DOI:
10.1039/D2QI01442D
(Research Article)
Inorg. Chem. Front., 2022,
9, 5688-5696
Decisive role of non-rare earth metals in high-regioselectivity addition of μ3-carbido clusterfullerene†
Received
5th July 2022
, Accepted 7th September 2022
First published on 14th September 2022
Abstract
Endohedral clusterfullerenes featuring encapsulation of metal clusters which transfer electrons to the outer fullerene cages show intriguing chemical properties different from empty fullerenes. Despite the extensive studies on the chemical properties of empty fullerenes, especially C60, chemical functionalization of endohedral clusterfullerenes has been less explored, and previous reports are primarily limited to the well-known metal nitride and carbide clusterfullerenes. Herein, we report the first chemical functionalization of an emerging endohedral clusterfullerene μ3-carbido clusterfullerene (abbreviated as μ3-CCF) bearing central μ3-C and Ti(IV) atoms forming a Ti
C double bond. A μ3-CCF Dy2TiC@Ih-C80 is synthesized, and its molecular structure is unambiguously determined by single-crystal X-ray diffraction for the first time. A photochemical cycloaddition reaction of Dy2TiC@Ih-C80 with 2-adamantane-2,3-[3H]-diazirine (abbreviated as AdN2) is then carried out and only one monoadduct Dy2TiC@Ih-C80-Ad is obtained, indicating its high regioselectivity. According to the X-ray single-crystal structure of Dy2TiC@Ih-C80-Ad, the Ad moiety selectively attacks the [6,6]-bond (conjunction of two fused hexagons), which is adjacent to the Ti4+ ion instead of the two Dy3+ ions, affording a [6,6]-open addition pattern. Theoretical calculations unveil that the Ti(IV) ion plays a decisive role in high regioselectivity, and the formation of [6,6]-open Dy2TiC@Ih-C80-Ad is thermodynamically preferred. Contrarily, a similar reaction of a Ti(III)-containing nitride clusterfullerene Y2TiN@C80 with AdN2 is predicted to generate a different type of adduct with the addition sites adjacent to the Y3+ ion instead of the Ti3+ ion. This reveals the peculiarity of the chemical property of μ3-CCF resulting from the existence of the non-rare earth metal Ti with a high oxidation state.
Introduction
Chemical functionalization of fullerenes offers an opportunity to expand the properties and functionalities of the pristine fullerenes and is essential for applications of fullerene materials in versatile fields such as organic photovoltaic, biomedicine, and catalysis.1–3 During the past three decades, extensive studies on the chemical properties of empty fullerenes, especially C60, have been reported.4,5 Upon encapsulating metal clusters into fullerene cages, endohedral clusterfullerenes form and exhibit intriguing chemical properties different from empty fullerenes owing to electron transfer from the encapsulated metal cluster to the outer fullerene cage.6 Among the known clusterfullerenes, metal nitride and carbide clusterfullerenes were discovered first, and thus their chemical properties have been widely studied.7–9 Up to now, several different types of chemical reactions, including [2 + 2]-cycloadditions,10,11 1,3-dipolar cycloaddition reactions,12–14 disilylations,15 Bingel-Hirsch reaction,16,17 azide addition reactions,18 Lewis acid–base addition reactions,19–21 carbene addition,22–25 and radical reactions,26,27 have been applied in functionalizing metal nitride and carbide clusterfullerenes. However, chemical functionalization of other types of endohedral clusterfullerenes has been less explored due mainly to their relatively low yield compared to the metal nitride clusterfullerenes (NCFs) such as Sc3N@C80.
As an emerging endohedral clusterfullerene, the μ3-carbido cluster-fullerene (abbreviated as μ3-CCF) discovered in 2014 appears quite special since a non-rare earth (RE) metal such as titanium (Ti) is needed, which bonds with a central μ3-C atom via a Ti
C double bond along with two RE metals.28 So far, a few Ti-based μ3-CCFs have been isolated, including TiM2C@C80 (M = Sc, Y, Nd, Gd, Tb, Dy, Er, and Lu)29–33 and TiSc2C@C78,29 featuring the electronic configurations of [Ti4+(M3+)2C4−]6+@C2n6− involving a Ti4+ cation. Noteworthily, this is distinctly different from the conventional metal nitride clusterfullerenes (NCFs) M3N@C2n in which the encapsulated RE and non-RE metals take formal oxidation states of 3+, affording electronic configurations of [(M3+)3N3−]6+@C2n6−.34–36 Thus, an open question arises: given that μ3-CCFs have similar trimetallic cluster compositions and the same charge state of the outer fullerene cage (C2n6−) with NCFs, whether are their chemical properties similar to those of NCFs? However, to the best of our knowledge, the chemical functionalization of μ3-CCFs has never been reported yet, and hence their chemical properties, specifically, the impact of the encapsulated Ti4+ cation, remain unknown.
Herein, we report the first chemical functionalization of μ3-CCF by a cycloaddition reaction of Dy2TiC@Ih-C80 with 2-adamantane-2,3-[3H]-diazirine (abbreviated as AdN2). Dy2TiC@Ih-C80 is synthesized and its molecular structure is unambiguously determined by single-crystal X-ray diffraction for the first time. After a photochemical reaction of Dy2TiC@Ih-C80 with AdN2, only one monoadduct Dy2TiC@Ih-C80-Ad is obtained, indicating its high regioselectivity. On the basis of the X-ray single-crystal structure of Dy2TiC@Ih-C80-Ad, a [6,6]-open addition pattern is identified. Theoretical calculations are carried out to unveil the role of the Ti4+ ion within Dy2TiC@Ih-C80 μ3-CCF in its high regioselectivity.
Results and discussion
Synthesis, isolation and X-ray crystallographic structure of Dy2TiC@Ih-C80
The synthesis procedure of Dy2TiC@C80 by a modified Krätschmer–Huffman DC-arc discharge method is similar to that of Tb2TiC@C80 we reported previously.32 Isolation of Dy2TiC@Ih-C80 was fulfilled by a three-step high performance liquid chromatography (HPLC) procedure, and its high purity was confirmed by matrix-assisted laser desorption ionization time-of-flight (MALDI-TOF) mass spectroscopy and HPLC (see ESI Fig. S1†).
Although Dy2TiC@C80 was reported by Popov et al. before,33 its molecule structure has not been determined unambiguously yet. In this work, we succeeded in determining the molecular structure of Dy2TiC@C80 unambiguously by single-crystal X-ray diffraction for the first time on the basis of growing high-quality cocrystals with decapyrrylcorannulene (DPC) as the host. The relative orientation of Dy2TiC@Ih-C80 and two DPC molecules within a 2DPC·{Dy2TiC@Ih-C80} cocrystal is shown in Fig. 1a, which includes only one orientation of the fullerene cage together with the major site of the Dy2TiC cluster for clarity (see ESI Table S1† for detailed crystallographic data). Similar to other reported clusterfullerene-DPC cocrystals,37–39 the asymmetric crystal unit cell consists of one pair of fully ordered DPC molecules and one Dy2TiC@Ih-C80 molecule. Meanwhile, two DPC molecules within 2DPC·{Dy2TiC@Ih-C80} exhibit a V-shape geometry with a dihedral angle of 65.1°, and the nearest DPC-C80 cage center distances are 7.08 Å/6.50 Å, which is evidently different from that of 2DPC·{Sc3N@Ih-C80} with a dihedral angle of 1.4° and a DPC-C80 cage center distance of 6.77/6.77 Å,39 predicting the distinct electronic configuration of Dy2TiC@Ih-C80, although they share the same Ih-symmetry C80 cage.
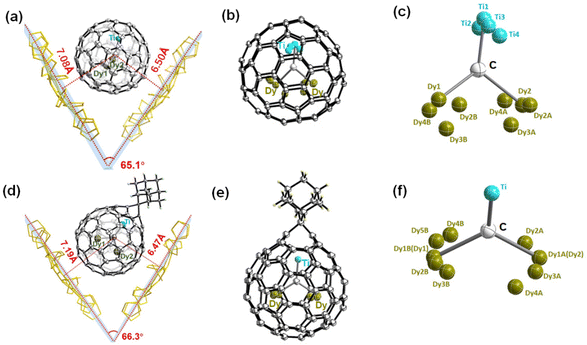 |
| Fig. 1 Crystal structures of (a) 2DPC·{Dy2TiC@Ih-C80} and (d) 2DPC·{Dy2TiC@Ih-C80-Ad}. Geometric configurations of (b) Dy2TiC@Ih-C80 and (e) Dy2TiC@Ih-C80-Ad along with the metal positional disorder of the Dy2TiC cluster within (c) Dy2TiC@Ih-C80 and (f) Dy2TiC@Ih-C80-Ad. | |
The ordered Ih-C80 cage and the major Dy/Ti sites with the largest occupancies (0.82, 0.91, and 0.80 for Dy1, Dy2, and Ti1, respectively) were extracted from the cocrystal for clarity (ESI Table S2†), showing that the Ti atom is located beneath a [5,6] bond, while the two Dy atoms are positioned beneath a hexagon and a [5,5,6] junction, respectively. Meanwhile, the shortest distances of the encapsulated metals and the outer cage carbons are 2.399 (9) Å, 2.344 (7) Å, and 2.132 (6) Å for Dy1–C75, Dy2–C11, and Ti–C42, respectively (ESI Table S3†), indicating strong metal–cage interactions which were verified by the high occupancy of two Dy atom and one Ti atom disorders. Furthermore, the bond length of Ti–C81 (1.824 (6) Å) is much shorter than those of Dy1–C81 (2.144(6) Å) and Dy2–C81 (2.175(6) Å) (Fig. 2b), indicating the existence of a Ti
C double bond as the characteristic of μ3-CCF.28,33 It is intriguing to investigate whether the encapsulated Dy2TiC cluster takes a planar or pyramidal configuration. We find that the sum value of ∠Dy1–C81–Ti, ∠Dy2–C81–Ti, and ∠Dy1–C81–Dy2 is 355.4° (Fig. 2c), indicating that the encapsulated Dy2TiC cluster is pyramidal, in which the C81 atom is 0.25 Å deviated from the plane composed of the TiDy2 unit. This is similar to the case of Tb2TiC@C80 but different from the planar cluster found in Lu2TiC@C80, in which the central carbon is 0.07 Å deviated from the trimetallic plane,28 revealing the influence of the size of RE metal on the geometry of the M2TiC cluster.
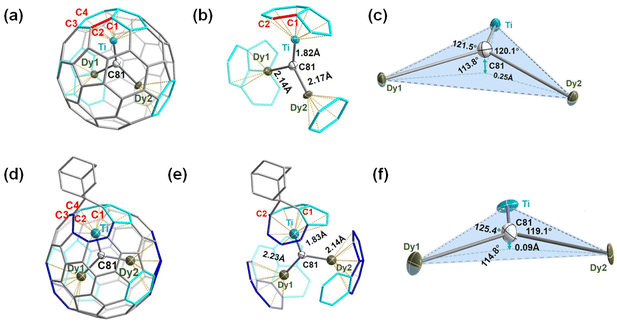 |
| Fig. 2 The crystallographic orientation of the encapsulated Dy2TiC cluster inside (a and b) Dy2TiC@Ih-C80 and (d and e) Dy2TiC@Ih-C80-Ad, as well as (c and f) the configurations of the Dy2TiC cluster inside both fullerene cages, are also shown. The blue and cyan regions indicate the coordination interactions between the encapsulated metals and the fullerene cage, and the C1–C2 bond is highlighted in red to show the difference before and after the Ad addition. | |
Photochemical reactions of Dy2TiC@Ih-C80 with AdN2
Among the chemical functionalization methods developed for metal nitride and carbide clusterfullerenes, a cycloaddition reaction using AdN2 as a reagent has been demonstrated to exhibit high reactivity and regioselectivity.22,24,25,40–43 Hence, in our present work we managed to investigate the cycloaddition reaction of Dy2TiC@Ih-C80 μ3-CCF with AdN2. The photochemical reaction of Dy2TiC@Ih-C80 with AdN2 is shown in Fig. 3a. A mixture solution of Dy2TiC@Ih-C80 and excess AdN2 in toluene was irradiated with a high-pressure mercury lamp, and the reaction process was monitored by analytical HPLC (Fig. 3b). Before the reaction, the HPLC profile of the pristine mixture solution showed two peaks at 3.6 and 39.8 min which were assigned to the AdN2/solvent peak and the pristine Dy2TiC@Ih-C80, respectively. After light irradiation for 5 min, a new peak appeared at 16.5 min, indicating the generation of the monoadduct Dy2TiC@Ih-C80-Ad as confirmed by MALDI-TOF mass spectroscopic characterization. After prolonging the reaction time up to 60 min, the peak at 16.5 min continued to increase along with a decrease of the peak corresponding to the pristine Dy2TiC@Ih-C80, indicating the promoted conversion of Dy2TiC@Ih-C80 to the monoadduct Dy2TiC@Ih-C80-Ad. Finally, the reaction was stopped when an obvious peak of bisadduct at 13.3 min appears. Noteworthily, only one monoadduct Dy2TiC@Ih-C80-Ad was obtained even after the reaction proceeded for 60 min, indicating its high regioselectivity. The resultant solution was then subjected to preparative HPLC separation, and the purity of Dy2TiC@Ih-C80-Ad was verified by analytical HPLC (ESI Fig. S2a†). The purified Dy2TiC@Ih-C80-Ad product was characterized by MALDI-TOF mass spectroscopy, showing an intense mass peak at m/z = 1479.8 (ESI Fig. S2b†).
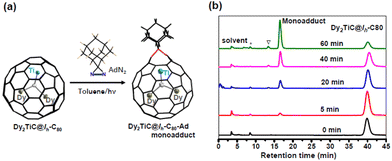 |
| Fig. 3 (a) Synthetic route of Dy2TiC@Ih-C80-Ad through the photochemical reaction of Dy2TiC@Ih-C80 with AdN2; (b) HPLC profiles of the reaction mixtures during the photochemical reaction process of Dy2TiC@Ih-C80 with AdN2 with different reaction times. Conditions: Buckyprep column (∅ 4.6 mm × 250 mm), 20 μL injection volume, 1.0 mL min−1 toluene flow. The inverted triangle and asterisk present the bisadduct and impurity, respectively. | |
To identify the addition pattern of Dy2TiC@Ih-C80-Ad, we grew high-quality cocrystals using DPC as the host similar to the case of the pristine Dy2TiC@Ih-C80, and successfully determined the molecular structure of Dy2TiC@Ih-C80-Ad unambiguously by single-crystal X-ray diffraction. As illustrated in Fig. 1d, the asymmetric crystal unit cell of the 4DPC·2{Dy2TiC@Ih-C80-Ad} cocrystal contains two pairs of fully ordered DPC molecules and two Dy2TiC@Ih-C80-Ad molecules. DPC molecules within 2DPC·{Dy2TiC@Ih-C80-Ad} exhibit a V-shape geometry with a dihedral angle of 66.3° similar to the pristine 2DPC·{Dy2TiC@Ih-C80} cocrystal and the nearest DPC-C80 cage center distances (7.19 Å/6.47 Å) exhibit only minor changes. However, different from the case of the pristine Dy2TiC@Ih-C80 with multiple position disorders of Dy and Ti atoms, the encapsulated Ti atom within Dy2TiC@Ih-C80-Ad exhibits no positional disorders while the two Dy atoms still show several disorders (Fig. 1f, Table S2†). This indicates that the attachment of the Ad group between the C1–C2 bond which is fractured effectively traps the underneath Ti atom as a result of the strengthened Ti-cage interaction as discussed in detail below. Furthermore, the main occupancies of the endohedral two Dy atom disorder are 0.42 and 0.43 for Dy2TiC@Ih-C80-Ad, which are obviously less than those (0.82 and 0.91) of pristine Dy2TiC@Ih-C80, suggesting the weakened interaction of Dy atoms and the Ih-C80 cage after carbene addition.
According to a close analysis of the single crystal structure of Dy2TiC@Ih-C80-Ad, the Ad moiety selectively attacks the [6,6]-bond (conjunction of two fused hexagons, C1–C2) which is adjacent to the Ti4+ ion instead of the two Dy3+ ions shown in Fig. 2d and e. Intriguingly, the distance (2.216(2) Å) of the addition sites (C1, C2) is much longer than that of the conventional C–C single bond (1.54 Å), indicating an open-cage addition pattern.22,41 Hence, a [6,6]-open addition pattern of the Ad moiety can be deduced, which is similar to the cases of photochemical cycloaddition reactions of Ad with metal nitride and carbide clusterfullerenes.22,24,25,40 Based on the largest occupancy, Dy1, Dy2, and Ti atoms within Dy2TiC@Ih-C80-Ad locate underneath a [5,6] bond, a [5,6]-bond, and a fractured [6,6]-bond, respectively (Fig. 2d and e). It is obvious that Ad addition leads to rotation of the Dy2TiC cluster from the coordinated blue region to the cyan region (see Fig. 2ab, d and e) due to the self-adaptive interaction between the endohedral cluster and the outer cage. For the encapsulated Dy2TiC cluster within Dy2TiC@Ih-C80-Ad, the bond lengths of Dy1–C81 (2.242 (5) Å), Dy2–C81 (2.140(5) Å), and Ti–C81 (1.829 (5) Å) are close to those within the pristine Dy2TiC@Ih-C80 as discussed above (ESI Table S3†). These results indicate that the μ3-carbido nature of the encapsulated Dy2TiC cluster remains unchanged after grafting the Ad moiety. Moreover, the sum value of ∠Dy1–C81–Ti, ∠Dy2–C81–Ti and ∠Dy1–C81–Dy2 becomes 359.3°(Fig. 2f), which is larger than that observed for the pristine Dy2TiC@Ih-C80 (355.4°), indicating that the cycloaddition of the Ad moiety to μ3-CCF induces an obvious geometric change of the encapsulated μ3-carbido cluster. A plausible explanation is that the grafted Ad moiety results in the deformation of the Ih-C80 cage with an enlargement of the confined internal space, and thus the strain of the Dy2TiC cluster is reduced.22,24,25,40
Theoretical calculation of Dy2TiC@Ih-C80-Ad
To understand the high regioselectivity of Ad addition to Dy2TiC@Ih-C80 μ3-CCF and unveil the role of the non-RE metal Ti within the μ3-carbido cluster, we performed comprehensive theoretical studies. Density functional theory (DFT) computations on the pristine Dy2TiC@Ih-C80 μ3-CCF with S = 5 spin ground state (spin multiple state 2S + 1 = 11) were carried out, verifying that the encapsulated Dy2TiC cluster takes a μ3-carbido structure bearing a Ti4+ ion and Ti
C double bond (1.79 Å) (ESI Tables S4–S5, Fig. S3–S4†). Besides, the electronic configuration of [(Dy3+)2Ti4+C4−]6+@[Ih(31924)-C80]6− is confirmed similar to the case of the first μ3-CCF Lu2TiC@Ih-C80.28
The high regioselectivity of Ad addition around Ti instead of Dy can be understood by further analyzing the geometric and electronic configuration of Dy2TiC@Ih-C80. First, the shorter Ti–C (cage) distances than those of Dy–C (cage) suggest much stronger interactions between Ti4+ with the Ih-C80 cage (ESI Table S6†), which is also confirmed by the larger Mayer bond order (MBO), density of electrons, and the Laplacian of the electron density between the Ti4+ ion and the Ih-C80 cage than those of Dy3+ ions with the Ih-C80 cage (ESI Tables S6 and S7†). Besides, the Ti4+ ion and its adjacent cage carbons with much more negative charges also contribute to the stronger interactions of Ti4+-C (cage) than that of Dy3+-C (cage) (ESI Fig. S4d†).
Considering the simultaneously appeared [5,6]-adduct and [6,6]-adduct isomers in the reaction of M3N@Ih-C80 (M = Sc, Lu) with carbene Ad: under light irradiation,22 it is interesting that our experimental finding shows that only [6,6]-adduct around the Ti4+ ion obtained with C1 and C2 as the addition sites instead of C2 and C3 ([5,6]-adduct isomer). The theoretical calculations indicate that the [6,6]-adduct has 3.3 kcal mol−1 potential energy (Fig. 4) lower than the [5,6]-adduct. Furthermore, statistical thermodynamic analysis considering the entropy–enthalpy effect shows that [6,6]-open Dy2TiC@Ih-C80-Ad (C1, C2) has the 99.97% distribution relative to that (0.03%) of [5,6]-open Dy2TiC@Ih-C80-Ad (C2-C3) at 298.15 K. Thus, the [6,6]-open Dy2TiC@Ih-C80-Ad obtained in experiment is the thermodynamic product, which is the same as the reactions of AdN2 with several endohedral metallofullerenes previously reported.23,43
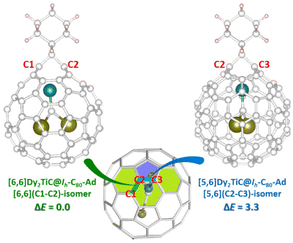 |
| Fig. 4 Relative energies (ΔE, in kcal mol−1) of [5,6]-Dy2TiC@Ih-C80-Ad (in blue) and [6,6]-Dy2TiC@Ih-C80-Ad (in green). The cyan, brown, and light gray balls represent the titanium, dysprosium and carbon atoms, respectively. | |
In order to consolidate the universal role of the Ti(IV) within the μ3-carbido cluster in determining the regioselectivity and reaction mechanism of μ3-CCF, we further computed similar cycloaddition products of the analogously reported μ3-CCF Y2TiC@Ih-C80
33 and nitride clusterfullerene Y2TiN@C80 bearing Ti(III)44,45 with AdN2, in which replacing Dy3+ with Y3+ is to ensure the validity of μ3-CCF clusterfullerenes. Fig. 5 shows that without the presence of Ti(IV), the Ad moiety of Ti(III)-based [6,6]-Y2TiN@Ih-C80-Ad with the lowest potential energy is located nearly around the Y atom. However, similar to Dy2TiC@Ih-C80-Ad, the Ad moiety selectively attacks the [6,6]-bond of Ih-C80 adjacent to the Ti(IV) instead of the Y atom to form a Y2TiC@Ih-C80-Ad adduct with energy superiority. Further frontier molecular orbital (FMO) computations in Fig. S6† show that in the presence of Ti(IV), the FMO occupancy of Dy2TiC@Ih-C80 and Y2TiC@Ih-C80 are similar to each other (ESI Fig. S5†), but different to that of Y2TiN@Ih-C80 bearing Ti(III). This reveals the decisive role of the Ti4+ ion with a high formal oxidation state (IV) in the electronic configuration of μ3-CCF M2TiC@Ih-C80, which directly affects the reactivity. Thus, the cycloaddition reaction of Y2TiC@Ih-C80 with AdN2 is expected to exhibit similar regioselectivity and addition pattern to that of Dy2TiC@Ih-C80.
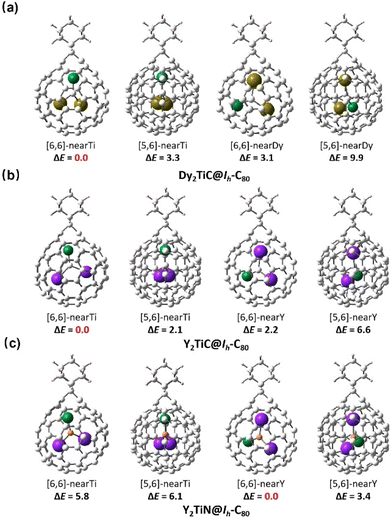 |
| Fig. 5 Relative energies (ΔE, in kcal mol−1) of Dy2TiC@Ih-C80 (a), Y2TiC@Ih-C80 (b) and Y2TiN@Ih-C80 (c) with different addition sites of Ad. The green, brown, purple and light gray balls represent the titanium, dysprosium, yttrium and carbon atoms, respectively. | |
Effect of Ad addition on the electronic properties of Dy2TiC@Ih-C80
In order to unveil the effect of Ad addition on the electronic properties of Dy2TiC@Ih-C80, we carried out UV-vis-NIR spectroscopic characterization and electrochemical studies (Fig. 6). Their characteristic absorption data and redox potentials along with those of other analogous clusterfullerenes are summarized (ESI Table S8†). The absorption spectrum of Dy2TiC@Ih-C80 in the range of 300–1600 nm is featureless, with two broad shoulder peaks at 390 and 676 nm. After grafting an Ad moiety, the shoulder peak at 390 nm becomes more apparent in the absorption spectrum of Dy2TiC@Ih-C80-Ad (Fig. 6a) and the shoulder peak at 676 nm exhibits a negligible change. These results indicate that Ad addition induces little influence on the optical absorption of Dy2TiC@Ih-C80.
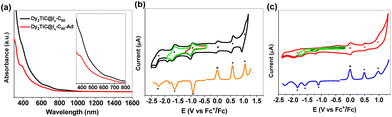 |
| Fig. 6 (a) UV–vis–NIR spectra of Dy2TiC@Ih-C80 and Dy2TiC@Ih-C80-Ad dissolved in toluene. Inset: Enlarged spectral region of 350–800 nm. Cyclic voltammograms of (b) Dy2TiC@Ih-C80 and (c) Dy2TiC@Ih-C80-Ad in 1,2-dichlorobenzene solution with ferrocene (Fc) as the internal standard under a nitrogen atmosphere. Scan rate: 100 mV s−1, tetrabutylammonium hexafluorophosphate (TBAPF6) as the supporting electrolyte. Each redox step is marked with a solid dot to aid comparison; the asterisk denotes the oxidation peak of ferrocene. | |
Cyclic voltammometry (CV) and differential pulse voltammetry (DPV) of the pristine Dy2TiC@Ih-C80 and Dy2TiC@Ih-C80-Ad are then compared as illustrated in Fig. 6b and c. The pristine Dy2TiC@Ih-C80 exhibits two reversible reduction steps, one irreversible reduction step and two reversible oxidation steps which are similar to those reported by Popov et al.33 After Ad addition, all three reduction steps become irreversible, and the first reduction potential shifts negatively from −0.93 V (half-wave potential) to −1.10 V (peak potential), whereas the second and third reduction potentials exhibit a negligible cathodical shift. Only one reversible oxidation step and one irreversible oxidation peak are observed for Dy2TiC@Ih-C80-Ad, with the first oxidation potential cathodically shifting from 0.57 V to 0.49 V relative to that of the pristine Dy2TiC@Ih-C80 (ESI Table S8†). This cathodical shift of redox potentials in Dy2TiC@Ih-C80 after carbene functionalization is similar to those of Ad adducts of M3N@C80 (M = Sc, Lu) reported previously.22
Conclusion
In summary, the first chemical functionalization of μ3-CCF exemplified by Dy2TiC@Ih-C80 is studied, revealing the decisive role of the non-rare earth metal Ti(IV) in high-regioselectivity addition. The molecular structure of the pristine Dy2TiC@Ih-C80 is unambiguously determined by single-crystal X-ray diffraction for the first time, revealing the feature of the Ti
C double bond and the influence of the size of rare earth metals on the geometry of the M2TiC cluster. A photochemical cycloaddition reaction of Dy2TiC@Ih-C80 with I2 affords only one monoadduct Dy2TiC@Ih-C80-Ad, indicating its high regioselectivity. On the basis of the X-ray single crystal structure of Dy2TiC@Ih-C80-Ad, the Ad moiety selectively attacks the [6,6]-bond which is adjacent to the Ti4+ ion instead of the two Dy3+ ions, and the presence of a [6,6]-open addition pattern is confirmed. According to theoretical calculations, Dy2TiC@Ih-C80-Ad is thermodynamically preferred, and the Ti(IV) ion plays a decisive role in high regioselectivity. In contrast, a similar reaction of a Ti(III)-containing nitride clusterfullerene Y2TiN@C80 with AdN2 is predicted theoretically to generate a different type of adduct with the addition site adjacent to the Y3+ ion instead of the Ti3+ ion. Hence, the peculiarity of the chemical properties of μ3-CCF resulting from the existence of the non-rare earth metal Ti with a high oxidation state is unveiled. As the first chemical functionalization of μ3-carbido clusterfullerene with high regioselectivity, our finding on the decisive role of non-rare earth metal titanium in the regioselectivity fulfills an in-depth understanding of the fascinating chemical properties of endohedral fullerenes.
Experimental
Detailed experimental process
A toluene solution (25 mL) containing approximately 5 mg of Dy2TiC@Ih-C80 and excessive AdN2 was first degassed for about 15 minutes and later was irradiated with a high-pressure mercury-arc lamp (cutoff <350 nm) at room temperature. The reaction process was monitored by analytical HPLC. The detailed separation procedure of monoadduct Dy2TiC@Ih-C80-Ad using preparative HPLC is presented in the ESI† (LC-908 instrument, Japan Analytical Industry Co., Ltd, toluene as the mobile phase). MALDI-TOF MS was performed on a BIFLEX III (Bruker, Germany) with 1,1,4,4-tetraphenyl-1,3-butadiene (TPB) as the matrix.
Spectroscopic and electrochemical studies
UV-Vis-NIR spectra were recorded on a UV 3150 (Shimadzu, Japan) in toluene. Cyclic voltammetry (CV) and differential pulse voltammetry (DPV) were obtained in 1,2-dichlorobenzene with 0.1 M (n-Bu)4NPF6 as the supporting electrolyte using a CHI660E workstation. Pt disc, Pt wire and silver wire were used as the working electrode, reference electrode and auxiliary electrode, respectively. The scan rate for CV was 20 mV s−1. Conditions for DPV: pulse amplitude, 50 mV; scan rate, 20 mV s−1.
X-ray crystallographic study
Crystal blocks of Dy2TiC@Ih-C80 and Dy2TiC@Ih-C80-Ad were obtained by slow volatilization of a toluene solution of DPC and Dy2TiC@Ih-C80/Dy2TiC@Ih-C80-Ad in a glass tube. After two weeks, black block crystals suitable for crystal measurements were formed. Single-crystal XRD measurements of Dy2TiC@Ih-C80 and Dy2TiC@Ih-C80-Ad crystals were conducted at 100 K at the BL17B station of Shanghai Synchrotron Radiation Facility. The crystal structures were solved with the ShelXT structure solution program using the Intrinsic Phasing method, later refined with the ShelXL46 refinement package embedded within OLEX2.47 CCDC 2036864 and 2036865† contain the supplementary crystallographic data for Dy2TiC@Ih-C80 and Dy2TiC@Ih-C80-Ad, respectively.
Author contributions
M. C. and Y. Z. contributed equally to this work. M. C. performed the chemical reaction of Dy2TiC@C80 with AdN2 and confirmed the crystal structures of Dy2TiC@C80 and Dy2TiC@C80-Ad by the crystal growth, crystal measurements and resolution. Meanwhile, M. C. wrote the original draft. Y. Z., M. L. and X. Z. carried out the theoretical calculation and analysis of Dy2TiC@C80 and Dy2TiC@C80-Ad. F. J., J. X. and R. G. synthesized and separated Dy2TiC@C80 for crystal growth and further chemical functionalization. Q. Z. provided decapyrrylcorannulene (DPC) which was used as the host to obtain high-quality single crystals of Dy2TiC@C80 and Dy2TiC@C80-Ad. S. X., G. W. and S. Y. provided the discussion and article revision.
Conflicts of interest
The authors declare no competing financial interest.
Acknowledgements
This work was partially supported by the National Key Research and Development Program of China (2017YFA0402800) and the National Natural Science Foundation of China (52172053, 51925206, 21772189, U1932214, 21773181, 21573172 and 91961113). We thank the staff from BL17B1 at Shanghai Synchrotron Radiation Facility for assistance during data collection. We thank the staff from the BL17B1beamline of the National Facility for Protein Science in Shanghai (NFPS) at Shanghai Synchrotron Radiation Facility, for assistance during data collection.
References
- R. B. Ross, C. M. Cardona, D. M. Guldi, S. G. Sankaranarayanan, M. O. Reese, N. Kopidakis, J. Peet, B. Walker, G. C. Bazan, E. Van Keuren, B. C. Holloway and M. Drees, Endohedral fullerenes for organic photovoltaic devices, Nat. Mater., 2009, 8, 208–212 CrossRef CAS.
- J. Tang, R. Zhang, M. Guo, H. Zhou, Y. Zhao, Y. Liu, Y. Wu and C. Chen, Gd-metallofullerenol drug delivery system mediated macrophage polarization enhances the efficiency of chemotherapy, J. Controlled Release, 2020, 320, 293–303 CrossRef CAS PubMed.
- A. R. Puente Santiago, O. Fernandez-Delgado, A. Gomez, M. A. Ahsan and L. Echegoyen, Fullerenes as Key Components for Low-Dimensional (Photo)electrocatalytic Nanohybrid Materials, Angew. Chem., Int. Ed., 2021, 60, 122–141 CrossRef CAS PubMed.
- M. D. Tzirakis and M. Orfanopoulos, Radical Reactions of Fullerenes: From Synthetic Organic Chem-istry to Materials Science and Biology, Chem. Rev., 2013, 113, 5262–5321 CrossRef CAS PubMed.
- M. Yamada, T. Akasaka and S. Nagase, Carbene Additions to Fullerenes, Chem. Rev., 2013, 113, 7209–7264 CrossRef CAS PubMed.
- S. Yang, T. Wei and F. Jin, When metal clusters meet carbon cages: endohedral clusterfullerenes, Chem. Soc. Rev., 2017, 46, 5005–5058 RSC.
- P. Jin, Y. Li, S. Magagula and Z. Chen, Exohedral functionalization of endohedral metallofullerenes: Interplay between inside and outside, Coord. Chem. Rev., 2019, 388, 406–439 CrossRef CAS.
- M. N. Chaur, F. Melin, A. L. Ortiz and L. Echegoyen, Chemical, electrochemical, and structural properties of endohedral metallofullerenes, Angew. Chem., Int. Ed., 2009, 48, 7514–7538 CrossRef CAS PubMed.
- X. Lu, L. Feng, T. Akasaka and S. Nagase, Current status and future developments of endohedral metallofullerenes, Chem. Soc. Rev., 2012, 41, 7723–7760 RSC.
- F.-F. Li, J. R. Pinzon, B. Q. Mercado, M. M. Olmstead, A. L. Balch and L. Echegoyen, [2+2] Cycloaddition Reaction to Sc3N@Ih,-C80. The Formation of Very Stable [5,6]- and [6,6]-Adducts, J. Am. Chem. Soc., 2011, 133, 1563–1571 CrossRef CAS PubMed.
- G.-W. Wang, T.-X. Liu, M. Jiao, N. Wang, S.- E. Zhu, C. Chen, S. Yang, F. L. Bowles, C. M. Beavers, M. M. Olmstead, B. Q. Mercado and A. L. Balch, The cycloaddition reaction of I(h)-Sc3N@C80 with 2-amino-4,5-diisopropoxybenzoic acid and isoamyl nitrite to produce an open-cage metallofullerene, Angew. Chem., Int. Ed., 2011, 50, 4658–4662 CrossRef CAS PubMed.
- C. M. Cardona, A. Kitaygorodskiy, A. Ortiz, M. A. Herranz and L. Echegoyen, The first fulleropyrrolidine derivative of Sc3N@C80: pronounced chemical shift differences of the geminal protons on the pyrrolidine ring, J. Org. Chem., 2005, 70, 5092–5097 CrossRef CAS PubMed.
- M. Chen, R. Guan, B. Li, L. Yang, C. Niu, P. Jin, G.-W. Wang and S. Yang, Anomalous Cis-Conformation Regioselectivity of Heterocycle-Fused Sc3N@D3h,-C78 Derivatives, Angew. Chem., Int. Ed., 2021, 60, 7880–7886 CrossRef CAS.
- O. Semivrazhskaya, A. Romero-Rivera, S. Aroua, S. I. Troyanov, M. Garcia-Borras, S. Stevenson, S. Osuna and Y. Yamakoshi, Structures of Gd3N@C80 Prato Bis-Adducts: Crystal Structure, Thermal Isomerization, and Computational Study, J. Am. Chem. Soc., 2019, 141, 10988–10993 CrossRef CAS PubMed.
- T. Wakahara, Y. Iiduka, O. Ikenaga, T. Nakahodo, A. Sakuraba, T. Tsuchiya, Y. Maeda, M. Kako, T. Akasaka, K. Yoza, E. Horn, N. Mizorogi and S. Nagase, Characterization of the bis-silylated endofullerene Sc3N@C80, J. Am. Chem. Soc., 2006, 128, 9919–9925 CrossRef CAS PubMed.
- Y. Hu, A. Sole-Daura, Y.-R. Yao, X. Liu, S. Liu, A. Yu, P. Peng, J. M. Poblet, A. Rodriguez-Fortea, L. Echegoyen and F.-F. Li, Chemical Reactions of Cationic Metallofullerenes: An Alternative Route for Exohedral Functionalization, Chem. – Eur. J., 2020, 26, 1748–1753 CrossRef CAS PubMed.
- T. Cai, L. Xu, C. Shu, H. A. Champion, J. E. Reid, C. Anklin, M. R. Anderson, H. W. Gibson and H. C. Dorn, Selective formation of a symmetric Sc3N@C78 bisadduct: Adduct docking controlled by an internal trimetallic nitride cluster, J. Am. Chem. Soc., 2008, 130, 2136–2137 CrossRef CAS PubMed.
- T.-X. Liu, T. Wei, S.-E. Zhu, G.-W. Wang, M. Jiao, S. Yang, F. L. Bowles, M. M. Olmstead and A. L. Balch, Azide Addition to an Endohedral Metallofullerene: Formation of Azafulleroids of Sc3N@Ih-C80, J. Am. Chem. Soc., 2012, 134, 11956–11959 CrossRef CAS PubMed.
- M. Chen, L. Bao, M. Ai, W. Shen and X. Lu, Sc3N@Ih,-C80 as a novel Lewis acid to trap abnormal N-heterocyclic carbenes: the unprecedented formation of a singly bonded [6,6,6]-adduct, Chem. Sci., 2016, 7, 2331–2334 RSC.
- M. Chen, W. Shen, P. Peng, L. Bao, S. Zhao, Y. Xie, P. Jin, H. Fang, F.-F. Li and X. Lu, Evidence of Oxygen Activation in the Reaction between an N-Heterocyclic Carbene and M3N@Ih(7)-C80: An Unexpected Method of Steric Hindrance Release, J. Org. Chem., 2017, 82, 3500–3505 CrossRef CAS.
- L. Bao, M. Chen, W. Shen, L. Yang, P. Jin and X. Lu, Lewis Acid-Base Adducts of Sc2C2@C3v(8)-C82/N-Heterocyclic Carbene: Toward Isomerically Pure Metallofullerene Derivatives, Inorg. Chem., 2017, 56, 14747–14750 CrossRef CAS PubMed.
- M. Yamada, T. Abe, C. Saito, T. Yamazaki, S. Sato, N. Mizorogi, Z. Slanina, F. Uhlik, M. Suzuki, Y. Maeda, Y. Lian, X. Lu, M. M. Olmstead, A. L. Balch, S. Nagase and T. Akasaka, Adamantylidene Addition to M3N@Ih-C80 (M=Sc, Lu) and Sc3N@D5h,-C80: Synthesis and Crystallographic Characterization of the [5,6]-Open and [6,6]-Open Adducts, Chem. – Eur. J., 2017, 23, 6552–6561 CrossRef CAS PubMed.
- M. Yamada, Y. Tanabe, J. Dang, S. Sato, N. Mizorogi, M. Hachiya, M. Suzuki, T. Abe, H. Kurihara, Y. Maeda, X. Zhao, Y. Lian, S. Nagase and T. Akasaka,
D
2d
,(23)-C84 versus Sc2C2@D2d(23)-C84: Impact of Endohedral Sc2C2 Doping on Chemical Reactivity in the Photolysis of Diazirine, J. Am. Chem. Soc., 2016, 138, 16523–16532 CrossRef CAS PubMed.
- Y. Iiduka, T. Wakahara, T. Nakahodo, T. Tsuchiya, A. Sakuraba, Y. Maeda, T. Akasaka, K. Yoza, E. Horn, T. Kato, M. T. H. Liu, N. Mizorogi, K. Kobayashi and S. Nagase, Structural determination of metallofullerene Sc3C82 revisited: a surprising finding, J. Am. Chem. Soc., 2005, 127, 12500–12501 CrossRef CAS.
- Y. Iiduka, T. Wakahara, K. Nakajima, T. Nakahodo, T. Tsuchiya, Y. Maeda, T. Akasaka, K. Yoza, M. T. H. Liu, N. Mizorogi and S. Nagase, Experimental and theoretical studies of the scandium carbide endohedral metallofullerene Sc2C2@C82 and its carbene derivative, Angew. Chem., Int. Ed., 2007, 46, 5562–5564 CrossRef CAS PubMed.
- C. Shu, C. Slebodnick, L. Xu, H. Champion, T. Fuhrer, T. Cai, J. E. Reid, W. Fu, K. Harich, H. C. Dorn and H. W. Gibson, Highly Regioselective Derivatization of Trimetallic Nitride Templated Endohedral Metallofullerenes via a Facile Photochemical Reaction, J. Am. Chem. Soc., 2008, 130, 17755–17760 CrossRef CAS PubMed.
- N. B. Shustova, A. A. Popov, M. A. Mackey, C. E. Coumbe, J. P. Phillips, S. Stevenson, S. H. Strauss and O. V. Boltalina, Radical trifluoromethylation of Sc3N@C80, J. Am. Chem. Soc., 2007, 129, 11676–11677 CrossRef CAS PubMed.
- A. L. Svitova, K. B. Ghiassi, C. Schlesier, K. Junghans, Y. Zhang, M. M. Olmstead, A. L. Balch, L. Dunsch and A. A. Popov, Endohedral fullerene with μ3-carbido ligand and titanium-carbon double bond stabilized inside a carbon cage, Nat. Commun., 2014, 5, 3568 CrossRef CAS PubMed.
- K. Junghans, K. B. Ghiassi, N. A. Samoylova, Q. Deng, M. Rosenkranz, M. M. Olmstead, A. L. Balch and A. A. Popov, Synthesis and Isolation of the Titanium-Scandium Endohedral Fullerenes-Sc2TiC@Ih,-C80, Sc2TiC@D5h-C80 and Sc2TiC2@Ih-C80: Metal Size Tuning of the Ti(IV)/Ti(III) Redox Potentials, Chem. – Eur. J., 2016, 22, 13098–13107 CrossRef CAS PubMed.
- C. Fuertes-Espinosa, A. Gomez-Torres, R. Morales-Martinez, A. Rodriguez-Fortea, C. Garcia-Simon, F. Gandara, I. Imaz, J. Juanhuix, D. Maspoch, J. M. Poblet, L. Echegoyen and X. Ribas, Purification of Uranium-based Endohedral Metallofullerenes (EMFs) by Selective Supramolecular Encapsulation and Release, Angew. Chem., Int. Ed., 2018, 57, 11294–11299 CrossRef CAS PubMed.
- A. Brandenburg, D. S. Krylov, A. Beger, A. U. B. Wolter, B. Buchner and A. A. Popov, Carbide clusterfullerene DyYTiC@C80 featuring three different metals in the endohedral cluster and its single-ion magnetism, Chem. Commun., 2018, 54, 10683–10686 RSC.
- F. Liu, F. Jin, S. Wang, A. A. Popov and S. Yang, Pyramidal TiTb2C cluster encapsulated within the popular Ih(7)-C80 fullerene cage, Inorg. Chim. Acta, 2017, 468, 203–208 CrossRef CAS.
- K. Junghans, C. Schlesier, A. Kostanyan, N. A. Samoylova, Q. M. Deng, M. Rosenkranz, S. Schiemenz, R. Westerstrom, T. Greber, B. Buchner and A. A. Popov, Methane as a Selectivity Booster in the Arc-Discharge Synthesis of Endohedral Fullerenes: Selective Synthesis of the Single-Molecule Magnet Dy2TiC@C80 and Its Congener Dy2TiC2@C80, Angew. Chem., Int. Ed., 2015, 54, 13411–13415 CrossRef CAS PubMed.
- M. N. Chaur, R. Valencia, A. Rodriguez-Fortea, J. M. Poblet and L. Echegoyen, Trimetallic Nitride Endohedral Fullerenes: Experimental and Theoretical Evidence for the M3N6+@C2n(6−) model, Angew. Chem., Int. Ed., 2009, 48, 1425–1428 CrossRef CAS PubMed.
- J. M. Campanera, C. Bo and J. M. Poblet, General rule for the stabilization of fullerene cages encapsulating trimetallic nitride templates, Angew. Chem., Int. Ed., 2005, 44, 7230–7233 CrossRef CAS PubMed.
- A. A. Popov and L. Dunsch, Structure, stability, and cluster-cage interactions in nitride clusterfullerenes M3N@C2n (M = Sc, Y; 2n = 68-98): a density functional theory study, J. Am. Chem. Soc., 2007, 129, 11835–11849 CrossRef CAS PubMed.
- Y. Hu, Y.-R. Yao, X. Liu, A. Yu, X. Xie, L. Abella, A. Rodriguez-Fortea, J. M. Poblet, T. Akasaka, P. Peng, Q. Zhang, S.-Y. Xie, F.-F. Li and X. Lu, Unexpected formation of 1,2- and 1,4-bismethoxyl Sc3N@Ih,-C80 derivatives via regioselective anion addition: an unambiguous structural identification and mechanism study, Chem. Sci., 2021, 12, 8268–8268 RSC.
- F. Jin, J. Xin, R. Guan, X. Xie, M. Chen, Q. Zhang, A. A. Popov, S.-Y. Xie and S. Yang, Stabilizing a three-center single-electron metal-metal bond in a fullerene cage, Chem. Sci., 2021, 12, 6890–6895 RSC.
- Y.-Y. Xu, H.-R. Tian, S.-H. Li, Z.-C. Chen, Y.-R. Yao, S.-S. Wang, X. Zhang, Z.-Z. Zhu, S.-L. Deng, Q. Zhang, S. Yang, S.-Y. Xie, R.-B. Huang and L.-S. Zheng, Flexible decapyrrylcorannulene hosts, Nat. Commun., 2019, 10, 485 CrossRef CAS PubMed.
- H. Kurihara, X. Lu, Y. Iiduka, H. Nikawa, N. Mizorogi, Z. Slanina, T. Tsuchiya, S. Nagase and T. Akasaka, Chemical Understanding of Carbide Cluster Metallofullerenes: A Case Study on Sc2C2@C2v(5)-C80 with Complete X-ray Crystallographic Characterizations, J. Am. Chem. Soc., 2012, 134, 3139–3144 CrossRef CAS PubMed.
- X. Liu, B. Li, W. Yang, Y.-R. Yao, L. Yang, J. Zhuang, X. Li, P. Jin and N. Chen, Synthesis and characterization of carbene derivatives of Th@C3v(8)-C82 and U@C2v(9)-C82: exceptional chemical properties induced by strong actinide-carbon cage interaction, Chem. Sci., 2021, 12, 2488–2497 RSC.
- W. Zhang, M. Suzuki, Y. Xie, L. Bao, W. Cai, Z. Slanina, S. Nagase, M. Xu, T. Akasaka and X. Lu, Molecular Structure and Chemical Property of a Divalent Metallofullerene Yb@C2(13)-C84, J. Am. Chem. Soc., 2013, 135, 12730–12735 CrossRef CAS PubMed.
- X. Lu, H. Nikawa, L. Feng, T. Tsuchiya, Y. Maeda, T. Akasaka, N. Mizorogi, Z. Slanina and S. Nagase, Location of the yttrium atom in Y@C82 and its influence on the reactivity of cage carbons, J. Am. Chem. Soc., 2009, 131, 12066–12067 CrossRef CAS PubMed.
- C. Chen, F. Liu, S. Li, N. Wang, A. A. Popov, M. Jiao, T. Wei, Q. Li, L. Dunsch and S. Yang, Titanium/Yttrium Mixed Metal Nitride Clusterfullerene TiY2N@C80: Synthesis, Isolation, and Effect of the Group-III Metal, Inorg. Chem., 2012, 51, 3039–3045 CrossRef CAS PubMed.
- S. Wang, J. Huang, C. Gao, F. Jin, Q. Li, S.-Y. Xie and S. Yang, Singly Bonded Monoadduct rather than Methanofullerene: Manipulating the Addition Pattern of Trimetallic Nitride Clusterfullerene through One Endohedral Metal Atom Substitution, Chem. – Eur. J., 2016, 22, 8309–8315 CrossRef CAS PubMed.
- G. M. Sheldrick, Crystal structure refinement with SHELXL, Acta Crystallogr., Sect. C: Struct. Chem., 2015, 71, 3–8 Search PubMed.
- O. V. Dolomanov, L. J. Bourhis, R. J. Gildea, J. A. Howard and H. Puschmann, OLEX2: a complete structure solution, refinement and analysis program, J. Appl. Crystallogr., 2009, 42, 339–341 CrossRef CAS.
Footnotes |
† Electronic supplementary information (ESI) available. CCDC 2036864 and 2036865. For ESI and crystallographic data in CIF or other electronic format see DOI: https://doi.org/10.1039/d2qi01442d |
‡ These authors contributed equally to this work. |
|
This journal is © the Partner Organisations 2022 |