Current and emerging technologies for the remediation of difficult-to-measure radionuclides at nuclear sites
Received
5th May 2023
, Accepted 16th October 2023
First published on 26th October 2023
Abstract
Difficult-to-measure radionuclides (DTMRs), defined by an absence of high energy gamma emissions during decay, are problematic in groundwaters at nuclear sites. DTMRs are common contaminants at many nuclear facilities, with (often) long half-lives and high radiotoxicities within the human body. Effective remediation is, therefore, essential if nuclear site end-state targets are to be met. However, due to a lack of techniques for in situ DTMR detection, technologies designed to remediate these nuclides are underdeveloped and tend to be environmentally invasive. With a growing agenda for sustainable remediation and reduction in nuclear decommissioning costs, there is renewed international focus on the development of less invasive technologies for DTMR clean-up. Here, we review recent developments for remediation of selected problem DTMRs (129I, 99Tc, 90Sr and 3H), with a focus on industrial and site-scale applications. We find that pump and treat (P&T) is the most used technique despite efficacy issues for 129I and 3H. Permeable reactive barriers (PRBs) are a less invasive alternative but have only been demonstrated for removal of 99Tc and 90Sr at scale. Phytoremediation shows promise for site-scale removal of 3H but is unsuitable for 129I and 99Tc due to biotoxicity and bioavailability hazards, respectively. No single technique can remediate all DTMRs of focus. Likewise, there has been no successful site-applied technology with high removal efficiencies for iodine species typically present in groundwaters (iodide/I−, iodate/IO3− and organoiodine). Further work is needed to adapt and improve current techniques to field scales, as well as further research into targeted application of emerging technologies.
Environmental significance
The presence of difficult-to-measure radionuclides (DTMRs) in groundwaters at nuclear sites poses significant threats to ecosystems and human health due to their long half-lives and high radiotoxicities. Addressing this remedial challenge is vital for achieving nuclear site end-state targets and minimizing environmental impacts. Our study critically evaluates current and emerging technologies for DTMR remediation, revealing that no single technique is universally effective for all DTMRs of focus, and highlights areas for further research. This work contributes to the broader understanding of DTMR remediation in the context of sustainable environmental management, emphasising the need for continued development of less invasive and more efficient remediation technologies to protect ecosystems and public health.
|
1 Introduction
Groundwater contamination at nuclear sites is extensive, containing many types of organic, inorganic and radioactive contaminants e.g. Hanford Site (Washington, USA)1 and Sellafield (Cumbria, UK).2 Some of these radioactive contaminants (e.g.137Cs and 60Co) emit high-energy gamma radiation during decay, making them detectable and quantifiable remotely using in situ gamma spectrometry.3 However, several isotopes found in groundwaters and soils emit alpha, beta or low-energy gamma radiation, making them challenging to identify using conventional, non-intrusive methods.4 Complex laboratory analysis is needed for detailed measurements of these Difficult to Measure Radionuclides (DTMRs), which include 241,240,239Pu, 129I, 99Tc, 90Sr, 14C, and 3H. Further, DTMRs have varying mobilities in groundwater, making their site distributions difficult to characterise. Development of targeted remediation and risk management strategies is consequently hindered, potentially reducing their efficacy. The range of mobilities also limits the application of scaling factors, a commonly used technique at nuclear facilities that involves measuring the ratio of an easy-to-measure radionuclide to a DTMR in a material, e.g. ratio of 137Cs
:
90Sr in a spent nuclear fuel container, and applies it to any on-site instance where that material is present, e.g. spent nuclear fuel leak in the subsurface.4 If, in this example, 90Sr migrates through groundwater slower than 137Cs, the two radionuclides will eventually form separated plumes in different areas of the site. When the leak is discovered, it will be assumed that the 90Sr is present with the 137Cs in the ratio previously ascertained. This means that the true 90Sr plume extent may remain undetected, whilst facility time and money is spent remediating the 137Cs plume for 90Sr despite its reduced activity in this area of the subsurface.
DTMRs are abundant at many nuclear sites worldwide. For example, at the Hanford Site, the most contaminated nuclear facility in the United States,5129I, 99Tc and 3H plumes covered 58 km2, 2 km2 and 58 km2 of land area, respectively, in 2021.6 The plume boundaries were defined by concentrations in excess of 0.037 Bq L−1 (1 pCi L−1) of 129I, 33.3 Bq L−1 (900 pCi L−1) of 99Tc and 740.0 Bq L−1 (20
000 pCi L−1) of 3H, which are upper limits of the drinking water standard set by the U.S. Environmental Protection Agency (USEPA).7 Similarly, in 2016, Sellafield borehole monitoring detected up to 164
000 Bq L−1 of 3H, over 16 times greater than the World Health Organisation (WHO) drinking water limits.2 The same borehole continued to exceed the drinking water limits in 2021, although the exact activity was not stated.8 Many DTMRs are long-lived and will remain a significant problem over human (and site operation and decommissioning) lifetimes, making effective remediation at nuclear sites a necessity for the protection of local populations and for eventual, successful, release of this land to public use following decommissioning.
Although all forms of land remediation were once considered beneficial (or their costs considered acceptable), it is now recognised that remediation technologies require careful planning and management to avoid excessive negative impacts on the environment.9 The Sustainable Remediation Forum UK (SuRF-UK) define sustainable remediation as “the practice of demonstrating, in terms of environmental, economic and social indicators, that the benefit of undertaking remediation is greater than its impact and that the optimum remediation solution is selected through the use of a balanced decision-making process”.10 International guidelines have been produced to encourage standard practices in sustainable remediation e.g. the American Society for Testing and Materials (ASTM) Standard Guide for Greener Cleanups11 and the International Organization for Standardization (ISO) Soil Quality – Sustainable Remediation procedures,12 incorporating a wider socio-economic outlook alongside the assessment of a technology's feasibility and implementation at a particular site. Sustainable approaches to remediation are also being introduced to nuclear decommissioning documentation e.g. Section 8.2 of the Nuclear Decommissioning Authority Strategy 2021,13 encouraging standard practices at a number of sites. Responsible clean-up in the nuclear sector is especially important, owing to the hazardous nature of materials held on-site, making sustainable remediation an important area for development.
Several reviews have been published on nuclear site remediation, including those by Hossain,14 Alby et al.,15 Sharma et al.16 and the International Atomic Energy Agency (IAEA),17 with the development of more sustainable techniques in recent years well-documented. However, DTMR-specific work focuses mostly on laboratory analyses e.g. IAEA4 and Thierfeldt and Deckert18 rather than in situ detection and removal, and consequently there are no comprehensive reviews on remediation for these nuclides. Technologies and applications focussing on in situ DTMR removal from groundwater remain underdeveloped compared to radionuclides that are easier to detect. This knowledge gap hinders the ability of assessors to fully remediate a site effectively, as numerous groundwater and soil samples must be obtained and tested. This is a slow, expensive, and labour-intensive process, as laboratory analysis increases the time taken for DTMR data to be collated and acted upon, slowing the implementation of immediate measures such as locating and isolating radionuclide leaks. Greater subsurface sampling also increases the risk to site operators, as working in close proximity to radionuclides for prolonged periods may expose workers to substantial dose.
Today, many nuclear sites are nearing or have reached the end of their operational lifetimes,19 and require decommissioning in order to achieve end-state land quality targets. At a number of these facilities, DTMRs comprise large proportions of the remaining radionuclides (examples given above), making effective, low-cost clean-up technologies vitally important for achieving remediation targets. Here, we review both conventional and sustainable remediation techniques at site-scale for four DTMRs that are highly mobile in groundwaters and compare advantages and disadvantages for each technology. Techniques such as subsurface walls (for example, as applied at the Fukushima Daiichi Nuclear Power Plant to limit off-site 3H transport20) have been excluded from this review as they do not actively remediate groundwater, but instead channel plumes to allow further radioactive decay to occur before off-site migration can happen. We aim to highlight knowledge gaps in DTMR remediation and inform future work by addressing key issues that remain in the management of these radionuclides and their risk. The reviewed DTMRs are 129I, 99Tc, 90Sr and 3H. These radionuclides have been selected as they either emit gamma radiation of <100 keV or do not produce gamma emissions during decay.21 In addition, 129I and 99Tc both have high abundances in nuclear wastes22–24 and long half-lives of 15.7 × 106 years and 2.11 × 105 years, respectively.21 As a result, hazard mitigation through natural attenuation is not feasible over human lifetimes, making effective nuclide remediation the only option for achieving nuclear site end-state targets. In comparison, 90Sr and 3H have much shorter half-lives and are likely to only present issues over decadal to century timescales but are included here due to their presence in large quantities at a number of nuclear facilities internationally. High abundances in wastes are especially pertinent for 3H and make development in its remediation globally important; frequent progression reviews can enhance the growth and implementation of new technologies to combat this problem.
2 DTMRs in the environment
2.1 Interaction between soils and groundwater
The movement of nuclides in the environment can vary depending on the conditions and materials present. The degree of ion mobility can be described using the partition coefficient (Kd; eqn (1)), which shows the ability of an ion to adsorb onto a given medium, rather than remaining in solution. Kd (mL g−1) is defined as: |  | (1) |
A Kd of <1 mL g−1 shows that negligible adsorption is occurring and the radionuclides are remaining in solution, whilst high Kd values (e.g. 1000 mL g−1 or greater) indicate a high level of contaminant sorption.
While the Kd test approach can provide a simple method for demonstrating the proportion of an ion in groundwater and soil, the approach has drawbacks. It is highly dependent on the starting concentrations of radionuclide, as a greater abundance of the contaminant will likely bind to the adsorption sites more quickly and result in the adsorbent reaching capacity in smaller volume of solution. The method is also influenced by various factors of environmental aqueous geochemistry (e.g. the preferential uptake of competing ions onto adsorption sites, pH, redox potential etc.),25 and groups additional attenuation processes, such as precipitation and diffusion into pores, into a single ‘sorption’ term.26 The Kd test method also assumes that adsorption is instantaneous and fully reversible which may not be true under real-world conditions.25 Despite the limitations, Kd test methods provide an intuitive method for comparing the extent of elemental partitioning between two media and are consequently popular in sorption-based literature (and are therefore utilised in this review).
2.2 DTMRs of focus
2.2.1 Iodine.
129I is a fission product of 235U (0.71% yield) with a half-life of 1.57 × 107 years.21 Significant groundwater contamination from 129I exists at the Hanford Site and Savannah River Site (SRS) nuclear facilities in the USA,6,27 although the isotope is also found in smaller concentrations at other facilities, such as Sellafield.2 The dominant forms of iodine in the environment are iodate (IO3−) and iodide (I−).28 Laboratory studies have shown that reactions between iodine and organic material in soils and groundwater (e.g. humic and fulvic acids29,30) lead to the formation of organoiodine (org-I) compounds, which can increase or decrease the environmental mobility of iodine depending on the products created.31 IO3−, I− and org-I compounds can co-exist in groundwaters, further complicating the behaviour and mobility of iodine in these environments.27 Typical Hanford sediments of quartz- and feldspar-rich sands have a low I sorption, with Kds of 0–8.1 mL g−1 and 0.8–7.6 mL g−1 for I− and IO3−, respectively.32–34 Quartz-rich surface aquifer sediments from SRS also have low I−Kds, ranging from 0.06 to 8.8 in starting solutions of 10−2 to 103 μM I−.31 These high mobilities in groundwater are a result of IO3− and I− existing as anions, which are repelled by the negative surface charges of material in soils at typical environmental pHs.35 Laboratory experiments have shown that IO3− can have Kds up to 90 times greater than I− when mineral phases such as iron oxides are present in soils.36,37 However, the aforementioned nuclear sites generally have low abundances of these in the subsurface, as reflected by the low iron content in experimental samples e.g. SRS31 and Hanford.34 Further, I−Kds remain almost unaffected in comparison to Hanford and SRS values, suggesting that iodine migration in groundwater would still be a concern at nuclear sites with higher iron oxide concentrations within the subsurface where I− is the dominant form of I present.
2.2.2 Technetium.
99Tc is a high-yield fission product of 235U (6.13%), with a half-life of 2.11 × 105 years.21,22 In oxidising conditions, Tc(VII) is the dominant species in the form of pertechnetate, TcO4−, whereas reducing conditions produce Tc(IV) and primarily form TcO2·nH2O,38 where n can range between 0.44 and 4.22.39 Tc(VII) has a high solubility and, hence, high mobility in water, with an approximate Kd of 0–1 mL g−1,22,32,40 but Tc(IV) is only sparingly soluble e.g. Kds of 15–280 mL g−1 have been estimated for sediment at Forsmark and Laxemar-Simpevarp sites in Sweden.41
2.2.3 Strontium.
90Sr is another high-yield 235U fission product (5.73%) with a 28.91 year half-life.21 The chemistry of Sr is similar to that of Ca,42 with Sr often existing in aqueous environments as a divalent ion that is either hydrated or bound to small organic compounds.43 Sr(II) has been found to have Kds of 10–25 mL g−1 in quartz-rich Hanford sediment.44 However, mobility can be reduced through sorption onto fulvic and humic acids45 and clays, resulting in much higher Kds e.g. ∼103 estimated for the subsurface at Sellafield.46
2.2.4 Tritium.
3H is an activation product with a half-life of 12.32 years.21 It results from collisions between neutrons and stable 2H nuclei found within reactor materials, such as control rods and water coolant.24 These materials are commonplace at nuclear facilities, and so 3H remobilisation and leakage during storage is a risk for sites globally.473H is also generated through ternary fission, or through neutron interaction with boric acid dissolved in the coolant/moderator of pressurised water reactors. 3H exists as tritiated groundwater at nuclear sites,2,48 resulting from isotopic exchange between 3H and 1H2O already present in groundwater.40 As a result, the Kd for 3H is considered to be 0 mL g−1, although slight sorption in sandy soils (Kd range of 0.04–1 mL g−1) has been previously reported.49 In some circumstances, where organically-bound tritium (OBT) is the dominant form of 3H present (e.g. in discharges from radiopharmaceutical facilities), greater retention of 3H on solid phase materials may be observed.50
Key characteristics of each of the above DTMRs are given in Table 1. All of 129I, 99Tc, 90Sr and 3H emit beta particles,21 which can result in health issues for humans if significant quantities build in the body through ingestion or inhalation. For example, thyroid cancers can be caused by both 129I51 and 99Tc52 exposure, and skeletal abnormalities can develop as a result of 90Sr incorporation into bones.53 Whilst 3H does not accumulate in specific parts of the body, the 10 day and 40 day biological half-lives of tritiated water and OBT, respectively, make the radionuclide a potential hazard once inside humans.54 Defining the specific risk that DTMRs pose can be complex due to a number of variables, including the exposure pathway to an individual (inhalation, ingestion, skin contact, etc.), the body part(s) that come into contact with radiation, the age of the person (children and the elderly may potentially be more affected compared to adults), etc.55 This has led to differences in the dose limits and risk management guidelines given by regulatory bodies at both national and international levels. Many countries regulate radiation exposure based on IAEA recommendations and WHO guidelines, although the USA is a notable exception to this with the Environmental Protection Agency (EPA), Department of Energy (DOE) and Nuclear Regulatory Commission (NRC) overseeing public and nuclear site worker protection. The limits and recommendations set by these organisations provide clarity on the end-state targets for remediation projects and can also determine the suitability of a particular technology for the clean-up or risk schemes implemented.
Table 1 Summary of the radionuclides of focus
|
129I |
99Tc |
90Sr |
3H |
Half-life (years)21 |
15.7 million |
211 100 |
28.91 |
12.32 |
Gamma decay energy (keV) [absolute intensity (%)]21 |
39.6 [7.51] |
89.5 [6.5 × 10−4] |
No gamma emitted |
No gamma emitted |
Oxidation state(s) in groundwater [speciation] |
+5 [IO3−]28 |
+7 [TcO4−]22 |
+2 [Sr(II)]43 |
0 [3H1HO]2 |
−1 [I−]28 |
+4 [typically TcO2]38 |
0 [3H2O]2 |
Typical Kds (mL g−1) |
0.8–7.6 [IO3−]33 |
0–1 [Tc(VII)]32 |
10–25 [quartz-rich]44 |
0–1 [all sediment]49 |
0–8.1 [I−]32,33 |
15–280 [Tc(IV)]41 |
∼103 [clays]46 |
3 Current treatment methods
3.1 Pump and treat
Pump and treat (P&T) is the most commonly applied method for ex situ groundwater clean-up at nuclear sites.56,57 The technique works by pumping groundwater from the subsurface and treating it through adsorption, ion-exchange or chemical (e.g. redox) processes (Fig. 1). Decontaminated water can then be discharged at the surface or pumped back into the ground.58 The present-day appeal of the technique for site remediators is its proven track record of generally remediating facilities to a desired level.59 P&T can be very effective at removing groundwater-mobile elements (e.g. Cr(VI)60) but is less effective for elements that strongly sorb to sediments (e.g. Pu and Am61). Subsurface permeability can also affect remedial efficiencies, with finer-grained media requiring longer time periods to reach the same clean-up goals as coarser sediment.62 This can further complicate remediation in substrates with dual porosities (caused either through materials with differing permeabilities or as a result of a variation between matrix and fracture porosities). The technology also incurs large financial costs during both the initial setup and operation and maintenance phases, making only long-term projects economically viable.
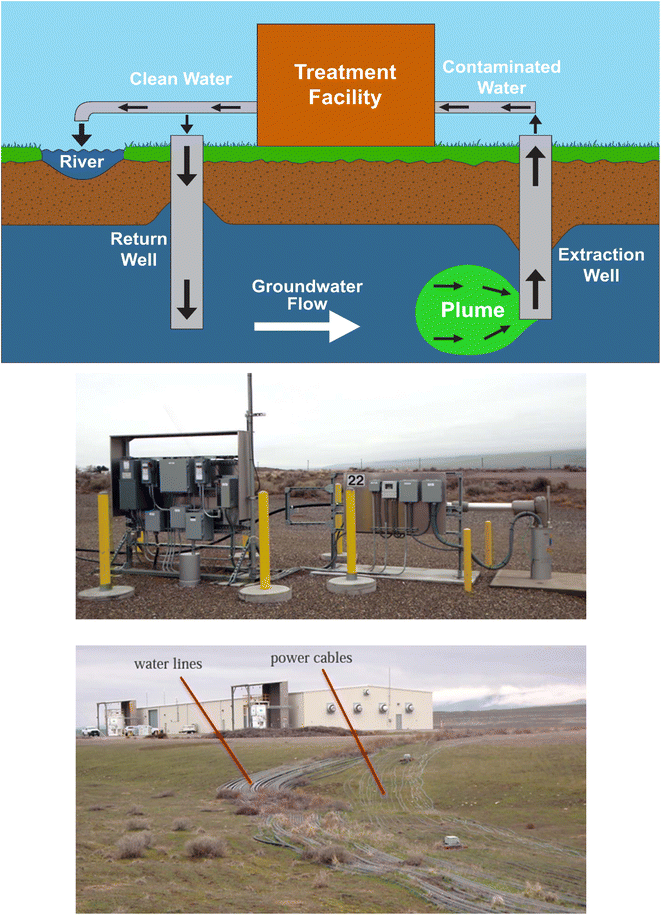 |
| Fig. 1 Top – Diagram illustrating the P&T process. The plume of contaminated groundwater (green) is extracted and treated, before being either pumped back into the subsurface or released as surface waters. Middle – Photo from Mackley et al.58 showing a typical P&T extraction well at Hanford, with monitoring equipment attached. Bottom – Photo from Mackley et al.58 showing water pipes and power cables running between a Hanford P&T facility and monitoring wells. | |
Adsorption is performed by pumping extracted groundwater through a column filled with one or more adsorbent materials such as clay minerals, activated carbon, iron oxides and metal–organic frameworks.63,64
Radionuclides bind to the surface of the adsorbents, whilst groundwater passes through the column. Ion exchange is a similar process, but the adsorbent contains a non-toxic ion, such as Cl−,65 that counter-balances the charge of the adsorption sites.66 If the radionuclide has a greater electrochemical potential than the starting ion, the two are exchanged and the radionuclide is retained on the adsorbent.
Site-scale iodine remediation through P&T has not been effectively implemented at nuclear facilities, despite 129I plumes being present at sites such as Hanford and SRS.28 Laboratory trials of various materials including Purolite A530E ion exchange resin and granular activated carbon (GAC) were performed at Hanford, with Kds of 78 and 76 mg L−1 observed, respectively (in simulated Hanford groundwater containing 1.0 mg L−1 IO3−).67,68 However, none of the tested adsorbents will be efficient enough to decrease the activity below 0.037 Bq L−1 (1 pCi L−1; 6 μg L−1) – the USEPA drinking water standard67 – before the area's active remediation period ceases in 2047.69 The poor 129I uptakes were generally caused by the adsorbents' preference for I− over IO3−, whilst on-site groundwater conditions favour IO3− speciation over I− (77.5% of stable iodine at the site exists as IO3−, 19.6% as I− and 2.6% as org-I).67 Due to being only a small percentage of total 129I speciation67 and having a variety of potential properties depending the organic molecules present,70 laboratory studies do not focus of determining Kds for org-I for Hanford. As a result of the poor adsorbent uptake capacities, as well as alternative remedial technologies also being ineffective, there is no current strategy for long-term 129I clean-up of the Hanford site.71 A Technical Impracticability waiver has therefore been drafted for the USEPA.72
Adsorption of technetium, in combination with other contaminants, onto Purolite A532E and DOWEX 21K ion exchange resins is being utilised at the Hanford Site.73,74 Groundwater P&T influent streams are pumped into the facility where they are passed through a train of DOWEX columns followed by A532E columns, before being released back into the subsurface.73,75 DOWEX was first installed into the P&T system in 2015 and is used primarily for U adsorption.75 As a result, despite laboratory Kds measuring 1800–19
800 mg L−1 in double deionised water (DDI) containing NO3− (90–99% uptake to resin; 0–104 mM NO3−; all with 200 ppb 99Tc) and >19
800 mL g−1 in DDI containing SO42− (>99% uptake; 0–10 mM SO42−; all with 200 ppb 99Tc),74 resin used in the Hanford P&T columns between 2015 and 2017 showed no Tc adsorption.75 By contrast, Purolite A532E has been chosen for targeted 99Tc uptake, and is the successor to the A530E resin, a microporous form of the A532E73 which had a 92.1% 99Tc removal efficiency in the Hanford P&T facility in 2020.76 No information on A532E Tc uptakes in the same P&T system could be found, although Saslow and coworkers have recorded Kds of >19
800 mL g−1 (>99% resin uptake) in double deionised water containing 200 ppb 99Tc.73 This figure remained constant with NO3− and SO42− present at concentrations up to 20 mM and 10 mM, respectively, exceeding the levels seen in P&T influent streams (1.61 and 0.60 mM for NO3− and SO42−, respectively) between 1st June 2021 and 30th May 2022 (data cited in ref. 73). Whilst this high degree of 99Tc selectivity shows promise for A532E's application at site scales, there is a need for larger pilot trials to support results of simplified laboratory set ups. As the P&T system continues to operate at Hanford, future work by Saslow and coworkers will look at the performance of both A532E and DOWEX 21K with flowing groundwater, and scale up models to predict the efficacy with varying P&T influent chemistry.
P&T remediation was also attempted for the removal of 90Sr plumes from the Brookhaven Graphite Research Reactor (BGRR), former Pile Fan Sump (PFS) and the Waste Concentration Facility (WCF) areas at Brookhaven National Laboratories (BNL) in 2005.77 Although Sr Kds in the sediment were high enough to indicate that plume migration off site was unlikely, the project was initiated to avoid public concerns over a managed natural attenuation approach.78 Maximum pre-treatment 90Sr activities for the BGRR, PFS and WCF plumes were 116.6 Bq L−1 (3150 pCi L−1; recorded in 2003), 20.9 Bq L−1 (566 pCi L−1; recorded in 1997) and 57.7 Bq L−1 (1560 pCi L−1; recorded in 2003), respectively.77 By the end of 2021, borehole activities had decreased to 0.47 Bq L−1 (12.7 pCi L−1), 0.88 Bq L−1 (23.9 pCi L−1) and 1.93 Bq L−1 (52.2 pCi L−1) in the BGRR, PFS and WCF – reductions of 99.5%, 96% and 97%, respectively.79 The ion exchange resin used, and its respective Kd, have not been disclosed publicly by the site. Plume remediation efforts were assisted by the low mobility of Sr in the sediment, allowing the most contaminated subsurface areas to be easily identified and targeted. However, between 2003 and 2021 the plumes split into multiple smaller entities, possibly as a result of remediation efforts targeting and removing the highest-activity zones which are typically in the middle of plumes, making the residual contamination difficult to track. Further, plumes had migrated underneath buildings which complicated monitoring. As a result, 90Sr activities are anticipated to be higher in certain areas where monitoring wells are not currently set up, although the areal extent of these high-activity regions are thought to have decreased significantly compared to 2004 plume boundaries.79 The remediation project aims to reduce 90Sr activities to below the 0.3 Bq L−1 (8 pCi L−1) drinking water standard by 2070.
P&T is ineffective for 3H remediation as the radionuclide can be exchanged with protons in water.2,48 Adsorbents and ion exchange resins cannot distinguish between 1H2O, 3H2O and 3H1HO, rendering P&T unsuitable for 3H clean-up projects. Despite this, sites such as SRS and Brookhaven have previously attempted to pump tritiated water up-gradient of a plume and keep it in a continuous loop until radioactive decay reduces the activities to acceptable levels.80 However, the SRS system was costing $1
000
000 per month to maintain and, in the process, was potentially mobilising more contamination.80 The operations were ceased after 6 years, with approximately $50 million having been spent on setup and $100 million on maintenance over their lifetimes.
3.2 Permeable reactive barriers
Permeable Reactive Barriers (PRBs) are a relatively inexpensive, passive and in situ remediation method for groundwater. They consist of a reactive medium that is placed into the subsurface, perpendicular to the direction of groundwater flow (Fig. 2).81,82 The reactive medium converts contaminants into an immobile or less harmful form, whilst allowing groundwater to pass through the barrier.81,83
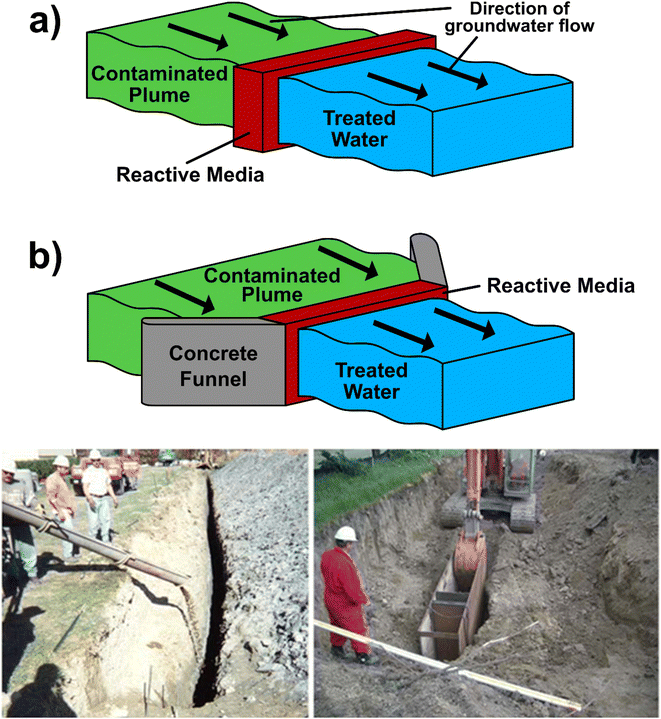 |
| Fig. 2 Top – Diagram illustrating permeable reactive barriers in (a) continuous and (b) funnel and gate configurations at the Oak Ridge Y-12 Site, Tennessee. In both instances, contaminated groundwater passes through a reactive medium, where contaminants are removed, and clean water passes out the other side. Funnel and gate configurations have the addition of concrete barriers to channel groundwater flow. Bottom – Photos from ITRC82 showing unsupported (left) and temporary trench box (right) excavation during PRB installation at non-nuclear industrial sites. Reactive media is poured into the hole to complete the PRB. | |
Records of iodine remediation through PRBs at nuclear sites could not be found. This is surprising considering substances such as organic material may appear as good candidates for a low-cost reactive medium. Covalent bonding and complexation of I onto organic material such as humic acid has been observed at bench scale84,85 and in field observations at facilities such as Hanford86 and SRS.5 However, the lack of application is likely due to a poor understanding of interactions between iodine and natural organic matter87 in addition to its susceptibility to redox reactions within organic compounds, caused by microorganisms and materials within the subsurface.88 Bench-scale experiments performed on organic-rich SRS soil have shown conversion of inorganic I into particulates, colloids and dissolved org-I after a 31 day equilibration period.89 This is thought to be caused by the oxidising and reducing capacity for I− and IO3− in the soil, creating reactive intermediates such as I2 and HOI during the conversion between the iodine +5 and −1 species. These intermediates may subsequently react with organic material before they can be converted back to I− and IO3−. Due to the variety of water solubilities that organic molecules can have, long-term iodine immobilization could be compromised depending on the organic material present, making it an unsuitable adsorbent.
99Tc is amenable to PRB remediation via reduction due to the variation in solubility between Tc(VII) (as TcO4−; soluble in water) and Tc(IV) (as TcO2; solid).38 When Tc(VII) in groundwater comes into contact with a suitable reactive barrier, it may be reduced to Tc(IV) which facilitates precipitation, immobilising the Tc and limiting its environmental spread.90 Examples of suitable reactive barrier materials include zero-valent iron (ZVI), a cheap, frequently used medium in PRBs.91 Laboratory experiments on ZVI obtained a Kd of 2700 mL g−1 for 99Tc at an initial concentration of 38.2 Bq L−1 (1031 pCi L−1).92 ZVI adsorbents have been deployed at scale, for example, in two PRBs at the Oak Ridge Site, Tennessee, 1997, to chemically reduce and immobilise Tc.93 In this study the radionuclide was found within the Bear Creek Valley and Upper East Fork Poplar Creek watersheds, both comprising of Maynardville Limestone.94 The first PRB, referred to here as ‘PRB 1’ (for configuration see Fig. 2a), was a 68 m long × 6–9 m deep section comprising of 80 tonnes of ZVI, and the second, ‘PRB 2’ (for configuration see Fig. 2b), was a 67 m long × 8 m deep trench containing 1500 L of ZVI and peat moss.93 Monthly testing over a 3 year period established that the two PRBs reduced Tc levels in groundwater, although final concentrations were not publicly reported. However, precipitation of iron-based minerals decreased permeability in both barriers, reducing the lifespan of PRB 1 to between 15 and 30 years, whilst causing PRB 2 to completely cease operations within an unspecified time period.
A typical setup for a PRB requires infrastructure to contain the reactive medium, with optional features such concrete walls (Fig. 2b) that channel water flow to be added at the site remediators' discretion. However, a less invasive approach was adopted at the Hanford site to reduce the 90Sr activity reaching the Columbia River.95 A solution of Ca, citrate and PO4− was added to the groundwater through boreholes,96,97 creating a diffuse, continuous calcium phosphate (apatite) barrier that formed in situ from a series of discrete points at the surface.98 Once formed, Ca in the apatite will substitute with Sr in the plume, as the existence of strontiapatite is more thermodynamically favourable than the initial hydroxyapatite.99 Four pilot boreholes were initially injected between 2006 and 2008, with pre-barrier activities ranging from 36.5 Bq L−1 to 171.3 Bq L−1 (972 pCi L−1 to 4630 pCi L−1) 90Sr.6 As of 2021, activities within the boreholes have dropped to 4.1–23.5 Bq L−1 (111–635 pCi L−1) – a decrease of 77–91% compared to the respective pre-barrier maximum 90Sr activities. The variance in reduction efficiencies is likely caused by a variability in the apatite concentrations surrounding the boreholes. To avoid a significant loss of apatite over time, the mineral constituents are reinjected into boreholes with increasing 90Sr activities, allowing specific areas of the barrier to be targeted and rejuvenated without the need to dig up large sections of the barrier. 3H is not amenable to PRB attenuation for two reasons; the technique is typically limited to redox-sensitive elements that have variable solubilities in groundwaters, and tritiated groundwater is permeable to PRBs.
3.3 Phytoremediation
Phytoremediation is an in situ, passive and low-cost method that uses plants (and their associated microorganisms) for remediation over medium- to long-term periods.100,101 The technique involves the use of at least one of the following main processes102,103 (Fig. 3):
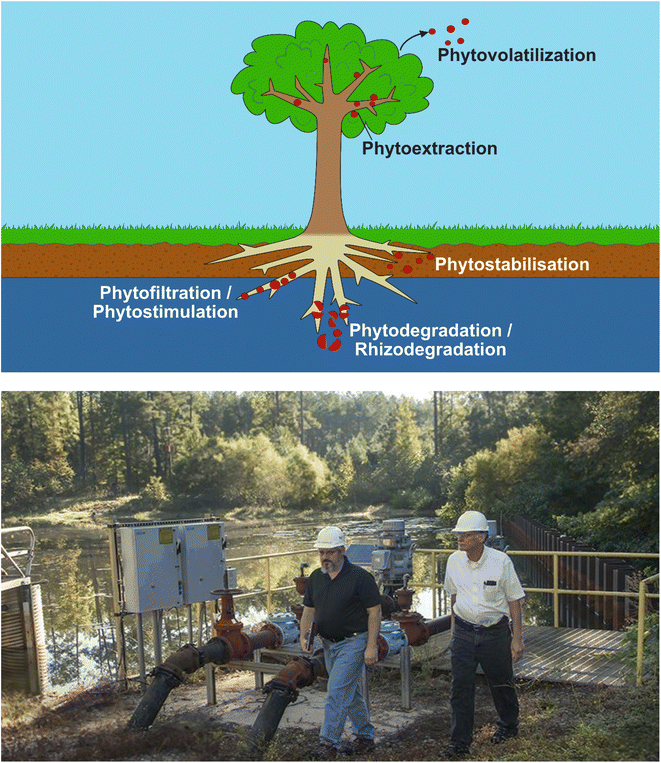 |
| Fig. 3 Top – Sub-processes of phytoremediation, showing phytovolatilization, phytoextraction, rhizofiltration and phytostabilization. 3H is released into the environment through the former-most option, diffusing into the atmosphere through leaves rather than being expelled by the roots. Bottom – Photo from DOE112 showing Savannah River Nuclear Solutions engineers examining pumping equipment next to the 3H-contaminated groundwater storage pond. Water is pumped from the pond and used to irrigate the surrounding woodland, where evaporation and phytovolatilization release 3H into the atmosphere. | |
(1) Phytoextraction – removal of contaminants from soils and shallow groundwaters and accumulating them in above-ground shoots.
(2) Phytovolatilization – extraction of volatile radionuclides from soils and subsequently releasing them in a gaseous form.
(3) Phytostabilisation – reduction of a contaminant's bioavailability within the subsurface.
(4) Phytofiltration – filtration of contaminants by plant roots or seedlings through adsorption or absorption.
(5) Phytostimulation – removal of contaminants through plant roots and their rhizospheric microorganisms.
(6) Phyto/rhizodegradation – degradation of organic contaminants by plants/rhizospheric microorganisms.
129I has been considered as a target contaminant for phytovolatilization, but concerns have been raised over the health risks associated with its bioaccumulation within humans if inhaled.104 As a result, phytoremediation of 129I has not been performed at nuclear sites. 99Tc can also be theoretically remediated through phytoextraction, but is hindered by sulfate competition caused by preferential SO42− binding over TcO4− in amino acids residues within the roots.105
Whilst a number of phytoremediation schemes have been performed in proximity to nuclear sites (e.g. Fukushima106 and Chernobyl57), fewer examples exist for direct on-site applications. However, a 1999 investigation at Sellafield examined the use of spear thistles (Cirsium vulgare) and broad-leaved docks (Rumex obtusifolius) for 90Sr uptake.107 It was found that the thistles removed 64–89% of 90Sr, with a mean efficiency of 84%, whilst the docks extracted 50–87% of 90Sr, with a mean removal rate of 76%. The large variation in Sr removal for both species is likely caused by intra-specific competition, in addition to site heterogeneities including microbial activity and soil composition. Further discussion and additional examples of 90Sr phytoremediation are described in Purkis et al.108
Phytovolatilization of 3H has been utilised at the SRS, South Carolina, in conjunction with evaporation.109,110 Tritiated groundwater from the on-site Old Radioactive Waste Burial Ground was continuously brought to the surface by a spring, discharging between 15
170 Bq L−1 and 24
235 Bq L−1 (410
000 pCi L−1 and 655
000 pCi L−1) of 3H into the nearby stream, the Fourmile Branch.109 These levels are between 20 and 32 times the 740.0 Bq L−1 (20
000 pCi L−1) 3H Environmental Protection Agency drinking water standard.111 A dam was built downstream of the spring which diverted water into a storage pond used for irrigation of the surrounding 89
000 m2 of mixed woodland80,112,113 (Fig. 3). During the irrigation process, 3H is released into the atmosphere through a combination of phytovolatilization, after being taken up by tree roots, and evaporation from the dam and woodland floor. Closure of the dam in 2000 caused a 71% decrease in 3H levels (to 7067 Bq L−1; 191
000 pCi L−1) in the stream within 2 weeks, and a 82% decrease (to 4329 Bq L−1; 117
000 pCi L−1) within 10 months.109 The long-term success of the system resulted in an additional 78 acres of pine trees being planted, greatly expanding the irrigation project.110 The general downward trend of average 3H activity in the Fourmile Branch has continued over the last decade, with 2021 data showing only ∼629 Bq m−3 (∼17
000 pCi L−1) of 3H111 – a 97% reduction from the 24
235 Bq L−1 of 3H recorded shortly before the dam closure in 2000. In addition, the volatilization of 3H poses minimal risk to human health, as shown by a 2019 maximum on-site concentration of 28.19 Bq m−3 (762 pCi m−3) in air, decreasing to a maximum of 0.99 Bq L−1 (26.7 pCi m−3) in air at the site perimeter. The 97% reduction to ∼629 Bq L−1 in the Fourmile Branch is enough to reduce concentrations below the 740.0 Bq L−1 Environmental Protection Agency drinking water standard for 3H.111 Furthermore, because this project also utilises evaporation, 3H remediation caused exclusively by the phytovolatilization component is difficult to practically determine and, hence, is not specifically reported in the literature.
4 Emerging treatment methods
In efforts to produce more effective and sustainable remediation, additional techniques have been applied at nuclear sites over recent decades. These technologies have been used less extensively than those previously mentioned but could provide an alternative, viable, approach if more traditional remediation strategies are deemed unsuitable for a given scenario.
4.1 Bioimmobilization
Bioimmobilization involves the use of bacteria or plants to decrease the water solubility of aqueous contaminants through changes in oxidation state and therefore promoting precipitation of solid phases.114,115 The process can be performed either through the introduction of new organisms to an area or by promoting the growth of organisms already present in the target environment.114,116 Field-scale, in situ bioimmobilization of 99Tc was conducted at the Oak Ridge site by Istok and coworkers in 2004.114 It was suggested that chemical reduction of radionuclides including Tc could be performed by microbial colonies already present within facility sediments. Although the microorganisms required for this are apparently present within the subsurface,117 factors such as competing electron acceptors (e.g. NO3−) and numbers of microbial colonies within the sediments limit the efficiency of Tc reduction.118 Further, reducing conditions are required within the subsurface, both for promoting the growth of metal-reducing bacteria (e.g. Geobacter) and for maintaining Tc in its insoluble 4+ oxidation state.114 These anaerobic conditions can be generated by the addition of electron donors into a system, allowing bacteria to reduce NO3− and, subsequently, metals for respiration in the absence of O2. In this field trial, single-well, push–pull tests were used to inject multiple rounds of ethanol (acting as an electron donor) into a shallow aquifer to monitor 99Tc reduction. All results were corrected to account for the Tc dilution that occurred when the clean test solution mixed with contaminated borehole groundwater. Initial tests showed that Tc(VII) could not be reduced when NO3− was present, as microorganisms would preferentially reduce NO3− during respiration. However, the first ethanol injection resulted in Tc(VII) concentrations reducing from ∼13
200 pM to ∼5800 pM (56.1% reduction) within 400 hours and to ∼3700 pM (72.0% reduction) within 600 hours. In comparison, NO3− concentrations dropped from ∼140 mM to ∼40 mM (71.4% reduction) within 400 hours and ∼25 mM (82.1% reduction) by 600 hours. Multiple injections were performed into the same borehole over several months, with the seventh ethanol injection causing a Tc(VII) reduction from ∼12
000 pM to ∼1000 pM (91.7% reduction) within 400 hours, although no further data were collected after this time period. Concurrently, the initial ∼125 mM of NO3− dropped to negligible levels (∼100% reduction) after ∼35 hours and stayed constant for the following ∼365 hours. After the seven biostimulating ethanol injections, a test solution was created without the electron donor, and inserted into the borehole. The absence of ethanol had no negative effect on Tc(VII) reduction, with concentrations decreasing from ∼11
000 pM to ∼1000 pM (90.9% reduction) within 400 hours and remaining constant for up to 500 hours post-injection. Initial NO3− concentrations of ∼120 mM dropped to ∼70 mM (41.7% reduction) within 400 hours and ∼35 mM (70.8% reduction) by 600 hours. These experiments show that in situ99Tc reduction is possible when NO3− is simultaneously consumed through bacterial denitrification; promoted via the use of cheap electron donors such as ethanol. Despite this, there does not appear to have been further work on this at site scales. In addition, pilot-scale bioimmobilization studies for 129I, 90Sr and 3H could not be found. In addition to the reasons given for their unsuitability regarding phytoremediation (see Section 3.3) the absence of 129I, 90Sr and 3H studies is likely because the dominant chemical species present in water for 129I (5+ and 1−) and 90Sr (2+) are all highly soluble and would therefore have further requisites for immobilization to occur (adsorption sites, reactive media, etc.), whilst tritiated water would not be immobilised as it cannot be distinguished from light water with this technique.
4.2 Chemical/colloid injection
Injection is the process of inserting a material into the subsurface that can either manipulate the oxidation states of pollutants to promote their precipitation, or provide active sites for contaminants to adsorb onto.119 The injected material can be chosen to remove multiple compounds simultaneously, such as variants of zeolites that can treat heavy metals,120 or to target specific contaminants, such as Savannah River Site's project for multiple submicron AgCl injections to treat a migrating 129I plume.121 AgCl was intruded into the subsurface as it can react with this radionuclide to form Ag129I, which is insoluble in water and, thus, is immobilised and retained within the aquifer.122 The first set of injections occurred throughout 2011, in 7 temporary boreholes that were distributed in an approximately semi-circular shape with a diameter of ∼70 m. Only the well closest to the injection sites showed a definitive 129I decrease after treatment. Activities for this well between October 2009 and October 2011 averaged at 5.0 Bq L−1 (135 pCi L−1), but decreased to 2.9 Bq L−1 (77 pCi L−1) shortly before the injections.121 Measurements of 1.7–2.6 Bq L−1 (46–70 pCi L−1) were recorded in the first 6 months, before a sharp increase to 3.7 Bq L−1 (99 pCi L−1) in the 7th month. A range of 2.9 to 4.0 Bq L−1 (79 to 109 pCi L−1) was reported for the remaining 5 months of monitoring. All other wells, which were further away from the injection boreholes, show ambiguous or no evidence of 129I activity reductions, suggesting that remediation is only effective within close proximity to the injection sites. Ag migration data indicated limited particle movement after subsurface deposition. Of the total AgCl solution volume that was permitted to be injected in 2011, only half was used. As a result, the remaining volume was used in 2015 to boost the project's long-term efficiency.122 7 new boreholes were used to concentrate the AgCl barrier to the east of the original wells, with the 2015 group spaced across ∼35 m. It was reported that 5 of the 7 wells had 129I decreases between 25% and 65% and one well upgradient of the barrier detected no change, with the final borehole not discussed.122,123 However, activities for each of the wells were not provided in these reports, and other possible sources for raw borehole data are not publicly available, meaning the percentage reductions given here could not be independently verified. Given the apparent success of both 2011 and 2015 injections, a third round was approved in 2018 and performed in 2019.123 The insertion site distribution consisted of 15 wells with a broadly linear configuration spanning ∼35 m, aligned perpendicular to groundwater flow. This was based on the 1.2–2.4 m AgCl diffusion radius that formed around previous boreholes, allowing the formation of a single, continuous zone in the subsurface122 comparable to Hanford's 90Sr permeable treatment barrier (see Section 3.2). Results from the most recent injections will be disclosed in the site's 2022 Corrective Action Report.123 Subsurface AgCl insertion will continue until 2040, when the area will transition to natural monitored attenuation.110 However, whilst this technique shows promise at SRS, applications on other nuclear sites may not be feasible due to the toxic nature of Ag,88 and so careful consideration and planning must be taken to avoid adverse consequences on the ecology and surrounding environments of nuclear facilities.
4.3 Combined electrolysis catalytic exchange
Combined Electrolysis Catalytic Exchange (CECE) is a 3H remediation technique for detritiating effluent and groundwaters.124 Contaminated feed water is pumped into a containment cell and electrolysed, converting liquid H2O into H2 and O2 gases. The energy required to electrolyse tritiated water is greater than that required for light water, meaning that 3H will concentrate in the remaining feed solution whilst light water is driven off. Deionised water is also added to the system to recapture any 3H that is electrolysed, as the radionuclide will preferentially exist as liquid H2O over gaseous H2.125 Pilot-scale trials have shown high detritiation factors e.g. >100
;126 1000 when used with heavy water – a more difficult separation than with light water.127 However, the system is expensive to operate and at present is not able to remediate large volumes of water, making it unsuitable for processing large water flows or volumes with relatively low 3H activities.126 The application of CECE has been evaluated for 3H contaminated groundwater at the Fukushima site (known as ALPS-treated water) but, due to the high cost and small treatment volumes, the technology was deemed unsuitable for 3H remediation126 and these waters will instead be discharged to sea.128 For similar reasons, the 2022 Hanford 3H wastewater treatment review determined that whilst the Modular Detritiation System® (a commercialised version of the CECE process) shows promise for effective 3H removal in the future, the modifications required for use with large volumes of liquid make it currently infeasible for on-site application with groundwaters.129
5 Comparison of main techniques
The “tried and tested” attitude towards P&T at nuclear sites makes it appealing for site remediators. Many government-funded remediation projects are ultimately paid for by the taxpayer, who want cheap and effective technologies that optimise project efficiencies. The financial, and potentially political, consequences of a technique that is ineffective or that mobilises additional contaminants could be high, often make P&T the most attractive option. In addition, the technique allows for convenient testing of groundwater before discharge, ensuring that remediation targets are always being met. However, P&T can be expensive to operate due to the infrastructure required for an ex situ technique. This was highlighted in a 2001 study on 32 United States Environmental Protection Agency (USEPA) P&T sites in North America, where the average capital and yearly operational costs were $4
900
000 and $770
000, respectively at 2001 prices.130 Site selection criteria included the presence of a full-scale remediation system and operator reports on aquifer clean-up targets and technology costings. Although the technique is expensive, the total cost for each project will depend on the price per unit of reactive medium as well as the frequency at which the medium needs to be replaced. Further, multiple types of reactive media may be required if different contaminants are to be treated simultaneously, further increasing operational costs, but this can reduce the remediation timeframe in comparison to using a single, less selective medium that removes a lower proportion of radionuclides in favour of competing ions such as HCO3−, NO3−etc.
The passive nature of PRBs offers benefits over P&T including a decrease in the number of staff required for daily operation, lowering the long-term function and maintenance costs, and minimal infrastructure, which reduces initial costs. 15 USEPA facilities in North America and an additional site in Northern Ireland were also examined in the EPA130 P&T study, and their costings compared. The selection criteria for the survey required a full-scale system with capital costs provided, although annual maintenance costs were a necessity as only 2 of the sites had been operating long enough for accurate prices to be determined. The average capital costs for the 16 PRB sites was $730
000 – more than 6 times cheaper than the average P&T capital costs, as determined by the same study. Annual operational and maintenance costs of $78
000 and $120
000 were recorded for the US National Coast Guard Support Centre (North Carolina) and the Intersil (California) sites. Although the lack of case studies reduces the certainty as to whether these costs are truly reflective of PRB projects, both available examples are ∼7 times lower than the $770
000 equivalent for P&T. From this study, PRBs appear to be a much cheaper alternative to P&T but multiple factors can influence the final cost, including the price of the reactive medium and the lifetimes of both the barrier and the wider remediation project. PRB placement is important for financial and remedial success, as the technique can only remediate groundwater that passes through it, making it unsuitable for contamination spread over large areas. Consequently, the decision as to whether a PRB or P&T is the best remediation approach will be highly site-specific. An updated version of a cost comparison report for different remediation techniques could not be found. Further, no list of remediation techniques deployed specifically at nuclear sites could be obtained, and only one site in the EPA report included radionuclide clean-up (Oak Ridge Site, Tennessee (see Section 4); capital costs of $900
000). This highlights the absence of financial data available to site operators when designing remedial strategies and demonstrates the need for readily accessible information to promote more sustainable options in the nuclear industry.
The primary appeal to phytoremediation is its passive nature, although active enhancements such as the SRS irrigation system can boost the removal efficiency (and may be necessary in some cases to allow plant establishment and growth). A direct result of passive techniques are the relatively low costs compared to conventional pump and treat methods. For example, implementation of the irrigation system at the SRS came at an initial cost of $5
000
000, with an additional $500
000 needed per year.80 In comparison, the average set up cost for 32 American P&T sites was $4
900
000, followed by $770
000 per annum. Although these figures are similar, phytoremediation without an irrigation system would drastically reduce the overall cost and make the technique much more financially favourable. Additional, unique benefits can come from phytoremediation projects, including greater protection from flooding, improved site aesthetics and supporting or reintroducing native plant and animal species to the local area.131 However, the depth at which remediation can occur is limited to the depth of plant roots, only making it a suitable alternative to P&T in shallow groundwaters. The same constraint applies to areal extent, meaning that large areas of contaminated land would require large numbers of plants for remediation to be effective. The long-term chemical and physical forms of targeted radionuclides must be understood before implementation, as processes such as phytovolatilization may mobilise contaminants that pose a greater risk to wildlife and humans in air than when retained within sediments (or within the subsurface). Further, phytoremediation is only able to extract radionuclides that are taken up by the plants' roots, resulting in contaminants with lower bioavailabilities remaining in the subsurface. In comparison, P&T can extract any radionuclides that are mobile in groundwater regardless of their bioavailability, takes up less space at a site and extracts groundwater from any depth, although higher costs may be associated with deeper extraction wells.
Both phytoremediation and PRBs had a range of field-scale pilot projects performed in the late 1990s and early 2000s,48,93,132 likely as a result of the globally increasing costs and tightening of restrictions associated with the ‘dig and dump’ approach to contaminated materials.133 Although each technique on its own is unlikely to be used in favour of P&T for simultaneous treatment of all DTMRs discussed here, a combination of these more sustainable methods may be superior to conventional remediation; for example, phytovolatilization and PRBs could be used to remove 99Tc, 90Sr and 3H with minimal upkeep and maintenance. However, limitations of these practices, such as the area of land available and radionuclide selectivity of each technique, make this unsuitable for groundwater application on the majority of nuclear sites. This highlights the need for new techniques that can be used in combination with PRBs and phytoremediation to enhance the number of DTMRs that can be remediated in a sustainable way. Emerging in situ technologies under active development for DTMRs include electrokinetic remediation134 and silica grouting,135 although further work is needed to show efficacy for both DTMRs and for field-scale application.
6 Conclusions
In line with the growing demand for sustainable remediation at nuclear sites, we have reported on techniques for the removal of DTMRs from groundwaters. Whilst a variety of possible remediation techniques exist for 129I, 99Tc, 90Sr and 3H, the differing physical and chemical properties of these DTMRs result in a need for site-specific implementations. P&T is, by far, the most commonly applied technique and can effectively remediate 99Tc and 90Sr. However, 129I and 3H cannot be treated by this method, as current adsorbents are not capable of effective 129I uptake, and 3H2O and 3H1HO are not differentiable from groundwater H2O. In situ techniques can also be applied for remediation, although their application should be evaluated to determine their suitability on a site-by-site basis. PRBs (including colloidal or chemical injection systems, such as that applied at the Hanford site for 90Sr) can be a more financially favourable technology in comparison to P&T due to the minimal infrastructure required but offer fewer advantages for less sensitive radionuclides and depend upon the selection of an appropriate reactive media. Phytoremediation is a low-cost method for 3H removal and offers secondary environmental and social benefits but operates over long timescales and is not suitable for elements with low bioavailabilities (99Tc) or where bioaccumulation presents a risk to humans (129I).
Of the examined technologies, none are effective at removing all of 129I, 99Tc, 90Sr and 3H. This highlights the need for simultaneous growth in research areas looking at improving the efficiency of current techniques, enhancing the development of “treatment train” approaches (potentially including natural attenuation for shorter-lived DTMRs), and continued progression of emerging technologies to minimise scenarios where no remediation strategies are appropriate. Alternative techniques such as electrokinetic remediation and silica grouting are being developed but their application towards DTMRs (with the exception of 90Sr134,136) remains unreported, particularly at larger (field or field pilot) scale. The need for advancement in this area is clear but current progress is hindered due to the difficulty associated with measuring DTMRs in situ. Although rapid screening techniques for these radionuclides is a developing area (e.g. studies by Hou,137 Warwick et al.138 and Zaffora et al.139), a co-evolution with the advancement of remediation technologies would help to optimise both research fields. If this were to occur, site operators would be able to make better-informed decisions on remediation strategies using real-time, high-resolution plume data, leading to more intelligent groundwater clean-up. This synergy would greatly benefit the capability of remedial projects globally, consequently reducing the financial burden for nuclear sites and governments as more effective action can be taken to ensure that thorough and comprehensive DTMR removal occurs.
Conflicts of interest
There are no conflicts to declare.
Acknowledgements
We thank two anonymous reviewers for helpful comments which improved the overall quality of the information and arguments presented in this paper. We are grateful for comments and information from Dr James Graham from the National Nuclear Laboratory (UK) on remediation strategies at the Sellafield facility (UK), and to the TRANSCEND Consortium (EPSRC grant number EP/S01019X/1) for funding support. Shaun Hemming is also grateful to the INSPIRE Doctoral Training Partnership (NERC grant number NE/S007210/1) for PhD funding.
References
-
DOE, Hanford Site RCRA Groundwater Monitoring Report For 2022, U.S. Department of Energy, Richland, Washington, 2023 Search PubMed.
-
Sellafield Ltd., Groundwater Monitoring Annual Data Review 2016, Sellafield Ltd., 2017 Search PubMed.
- N. M. Hassan, Y. J. Kim, J. Jang, B. U. Chang and J. S. Chae, Comparative study of precise measurements of natural radionuclides and radiation dose using in-situ and laboratory γ-ray spectroscopy techniques, Sci. Rep., 2018, 8, 14115 CrossRef CAS PubMed.
-
IAEA, Determination and Use of Scaling Factors for Waste Characterization in Nuclear Power Plants, International Atomic Energy Agency, Vienna, 2009 Search PubMed.
- S. Zhang, C. Xu, D. Creeley, Y.-F. Ho, H.-P. Li, R. Grandbois, K. A. Schwehr, D. I. Kaplan, C. M. Yeager, D. Wellman and P. H. Santschi, Iodine-129 and Iodine-127 Speciation in Groundwater at the Hanford Site, U.S.: Iodate Incorporation into Calcite, Environ. Sci. Technol., 2013, 47, 9635–9642 CrossRef CAS PubMed.
-
DOE, Hanford Site Groundwater Monitoring Report for 2021, U.S. Department of Energy, Richland, Washington, 2022 Search PubMed.
-
DOE, Hanford Site Groundwater Monitoring Report for 2019, U.S. Department of Energy, Richland, Washington, 2020 Search PubMed.
-
Sellafield Ltd., Monitoring Our Environment - Discharges and Environmental Monitoring 2021, Sellafield Ltd., Cumbria, UK, 2022 Search PubMed.
- D. Hou, P. Guthrie and M. Rigby, Assessing the trend in sustainable remediation: A questionnaire survey of remediation professionals in various countries, J. Environ. Manage., 2016, 184, 18–26 CrossRef PubMed.
-
CL:AIRE, A Framework for Assessing the Sustainability of Soil and Groundwater Remediation, Contaminated Land: Applications in Real Environments, London, 2010 Search PubMed.
-
ASTM International, ASTM E2893 - 16e1: Standard Guide for Greener Cleanups, ASTM International, 2016 Search PubMed.
-
ISO, ISO 18504:2017: Soil quality - Sustainable remediation, International Standards Organisation, 2017 Search PubMed.
-
NDA, Nuclear Decommissioning Authority Strategy 2021, Nuclear Decommissioning Authority, Cumbria, UK, 2021 Search PubMed.
- F. Hossain, Natural and anthropogenic radionuclides in water and wastewater: Sources, treatments and recoveries, J. Environ. Radioact., 2020, 225, 106423 CrossRef CAS PubMed.
- D. Alby, C. Charnay, M. Heran, B. Prelot and J. Zajac, Recent developments in nanostructured inorganic materials for sorption of cesium and strontium: Synthesis and shaping, sorption capacity, mechanisms, and selectivity-A review, J. Hazard. Mater., 2018, 344, 511–530 CrossRef CAS PubMed.
- S. Sharma, B. Singh and V. K. Manchanda, Phytoremediation: role of terrestrial plants and aquatic macrophytes in the remediation of radionuclides and heavy metal contaminated soil and water, Environ. Sci. Pollut. Res., 2015, 22, 946–962 CrossRef CAS PubMed.
-
IAEA, Technologies for remediation of radioactively contaminated sites, International Atomic Energy Agency, Vienna, 1999 Search PubMed.
-
S. Thierfeldt and A. Deckert, Radionuclides Difficult to Measure in Waste Packages, Swedish Nuclear Power Inspectorate, Stockholm, 1995 Search PubMed.
-
IAEA, Nuclear Power Reactors in the World 2022 Edition, International Atomic Energy Agency, Vienna, 2022 Search PubMed.
- K. Shozugawa, M. Hori, T. E. Johnson, N. Takahata, Y. Sano, N. Kavasi, S. K. Sahoo and M. Matsuo, Landside tritium leakage over through years from Fukushima Dai-ichi nuclear plant and relationship between countermeasures and contaminated water, Sci. Rep., 2020, 10, 19925 CrossRef CAS PubMed.
-
IAEA, IAEA Nuclear Data Services, https://www-nds.iaea.org/, accessed 6 October 2023 Search PubMed.
- D. Banerjee, D. Kim, M. J. Schweiger, A. A. Kruger and P. K. Thallapally, Removal of TcO4− ions from solution: materials and future outlook, Chem. Soc. Rev., 2016, 45, 2724–2739 RSC.
- B. J. Riley, J. D. Vienna, D. M. Strachan, J. S. McCloy and J. L. Jerden, Materials and processes for the effective capture and immobilization of radioiodine: A review, J. Nucl. Mater., 2016, 470, 307–326 CrossRef CAS.
-
IAEA, Management of Waste Containing Tritium and Carbon-14, International Atomic Energy Agency, Vienna, 2004 Search PubMed.
- D. Bugai, J. Smith and M. A. Hoque, Solid-liquid distribution coefficients (Kd-s) of geological deposits at the Chernobyl Nuclear Power Plant site with respect to Sr, Cs and Pu radionuclides: A short review, Chemosphere, 2020, 242, 125175 CrossRef CAS PubMed.
-
S. Sheppard, G. Sohlenius, L. G. Omberg, M. Borgiel, S. Grolander and S. Nordén, Solid/liquid partition coefficients (Kd) and plant/soil concentration ratios (CR) for selected soils, tills and sediments at Forsmark, Swedish Nuclear Fuel and Waste Management Co., Stockholm, 2011 Search PubMed.
-
M. Denham, D. Kaplan and C. Yeager, Groundwater Radioiodine: Prevalence, Biogeochemistry, and Potential Remedial Approaches, Savannah River National Laboratory, South Carolina, 2009 Search PubMed.
- D. I. Kaplan, M. E. Denham, S. Zhang, C. Yeager, C. Xu, K. A. Schwehr, H. P. Li, Y. F. Ho, D. Wellman and P. H. Santschi, Radioiodine Biogeochemistry and Prevalence in Groundwater, Crit. Rev. Environ. Sci. Technol., 2014, 44, 2287–2335 CrossRef CAS PubMed.
- F. Keppler, H. Biester, A. Putschew, P. J. Silk, H. F. Schöler and G. Müller, Organoiodine formation during humification in peatlands, Environ. Chem. Lett., 2003, 1, 219–223 CrossRef.
- V. Moulin, P. Reiller, B. Amekraz and C. Moulin, Direct characterization of iodine covalently bound to fulvic acids by electrospray mass spectrometry, Rapid Commun. Mass Spectrom., 2001, 15, 2488–2496 CrossRef CAS PubMed.
- K. A. Schwehr, P. H. Santschi, D. I. Kaplan, C. M. Yeager and R. Brinkmeyer, Organo-Iodine Formation in Soils and Aquifer Sediments at Ambient Concentrations, Environ. Sci. Technol., 2009, 43, 7258–7264 CrossRef CAS PubMed.
-
K. J. Cantrell, R. J. Serne and G. V. Last, Hanford Contaminant Distribution Coefficient Database and Users Guide, Pacific Northwest National Laboratory, Richland, Washington, 2003 Search PubMed.
- D. I. Kaplan, K. A. Roberts, K. A. Schwehr, M. S. Lilley, R. Brinkmeyer, M. E. Denham, D. DiPrete, H.-P. Li, B. A. Powell, C. Xu, C. M. Yeager, S. Zhang and P. H. Santschi, Evaluation of a Radioiodine Plume Increasing in Concentration at the Savannah River Site, Environ. Sci. Technol., 2011, 45, 489–495 CrossRef CAS PubMed.
- C. Xu, D. I. Kaplan, S. Zhang, M. Athon, Y.-F. Ho, H.-P. Li, C. M. Yeager, K. A. Schwehr, R. Grandbois, D. Wellman and P. H. Santschi, Radioiodine sorption/desorption and speciation transformation by subsurface sediments from the Hanford Site, J. Environ. Radioact., 2015, 139, 43–55 CrossRef CAS PubMed.
- R. Naidu, N. S. Bolan, R. S. Kookana and K. G. Tiller, Ionic-strength and pH effects on the sorption of cadmium and the surface charge of soils, Eurasian J. Soil Sci., 1994, 45, 419–429 CrossRef CAS.
- J. L. Dai, M. Zhang, Q. H. Hu, Y. Z. Huang, R. Q. Wang and Y. G. Zhu, Adsorption and desorption of iodine by various Chinese soils: II. Iodide and iodate, Geoderma, 2009, 153, 130–135 CrossRef CAS.
- J.-L. Dai, M. Zhang and Y.-G. Zhu, Adsorption and desorption of iodine by various Chinese soils: I. Iodate, Environ. Int., 2004, 30, 525–530 CrossRef CAS PubMed.
- D. Fan, R. P. Anitori, B. M. Tebo, P. G. Tratnyek, J. S. Lezama Pacheco, R. K. Kukkadapu, L. Kovarik, M. H. Engelhard and M. E. Bowden, Oxidative Remobilization of Technetium Sequestered by Sulfide-Transformed Nano Zerovalent Iron, Environ. Sci. Technol., 2014, 48, 7409–7417 CrossRef CAS PubMed.
- R. E. Meyer, W. D. Arnold, F. I. Case and G. D. O'Kelley, Solubilities of Tc(IV) Oxides, Radiochim. Acta, 1991, 55, 11–18 CrossRef CAS.
-
R. J. Serne, Kd Values for Agricultural and Surface Soils for Use in Hanford Site Farm, Residential, and River Shoreline Scenarios, U.S Department of Energy, 2007 Search PubMed.
-
S. Sheppard, J. Long, B. Sanipelli and G. Sohlenius, Solid/liquid partition coefficients (Kd) for selected soils and sediments at Forsmark and Laxemar-Simpevarp, Swedish Nuclear Fuel and Waste Management Co., Stockholm, 2009 Search PubMed.
- A. Cleary, J. R. Lloyd, L. Newsome, S. Shaw, C. Boothman, G. Boshoff, N. Atherton and K. Morris, Bioremediation of strontium and technetium contaminated groundwater using glycerol phosphate, Chem. Geol., 2019, 509, 213–222 CrossRef CAS.
- D. K. Gupta, W. Schulz, G. Steinhauser and C. Walther, Radiostrontium transport in plants and phytoremediation, Environ. Sci. Pollut. Res., 2018, 25, 29996–30008 CrossRef CAS PubMed.
-
R. J. Serne, V. L. LeGore, K. J. Cantrell, C. W. Lindenmeiser, J. A. Campbell, J. E. Amonette and J. L. Conca, Solid-waste leach characteristics and contaminant-sediment interactions. Volume 1, Batch leach and adsorption tests and sediment characterization, Pacific Northwest Lab., Richland, Washington, 1993 Search PubMed.
- J. Guillén, A. Baeza, J. A. Corbacho and J. G. Muñoz-Muñoz, Migration of 137Cs, 90Sr, and 239+240Pu in Mediterranean forests: influence of bioavailability and association with organic acids in soil, J. Environ. Radioact., 2015, 144, 96–102 CrossRef PubMed.
- S. H. Wallace, S. Shaw, K. Morris, J. S. Small, A. J. Fuller and I. T. Burke, Effect of groundwater pH and ionic strength on strontium sorption in aquifer sediments: Implications for 90Sr mobility at contaminated nuclear sites, Appl. Geochem., 2012, 27, 1482–1491 CrossRef CAS.
- M.-S. Yim and F. Caron, Life cycle and management of carbon-14 from nuclear power generation, Prog. Nucl. Energy, 2006, 48, 2–36 CrossRef CAS.
-
M. C. Negri, R. Hinchman and J. Wozniak, Capturing a ‘Mixed’ Contaminant Plume: Tritium Phytoevaporation at Argonne National Laboratory's Area 319, Argonne National Laboratory, Argonne, Illinois, 2000 Search PubMed.
-
D. H. Thibault, M. I. Sheppard and P. A. Smith, A Critical Compilation and Review of Default Soil Solid/Liquid Partition Coefficients, Kd, for Use in Environmental Assessments, Whiteshell Nuclear Research Establishment, Pinawa, Manitoba, Canada, 1990 Search PubMed.
- I. W. Croudace, P. E. Warwick and J. E. Morris, Evidence for the Preservation of Technogenic Tritiated Organic Compounds in an Estuarine Sedimentary Environment, Environ. Sci. Technol., 2012, 46, 5704–5712 CrossRef CAS PubMed.
- S. Masiuk, M. Chepurny, V. Buderatska, A. Kukush, S. Shklyar, O. Ivanova, Z. Boiko, N. Zhadan, G. Fedosenko, A. Bilonyk, T. Lev, M. Talerko, S. Kutsen, V. Minenko, K. Viarenich and V. Drozdovitch, Thyroid doses in Ukraine due to 131I intake after the Chornobyl accident. Report I: revision of direct thyroid measurements, Radiat. Environ. Biophys., 2021, 60, 267–288 CrossRef CAS PubMed.
-
IAEA, Technetium-99m Radiopharmaceuticals: Status and Trends, International Atomic Energy Agency, Vienna, 2009 Search PubMed.
- S. G. Dahl, P. Allain, P. J. Marie, Y. Mauras, G. Boivin, P. Ammann, Y. Tsouderos, P. D. Delmas and C. Christiansen, Incorporation and distribution of strontium in bone, Bone, 2001, 28, 446–453 CrossRef CAS PubMed.
- H. Matsumoto, Y. Shimada, A. J. Nakamura, N. Usami, M. Ojima, S. Kakinuma, M. Shimada, M. Sunaoshi, R. Hirayama and H. Tauchi, Health effects triggered by tritium: how do we get public understanding based on scientifically supported evidence?, J. Radiat. Res., 2021, 62, 557–563 CrossRef CAS PubMed.
-
J. L. Downs, B. A. Napier, M. J. Truex and A.
L. Bunn, Evaluation of Dose- and Risk-Based Groundwater Cleanup Levels for Low Energy Beta Radioisotopes, Pacific Northwest National Laboratory, Richland, Washington, 2020 Search PubMed.
- C. I. Pearce, R. C. Moore, J. W. Morad, R. M. Asmussen, S. Chatterjee, A. R. Lawter, T. G. Levitskaia, J. J. Neeway, N. P. Qafoku, M. J. Rigali, S. A. Saslow, J. E. Szecsody, P. K. Thallapally, G. Wang and V. L. Freedman, Technetium immobilization by materials through sorption and redox-driven processes: A literature review, Sci. Total Environ., 2020, 716, 132849 CrossRef CAS PubMed.
- S. Dushenkov, Trends in phytoremediation of radionuclides, Plant Soil, 2003, 249, 167–175 CrossRef CAS.
-
R. D. Mackley, D. M. Anderson, J. N. Thonle and C. E. Strickland, Technical and Economic Assessment of Solar Photovoltaic for Groundwater Extraction on the Hanford Site, Pacific Northwest National Laboratory, Richland, Washington, 2015 Search PubMed.
- O. Al-Hashimi, K. Hashim, E. Loffill, T. Marolt Čebašek, I. Nakouti, A. A. H. Faisal and N. Al-Ansari, A Comprehensive Review for Groundwater Contamination and Remediation: Occurrence, Migration and Adsorption Modelling, Molecules, 2021, 26, 5913 CrossRef CAS PubMed.
-
EPA, Pump and Treat and In Situ Chemical Treatment of Contaminated Groundwater at the Odessa Chromium II South Plume Superfund Site Odessa, Ector County, Texas, U. S. Environmental Protection Agency, 2005 Search PubMed.
-
R. V. Fox and B. Mincher, Supercritical Fluid Extraction of Plutonium and Americium from Soil, Idaho National Engineering and Environmental Laboratory, 2002 Search PubMed.
-
CRC CARE, Technology guide: Pump and treat, Cooperative Research Centre for Contamination Assessment and Remediation of the Environment, 2018 Search PubMed.
- W. C. Jolin and M. Kaminski, Sorbent Materials for Rapid Remediation of Wash Water during Radiological Event Relief, Chemosphere, 2016, 162, 165–171 CrossRef CAS PubMed.
- C. I. Pearce, E. A. Cordova, W. L. Garcia, S. A. Saslow, K. J. Cantrell, J. W. Morad, O. Qafoku, J. Matyáš, A. E. Plymale, S. Chatterjee, J. Kang, F. C. Colon, T. G. Levitskaia, M. J. Rigali, J. E. Szecsody, S. M. Heald, M. Balasubramanian, S. Wang, D. T. Sun, W. L. Queen, R. Bontchev, R. C. Moore and V. L. Freedman, Evaluation of materials for iodine and technetium immobilization through sorption and redox-driven processes, Sci. Total Environ., 2020, 716, 136167 CrossRef CAS PubMed.
- D. Banerjee, W. Xu, Z. Nie, L. E. V. Johnson, C. Coghlan, M. L. Sushko, D. Kim, M. J. Schweiger, A. A. Kruger, C. J. Doonan and P. K. Thallapally, Zirconium-Based Metal-Organic Framework for Removal of Perrhenate from Water, Inorg. Chem., 2016, 55, 8241–8243 CrossRef CAS PubMed.
-
ONR, Remediation Techniques for Radioactive Contaminated Land on Nuclear Licensed Sites, Office for Nuclear Regulation, 2020 Search PubMed.
-
M. J. Truex, V. L. Freedman, C. I. Pearce and J. E. Szecsody, Assessment of Technologies for I-129 Remediation in the 200UP-1 Operable Unit, Pacific Northwest National Laboratory, Richland, Washington, 2019 Search PubMed.
-
J. E. Szecsody, C. Pearce, K. J. Cantrell, N. P. Qafoku, G. Wang, E. C. Gillispie, A. R. Lawter, B. N. Gartman and C. M. Brown, Evaluation of Remediation Technologies for Iodine-129: FY18 Bench Scale Results, Pacific Northwest National Laboratory, Richland, Washington, 2018 Search PubMed.
-
USEPA, Washington State Department of Ecology and USDOE, Record of Decision for Interim Remedial Action Hanford 200 Area Superfund Site 200-UP-1 Operable Unit., U. S. Environmental Protection Agency, Olympia, Washington, 2012 Search PubMed.
- J. J. Neeway, D. I. Kaplan, C. E. Bagwell, M. L. Rockhold, J. E. Szecsody, M. J. Truex and N. P. Qafoku, A review of the behavior of radioiodine in the subsurface at two DOE sites, Sci. Total Environ., 2019, 691, 466–475 CrossRef CAS PubMed.
-
M. L. Rockhold, S. R. Waichler, J. L. Downs, X. He, J. D. Tagestad, Y. Fang, V. L. Freedman, M. J. Truex and C. M. R. Yonkofski, Information on Technical Impracticability for Remediation of Iodine-129 Contamination, Pacific Northwest National Laboratory, Richland, Washington, 2019 Search PubMed.
-
M. L. Rockhold, J. L. Downs, S. R. Waichler, C. M. R. Yonkofski, V. L. Freedman and M. J. Truex, Draft Technical Impracticability Evaluation for Iodine-129 in Groundwater at Hanford: 200-UP-1 Operable Unit, Pacific Northwest National Laboratory, Richland, Washington, 2018 Search PubMed.
- S. A. Saslow, T. G. Levitskaia, E. A. Cordova, N. M. Avalos, D. Boglaienko, Y. Fang, X. Song, A. Lawter, H. Emerson, J. Szecsody, C. D. Johnson, C. I. Pearce, V. L. Freedman and R. D. Mackley, Part I: Predicting performance of Purolite A532E resins for remediation of comingled contaminants in groundwater, J. Environ. Chem. Eng., 2023, 11, 109618 CrossRef CAS.
- S. A. Saslow, T. G. Levitskaia, E. A. Cordova, N. M. Avalos, D. Boglaienko, Y. Fang, X. Song, A. Lawter, H. Emerson, J. Szecsody, C. D. Johnson, C. I. Pearce, V. L. Freedman and R. D. Mackley, Part II: Predicting performance of DOWEX 21K resin for remediation of comingled contaminants in groundwater, J. Environ. Chem. Eng., 2023, 11, 109620 CrossRef CAS.
-
E. L. Campbell, T. G. Levitskaia, M. S. Fujimoto, V. E. Holfeltz, S. D. Chatterjee and G. B. Hall, Analysis of Uranium Ion Exchange Resin from the 200 West Pump-and-Treat Facility, Pacific Northwest National Laboratory, Richland, Washington, 2018 Search PubMed.
-
DOE, Calendar Year 2020 Annual Summary Report For Pump And Treat Operations In the Hanford Central Plateau Operable Units, U.S. Department of Energy, Richland, Washington, 2022 Search PubMed.
-
BNL, 2005 Site Environmental Report Volume II Groundwater Status Report, Brookhaven National Laboratory, Upton, New York, 2006 Search PubMed.
-
T. Sullivan, M. Hauptmann and W. Gunther, Lessons Learned in Detecting, Monitoring, Modeling and Remediating Radioactive Ground-Water Contamination, United States Nuclear Regulatory Commission, Upton, New York, 2011 Search PubMed.
-
BNL, 2021 Site Environmental Report Volume II Groundwater Status Report (Appendix C), Brookhaven National Laboratory, Upton, New York, 2022 Search PubMed.
-
G. Blount, J. Thibault, M. Millings and P. Prater, 25 Years Of Environmental Remediation In The General Separations Area Of The Savannah River Site: Lessons Learned About What Worked And What Did Not Work In Soil And Groundwater Cleanup – 15270, Waste Management Symposia, Phoenix, Arizona, 2015 Search PubMed.
- A. A. H. Faisal, A. H. Sulaymon and Q. M. Khaliefa, A review of permeable reactive barrier as passive sustainable technology for groundwater remediation, Int. J. Environ. Sci. Technol., 2018, 15, 1123–1138 CrossRef CAS.
-
ITRC, Permeable Reactive Barrier: Technology Update, International Technology and Regulatory Council, Washington D.C., 2011 Search PubMed.
- F. Obiri-Nyarko, S. J. Grajales-Mesa and G. Malina, An overview of permeable reactive barriers for in situ sustainable groundwater remediation, Chemosphere, 2014, 111, 243–259 CrossRef CAS PubMed.
- S. Smoleń, I. Ledwożyw-Smoleń and W. Sady, The role of exogenous humic and fulvic acids in iodine biofortification in spinach (Spinacia oleracea L.), Plant Soil, 2016, 402, 129–143 CrossRef.
- S. M. Steinberg, G. T. Schmett, G. Kimble, D. W. Emerson, M. F. Turner and M. Rudin, Immobilization of fission iodine by reaction with insoluble natural organic matter, J. Radioanal. Nucl. Chem., 2008, 277, 175–183 CrossRef CAS.
-
N. P. Qafoku, C. Bagwell, A. R. Lawter, M. J. Truex, J. E. Szecsody, O. Qafoku, L. Kovarik, L. Zhong, A. Mitroshkov and V. Freedman, Conceptual Model of Subsurface Processes for Iodine at the Hanford Site, Pacific Northwest National Laboratory, Richland, Washington, 2018 Search PubMed.
-
P. H. Santschi and D. I. Kaplan, Using Radioiodine Speciation to Address Environmental Remediation and Waste Stream Sequestration Problems at the Fukushima Daiichi Nuclear Power Plant and a DOE Site, Texas A&M University, Galveston, Texas, 2020 Search PubMed.
- R. C. Moore, C. I. Pearce, J. W. Morad, S. Chatterjee, T. G. Levitskaia, R. M. Asmussen, A. R. Lawter, J. J. Neeway, N. P. Qafoku, M. J. Rigali, S. A. Saslow, J. E. Szecsody, P. K. Thallapally, G. Wang and V. L. Freedman, Iodine immobilization by materials through sorption and redox-driven processes: A literature review, Sci. Total Environ., 2020, 716, 132820 CrossRef CAS PubMed.
- C. Xu, E. J. Miller, S. Zhang, H.-P. Li, Y.-F. Ho, K. A. Schwehr, D. I. Kaplan, S. Otosaka, K. A. Roberts, R. Brinkmeyer, C. M. Yeager and P. H. Santschi, Sequestration and Remobilization of Radioiodine (129I) by Soil Organic Matter and Possible Consequences of the Remedial Action at Savannah River Site, Environ. Sci. Technol., 2011, 45, 9975–9983 CrossRef CAS PubMed.
- L. Newsome, K. Morris, A. Cleary, N. K. Masters-Waage, C. Boothman, N. Joshi, N. Atherton and J. R. Lloyd, The impact of iron nanoparticles on technetium-contaminated groundwater and sediment microbial communities, J. Hazard. Mater., 2019, 364, 134–142 CrossRef CAS PubMed.
- D. Boglaienko, O. Qafoku, R. K. Kukkadapu, L. Kovarik, Y. P. Katsenovich, D. E. Cherkasov, H. P. Emerson and T. G. Levitskaia, Elemental iron: reduction of pertechnetate in the presence of silica and periodicity of precipitated nano-structures, Environ. Sci.: Nano, 2020, 8, 97–109 RSC.
- L. Liang, B. Gu and X. Yin, Removal of technetium-99 from contaminated groundwater with sorbents and reductive materials, Sep. Technol., 1996, 6, 111–122 CrossRef CAS.
-
K. Bronstein, Permeable Reactive Barriers for Inorganic and Radionuclide Contamination, National Network of Environmental Studies, Washington, DC, 2005 Search PubMed.
-
Bechtel Jacobs Company LLC, Bechtel Jacobs Company LLC Sampling and Analysis Plan for the Water Resources Restoration Program for Fiscal Year 2009 Oak Ridge Reservation, Oak Ridge, Tennessee, Bechtel Jacobs Company LLC, Oak Ridge, Tennessee, 2008 Search PubMed.
-
DOE, Hanford Site Groundwater Monitoring Report for 2020, U.S. Department of Energy, Richland, Washington, 2021 Search PubMed.
-
V. M. Vermeul, B. G. Fritz, J. S. Fruchter, J. E. Szecsody and M. D. Williams, 100-NR-2 Apatite Treatability Test: High-Concentration Calcium-Citrate-Phosphate Solution Injection for In Situ Strontium-90 Immobilization Final Report, Pacific Northwest National Laboratory, Richland, Washington, 2010 Search PubMed.
- V. R. Vermeul, J. E. Szecsody, B. G. Fritz, M. D. Williams, R. C. Moore and J. S. Fruchter, An Injectable Apatite Permeable Reactive Barrier for In Situ90Sr Immobilization, Groundwater Monit. Rem., 2014, 34, 28–41 CrossRef CAS.
-
USEPA, US Department of Energy 100-NR-1 and 100-NR-2 Operable Units Hanford Site 100 Area Benton County Washington Amended Record of Decision Decision Summary and Responsiveness Summary September 2010, United States Environmental Protection Agency, Seattle, Washington, 2010 Search PubMed.
- R. M. H. Verbeeck, M. Hauben, H. P. Thun and F. Verbeek, Solubility and Solution Behaviour of Strontiumhydroxyapatite, Z. Phys. Chem., 1977, 108, 203–215 CrossRef CAS.
- C. Macci, E. Peruzzi, S. Doni and G. Masciandaro, Monitoring of a long term phytoremediation process of a soil contaminated by heavy metals and hydrocarbons in Tuscany, Environ. Sci. Pollut. Res., 2020, 27, 424–437 CrossRef CAS PubMed.
- D. E. Salt, M. Blaylock, N. P. B. A. Kumar, V. Dushenkov, B. D. Ensley, I. Chet and I. Raskin, Phytoremediation: A Novel Strategy for the Removal of Toxic Metals from the Environment Using Plants, Bio/Technology, 1995, 13, 468–474 CAS.
- H. Ali, E. Khan and M. A. Sajad, Phytoremediation of heavy metals—Concepts and applications, Chemosphere, 2013, 91, 869–881 CrossRef CAS PubMed.
- I. Alkorta, J. Hernández-Allica, J. M. Becerril, I. Amezaga, I. Albizu and C. Garbisu, Recent Findings on the Phytoremediation of Soils Contaminated with Environmentally Toxic Heavy Metals and Metalloids Such as Zinc, Cadmium, Lead, and Arsenic, Rev. Environ. Sci. Bio/Technol., 2004, 3, 71–90 CrossRef CAS.
-
J. T. Brown, G. Matthern, A. Glenn, J. Kauffman, S. Rock, M. Kuperberg, C. Ainsworth and J. Waugh, Proceedings from the Workshop on Phytoremediation of Inorganic Contaminants, Idaho National Engineering and Environmental Laboratory, Chicago, Illinois, 2000 Search PubMed.
- R. Bennett and N. Willey, Soil availability, plant uptake and soil to plant transfer of 99Tc—A review, J. Environ. Radioact., 2003, 65, 215–231 CrossRef CAS PubMed.
- M. Tamaoki, T. Yabe, J. Furukawa, M. Watanabe, K. Ikeda, I. Yasutani and T. Nishizawa, Comparison of Potentials of Higher Plants for Phytoremediation of Radioactive Cesium from Contaminated Soil, Environ. Control Biol., 2016, 54, 65–69 CrossRef CAS.
-
M. V. Dutton, Accumulation of Caesium and Strontium by Cirsium vulgare and Rumex obtusifolius on the Southern Soil Disposal Tip at Sellafield: Part I, Westlake Scientific Consultin, Cumbria, UK, 2000 Search PubMed.
- J. M. Purkis, R. P. Bardos, J. Graham and A. B. Cundy, Developing field-scale, gentle remediation options for nuclear sites contaminated with 137Cs and 90Sr: The role of Nature-Based Solutions, J. Environ. Manage., 2022, 308, 114620 CrossRef CAS PubMed.
-
C. Lewis and R. Van Pelt, Natural Remediation at Savannah River Site, Waste Management Symposia, Tucson, Arizona, 2002 Search PubMed.
-
SRNS, Savannah River Site Groundwater Management Strategy and Implementation Plan (U), Savannah River Nuclear Solutions, LLC, Aiken, South Carolina, 2006 (updated 2020) Search PubMed.
-
SRNS, Savannah River Site Environmental Report 2021, Savannah River Nuclear Solutions, LLC, Aiken, South Carolina, 2022 Search PubMed.
-
DOE, Trees Help Clean Groundwater in SRS Project, 2016, https://www.energy.gov/management/articles/trees-help-clean-groundwater-srs-project, accessed 6 October 2023 Search PubMed.
- D. R. Hitchcock, C. D. Barton, K. T. Rebel, J. Singer, J. C. Seaman, J. D. Strawbridge, S. J. Riha and J. I. Blake, A containment and disposition strategy for tritium-contaminated groundwater at the Savannah River Site, South Carolina, United States, Environ. Geosci., 2005, 12, 17–28 CrossRef.
- J. D. Istok, J. M. Senko, L. R. Krumholz, D. Watson, M. A. Bogle, A. Peacock, Y.-J. Chang and D. C. White,
In Situ Bioreduction of Technetium and Uranium in a Nitrate-Contaminated Aquifer, Environ. Sci. Technol., 2004, 38, 468–475 CrossRef CAS PubMed.
- M. Radziemska, M. Z. Gusiatin, A. Cydzik-Kwiatkowska, G. Majewski, A. Blazejczyk and M. Brtnicky, New approach strategy for heavy metals immobilization and microbiome structure long-term industrially contaminated soils, Chemosphere, 2022, 308, 136332 CrossRef CAS PubMed.
- W. Qiao, Y. Zhang, H. Xia, Y. Luo, S. Liu, S. Wang and W. Wang, Bioimmobilization of lead by Bacillus subtilis X3 biomass isolated from lead mine soil under promotion of multiple adsorption mechanisms, R. Soc. Open Sci., 2019, 6, 181701 CrossRef CAS PubMed.
- D. R. Lovley, E. J. P. Phillips, Y. A. Gorby and E. R. Landa, Microbial reduction of uranium, Nature, 1991, 350, 413–416 CrossRef CAS.
- I. T. Burke, C. Boothman, J. R. Lloyd, R. J. G. Mortimer, F. R. Livens and K. Morris, Effects of Progressive Anoxia on the Solubility of Technetium in Sediments, Environ. Sci. Technol., 2005, 39, 4109–4116 CrossRef CAS PubMed.
- J. Wikström, S. Bonaglia, R. Rämö, G. Renman, J. Walve, J. Hedberg and J. S. Gunnarsson, Sediment Remediation with New Composite Sorbent Amendments to Sequester Phosphorus, Organic Contaminants, and Metals, Environ. Sci. Technol., 2021, 55, 11937–11947 CrossRef PubMed.
- C. Xiong, D. Wang, N. F. Tam, Y. Dai, X. Zhang, X. Tang and Y. Yang, Enhancement of active thin-layer capping with natural zeolite to simultaneously inhibit nutrient and heavy metal release from sediments, Ecol. Eng., 2018, 119, 64–72 CrossRef.
-
M. E. Denham, H. Gonzalez-Raymat and C. A. Eddy-Dilek, Applied Science Investigations to Facilitate Closure of the F-Area and H-Area Seepage Basins, Savannah River National Laboratory, Aiken, South Carolina, 2020 Search PubMed.
-
K. Boerstler, J. Thibault, D. Stefanko and P. Prater, The Use of Silver Chloride Injection in Remediation of Iodine-129 by In Situ Capture as Silver Iodide at the F-Area Seepage Basin - 20225, Waste Management Symposia, Phoenix, Arizona, 2020 Search PubMed.
-
SRNS, Annual Corrective Action Report for the F-Area Hazardous Waste Management Facility, the H-Area Hazardous Waste Management Facility, and the Mixed Waste Management Facility (U), Savannah River Nuclear Solutions, LLC, Aiken, South Carolina, 2021 Search PubMed.
-
D. J. Geniesse and G. E. Stegen, 2009 Evaluation of Tritium Removal and Mitigation Technologies for Wastewater Treatment, U.S. Department of Energy, Richland, Washington, 2009 Search PubMed.
- C. J. Sienkiewicz and J. E. Lentz, Recovery of Tritium from Water, Fusion Technol., 1988, 14, 444–449 CrossRef CAS.
- T. Yamanishi, H. Kakiuchi, H. Tauchi, T. Yamamoto and I. Yamamoto, Discussions on Tritiated Water Treatment for Fukushima Daiichi Nuclear Power Station, Fusion Sci. Technol., 2020, 76, 430–438 CrossRef.
- S. D. Bondarenko, I. A. Alekseev, O. A. Fedorchenko, T. V. Vasyanina, K. A. Konoplev, E. A. Arkhipov and V. V. Uborsky, Improvement of PNPI Experimental Industrial Plant Based on CECE Process for Heavy Water Detritiation, Fusion Sci. Technol., 2008, 54, 446–449 CrossRef CAS.
-
Government of Japan, Events and highlights on the progress related to recovery operations at TEPCO's Fukushima Daiichi Nuclear Power Station, Government of Japan, 2022 Search PubMed.
-
DOE, Evaluation of Tritium Removal and Mitigation Technologies for Wastewater Treatment, U.S. Department of Energy, Richland, Washington, 2022 Search PubMed.
-
EPA, Cost Analyses for Selected Groundwater Cleanup Projects: Pump and Treat Systems and Permeable Reactive Barriers, U. S. Environmental Protection Agency, 2001 Search PubMed.
- A. B. Cundy, L. LaFreniere, R. P. Bardos, E. Yan, R. Sedivy and C. Roe, Integrated phytomanagement of a carbon tetrachloride-contaminated site in Murdock, Nebraska (USA), J. Cleaner Prod., 2021, 290, 125190 CrossRef CAS.
-
M. C. Negri, G. Gopalakrishnan and C. Hamilton, 317/319 Phytoremediation Site Monitoring Report – 2003 Growing Season, Argonne National Laboratory, Argonne, Illinois, 2004 Search PubMed.
-
S. Withana, P. Ten Brink, A. Illes, S. Nanni and E. Watkins, Environmental Tax Reform in Europe: Opportunities for the future, Institute for European Environmental Policy, Brussels, 2014 Search PubMed.
- J. M. Purkis, P. E. Warwick, J. Graham, S. D. Hemming and A. B. Cundy, Towards the application of electrokinetic remediation for nuclear site decommissioning, J. Hazard. Mater., 2021, 413, 125274 CrossRef CAS PubMed.
- M. Pedrotti, C. Wong, G. El Mountassir, J. C. Renshaw and R. J. Lunn, Desiccation behaviour of colloidal silica grouted sand: A new material for the creation of near surface hydraulic barriers, Eng. Geol., 2020, 270, 105579 CrossRef.
- P. Bots, J. C. Renshaw, T. E. Payne, M. J. Comarmond, A. E. P. Schellenger, M. Pedrotti, E. Calì and R. J. Lunn, Geochemical evidence for the application of nanoparticulate colloidal silica gel for in situ containment of legacy nuclear wastes, Environ. Sci.: Nano, 2020, 7, 1481–1495 RSC.
- X. Hou, Radiochemical analysis of radionuclides difficult to measure for waste characterization in decommissioning of nuclear facilities, J. Radioanal. Nucl. Chem., 2007, 273, 43–48 CrossRef CAS.
- P. E. Warwick, B. C. Russell, I. W. Croudace and Ž. Zacharauskas, Evaluation of inductively coupled plasma tandem mass spectrometry for radionuclide assay in nuclear waste characterisation, J. Anal. At. Spectrom., 2019, 34, 1810–1821 RSC.
- B. Zaffora, M. Magistris, J.-P. Chevalier, C. Luccioni, G. Saporta and L. Ulrici, A new approach to characterize very-low-level radioactive waste produced at hadron accelerators, Appl. Radiat. Isot., 2017, 122, 141–147 CrossRef CAS PubMed.
|
This journal is © The Royal Society of Chemistry 2023 |