content and microbial/enzymatic activity
Technologies |
High temperature |
Alkali/acid pH |
Uncharged ammonia |
Desiccation |
Microbial/enzymatic activity |
Not significant (−) when urea-hydrolysis is inhibited.
|
For urine |
Storage |
− |
+ |
+ |
− |
+ |
Solar heating and pasteurization |
+ |
+a |
+a |
− |
− |
Nitrification and distillation |
+ |
− |
− |
− |
+ |
Membrane technologies |
− |
+a |
+a |
− |
− |
Struvite precipitation |
− |
− |
− |
+ |
− |
Alkali dehydration |
− |
+ |
− |
+ |
− |
Ion exchange/sorption |
− |
− |
− |
− |
− |
For fecal sludge |
Storage |
|
|
|
|
|
- Bucket latrine and container |
− |
+ |
+ |
− |
+ |
- Single pit latrine |
− |
+ |
+ |
− |
+ |
- Septic tank |
− |
− |
− |
− |
+ |
- Twin pits for pour flush toilet |
− |
+ |
+ |
− |
+ |
- Double alternating dry pit |
− |
+ |
+ |
+ |
+ |
- Double dehydration vaults |
− |
+ |
− |
+ |
+ |
Alkali sanitization |
− |
+ |
+ |
− |
− |
Ammonia/urea sanitization |
− |
+ |
+ |
− |
− |
Solar drying and pasteurization |
+ |
− |
− |
+ |
− |
Composting |
+ |
− |
− |
+ |
+ |
Vermicomposting |
− |
− |
− |
− |
+ |
Black soldier fly composting |
− |
− |
− |
− |
+ |
Lactic acid fermentation |
− |
+ |
− |
− |
+ |
Anaerobic digestion |
+ |
+ |
− |
− |
+ |
Drying |
+ |
− |
− |
+ |
− |
Microwaving |
+ |
− |
|
+ |
− |
The operational conditions of individual technologies can be optimized for sufficient pathogen inactivation by controlling temperature, pH, ammonia concentration, and moisture content; however, they are not always controllable. We have distinguished the controllable parameters from the monitoring parameters in the Table 2. For example, a higher temperature is favorable for rapid inactivation, but the temperature inside a single pit latrine is merely changed owing to its large capacity. Meanwhile, smaller containers (e.g., buckets latrine) can be moved to sunlight to promote pathogen inactivation by increasing the temperature of the matrix. When biocidal factors cannot be intensified using a certain technology, greater inactivation is achieved by extending storage time.
This study investigated the operating conditions, with a focus on the pathogen inactivation performance of sanitation technologies. Nevertheless, we must emphasize that appropriate sanitization conditions do not always lead to stabilization. We identified resource recovery technologies that are not stand-alone processes for sanitization. Specifically, pathogens can remain infective during biological treatments without generating heat, such as nitrification, vermicomposting, BSF composting, and LAF. Increasing the operating temperature for pathogen inactivation affects valuable microorganisms involved in the organic matter stabilization. Similarly, ammonia treatment, drying, and thermophilic storage result in partial stabilization of organic matter because they hamper microbial activity in biological treatments.139 Liming is an effective disinfection method that is applicable for on-site treatments before manual emptying; however, an extremely alkaline pH inhibit indigenous microbial activity.140 Therefore, stabilization and sanitization may not be attained simultaneously, and the CL value should be set so as not to hinder biological treatment.
A multibarrier approach should be designed to attain stabilization and sanitization with an appropriate combination of technical barriers. To achieve this, we recommend that the pathogen inactivation efficiency of every technology be evaluated in advance under the optimum conditions for stabilization. An inactivation kinetics model for pathogens should be developed for individual technologies and used to estimate the level of pathogens under certain operating conditions. Additional sanitization processes can be introduced as long as they do not hinder the stabilization.
WHO recommends a combination of treatment technologies and measures other than treatments, including restrictions on the fertilization of edible plants, use of personal protection equipment (PPE), and farmland application techniques.6 We suggest that these technical and non-technical barriers be introduced and monitored as priorities when the target LRV is not achieved in a preceding multiple barrier treatment. Estimation of the LRV in resource recovery technology is important to provide a clear rationale for the intensive monitoring of non-technical barriers and barriers other than treatment technologies.
4.5 The limitations of this review
This review has two limitations. First, greenhouse gas emissions were not considered. We describe that long storage or retention times are necessary for disinfection; however, greenhouse gas emissions from fecal sludge can increase significantly with increased storage time.141,142 Reid et al. demonstrated that replacement to a low-CH4 emission technologies (e.g., composting toilet) yields co-benefits for both greenhouse gas mitigation and water and sanitation development.141 Second, some novel thermal treatment systems are not included that have gained traction in recent years, e.g., Omni Processors.143 These technologies may completely inactivate pathogens and produce a product that can be used for agriculture (e.g., biochar).
Conflicts of interest
There are no conflicts of interest to declare.
Acknowledgements
This work was supported by the Research Institute for Humanity and Nature (RIHN: a constituent member of NIHU) under Grant No. 14200107 and JSPS KAKENHI under Grant No. 20J10868 and 22K20442.
References
- J. T. Trimmer and J. S. Guest, Recirculation of human-derived nutrients from cities to agriculture across six continents, Nat. Sustain., 2018, 1, 427–435 CrossRef.
- R. Harder, R. Wielemaker, S. Molander, G. Öberg, M. R. Charbonneau and V. M. Isabella,
et al., Reframing human excreta management as part of food and farming systems, Water Res., 2020, 11(1), 1–8, DOI:10.1038/s41467-020-15508-1.
-
GmbH Technische Zusammenarbeit (GTZ), ecosan - closing the loop in wastewater management and sanitation, in Proceedings of the International Symposium, 30–31 October 2000, Bonn, Germany [Internet], ed. C. Werner, J. Schlick, G. Witte and A. Hildebrandt, Eschborn, Germany, 2001, p. 38, Available from: https://ceadserv1.nku.edu/longa/haiti/kids/sanitation/ecosan-Symposium-Bonn-proceedings.pdf.
-
Ellen MacArthur Foundation, Towards the circular economy: opportunities for the consumer goods sector, 2013 Search PubMed.
- R. Harder, R. Wielemaker, T. A. Larsen, G. Zeeman and G. Öberg, Recycling nutrients contained in human excreta to agriculture: Pathways, processes, and products, Crit. Rev. Environ. Sci. Technol., 2019, 49(8), 695–743 CrossRef.
-
WHO, WHO guidelines for the safe use of wastewater, excreta and greywater, Geneva, 2006, vol. 4 Excreta and greywater use in agriculture Search PubMed.
-
WHO, Sanitation safety planning: manual for safe use and disposal of wastewater, greywater and excreta [Internet], Geneva, 2015, [cited 2019 Feb 4], Available from: https://apps.who.int/iris/handle/10665/171753 Search PubMed.
-
US FDA, HACCP Principles and Application Guidelines [Internet], Silver Spring, Maryland, 1997, [cited 2020 Jan 3], Available from: https://www.fda.gov/food/hazard-analysis-critical-control-point-haccp/haccp-principles-application-guidelines Search PubMed.
- W. Oishi, S. S. Kadoya, O. Nishimura, J. B. Rose and D. Sano, Hierarchical Bayesian modeling for predictive environmental microbiology toward a safe use of human excreta: Systematic review and meta-analysis, J. Environ. Manage., 2021, 284(February), 112088, DOI:10.1016/j.jenvman.2021.112088.
-
Ministry of Health, Decision No. 08/2005/QD-BYT, regarding issuing the sector standards: Hygiene standards for various types of latrines, Hanoi, Vietnum, 2005 Search PubMed.
-
Ministry of Health of the People's Republic of China, Hygienic requirements for harmless disposal of night soil, China, 2012 Search PubMed.
- ISO, Non-sewered sanitation systems — Prefabricated integrated treatment units — General safety and performance requirements for design and testing (Reference number: ISO 30500:2018(E)), 2018.
- ISO, Faecal sludge treatment units — Energy independent, prefabricated, community-scale, resource recovery units — Safety and performance requirements (Reference number ISO 31800:2020(E)), 2020.
-
J. McConville, C. Niwagaba, A. Nordin, M. Ahlström, V. Namboozo and M. Kiffe, Guide to sanitation resource-recovery products and technologies: A supplement to the compendium of sanitation systems and technologies, Swedish University of Agricultural Sciences (SLU), Uppsala, 1st edn, 2020 Search PubMed.
- C. Schönning, T. Westrell, T. A. Stenström, K. Arnbjerg-Nielsen, A. B. Hasling and L. Høibye,
et al., Microbial risk assessment of local handling and use of human faeces, J. Water Health, 2007, 5(1), 117–128 CrossRef PubMed.
- Sanitation and Disease in the 21st Century: Health and Microbiological Aspects of Excreta and Wastewater Management [Internet], 2019, Available from: https://www.waterpathogens.org/toc.
- W. Oishi, B. Vinnerås, J. B. Rose and D. Sano, Predictive environmental microbiology for safe use of sanitation products in agriculture: Challenges and perspectives, Environ. Sci. Technol. Lett., 2021, 8(11), 924–931 CrossRef CAS.
-
T. Westrell, Microbial risk assessment and its implications for risk management in urban water systems, Linköping University, Sweden, 2004 Search PubMed.
-
A. Rohatgi, WebPlotDigitizer [Internet], 2019, [cited 2019 Jul 1], Available from: https://automeris.io/WebPlotDigitizer Search PubMed.
- L. W. Hom, Kinetics of chlorine disinfection in an ecosystem, J. Sanit. Eng. Div., 1972, 98(1), 183–194 CrossRef.
-
R Core Team, R: A language and environment for statistical computing [Internet], R Foundation for Statistical Computing, Vienna, Austria, 2016, Available from: https://www.r-project.org/ Search PubMed.
- H. Heinonen-Tanski and C. Van Wijk-Sijbesma, Human excreta for plant production, Bioresour. Technol., 2005, 96(4), 403–411 CrossRef CAS PubMed.
- K. M. Udert, T. A. Larsen and W. Gujer, Biologically induced precipitation in urine-collecting systems, Water Supply, 2003, 3(3), 71–78, DOI:10.2166/ws.2003.0010.
- K. M. Udert, C. A. Buckley, M. Wächter, C. S. McArdell, T. Kohn and L. Strande,
et al., Technologies for the treatment of source-separated urine in the eThekwini Municipality, Water SA, 2015, 41(2), 212–221 CrossRef CAS.
- J. Senecal and B. Vinnerås, Urea stabilisation and concentration for urine-diverting dry toilets: Urine dehydration in ash, Sci. Total Environ., 2017, 586, 650–657, DOI:10.1016/j.scitotenv.2017.02.038.
- R. Ito, E. Takahashi and N. Funamizu, Production of slow-released nitrogen fertilizer from urine, Environ. Technol., 2013, 34(20), 2809–2815, DOI:10.1080/09593330.2013.790069.
- C. Schönning, R. Leeming and T. A. Stenström, Faecal contamination of source-separated human urine based on the content of faecal sterols, Water Res., 2002, 36(8), 1965–1972 CrossRef PubMed.
- B. Vinnerås, G. Bölske, H. Wahlström and A. Albihn, Survival of Mycobacterium tuberculosis and Mycobacterium bovis in human urine, Water Sci. Technol., 2011, 63(6), 1075–1080, DOI:10.2166/wst.2011.344.
- W. Ahmed, K. A. Hamilton, A. Vieritz, D. Powell, A. Goonetilleke and M. T. Hamilton,
et al., Microbial risk from source-separated urine used as liquid fertilizer in sub-tropical Australia, Microb. Risk Anal., 2017, 5, 53–64 CrossRef.
- W. Oishi, I. Kato, N. Hijikata, K. Ushijima, R. Ito and N. Funamizu,
et al., Inactivation kinetics modeling of Escherichia coli in concentrated urine for implementing predictive environmental microbiology in sanitation safety planning, J. Environ. Manage., 2020, 268, 110672, DOI:10.1016/j.jenvman.2020.110672.
- B. Vinnerås, A. Nordin, C. Niwagaba and K. Nyberg, Inactivation of bacteria and viruses in human urine depending on temperature and dilution rate, Water Res., 2008, 42(15), 4067–4074 CrossRef PubMed.
- H. Jönsson and B. Vinnerås, Experiences and suggestions for collection systems for source-separated urine and faeces, Water Sci. Technol., 2007, 56(5), 71–76, DOI:10.2166/wst.2007.558.
- L. Decrey, S. Kazama, K. M. Udert and T. Kohn, Ammonia as an in situ sanitizer: Inactivation kinetics and mechanisms of the ssRNA virus MS2 by NH3, Environ. Sci. Technol., 2015, 49(2), 1060–1067 CrossRef CAS PubMed.
- C. Höglund, N. Ashbolt, T. A. Stenström and L. Svensson, Viral persistence in source-separated human urine, Adv. Environ. Res., 2002, 6(3), 265–275 CrossRef.
- L. Decrey and T. Kohn, Virus inactivation in stored human urine, sludge and animal manure under typical conditions of storage or mesophilic anaerobic digestion, Environ. Sci.: Water Res. Technol., 2017, 3(3), 492–501 RSC.
- A. Nordin, K. Nyberg and B. Vinnerås, Inactivation of Ascaris eggs in source-separated urine and feces by ammonia at ambient temperatures, Appl. Environ. Microbiol., 2009, 75(3), 662–667 CrossRef CAS PubMed.
- G. W. Park and F. Diez-Gonzalez, Utilization of carbonate and ammonia-based treatments to eliminate Escherichia coli O157:H7 and Salmonella Typhimurium DT104 from cattle manure, J. Appl. Microbiol., 2003, 94(4), 675–685 CrossRef CAS PubMed.
- L. Decrey, S. Kazama and T. Kohn, Ammonia as an in situ sanitizer: Influence of virus genome type on inactivation, Appl. Environ. Microbiol., 2016, 82(16), 4909–4920 CrossRef CAS PubMed.
- H. E. Goetsch, L. Zhao, M. Gnegy, M. J. Imperiale, N. G. Love and K. Wigginton, Fate of the urinary tract virus BK human polyomavirus in source-separated urine, Appl. Environ. Microbiol., 2018, 84, e02374-17 CrossRef PubMed.
- D. Sangare, A. L. Brou, M. Sou/dakoure and P. V. Tagro, Urine treatment by solar disinfection for agriculture reuse purpose in a poor rural context: case of Burkina Faso, J. Water, Sanit. Hyg. Dev., 2020, 1–9 Search PubMed.
- K. R. Wigginton and T. Kohn, Virus disinfection mechanisms: The role of virus composition, structure, and function, Curr. Opin. Virol., 2012, 2(1), 84–89, DOI:10.1016/j.coviro.2011.11.003.
- S. Kazama and M. Otaki, Mechanisms for the inactivation of bacteria and viruses in sawdust used in composting toilet, J. Water Environ. Technol., 2011, 9(1), 53–66 CrossRef.
- B. M. Pecson and K. L. Nelson, Inactivation of Ascaris suum eggs by ammonia, Environ. Sci. Technol., 2005, 39(20), 7909–7914 CrossRef CAS PubMed.
- A. Nordin, C. Niwagaba, H. Jönsson and B. Vinnerås, Pathogen and indicator inactivation in source-separated human urine heated by the sun, J. Water, Sanit. Hyg. Dev., 2013, 3(2), 181–188 CrossRef.
- C. M. Davies, D. J. Roser, A. J. Feitz and N. J. Ashbolt, Solar radiation disinfection of drinking water at temperate latitudes: Inactivation rates for an optimised reactor configuration, Water Res., 2009, 43(3), 643–652 CrossRef CAS PubMed , Available from: https://www.sciencedirect.com/science/article/pii/S0043135408005575.
-
B. Etter and K. M. Udert, VUNA handbook on urine treatment, Dübendorf, Switzerland, 2015 Search PubMed.
- H. N. Bischel, A. Schertenleib, A. Fumasoli, K. M. Udert and T. Kohn, Inactivation kinetics and mechanisms of viral and bacterial pathogen surrogates during urine nitrification, Environ. Sci.: Water Res. Technol., 2015, 1(1), 65–76 RSC.
- S. Zhao, L. Zou, C. Y. Tang and D. Mulcahy, Recent developments in forward osmosis: Opportunities and challenges, J. Membr. Sci., 2012, 396, 1–21 CrossRef CAS , Available from: https://www.sciencedirect.com/science/article/pii/S0376738811009215.
- W. Oishi, D. Sano, L. Decrey, S. Kadoya, T. Kohn and N. Funamizu, Identification of the inactivating factors and mechanisms exerted on MS2 coliphage in concentrated synthetic urine, Sci. Total Environ., 2017, 598, 213–219, DOI:10.1016/j.scitotenv.2017.04.088.
- L. Decrey, K. M. Udert, E. Tilley, B. M. Pecson and T. Kohn, Fate of the pathogen indicators phage ΦX174 and Ascaris suum eggs during the production of struvite fertilizer from source-separated urine, Water Res., 2011, 45(16), 4960–4972 CrossRef CAS PubMed.
- H. N. Bischel, S. Schindelholz, M. Schoger, L. Decrey, C. A. Buckley and K. M. Udert,
et al., Bacteria inactivation during the drying of struvite fertilizers produced from stored urine, Environ. Sci. Technol., 2016, 50(23), 13013–13023 CrossRef CAS PubMed.
- L. Decrey, K. M. Udert, E. Tilley, B. M. Pecson and T. Kohn, Fate of the pathogen indicators phage ΦX174 and Ascaris suum eggs during the production of struvite fertilizer from source-separated urine, Water Res., 2011, 45(16), 4960–4972 CrossRef CAS PubMed.
- J. Senecal and B. Vinnerås, Urea stabilisation and concentration for urine-diverting dry toilets: Urine dehydration in ash, Sci. Total Environ., 2017, 586, 650–657, DOI:10.1016/j.scitotenv.2017.02.038.
- J. Senecal, A. Nordin, P. Simha and B. Vinnerås, Hygiene aspect of treating human urine by alkaline dehydration, Water Res., 2018, 144, 474–481 CrossRef CAS PubMed.
- H. J. Busscher, R. J. B. Dijkstra, E. Engels, D. E. Langworthy, D. I. Collias and D. W. Bjorkquist,
et al., Removal of two waterborne pathogenic bacterial strains by activated carbon particles prior to and after charge modification, Environ. Sci. Technol., 2006, 40(21), 6799–6804 CrossRef CAS PubMed.
- K. Naka, S. Watarai, K. Inoue, Y. Kodama, K. Oguma, T. Yasuda and H. Kodama,
et al., Adsorption Effect of Activated Charcoal on Enterohemorrhagic Escherichia coli, J. Vet. Med. Sci., 2001, 63(3), 281–285 CrossRef CAS PubMed.
- W. A. M. Hijnen, G. M. H. Suylen, J. A. Bahlman, A. Brouwer-Hanzens and G. J. Medema, GAC adsorption filters as barriers for viruses, bacteria and protozoan (oo)cysts in water treatment, Water Res., 2010, 44(4), 1224–1234, DOI:10.1016/j.watres.2009.10.011.
- C. Jacquin, D. Yu, M. Sander, K. W. Domagala, J. Traber and E. Morgenroth,
et al., Competitive co-adsorption of bacteriophage MS2 and natural organic matter onto multiwalled carbon nanotubes, Water Res.: X, 2020, 9, 100058 CAS , Available from: https://www.sciencedirect.com/science/article/pii/S2589914720300189.
- T. Matsushita, H. Suzuki, N. Shirasaki, Y. Matsui and K. Ohno, Adsorptive virus removal with super-powdered activated carbon, Sep. Purif. Technol., 2013, 107, 79–84 CrossRef CAS , Available from: https://www.sciencedirect.com/science/article/pii/S1383586613000324.
- J. W. Chung, M. Breulmann, A. Clemens, C. Fühner, J. W. Foppen and P. N. L. Lens, Simultaneous removal of rotavirus and adenovirus from artificial ground water using hydrochar derived from swine feces, J. Water Health, 2016, 14(5), 754–767, DOI:10.2166/wh.2016.010.
-
C. B. Niwagaba, M. Mbéguéré and L. Strande, Faecal sludge quantification, characterisation and treatment objectives, in Faecal sludge management: systems approach for implementation and operation [Internet], ed. L. Strande, M. Ronteltap and D. Brdjanovic. IWA Publishing, London, 2014, pp. 19–44, Available from: https://www.un-ihe.org/sites/default/files/fsm_book_lr.pdf Search PubMed.
-
E. Tilley, L. Ulrich, C. Lüthi, Ph. Reymond and C. Zurbrügg, Compendium of sanitation systems and technologies, Swiss Federal Institute of Aquatic Science and Technology (Eawag), Dübendorf, Switzerland, 2nd revised edn, 2014 Search PubMed.
- K. D. Orner and J. R. Mihelcic, A review of sanitation technologies to achieve multiple sustainable development goals that promote resource recovery, Environ. Sci.: Water Res. Technol., 2018, 4(1), 16–32 RSC.
-
T. Kohn, L. Decrey and B. Vinnerås, Chemical Disinfectants, in Water and Sanitation for the 21st Century: Health and Microbiological Aspects of Excreta and Wastewater Management (Global Water Pathogen Project) [Internet], ed. B. Jiménez-Cisneros and J. B. Rose, Michigan State University, UNESCO, E. Lansing, MI, 2017, [cited 2019 Aug 1], Available from: https://www.waterpathogens.org/book/chemical-disinfectants Search PubMed.
-
G. Mikhael, D. M. Robbins, J. E. Ramsay and M. Mbéguéré, Methods and means for collection and transport of faecal sludge, in Fecal Sludge Management systems approach for implementation and operation, ed. L. Strande, M. Ronteltap and D. Brdjanovic, IWA Publishing, London, 1st edn, 2014, pp. 67–96 Search PubMed.
-
T. A. Stenström, R. Seidu, E. Nelson and Z. Christian, Microbial Exposure and Health Assessments in Sanitation Technologies and Systems [Internet], 2011, pp. 1–165, Available from: https://www.ecosanres.org Search PubMed.
- K. C. Russel, K. Hughes, M. Roach, D. Auerbach, A. Foote and S. Kramer,
et al., Taking container-based sanitation to scale: opportunities and challenges, Front. Environ. Sci., 2019, 7, 1–7 CrossRef.
- D. T. G. da Silva, E. Dias, J. Ebdon and H. Taylor, Assessment of recommended approaches for containment and safe handling of human excreta in emergency settings, PLoS One, 2018, 13(7), 1–20 Search PubMed.
- T. A. Ogunyoku, F. Habebo and K. L. Nelson, In-toilet disinfection of fresh fecal sludge with ammonia naturally present in excreta, J. Water, Sanit. Hyg. Dev., 2016, 6(1), 104–114 CrossRef.
- C. J. Brouckaert, K. M. Foxon and K. Wood, Modelling the filling rate of pit latrines, Water SA, 2013, 39(4), 555–562 Search PubMed.
- M. W. Jenkins, O. Cumming, B. Scott and S. Cairncross, Beyond “improved” towards “safe and sustainable” urban sanitation: Assessing the design, management and functionality of sanitation in poor communities of Dar es Salaam, Tanzania, J. Water, Sanit. Hyg. Dev., 2014, 4(1), 131–141 CrossRef.
-
K. D. Orner, C. Naughton and T. A. Stenstrom, in Pit toilets (latrines), ed. J. R. Mihelcic and M. E. Verbyla, Michigan State University, UNESCO, E. Lansing, MI, 2018 Search PubMed.
- S. Nabateesa, A. Zziwa, I. S. A. Kabenge, R. Kambugu, J. Wanyama and A. J. Komakech, Occurrence and survival of pathogens at different sludge depths in unlined pit latrines in kampala slums, Water SA, 2017, 43(4), 638–645 CrossRef CAS.
- A. Nakagiri, C. B. Niwagaba, P. M. Nyenje, R. K. Kulabako, J. B. Tumuhairwe and F. Kansiime, Assessing ambient and internal environmental conditions of pit latrines in urban slums of Kampala, Uganda: Effect on performance, J. Water, Sanit. Hyg. Dev., 2017, 7(1), 92–101 CrossRef.
-
A. Adegoke and T. A. Stenström, Septic systems, in Water and Sanitation for the 21st Century: Health and Microbiological Aspects of Excreta and Wastewater Management (Global Water Pathogen Project) [Internet], ed. J. B. Rose and B. Jiménez-Cisneros, Michigan State University, UNESCO, E. Lansing, MI, 2019, Available from: https://doi.org/10.14321/waterpathogens.59 Search PubMed.
- A. M. Kadam, G. H. Oza, P. D. Nemade and H. S. Shankar, Pathogen removal from municipal wastewater in constructed soil filter, Ecol. Eng., 2008, 33(1), 37–44 CrossRef.
- V. T. Yen-Phi, J. Clemens, A. Rechenburg, B. Vinneras, C. Lenßen and T. Kistemann, Hygienic effects and gas production of plastic bio-digesters under tropical conditions, J. Water Health, 2009, 7(4), 590–596, DOI:10.2166/wh.2009.127.
-
D. Mara, The design of pour-flush latrines, 1985 Search PubMed.
-
R. G. Feachem, D. J. Bradley, H. Garelick and D. Mara, Sanitation and disease: Health aspects of excreta and wastewater management, in World Bank Studies in Water Supply and Sanitation 3, 1983, p. 501 Search PubMed.
-
C. Naughton, K. D. Orner, T. A. Stenstrom and J. R. Mihelcic, Composting and dry desiccating toilets (latrines), Michigan State University, UNESCO, E. Lansing, MI, 2019 Search PubMed.
- L. M. Cruz Espinoza, D. Yeh, B. Vinnerås, L. Rajaram, J. Corvin and R. Izurieta, Inactivation of ascaris suum by ammonia in feces simulating the parameters of the solar toilet, J. Appl. Sci. Environ. Sanit., 2012, 7(3), 173–182 Search PubMed , Available from: http://libproxy.lib.unc.edu/login?url=https://search.proquest.com/docview/912918996?accountid=14244.
- M. E. Magri, L. S. Philippi and B. Vinnerås, Inactivation of pathogens in feces by desiccation and urea treatment for application in urine-diverting dry toilets, Appl. Environ. Microbiol., 2013, 79(7), 2156–2163 CrossRef CAS PubMed.
- A. Nordin, J. Ottoson and B. Vinnerås, Sanitation of faeces from source-separating dry toilets using urea, J. Appl. Microbiol., 2009, 107(5), 1579–1587 CrossRef CAS PubMed.
-
US EPA, A plain english guide to the EPA Part 503 biosolids rule, Washington, 1994 Search PubMed.
- S. Cha, Y. S. Kim, A. L. Lee, D.-H. Lee and N. Koo, Liming Alters the Soil Microbial Community and Extracellular Enzymatic Activities in Temperate Coniferous Forests, Forests, 2021, 12(2), 190 CrossRef.
- O. Franco-Hernández, A. Natalia Mckelligan-Gonzalez, A. Maria Lopez-Olguin, F. Espinosa-Ceron, E. Escamilla-Silva and L. Dendooven, Dynamics of carbon, nitrogen and phosphorus in soil amended with irradiated, pasteurized and limed biosolids, Bioresour. Technol., 2003, 87(1), 93–102 CrossRef PubMed , Available from: https://www.sciencedirect.com/science/article/pii/S0960852402001888.
- N. Hijikata, R. Tezuka, S. Kazama, M. Otaki, K. Ushijima and R. Ito,
et al., Bactericidal and virucidal mechanisms in the alkaline disinfection of compost using calcium lime and ash, J. Environ. Manage., 2016, 181, 721–727, DOI:10.1016/j.jenvman.2016.08.026.
- H. S. Darimani, R. Ito, S. K. Sossou, N. Funamizu and M. H. Amadou, Effect of post-treatment conditions on the inactivation
rate of pathogenic bacteria after the composting process, Compost Sci. Util., 2015, 23(3), 164–173 CrossRef CAS.
- W. Greya, B. Thole, C. Anderson, F. Kamwani, J. Spit and G. Mamani, Off-site lime stabilisation as an option to treat pit latrine faecal sludge for emergency and existing on-site sanitation systems, J. Waste Manage., 2016, 2016, 1–8 CrossRef.
- J. Senecal, A. Nordin and B. Vinnerås, Fate of ascaris at various ph, temperature and moisture levels, J. Water Health, 2020, 18(3), 375–382 CrossRef PubMed.
-
C. Moe and R. Izurieta, Longitudinal study of double vault urine diverting toilets and solar toilets in El Salvador, in Ecosan -- Closing the loop Proceeding of the 2nd international symposium on ecological sanitation, ed. C. Werner, Lübeck, 2004, pp. 295–302 Search PubMed.
- B. Vinnerås, M. Hedenkvist, A. Nordin and A. Wilhelmson, Peepoo bag: Self-sanitising single use biodegradable toilet, Water Sci. Technol., 2009, 59(9), 1743–1749 CrossRef PubMed.
- K. Lubkowski, Environmental impact of fertilizer use and slow release of mineral nutrients as a response to this challenge, Pol. J. Chem. Technol., 2016, 18(1), 72–79, DOI:10.1515/pjct-2016-0012.
-
R. Hasan, A. C. Nordin, S. Shakoor, I. Keenum and B. Vinneras, Salmonella, Enteric Fevers, and Salmonellosis, in Water and Sanitation for the 21st Century: Health and Microbiological Aspects of Excreta and Wastewater Management (Global Water Pathogen Project) [Internet], ed. A. Pruden, N. Ashbolt and J. Miller, Michigan State University, UNESCO, E. Lansing, MI, 2019, DOI:10.14321/waterpathogens.27.
- L. A. Harroff, J. L. Liotta, D. D. Bowman and L. T. Angenent, Current time-temperature relationships for thermal inactivation of Ascaris eggs at mesophilic temperatures are too conservative and may hamper development of simple, but effective sanitation, Water Res.: X, 2019, 5, 100036, DOI:10.1016/j.wroa.2019.100036.
- C. Niwagaba, M. Nalubega, B. Vinnerås, C. Sundberg and H. Jönsson, Bench-scale composting of source-separated human faeces for sanitation, Waste Manage., 2009, 29(2), 585–589, DOI:10.1016/j.wasman.2008.06.022.
- S. Kazama, N. Tameike, N. Nakagawa and M. Otaki, A fate model of pathogenic viruses in a composting toilet based on coliphage inactivation, J. Environ. Sci., 2011, 23(7), 1194–1198 CrossRef PubMed.
- M. Manga, M. A. Camargo-Valero and B. E. Evans, Inactivation of viable Ascaris eggs during faecal sludge co-composting with chicken feathers and market waste, Desalin. Water Treat., 2019, 163, 347–357 CrossRef CAS.
- B. Vinnerås, A. Björklund and H. Jönsson, Thermal composting of faecal matter as treatment and possible disinfection method - Laboratory-scale and pilot-scale studies, Bioresour. Technol., 2003, 88(1), 47–54 CrossRef PubMed.
-
W. Berger, Technology review of composting toilets: Basic overview of composting toilets (with or without urine diversion) [Internet], ed. E. von Muench, Deutsche Gesellschaft für Internationale Zusammenarbeit (GIZ) GmbH, Eschborn, Germany, 2011, [cited 2019 Dec 12], Available from: https://www.susana.org/_resources/documents/default/2-878-2-1383-gtz2011-en-technology-review-composting-toilets1.pdf Search PubMed.
- J. Mehl, J. Kaiser, D. Hurtado, D. A. Gibson, R. Izurieta and J. R. Mihelcic, Pathogen destruction and solids decomposition in composting latrines: Study of fundamental mechanisms and user operation in rural Panama, J. Water Health, 2011, 9(1), 187–199 CrossRef PubMed.
- J. Domínguez, M. Aira, A. R. Kolbe, M. Gómez-Brandón and M. Pérez-Losada, Changes in the composition and function of bacterial communities during vermicomposting may explain beneficial properties of vermicompost, Sci. Rep., 2019, 9(1), 1–11 CrossRef PubMed.
- A. Swati and S. Hait, A comprehensive review of the fate of pathogens during vermicomposting of organic wastes, J. Environ. Qual., 2018, 47(1), 16–29 CrossRef CAS PubMed.
- L. Zhao, Y. Wang, J. Yang, M. Xing, X. Li and D. Yi,
et al., Earthworm-microorganism interactions: A strategy to stabilize domestic wastewater sludge, Water Res., 2020, 44(8), 2572–2582, DOI:10.1016/j.watres.2010.01.011.
- K. D. Yadav, V. Tare and M. M. Ahammed, Vermicomposting of source-separated human faeces by Eisenia fetida: Effect of stocking density on feed consumption rate, growth characteristics and vermicompost production, Waste Manage., 2011, 31(6), 1162–1168, DOI:10.1016/j.wasman.2011.02.008.
- G. B. Hill and S. A. Baldwin, Vermicomposting toilets, an alternative to latrine style microbial composting toilets, prove far superior in mass reduction, pathogen destruction, compost quality, and operational cost, Waste Manage., 2012, 32(10), 1811–1820, DOI:10.1016/j.wasman.2012.04.023.
- G. B. Hill, C. Lalander and S. A. Baldwin, The effectiveness and safety of vermi- versus conventional composting of human feces with Ascaris suum ova as model helminthic parasites, J. Sustain. Dev., 2013, 6(4), 1–10 Search PubMed.
- C. H. Lalander, G. B. Hill and B. Vinnerås, Hygienic quality of faeces treated in urine diverting vermicomposting toilets, Waste Manage., 2013, 33(11), 2204–2210, DOI:10.1016/j.wasman.2013.07.007.
- S. Diener, C. Zurbrügg and K. Tockner, Conversion of organic material by black soldier fly larvae: establishing optimal feeding rates, Waste Manage. Res., 2009, 27(6), 603–610 CrossRef CAS PubMed.
-
M. Ronteltap, P.-H. Dodane and M. Bassan, Overview of treatment technologies, in Fecal Sludge Management systems approach for implementation and operation, ed. L. Strande, M. Ronteltap and D. Brdjanovic, IWA Publishing, London, 1st edn, 2014, pp. 97–120 Search PubMed.
- C. H. Lalander, S. Diener, M. E. Magri, C. Zurbrügg, A. Lindström and B. Vinnerås, High waste-to-biomass conversion and efficient Salmonella spp. reduction using black soldier fly for waste recycling, Sci. Total Environ., 2015, 35(1), 312–318 Search PubMed.
- S. De Gisi, L. Petta and C. Wendland, History and technology of Terra Preta sanitation, Sustainability, 2013, 6(3), 1328–1345 CrossRef.
- P. Saranraj, M. A. Naidu and P. Sivasakthivelan, Lactic acid bacteria and its antimicrobial a properties: A review, Int. J. Pharm. Biol. Arch., 2014, 4(6), 1124–1133 Search PubMed , Available from: https://www.ijpba.info.
- C. Anderson, D. H. Malambo, M. E. G. Perez, H. N. Nobela, L. de Pooter and J. Spit,
et al., Lactic acid fermentation, urea and lime addition: Promising faecal sludge sanitizing methods for emergency sanitation, Int. J. Environ. Res. Public Health, 2015, 12(11), 13871–13885 CrossRef CAS PubMed.
- N. Andreev, M. Ronteltap, B. Boincean and P. N. L. Lens, Lactic acid fermentation of human excreta for agricultural application, J. Environ. Manage., 2018, 206, 890–900, DOI:10.1016/j.jenvman.2017.11.072.
- E. A. Odey, Z. Li, X. Zhou and Y. Yan, Optimization of lactic acid fermentation for pathogen inactivation in fecal sludge, Ecotoxicol. Environ. Saf., 2018, 157(December 2017), 249–254, DOI:10.1016/j.ecoenv.2018.03.075.
- E. A. Odey, Z. Li, X. Zhou and Y. Yan, Locally produced lactic acid bacteria for pathogen inactivation and odor control in fecal sludge, J. Cleaner Prod., 2018, 184, 798–805, DOI:10.1016/j.jclepro.2018.02.276.
- N. Andreev, M. Ronteltap, B. Boincean and P. N. L. Lens, Treatment of source-separated human feces via lactic acid fermentation combined with thermophilic composting, Compost Sci. Util., 2017, 25(4), 220–230, DOI:10.1080/1065657X.2016.1277809.
- T. E. Kearney, M. J. Larkin and P. N. Levett, The effect of slurry storage and anaerobic digestion on survival of pathogenis bacteria, J. Appl. Bacteriol., 1993, 74, 86–93 CrossRef CAS PubMed.
- T. E. Kearney, M. J. Larkin, J. P. Frost and P. N. Levett, Survival of pathogenic bacteria during mesophilic anaerobic digestion of animal waste, J. Appl. Bacteriol., 1993, 75, 215–219 CrossRef CAS PubMed.
- J. Fidjeland, A. Nordin and B. Vinnerås, Inactivation of Ascaris eggs and Salmonella spp. in fecal sludge by treatment with urea and ammonia solution, J. Water, Sanit. Hyg. Dev., 2016, 6(3), 465–473 CrossRef.
- E. Bagge, L. Sahlström and A. Albihn, The effect of hygienic treatment on the microbial flora of biowaste at biogas plants, Water Res., 2005, 39(20), 4879–4886 CrossRef CAS PubMed , Available from: https://www.sciencedirect.com/science/article/pii/S0043135405001156.
- S. Septien, A. Singh, S. W. Mirara, L. Teba, K. Velkushanova and C. A. Buckley, ‘LaDePa’ process for the drying and pasteurization of faecal sludge from VIP latrines using infrared radiation, S. Afr. J. Chem. Eng., 2018, 25(October), 147–158, DOI:10.1016/j.sajce.2018.04.005.
- P. M. Mawioo, C. M. Hooijmans, H. A. Garcia and D. Brdjanovic, Microwave treatment of faecal sludge from intensively used toilets in the slums of Nairobi, Kenya, J. Environ. Manage., 2016, 184, 575–584, DOI:10.1016/j.jenvman.2016.10.019.
- T. A. Nguyen, S. Babel, S. Boonyarattanakalin and T. Koottatep, Rapid and decentralized human waste treatment by microwave radiation, Water Environ. Res., 2016, 89(7), 652–662 CrossRef PubMed.
- V. K. Tyagi and S. L. Lo, Microwave irradiation: A sustainable way for sludge treatment and resource recovery, Renewable Sustainable Energy Rev., 2013, 18(71), 288–305, DOI:10.1016/j.rser.2012.10.032.
- O. O. D. Afolabi and M. Sohail, Microwaving human faecal sludge as a viable sanitation technology option for treatment and value recovery – A critical review, J. Environ. Manage., 2017, 187, 401–415, DOI:10.1016/j.jenvman.2016.10.067.
- H. S. Darimani, R. Ito, Y. Maiga, M. Sou, N. Funamizu and A. H. Maiga, Effect of post-treatment conditions on the inactivation of helminth eggs (Ascaris suum) after the composting process, Environ. Technol., 2016, 37, 920–928 CrossRef CAS PubMed.
- S. K. Sossou, M. Sou/Dakouré, N. Hijikata, A. H. Maiga and N. Funamizu, Inactivation kinetics of indicator microorganisms during solar heat treatment for sanitizing compost from composting toilet, J. Water Environ. Technol., 2016, 14(2), 37–46 CrossRef.
- T. Ito, M. Kitajima, T. Kato, S. Ishii, T. Segawa and S. Okabe,
et al., Target virus log10 reduction values determined for two reclaimed wastewater irrigation scenarios in Japan based on tolerable annual disease burden, Water Res., 2017, 125, 438–448, DOI:10.1016/j.watres.2017.08.057.
- D. Mara, Water- and wastewater-related disease and infection risks: What is an appropriate value for the maximum tolerable additional burden of disease?, J. Water Health, 2011, 9, 217–224 CrossRef PubMed.
- F. V. M. Silva, Differences in the resistance of microbial spores to thermosonication, high pressure thermal processing and thermal treatment alone, J. Food Eng., 2018, 222, 292–297, DOI:10.1016/j.jfoodeng.2017.11.037.
-
T. Aw, Environmental aspects and features of critical pathogen groups, in Sanitation and Disease in the 21st Century: Health and Microbiological Aspects of Excreta and Wastewater Management, 2018 Search PubMed.
- C. Wolf, U. Von Gunten and T. Kohn, Kinetics of inactivation of waterborne enteric viruses by ozone, Environ. Sci. Technol., 2018, 52(4), 2170–2177 CrossRef CAS PubMed.
- S. Meister, M. E. Verbyla, M. Klinger and T. Kohn, Variability in disinfection resistance between currently circulating enterovirus B serotypes and strains, Environ. Sci. Technol., 2018, 52(6), 3696–3705 CrossRef CAS PubMed.
- S. Torii, M. Itamochi and H. Katayama, Inactivation kinetics of waterborne virus by ozone determined by a continuous quench flow system, Water Res., 2020, 186, 116291, DOI:10.1016/j.watres.2020.116291.
- E. Domingo, J. Sheldon and C. Perales, Viral quasispecies evolution, Microbiol. Mol. Biol. Rev., 2012, 76(2), 159–216 CrossRef CAS PubMed.
- M. Myrmel, I. Modahl, H. Nygaard and K. M. Lie, Infectious pancreatic necrosis virus in fish by-products is inactivated with inorganic acid (pH 1) and base (pH 12), J. Fish Dis., 2014, 37(4), 349–355 CrossRef CAS PubMed.
-
A. Nordin, Pathogens and safe reuse of products, in Guide to sanitation resource-recovery products and technologies: A supplement to the compendium of sanitation systems and technologies, Swedish University of Agricultural Sciences (SLU), Uppsala, 1st edn, 2020, pp. 99–101 Search PubMed.
- Q. Jin and M. F. Kirk, pH as a primary control in environmental microbiology: 1. thermodynamic perspective, Front. Environ. Sci., 2018, 6(MAY), 1–15 Search PubMed.
- M. C. Reid, K. Guan, F. Wagner and D. L. Mauzerall, Global Methane Emissions from Pit Latrines, Environ. Sci. Technol., 2014, 48(15), 8727–8734, DOI:10.1021/es501549h.
- J. Johnson, F. Zakaria, A. G. Nkurunziza, C. Way, M. A. Camargo-Valero and B. Evans, Whole-system analysis reveals high greenhouse-gas emissions from citywide sanitation in Kampala, Uganda, Commun. Earth Environ., 2022, 3(1), 80, DOI:10.1038/s43247-022-00413-w.
-
B. Herzen and L. Talsma, Conversion of human waste into biochar using pyrolysis at a community-scale facility in Kenya - Various documents on results from research grant, Stanford University and The Climate Foundation, Stanford, California, USA, 2014 Search PubMed.
- J. Fidjeland, M. E. Magri, H. Jönsson, A. Albihn and B. Vinnerås, The potential for self-sanitisation of faecal sludge by intrinsic ammonia, Water Res., 2013, 47(16), 6014–6023 CrossRef CAS PubMed.
- M. E. Magri, J. Fidjeland, H. Jönsson, A. Albihn and B. Vinnerås, Inactivation of adenovirus, reovirus and bacteriophages in fecal sludge by pH and ammonia, Sci. Total Environ., 2015, 520, 213–221, DOI:10.1016/j.scitotenv.2015.03.035.
- P. Seruga, M. Krzywonos, Z. Paluszak, A. Urbanowska, H. Pawlak-Kruczek and Ł. Niedźwiecki,
et al., Pathogen reduction potential in anaerobic digestion of organic fraction of municipal solid waste and food waste, Molecules, 2020, 25(2), 1–13 CrossRef PubMed.
- P. K. Pandey and M. L. Soupir, Escherichia coli inactivation kinetics in anaerobic digestion of dairy manure under moderate, mesophilic and thermophilic temperatures, AMB Express, 2011, 1(1), 1–10 CrossRef.
- L. Decrey and T. Kohn, Virus inactivation in stored human urine, sludge and animal manure under typical conditions of storage or mesophilic anaerobic digestion, Environ. Sci.: Water Res. Technol., 2017, 3(3), 492–501 RSC.
- L. Baert, B. De Gusseme, N. Boon, W. Verstraete, J. Debevere and M. Uyttendaele, Inactivation of murine norovirus 1 and Bacteroides fragilis phage B40-8 by mesophilic and thermophilic anaerobic digestion of pig slurry, Appl. Environ. Microbiol., 2010, 76(6), 2013–2017 CrossRef CAS PubMed.
- S. Kato, E. Fogarty and D. Bowman, Effect of aerobic and anaerobic digestion on the viability of Cryptosporidium parvum oocysts and Ascaris suum eggs, Int. J. Environ. Health Res., 2003, 13(2), 169–179 CrossRef PubMed.
|
This journal is © The Royal Society of Chemistry 2023 |