DOI:
10.1039/D2GC02332F
(Tutorial Review)
Green Chem., 2022,
24, 6435-6449
Cyrene: a bio-based novel and sustainable solvent for organic synthesis
Received
21st June 2022
, Accepted 28th July 2022
First published on 28th July 2022
Abstract
Dihydrolevoclucosenone (Cyrene is its market name) is a biomass-derived solvent that can be produced in only two steps from biomass, while being biodegradable, non-mutagenic and non-toxic. Its “green” profile combined with its physical properties makes Cyrene a plausible substitute for a number of widely used toxic organic solvents. The first attempt to assess Cyrene as a solvent dates back to 2014 and since then, numerous research groups have opted for this promising alternative. Cyrene has successfully been employed as a solvent in materials chemistry, peptide chemistry, organic synthesis and many more research fields, which are going to be discussed in the context of this review.
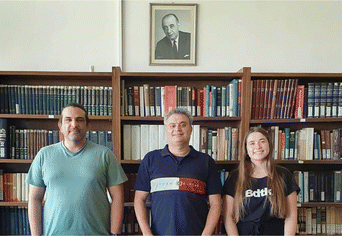 From left to right: Dr Petros L. Gkizis, Assoc. Professor Christoforos G. Kokotos and Naya A. Stini | Naya A. Stini is a MSc student at the Department of Chemistry, National and Kapodistrian University of Athens, under the supervision of Assoc. Professor Christoforos G. Kokotos. Naya studied Chemistry at the Department of Chemistry of the National and Kapodistrian University of Athens and graduated in 2021 with honors. She then begun her MSc studies in the Kokotos group and her research interests focus on Green Chemistry and Photochemistry. Dr Petros L. Gkizis was born in Athens, Greece. He obtained his BSc and MSc degrees and PhD from the Aristotle University of Thessaloniki. In 2012, he was appointed as a postdoctoral fellow at the Aristotle University of Thessaloniki in collaboration with the University of Crete (ERC-Grant). In 2014, he was appointed as a Technology Transfer Research Scientist at Pharmathen S.A. Pharmaceuticals, Thessaloniki, Greece. In 2020, he joined the group of Prof. Christoforos G. Kokotos at the National and Kapodistrian University of Athens as a postdoctoral researcher. His research interests focus on the development of photochemical reactions for the formation of C–C bonds and their application in the synthesis of pharmaceuticals and bioactive compounds. Prof. Christoforos G. Kokotos was born in Athens, Greece (1981) and studied Chemistry at the National and Kapodistrian University of Athens, Greece (2003). He completed his PhD studies at the University of Bristol, UK (2007) under the supervision of Prof. Varinder Aggarwal. He then carried out postdoctoral work at Princeton University, USA in the group of Prof. David W. C. MacMillan. He was elected as a Lecturer of Organic Chemistry in the Department of Chemistry of the National and Kapodistrian University of Athens, Greece and promoted to Assistant Professor (2016) and Associate Professor (2020). He was also elected as a Chemistry Europe Fellow in 2022 (class 2020–2021). His research interests focus on asymmetric organocatalysis, photocatalysis and green methodologies on oxidation reactions and their application in the synthesis of pharmaceuticals, bioactive molecules and high-added value chemicals. |
1. Introduction
Over the past few years, the increasing emphasis on green chemistry has turned the interest of many scientists worldwide towards research fields, such as the development of green solvents and water-mediated or solvent-free reactions. This high demand for environmentally friendly procedures to access products has increased the development of more sustainable processes in the last few years.1 Furthermore, one of the major issues faced by the chemical industry is the large amount of waste generated by the chemical processes every day. The amount of waste is mainly associated with solvents, thus the replacement of widely used, but toxic organic solvents, by safer and non-toxic alternatives is of great importance. The basic concern of a process chemist is to alter the employed reaction schemes, avoiding hazardous solvents or reagents, in order to decrease the amount of waste produced. This idea is exceptionally conceptualized by Anastas and coworkers under the title of the Twelve Principles of Green Chemistry.2
In the context of this review, we intend to focus on a novel bio-based solvent, which is produced by Circa under the trade name Cyrene.3 There is a number of articles or reviews focused on the earlier attempts at using Cyrene as a solvent4–6 and the readers can divert their attention to these for some of the early contributions. Our aim is to focus and discuss Cyrene-mediated reactions that provide environmentally friendly solutions. Cyrene is considered to be a potential alternative to dimethyl formamide (DMF), N-methylpyrrolidone (NMP), dimethyl sulfoxide (DMSO) or N,N-dimethylacetamide (DMAc), which are considered toxic organic solvents, both for the environment and human health.7 Lately, great effort has been devoted to replacing toxic and hazardous organic solvents with novel easily-disposable solvents, minimizing the ecological footprint.
2. General remarks
Cyrene synthesis from biomass
Dihydrolevoglucosenone or 6,8-dioxabicyclo[3.2.1] octanone is a cellulose-derived solvent, which can be synthesized in a two-step process from biomass. The first step for its synthesis was reported back in 1973 by Broido and coworkers,8a who characterized correctly the chemical structure of levoglucosenone (LGO), as a result of cellulose pyrolysis (Scheme 1). Although Cyrene was synthesized before, the first structural characterization of the molecule was incorrect. Since then, a lot of effort has been put in increasing the conversion of cellulose into LGO. Various methods have been developed concerning the pyrolysis of cellulose, mostly by investigating a wide range of acids to succeed in obtaining the highest yields.8 In 2011, the Circa group reported a thermal method which afforded LGO in 40% yield.9 The Circa group patented a method for the formation of LGO, which involves the catalytic pyrolysis of biomass using phosphoric acid in sulfolane.9 Having in hand the best results for producing the main precursor of dihydrolevoglucosenone, Circa initiated the industrial production of this promising derivative under the trade name Cyrene,3 and since then the interest in its use as a bio-based solvent has been gradually increasing.
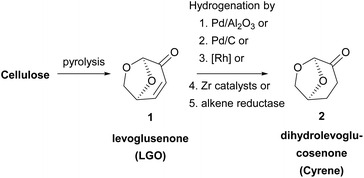 |
| Scheme 1 Production of Cyrene from cellulose. | |
The second and final step involves the reduction of levoglucosenone, by hydrogenation, to afford Cyrene (Scheme 1). In this context, various palladium catalysts have been widely employed, such as Pd/Al2O3
10 or Pd/C;6,10 however, rhodium catalysts and zirconia-supported catalysts have been used as well.11 Moreover, a one-pot synthesis of Cyrene from levoglucosenone was suggested by Wang and coworkers,12 while in 2018, levoglucosenone was reduced to Cyrene using an alkene reductase.13
Properties of Cyrene
Regarding its chemical structure, Cyrene is an optically active ketone composed of two fused rings, which form a cyclic acetal (Scheme 1). The ring fusion results in a double anomeric effect, which stabilizes the existence of the cyclic acetal.5 In general, acetals are stable towards bases and nucleophiles and Cyrene is very unstable toward strong acids and oxidizing or reducing agents.6
Cyrene is a colorless viscous liquid with a high boiling point (227 °C), which offers the possibility to mediate reactions taking place in a wide range of temperatures. Cyrene's polarity profile was studied using DFT experiments, complemented by the Kamlet–Abboud–Taft parameters that were also obtained (Table 1).5 The results showed that Cyrene has a similar π* value to N-methylpyrrolidone (NMP), which verifies its definition as an aprotic solvent. As far as the Hansen solubility parameters (HSP) are concerned, Cyrene presents a similar dispersion term (δD) to DMSO, a polar term (δp) similar to DMAc and hydrogen bonding interactions (δH) similar to NMP.6 The density of Cyrene is 1.25 g mL−1 at 293 K. Compared to NMP, DMF, DMSO or DMAc which are listed as hazardous (H360) by the Registration, Evaluation, Authorization and Restriction of CHemicals (REACH), Cyrene has no known toxicity issues.7 Thus, major pharmaceutical companies seek for an alternative reaction medium.7a Additionally, Cyrene is biodegradable, biorenewable, non-mutagenic and non-reprotoxic (Table 1).7 Cyrene is also miscible with water, which appears to be a very interesting property, since the high miscibility in water facilitates Cyrene removal from reaction mixtures using liquid–liquid extraction. This feature is extremely important, especially in industrial scale reactions, since Cyrene can be easily removed from the reaction mixture and upon water distillation, it can be easily reused.
Table 1 Physical properties of Cyrene versus other dipolar aprotic solvents
Properties |
Cyrene |
NMP |
DMF |
DMSO |
DMAc |
Boiling point |
227 °C |
202 °C |
153 °C |
189 °C |
165 °C |
Density [g ml−1] |
1.25 |
1.03 |
0.948 |
1.100 |
0.937 |
Solubility with water |
Miscible |
Miscible |
Miscible |
Miscible |
Miscible |
π* |
0.93 |
0.90 |
0.88 |
1.00 |
0.85 |
δD [MPa] |
18.8 |
18.0 |
17.4 |
18.4 |
16.8 |
δP[MPa] |
10.6 |
12.3 |
13.7 |
16.4 |
11.5 |
δH [MPa] |
6.9 |
7.2 |
11.3 |
10.2 |
10.2 |
3. Cyrene in organic chemistry
Cyrene as a reactant or a reagent
Since the interest in Cyrene began to rise, several research groups initially investigated its use in several benchmark reactions. Based on its structure, Cyrene behaves as a ketone bearing two α-reactive positions. Thus, Cyrene was examined as a reactant in various reactions, such as the Beckmann reaction,14 the Claisen–Schmidt reaction with aldehydes,15 the Bayer–Villiger oxidation16 and hydrogenation with various metals (Scheme 2).17 Additionally, its chiral chemical structure was used for the preparation of chiral auxiliaries for Diels–Alder reactions18a or sulfa-Michael reactions.18b Furthermore, in 2018, Mencer and coworkers reported the formation of exocyclic enones, upon condensation of Cyrene with aldehydes, which could further react with another molecule of the carbonyl compound, leading to a structure like 8,19 while in 2019, Ray and coworkers reported the polymerization of Cyrene, aiming at bio-based polymers.20
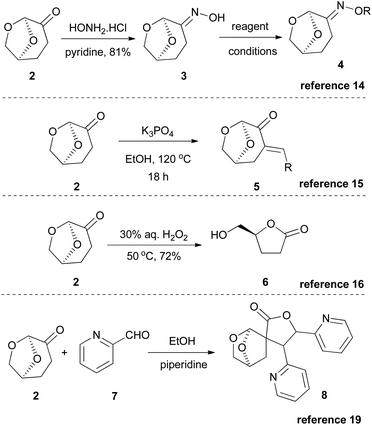 |
| Scheme 2 Examples of reactions where Cyrene was used as a reactant. | |
Reactions where Cyrene fails to become a suitable solvent
Interestingly in most literature reports, researchers tend to present their successful results; however, non-successful results have their own merit. For further reference, reactions where Cyrene is not a compatible solvent could be of high interest. Even though Cyrene appears to be an excellent choice of solvent, as we are going to discuss later in this review article, its reactivity that arises from its structure in some cases narrows down its use. In 2018, Hunt and coworkers reported that Cyrene had a very low performance when employed in biocatalytic esterifications, although it was commented that neither DMF nor NMP were effective.21 Their incompatibility with this reaction can be attributed to the fact that polar solvents disrupt the water layer around the enzyme, decreasing its activity.22 In 2017, Szekely and coworkers attempted to use Cyrene as the solvent in a diastereoselective synthesis of 2,4,5-trisubstituted-2-imidazolines from benzaldehyde and ammonia.23 The results were far from successful, since no product was obtained. The high reactivity of Cyrene when it is employed as the solvent may cause that failure. Under no circumstances should we forget the nature of Cyrene as a compound, which implies that in reactions, such as the Grignard reaction (Scheme 3A) or aldol reaction (Scheme 3B), it could not be used as the solvent, as it would react.
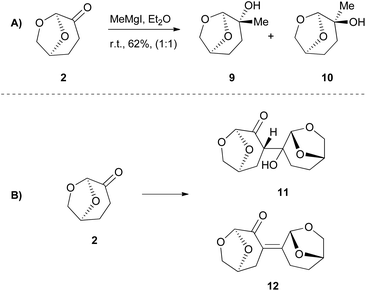 |
| Scheme 3 (A) Grignard reaction, (B) aldol condensation reaction of Cyrene. | |
Cyrene as the reaction medium in cross-coupling reactions
As already stated, our main intention in this review is to highlight the use of Cyrene as an alternative and eco-friendly solvent. Furthermore, we intend to discuss the replacement of dipolar aprotic solvents by Cyrene and its applications in numerous reactions, such as cross-coupling reactions. The latter was first reported by Sherwood and coworkers, who briefly studied the efficiency of Cyrene in the Menschutkin reaction4 and the fluorination nucleophilic substitution reactions,4 which are going to be discussed later in the context of this review.
Sonogashira reaction
In 2016, Watson and coworkers were the first to attempt a Pd-catalyzed cross coupling reaction using Cyrene as the solvent (Table 2).24 In general, the Sonogashira reaction takes place in a dipolar aprotic solvent, thus DMF is mostly used in this kind of reaction. The attempt to replace DMF posed some challenges, such as the need for a higher catalyst loading and/or higher reaction temperatures.24 Furthermore, the authors in this case experienced prolonged reaction times and lower yields. They initiated their investigations using iodobenzene (13) and phenylacetylene (14) as a model reaction to find the optimum reaction conditions (Table 2).24 The employed palladium catalyst was [Pd(PPh3)Cl2] and CuI was used as the additive. A variety of bases were tested, but only Et3N afforded the desired product, whereas in the cases where K3PO4 and Cs2CO3 were employed, no product was obtained (Table 2, entries 3 and 4 vs. 1, Table 1). In these cases, it was reported that the aldol reaction products of Cyrene, 11 and 12 (Scheme 3B), were formed. The authors examined the formation of the aldol condensation by-products using a variety of bases at different temperatures. All inorganic bases, apart from KOAc at 25 °C, resulted in the condensation products. As far as organic bases were concerned, pyridine and DBU delivered the condensation product at every temperature that was tested, whereas DIPEA and Et3N led to the condensation product, only when the reaction was performed at 100 °C. Following their observations, the authors employed Et3N at room temperature to suppress the aldol condensation reaction of Cyrene.24 With the optimized conditions in hand, they investigated the scope of aryl iodides in the Sonogashira reaction. Electron-deficient and electron-rich aryl iodides afforded the desired product in excellent yields. meta- or ortho-Substituted aryl and heterocyclic iodides were also examined, leading to the desired alkynes in good to excellent yields.24 Furthermore, aryl bromides were also employed, but the obtained yields were significantly lower. A vast variety of alkynes were also employed to react with iodobenzene, affording the desired product in excellent yields, as most of the reactions were quantitative. Finally, heteroaryl alkynes and ortho-substituted aryl iodides were used, affording the desired products in good to excellent yields.
Table 2 Sonogashira reaction of 13 with 14 using Cyrene as the solvent
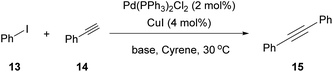
|
Entry |
Base |
Temperature (°C) |
Base equiv. |
Reaction time (h) |
Yield % |
All reactions were carried out with 13 (0.25 mmol), 14 (0.26 mmol), Pd(PPh3)2Cl2 (2 mol%), CuI (4 mol%), base, Cyrene, temperature, various reaction times, and N2. |
1 |
Et3N |
20 |
3 |
5 |
100 |
2 |
Et3N |
20 |
1.1 |
5 |
98 |
3 |
K3PO4 |
20 |
3 |
5 |
— |
4 |
Cs2CO3 |
20 |
3 |
5 |
— |
5 |
Et3N |
30 |
1.1 |
1 |
96 |
At the same time, a Cacchi-type reaction was reported with the use of ortho-amino or ortho-hydroxyaryl iodides (Scheme 4).24 The generated intermediate from the Sonogashira coupling reaction undergoes a 5-endo-dig cyclization, upon increasing the reaction temperature. The resulting substituted benzofurans, indoles or aza-indoles constitute important classes of molecules, widely used in biological and pharmaceutical sciences. In both reported protocols, the reaction output using both Cyrene and DMF is similar, indicating that Cyrene can be an excellent alternative medium for the Sonogashira cross-coupling reaction.
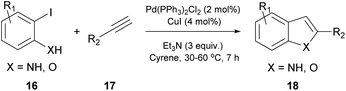 |
| Scheme 4 Cacchi-type annulations of α-aminohydroxy iodoarenes. | |
Suzuki–Miyaura cross-coupling reaction
Watson and coworkers, in their effort to expand the use of Cyrene in palladium-catalyzed cross coupling reactions, examined its use in a classical Suzuki–Miyaura coupling reaction (Table 3).25 In the literature, suitable solvents for the Suzuki–Miyaura reaction are usually THF, DMF or 1,4-dioxane. In 2018, the authors investigated the use of Cyrene, for a more environmentally friendly approach, affording similar reaction yields.25 In their efforts to find the optimized conditions, the authors employed 4-bromotoluene (19) and phenylboronic acid (20) as a model cross coupling reaction (Table 3).25 Different bases were examined and Cs2CO3 outperformed all others. The major drawback appeared to be the consistency of the reaction. The formation of dimer 12 (resulted from the aldol condensation of Cyrene) was not controllable, leading to different results each time. To overcome this problem, water was exploited as the co-solvent, facilitating the fluidity of the reaction mixture.25 They further examined the co-solvent prospect at different temperatures, and higher yields were obtained when a significantly higher reaction temperature was used.
Table 3 Suzuki–Miyaura reaction of 19 with 20 using Cyrene as the solvent
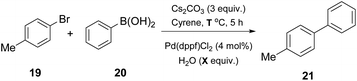
|
Entry |
Solvent |
H2O (equiv.) |
Temperature (°C) |
Yield (%) |
All reactions were carried out with 19 (0.25 mmol), 20 (0.25 mmol), and Pd(dppf)Cl2·CH2Cl2 (4 mol%). |
1 |
Cyrene |
5 |
20 |
82 |
2 |
Cyrene |
5 |
30 |
— |
3 |
Cyrene |
1.8 mL |
20 |
81 |
4 |
Cyrene |
1.8 mL |
30 |
80 |
5 |
Cyrene |
1.8 mL |
50 |
94 |
6 |
DMF |
1.8 mL |
50 |
98 |
7 |
THF |
1.8 mL |
50 |
92 |
8 |
1,4-Dioxane |
1.8 mL |
50 |
100 |
9 |
H2O |
|
50 |
31 |
Having the optimized conditions in hand, different classes of organoboron reagents were tested, as well as various organoboron nucleophiles and aryl electrophiles. Electron-rich and electron-deficient aryl halides afforded great to excellent results, as did all the organoboron nucleophiles that were tested.25 An interesting finding by the authors was that the use of a medium polarity solvent, such as 40% EtOAc in petroleum ether, facilitated the purification of the products, since dimer 12 was removed via filtration as it precipitated easily. They also continued by probing the synthesis of 4′-methyl-biphenylcarbonitrile (OTBN) 24, which is a key intermediate in the synthesis of an angiotensin II receptor antagonist (Scheme 5).25 Thus, they applied the optimized reaction conditions in a larger scale of four grams. The results were very promising, since the obtained yield agreed with the expected yield in smaller scales.
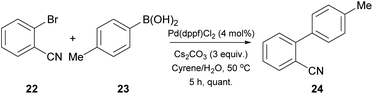 |
| Scheme 5 Gram-scale synthesis of OTBN 24. | |
Dimerization of sinapic esters
Among the most challenging tasks in organic synthesis is the preparation of β–β′ dimers of sinapic acid derivatives. The major drawback in all the methodologies reported in the literature is the formation of the β-O-4′ dimer. Having studied the β–β′ dimerization of sinapyl alcohol, Allais and coworkers concluded that a lot of presented problems could be easily circumvented by tuning the reaction parameters.26 Later in 2020, they proposed a copper(I)-catalyzed dimerization process.27 Ethyl sinapate was chosen as the model compound and they applied conditions that were found in previous work of sinapyl alcohol dimerization. Instead of the desired product 26, a complicated mixture comprising the desired dimer 26, the β-O-4′ dimer 27 and a dimer obtained by a rearrangement of the desired dimer ethyl sinapate 28, that did not react with other oligomers, was obtained (Scheme 6). The method involved the utilization of an enzyme (laccase from Trametes versicolor), whose high affinity with the dimers may explain the presence of oligomers. Another major issue was the formation of the rearrangement product, whose solution was not efficient by changing the solvent. Thus, the authors decided to use a different enzyme, Horseradish Peroxidase, HRP (types II and IV), but no better results were attained. After several unsuccessful attempts, they opted for mimicking the active site of the enzyme using copper(I) bromide, air (O2) and pyridine at 50 °C for 24 h. Under these conditions, the desired product was obtained with good selectivity. Later, they sought greener alternatives for the reaction, where Cyrene was found to be the most efficient solvent for the reaction. Apart from greening the process, the use of Cyrene as the reaction medium led to a decrease in the amount of pyridine used, since it did not play the role of the solvent and it was just used only as the oxidizing agent. The optimized conditions were applied to several sinapic esters with great results (Scheme 6). Longer aliphatic chains reacted very well, as did bulkier substituted groups, achieving high yields (87% to 91%). For purification, a simple extraction with EtOAc and HCl was enough to obtain the pure product.
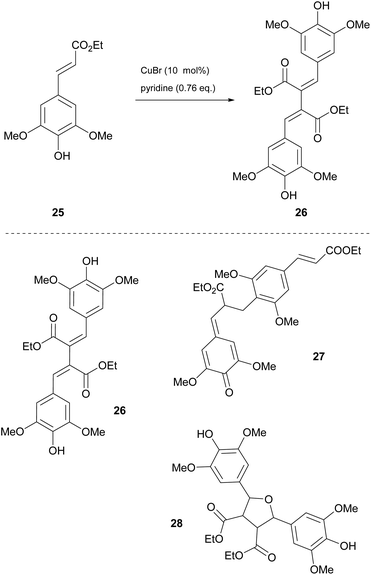 |
| Scheme 6 Synthesis of the sinapate β–β′ dimer 26 using Cyrene as the solvent and various products of ethyl sinapate dimerization. | |
C–H difluoromethylation reactions
In 2021, Quero and coworkers studied the difluoromethylation of heteroarenes (29) or terminal alkynes (31) (Scheme 7).28 They used copper iodide (CuI) particles anchored onto boron nitrate nanosheets (BNNs). Due to their unique properties, BNNs have gained a lot of attention lately. The authors anchored CuI nanoparticles and created a heterogenous catalyst, with which they performed the fluoromethylation reaction. They initiated their investigation using benzothiazole and TMSCF2H, as the fluorination source, using NMP as the solvent and various bases such as t-BuOK, Na2CO3, NaHCO3 and KOH. Finally, the best results were obtained with the use of KOH in the presence of Cyrene as the solvent (Scheme 7).28 The proposed mechanism for the reaction demands the existence of an oxidant, which oxidizes the substrate to produce an intermediate able to undergo a reductive elimination and afford the final product. Simultaneously, the catalyst is restored. They used 9,10-phenanthrequinone (PQ) as the oxidant.28 Having the best conditions in hand, the authors researched the scope of substrates which may effectively react. A wide range of substituted benzothiazoles were studied and electron-richer benzothiazoles seemed to be more effective than electron-deficient ones. They also examined the recovery and recyclability of the catalyst. Upon reaction completion, the catalyst was filtered and washed with ethyl acetate and water, and dried at 65 °C. The catalyst was reused up to five times, concluding in an effective protocol with the use of biosustainable Cyrene as the solvent. The authors indicated that replacing common solvents, such as NMP and DMF, with Cyrene led to short reaction times and greater yields. Furthermore, the reported protocol was applied successfully to a wide range of substrates.
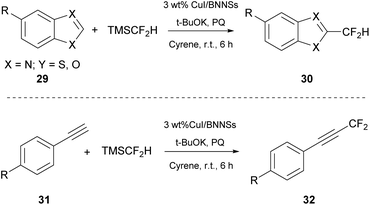 |
| Scheme 7 Difluoromethylation of heteroarenes (29) and terminal alkynes (31) using Cyrene as the solvent. All reactions were performed with 29 or 31 (1.0 equiv.), TMSCF2H (2.0 equiv.), catalyst (10 mg), t-BuOK (3 equiv.), and 9,10-phenanthrenequinone (PQ) (1.2 equiv.). | |
Synthesis of ureas
Having in mind the pivotal role of ureas in a variety of fields, in 2017, Camp and coworkers tried to synthesize ureas from amines and isocyanates using Cyrene, instead of DMF, as a greener approach (Scheme 8).29 The major drawback using DMF in the synthesis of urea derivatives appears to be the long reaction time. Additionally, extensive work-up and purification procedures are required. Since DMF is considered as a problematic solvent in industry, and additional work-up procedures increase the manufacturing cost, Cyrene was considered as an alternative solvent, eliminating these disadvantages. The investigation began with a model reaction which involved phenylisocyanate (33) and pyrrolidine (34) in Cyrene (Scheme 8).29 A simple optimization followed, whilst the major challenge was the development of a purification protocol to minimize the by-products. To develop an industrial applicable protocol the authors suggested a simple work-up process using water and dichloromethane for a liquid–liquid extraction process. However, in many cases, further purification was needed, since in some cases Cyrene co-eluted with the product. The authors in their effort to provide a greener reaction protocol investigated alternative purification protocols to minimize the amount of waste produced. They proceeded with trying different acid/base work-up procedures, but both Cyrene and the synthesized urea derivatives were decomposed. Surprisingly, the addition of water to the reaction mixture facilitated the purification of the formed urea, up to the point that all Cyrene was removed from the product. The water addition for the removal of Cyrene also eliminated the use of non-green solvents, used in other methods. Having found the optimum reaction conditions for both the reaction and the purification of the products, the authors tried to expand the scope of the amines used, when they reacted with phenyl isocyanate.29 Cyclic and aliphatic secondary amines led to the desired products in good to excellent yields. The authors also examined the effect of a substituent on the aryl ring of the isothiocyanate. It appears that electron-deficient aryl isocyanates gave better results than the electron-rich ones, which is attributed to the increased electrophilicity of the isothiocyanate carbon. The position of the substituent also plays a crucial role, since due to steric hindrance, ortho-substituted aryl isothiocyanate derivatives led to significantly lower yields. Overall, a greener alternative for the synthesis of ureas was proposed and it was proven that Cyrene can possibly be used industrially as a solvent, since its removal is possible, just by treating the reaction with water.
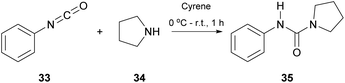 |
| Scheme 8 Synthesis of urea 35 in Cyrene. | |
Isothiocyanate synthesis
In 2021, Meier and coworkers, having underlined the importance of developing in situ procedures for the formation of isothiocyanates (ITCs) due to their toxicity, suggested a method that minimizes the negative impact.30 In that work, the authors employed elemental sulfur as the sulfurization agent to avoid the use of metal catalysts, widely used in other similar approaches. Knowing from the literature that amines could be used as organocatalysts in reactions that had isothiocyanates as isolable intermediates, they began their investigations by employing tertiary amines as organocatalysts. The use of tertiary amines to trap the intermediate is essential, since in the presence of primary or secondary amines, the ITC intermediate is instantly converted into a thiourea (Table 4).30 Next, optimization of the reaction conditions such as the temperature, solvent and stoichiometry was done. In addition, the optimization of the purification method was also done. With the optimum conditions in hand, a wide range of amines reacted with a model isocyanide, n-dodecyl isocyanide (36) (Table 4).30 Various bases were also tested, with 1,8-diazabicyclo(5.4.0)undec-7-ene (DBU) and triazabicyclodecene (TBD) leading to quantitative conversion, whereas 4-dimethylaminopyridine (DMAP) and 1-methylimidazole (NMI) were not as efficient (entries 1–3 and 6, Table 4). Triethylamine (TEA) and 1,4-diazabicyclo[2.2.2]octane (DABCO) also afforded remarkable results (entries 4 and 5, Table 4). With these results, the amount of base needed was also investigated and DBU demonstrated great results, when used in a catalytic amount, in just 4 hours (entries 11 and 12, Table 4). A temperature screening followed, and moderate temperatures were decided to be used. Finally, they sought the best solvent for the reaction among numerous green solvents to decrease the environmental impact. Cyrene led to the quantitative formation of the desired product after two hours reaction time, while GVL and DMSO afforded 92% and 85% yield, respectively. This can be easily attributed to its ability to dissolve and stabilize polysulfur chains.
Table 4 Base screening for the optimization of the reaction using DMSO as the solvent
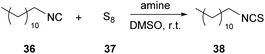
|
Entry |
Amine |
Base equivalents |
Reaction time (h) |
Conversiona (%) |
All reactions were carried out with 36 (1.0 equiv.), 37 (2.00 equiv. of sulfur atoms), a respective amount of amine, DMSO (1 mL), r.t. GC samples were taken after the respective time and conversions were calculated using biphenyl (0.25 equiv.) as an internal standard. Reaction was performed using Cyrene as the solvent, at 40 °C. |
1 |
DMAP |
1.00 |
2 |
38 |
2 |
NMI |
1.00 |
2 |
38 |
3 |
DBU |
1.00 |
2 |
99 |
4 |
DABCO |
1.00 |
2 |
84 |
5 |
TEA |
1.00 |
2 |
76 |
6 |
TBD |
1.00 |
2 |
100 |
7 |
DBU |
0.10 |
2 |
57 |
8 |
DBU |
0.10 |
22 |
67 |
9 |
TBD |
0.10 |
2 |
36 |
10 |
TBD |
0.10 |
22 |
69 |
11b |
DBU |
2 mol% |
4 |
100 |
12b |
DBU |
5 mol% |
4 |
100 |
Finally, numerous isothiocyanates were synthesized to assess the scope of the developed method (Scheme 9).30 Every isocyanide tested was successfully synthesized, with steric hindrance not playing a significant role. Electron-withdrawing and -donating groups, when aromatic and benzyl isothiocyanates were synthesized, did not affect differently the reaction outcome. The only substrate that presented problems and did not react was a carboxylic acid salt, perhaps because of solubility difficulties.
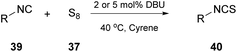 |
| Scheme 9 General reaction process for the synthesis of isothiocyanates. | |
Amide and peptide synthesis
HATU mediated amide coupling.
Synthesis of amides is becoming more and more popular, since the number of peptides, approved for drug use, is increasing rapidly. Usually for amide synthesis, coupling reagents, such as N-[(dimethylamino)-1H-1,2,3-triazolo-[4,5-b]pyridine-1-ylmethylene]-N-methyl-methanaminium hexafluorophosphate N-oxide (HATU) or (1-cyano-2-ethoxy-2-oxoethylidenaminooxy)dimethylamino-morpholino-carbenium hexafluorophosphate (COMU) are employed using dipolar aprotic solvents, such as DMF and NMP. As it is already established, Cyrene is a good alternative to these solvents, thus in 2018 Watson and coworkers, following their findings, examined Cyrene's use for amide coupling via HATU coupling, obtaining similar results.31 Initially, they studied the reaction between p-toluic acid (41) and aniline (42) in the presence of HATU as the coupling agent (Table 5).31 The reaction was completed in just one hour; however, some inconsistencies were observed. Due to Cyrene's high viscosity, the stirring rate plays an important role in the reaction outcome. Among all the tested bases, DIPEA was found to be the suitable base for the amide coupling reaction. On increasing the amount of the base, they observed that the stirring factor did not play such an important role.
Table 5 Reaction optimization for the amide coupling in Cyrene
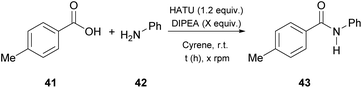
|
Entry |
DIPEA equiv. |
Reaction time (h) |
Stirring rate |
Conversiona (%) |
All reactions were carried out with 41 (0.25 mmol), 42 (0.275 mmol), and HATU (0.30 mmol). (%) conversion was calculated by 1H-NMR using 1,4-dinitrobenzene as an internal standard. DMF was used as the solvent. |
1 |
2 |
24 |
Variable |
87 |
2 |
2 |
1 |
Variable |
84 |
3 |
2 |
1 |
200 |
73 |
4 |
2 |
1 |
400 |
71 |
5 |
2 |
1 |
800 |
96 |
6 |
3 |
1 |
400 |
92 |
7b |
3 |
1 |
400 |
100 |
Having the best conditions in hand, they proceeded by expanding the scope of carboxylic acids and amines used.31 Aryl and alkyl carboxylic acids reacted with primary and secondary amines affording great to excellent results. The reaction protocol was successfully implemented in the synthesis of a variety of dipeptides in high yields, using N-protected amino acids and a series of amino esters bearing side chains with alkyl or aryl substituents. In certain occasions, increase of the amount of the HATU reagent was needed; however, the results were very promising. The amidation protocol was successfully applied in a larger scale and for the solid-phase synthesis of smaller peptides.
Solid-phase peptide synthesis
Using a similar concept, in 2019, Cabri and coworkers attempted the solid-phase peptide synthesis (SPPS), which is a faster synthetic approach for larger peptides (Scheme 10).32 In this approach, DMF is mostly used, and there have been many approaches for the replacement of DMF, due to its toxicity. In SPPS, generally the solvent is involved in the inflation of the resins used for the synthesis, the washing, the deprotection and the coupling. As it was difficult to find a solvent quite efficient for all these steps, a combination of solvents was introduced, to achieve the maximum efficiency. Hence in this study, Cyrene was combined with dimethyl or diethyl carbonate. Other solvent mixtures were also examined.32 The evaluation of the solvent mixture was performed by synthesizing a model peptide (Aib-Enkephalin). When the optimum solvent system was found, a more challenging sequence was synthesized with this protocol. Initially, resin swelling was assessed.32 Resin swelling is obligatory for the solid-phase synthesis for the reactive functionalities to be accessible. When the resin swelling is not well achieved, reactive functionalities are blocked in the polymer matrix and are unable to react.33 As far as PS-Wang resin is concerned, a lower percentage of Cyrene gave better swelling results; however, for other resins, a higher percentage of Cyrene was more efficient.32 The most efficient Cyrene mixture was the one with diethyl carbonate in a 30
:
70 ratio for the PS-Wang-OH (44) resin, which gave a better swelling outcome than DMF. They continued by evaluating the solubility of Fmoc-protected amino acids, more specifically Fmoc-Val-OH was used as the model amino acid (Scheme 10). Using a Cyrene mixture, the model amino acid was not dissolved very well, hence the solubility was increased by adding a combination of coupling reagents. Among all, DIC/Oxyma was the most efficient. With these conditions in hand, several amino acids were tested. The authors also examined other green solvent binary mixtures, which were found in many cases to be more efficient than the Cyrene mixture. Overall, Cyrene was more efficient than the often used solvents for peptide synthesis and this work is proof that Cyrene may be a valuable green alternative for solid peptide synthesis.
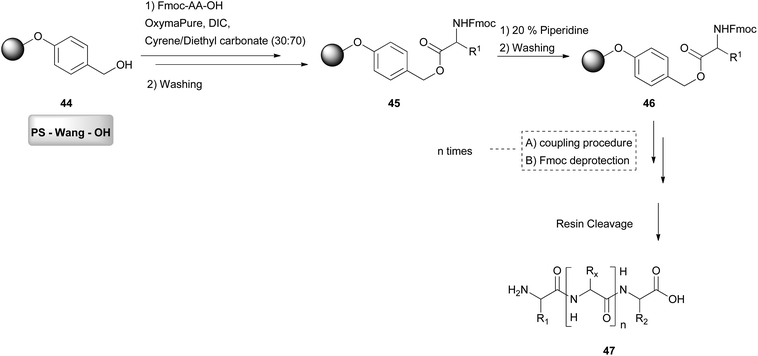 |
| Scheme 10 Solid-phase peptide synthesis in Cyrene. | |
Amide synthesis
In 2019, Camp and coworkers were also interested in amide synthesis, altering the coupling reaction. In that study, the authors examined the reaction of acid chlorides with amines (Table 6).34 Various solvents can be used for this reaction, namely DMF, dichloromethane or tetrahydrofuran, nonetheless they opted for Cyrene as a more sustainable solvent, and employed 4-fluorobenzoyl chloride (48) to react with pyrrolidine (34), aniline (42) or benzylamine (49), in the presence of triethylamine as the base (Table 6).34 Their investigations began by assessing different work-up and purification methods. The addition of water and an aqueous work-up was sufficient, when anilines, primary amines or benzylamine were employed, yet in some cases further purification with column chromatography was needed. In addition to their observation in urea synthesis,29 addition of water did not result in product precipitation and the produced amides formed an emulsion, when pyrrolidine was used.34
Table 6 Optimization of the synthesis of amides 50 in Cyrene
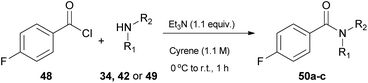
|
Entry |
Amine |
Work-up |
Yield (%) |
All reactions were carried out with 48 (1.0 equiv.), 34 or 42 or 49 (1.0 equiv.), triethylamine (1.1 equiv.), Cyrene (0.5 mL), 0 °C to r.t., 1 h. |
1 |
Pyrrolidine (34) |
Aqueous; then column |
91 (50a) |
2 |
Pyrrolidine (34) |
Column |
75 (50a) |
3 |
Aniline (42) |
Precipitate |
72 (50b) |
4 |
Benzylamine (49) |
Precipitate |
81 (50c) |
Switching to primary aliphatic or benzylic amines, the desired amides could precipitate. It was also observed that without the aqueous work-up, lower yields were obtained by column chromatography. The authors examined the scope of the reaction using a variety of acyl chlorides in the reaction with pyrrolidine (34), aniline (42) or benzylamine (49).34 Moderate yields were obtained when heterocyclic benzoyl chlorides were used and benzylamine afforded better results in comparison to the other amines employed. Moderate yields were also obtained from aliphatic acid chlorides. In their attempt to investigate the behavior of Cyrene in the presence of water, the authors investigated the hydration of Cyrene (Scheme 11). Cyrene reacts with D2O forming geminal diol 51, so they examined the effect of water percentage on the hydration of the ketone. Indeed, in a 1
:
1 ratio, the hydration of Cyrene is favored. When an organic solvent was also present, the keto form of Cyrene was favored instead. It is pointed out that the control of the hydration process of Cyrene facilitates its recycle. Furthermore, the authors reported that the use of Cyrene along with water facilitates the precipitation of the desired products more efficiently, compared to the precipitation protocol using DMF. To sum up, Cyrene seems to be a plausible solvent for peptide chemistry, but further research ought to be done, considering the synthesis of larger peptides and their purification.35
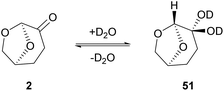 |
| Scheme 11 Production of germinal diol 51 from Cyrene. | |
Substitution reactions
The first attempts that were carried out to assess the effectiveness of Cyrene as the solvent mainly involved substitution reactions. As was previously mentioned, in 2014, Sherwood and coworkers employed Cyrene in two well-known and important pharmaceutical industry reactions, the Menschutkin reaction and a fluorination reaction.4
Menschutkin reaction
As far as the Menschutkin reaction is concerned, it is a SN2 type substitution, which involves two neutral reactants, leading to a salt. It is widely used for the preparation of imidazolium containing ionic liquids. Usually, the reaction is performed in polar solvents, such as DMF, so the performance of Cyrene could be a safe indicator of its ability to substitute toxic polar solvents. Cyrene afforded better results than widely used polar aprotic solvents, such as DMF, NMP and dioxane. Only DMSO slightly outperformed Cyrene (Scheme 12).4
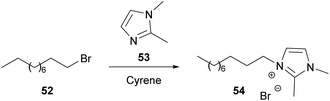 |
| Scheme 12 Menschutkin reaction between 52 and 53 using Cyrene as the solvent. | |
Fluorination reaction
A widely used substitution reaction with great pharmaceutical interest is the nucleophilic fluoride substitution. For the fluorination reaction, the authors assessed the efficacy of Cyrene in a nucleophilic aromatic substitution SNAr in 2-chloro-5-nitropyridine (55), to afford 2-fluoro-5-nitropyridine (56) (Scheme 13).4 Nucleophilic substitutions proceed via the formation of a Meisenheimer intermediate, which can be stabilized by the employed solvent. Sufficient stabilization of the intermediate succors in the augmentation of the reaction rate. Cyclohexanone, Cyrene and NMP performed similarly, but unfortunately not as well as other polar solvents, like DMSO, DMF or sulpholane. However, their performance did not lead to major losses, making Cyrene a plausible greener alternative.4
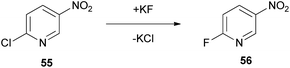 |
| Scheme 13 Fluorination reaction of 55 to afford 56. | |
Baylis–Hillman reaction
In 2020, Hunt and coworkers assessed various alternatives to dipolar aprotic solvents.36 One of the reactions studied in this process was the Baylis–Hillman reaction between 4-nitrobenzaldehyde and methyl acrylate (Scheme 14). A wide variety of solvents was tested, with great results, however, taking their toxicity under consideration. NMP, sulfolane and Cyrene led to reaction completion. However, NMP and sulfolane are reprotoxic.7a Thus, Cyrene was the most successful alternative, with both great yields and low toxicity.36 In that study, the authors examined a variety of potential green solvents in several coupling reactions. The rate of the examined reactions varied and depended on the solvent used. They mainly focused in the Baylis–Hillman coupling reaction, where great results afforded all the different substrates that were tested. Furthermore, the reaction conditions were successfully implemented in a gram scale and the results were comparable to the lab scale, proving that Cyrene is a plausible solvent for the Baylis–Hillman reaction for industrial use as well.
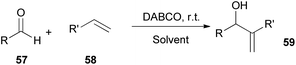 |
| Scheme 14 Baylis–Hillman reaction between 57 and 58. | |
Cyrene as a catalyst–solvent
In 2022, Yu and coworkers proposed a protocol for the conversion of amines to formamides 63, using CO2 and PhSiH as the reductant.37 They also successfully applied this protocol in the synthesis of benzothiazoles 65 from aminothiophenols 64 (Scheme 15).37 Cyrene presented excellent results playing simultaneously the role of the solvent and the catalyst, perhaps due to its polarity. Moreover, NMR mechanistic studies were carried out, indicating that Cyrene polarizes the N–C bond, making nitrogen a better nucleophile, thus enabling the reaction. Additionally, it was found that it also activates the Si–H bond via the polarization effect, enabling the insertion of the CO2. The authors continued their study by examining the substrate scope realizing that electron-rich substituted anilines afforded higher yields, since the electron-rich benzene ring increases the nucleophilicity of the nitrogen atom, making the nucleophilic attack on silanes more favorable.37
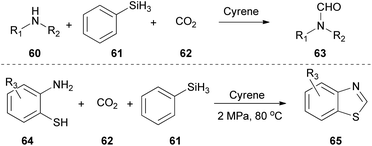 |
| Scheme 15 Formation of formamides (63) or benzothiazoles (65). | |
Construction of diverse bipyridine analogues
Bipyridine analogues constitute one of the most important classes of molecules found in many natural products and materials. In 2021, Lee and coworkers suggested the formation of bipyridine derivatives through a multicomponent reaction under microwave irradiation (Table 7).38 Towards this aim, they initially used aniline (42), 3-formylchromone (66) and 2-pyridineacetonitrile (67) under catalyst-free conditions. The use of Cyrene as the solvent enabled the researchers to attain an even higher yield than what was previously documented using DMF, as the temperature could be elevated, without further problems. Under the elevated temperature conditions (150 °C), an impressive yield of 94% was obtained (entry 7, Table 7).38 It is interesting that the authors did not observe any by-product derived from Cyrene. It was also reported that Cyrene's recovery was easily succeeded from the reaction mixture, just by removing water in vacuo. After removing water, Cyrene was available for further use, without additional purification.
Table 7 Optimization of the reaction leading to 68
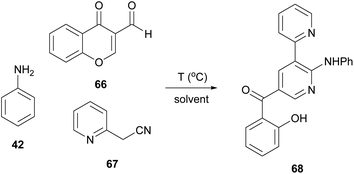
|
Entry |
Solvent |
Temperature (°C) |
Reaction time (h) |
Yielda (%) |
All reactions were carried out with 42 (1.00 mmol), 66 (1.00 mmol) and 67 (1.00 mmol) under 300 W temperature-controlled microwave irradiation. Yield of the isolated product. Reaction under traditional heating. |
1 |
— |
90 |
2 |
40 |
2 |
H2O |
90 |
1 |
— |
3 |
EtOH |
90 |
1 |
48 |
4 |
DMF |
90 |
1 |
66 |
5 |
Cyrene |
90 |
1 |
76 |
6 |
Cyrene |
110 |
1 |
83 |
7 |
Cyrene |
130 |
1 |
94 |
8 |
Cyrene |
150 |
1 |
91 |
9b |
Cyrene |
130 |
24 |
35 |
10 |
DMF |
130 |
1 |
85 |
11 |
— |
130 |
1 |
32 |
To explore in which extend this reaction could be efficient, a great variety of aromatic amines was tested.38 Excellent yields were obtained using ortho-, meta- or para-substituted anilines bearing either electron-withdrawing or electron-donating substituents. Excellent results were also afforded using aliphatic and cyclic amines irrespective of whether they were primary, secondary, or even heteroaromatic.
The authors explored the reaction of a variety of 3-formylchromones with aniline (42) or 2-aminopyridine and 2-pyridylacetonitrile (67). The presence of an electron-donating or electron-withdrawing group afforded the desired product in excellent yields (83–96%). Furthermore, combination of an electron-donating and an electron-withdrawing group on the chromone core did not alter the reaction outcome. Only in the case when two electron-deficient groups were present on the chromone moiety, the yield of the reaction was slightly lower. Expanding the scope of the protocol, the authors employed 3-(pyridinyl)acetonitrile and 4-(pyridine)acetonitrile, leading to the desired dipyridine analogue in good yields (72% and 64%, respectively).38 Adhering to this logic, the authors introduced other cyano-containing molecules, such as benzyl cyanides, instead of the pyridinyl acetonitriles. Excellent results were also afforded in the presence of electron-deficient groups on the aromatic ring, whereas benzyl cyanide, as well as 4-methyl derivative, did not afford the desired product. Finally, the reaction products were found to bind with some heavy metals, like Hg2+, Fe3+ or Cu2+, which makes them useful for various applications, such as catalysis and pollution monitoring (Scheme 16).
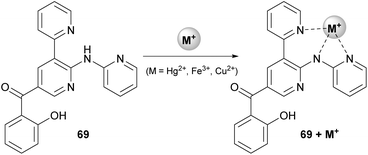 |
| Scheme 16 The proposed structure of 69 when it binds with a heavy metal atom. | |
Biocatalysis
Bioreduction of α-ketoesters to α-hydroxyesters.
In 2020, De Gonzalo suggested the use of Cyrene as a bio-based solvent, since water that has already been studied, led to unwanted side reactions, such as hydrolysis of the reactants.39 The authors indicated that Cyrene as the bio-based solvent for biocatalysis can replace other solvents, such as 2-Me-THF and cyclopentyl methyl ether (CPME). It was the first time that the use of Cyrene was studied for a reductive process, using alcohol dehydrogenases (ADHs). The authors introduced the bioreduction of α-ketoesters to α-hydroxyesters with the use of Cyrene (Scheme 17).39 Initially, ethyl benzoylformate was used as a model ketoester and various ADHs were used as biocatalysts (Scheme 17). Additionally, two nicotinamide co-factors or glucose dehydrogenase, coupled with glucose, were employed as recycling systems. With every combination used, the desired product was formed with excellent conversion and enantiomeric excess. It was observed that Cyrene's keto group remained intact in the presence of the reductive agents, since no reduction products were attained. However, with the use of some biocatalysts, Cyrene's concentration seemed to have a significant impact on the conversion and the optical purity. Another condition that seemed to play an important role was the substrate concentration. Generally, when the percentage of Cyrene in the reaction medium was quite low (2.5% v/v), both stereoisomers could be obtained, depending on the ADH that was employed.39 In addition, when alcohol dehydrogenase KRED P2-D03 was used, a larger percentage of Cyrene could be tolerated (30% v/v), without negatively influencing the reaction outcome.
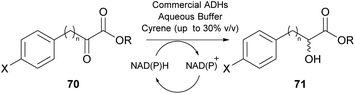 |
| Scheme 17 Optimized bioreduction of α-ketoesters (70) to 71 in Cyrene. | |
Biocatalytic esterification
As was previously noted, in 2016 and 2018, researchers attempted the use of Cyrene as the solvent in biocatalytic esterifications, but none of these efforts were successful.21 However, in 2020, Guajardo and coworkers successfully accomplished the esterification of benzoic acid 72 with glycerol 73, using lipase Novozym 435 and crosslinked aggregates of CAL-B (Scheme 18).40 The authors indicated that the in situ derivatization of Cyrene can afford a novel family of green solvents with enhanced properties. Initially, the authors tested the solubility of the reagents at different concentrations, where Cyrene demonstrated excellent solubility, like dioxane. As expected, since Cyrene does not perform well under acidic conditions, more equivalents of glycerol were added, to decrease the acidic conditions induced by the presence of benzoic acid 72. Not only the reaction occurred in both occasions, but in the case of CAL-B, the catalyst could be recycled up to six times using phosphate buffer of pH 7, albeit higher biocatalyst loadings were recommended and higher temperatures were required (60 °C).40 Furthermore, the authors examined Cyrene as the co-solvent for enzymatic hydrolysis, using the same enzymes as for the esterification. Cyrene was tested in various proportions to water (0–40%). The authors demonstrated that increasing the proportion of Cyrene led to the deactivation of the biocatalyst. This can probably be attributed also to the fact that upon decreasing the water proportion, the reaction equilibrium tends to the formation of ester 74. Even though Cyrene's performance is poor compared to other systems reported in the literature, such as deep eutectic solvents (DES), further optimization might provide new opportunities for its use in biocatalysis.
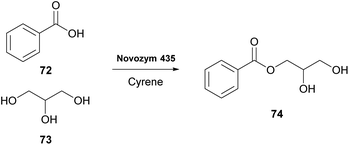 |
| Scheme 18 Lipase-catalyzed esterification of benzoic acid (72) with glycerol (73) using Cyrene as the reaction medium. | |
Materials chemistry
MOF synthesis.
In 2016, Katz and coworkers exploited Cyrene for the synthesis of metal organic frameworks (MOFs).41 MOFs are often synthesized in toxic organic solvents, such as DMF, due to their high boiling points, because MOF synthesis requires elevated temperatures. Firstly, the authors synthesized MOF HKUST-1 using a mixture of Cyrene and EtOH as the solvent system and successfully led to the desired crystalline product. The authors observed different reaction outcomes in the case where Cyrene was used instead of DMF. More specifically, different orientations were obtained and larger particles were formed when Cyrene was employed.41 Afterwards, they proceeded by evaluating the N2 gas-absorption isotherm of HKUST-1. Comparing the surface area (SA) of the MOF synthesized with the use of DMF, the BET SA was about 1400 m2 g−1 (Table 8). When a mixture of Cyrene and EtOH mediated the reaction, the SA was determined to be 600 m2 g−1. To explain the difference in the N2 gas-absorption isotherm, the authors examined various ratios of Cyrene/EtOH. It was observed that when dry ethanol was used as the additive with Cyrene, the BET SA was 1400 m2 g−1. The BET SA of the MOF decreased when the ethanol used was not absolute. This was attributed to the fact that in the presence of water, Cyrene might react to produce the geminal diol and other products (Scheme 11). Thus, the use of dry EtOH was obligatory. Furthermore, the authors pointed out the necessity of keeping the water content of Cyrene low. Next, the synthesis of other MOFs using Cyrene was investigated. Four more MOFs were synthesized: UiO-66, ZIF-8, MOF-74 and Zn2(BDC)2(DABCO) (Table 8). Both DMF and Cyrene gave the same products in all four cases, with some differences in the orientations. The authors further investigated the lower SA that was observed with the use of Cyrene, but this time they did the investigations with ZIF-8. Although PXRD showed different peak intensities, the peaks overall did not differ. Fortunately, examination using a microscope revealed the presence of an aldol condensation product of two Cyrene molecules (Scheme 3B) among the crystalline powder of ZIF-8, which could be avoided by decreasing the heating. Finally, it was observed that there is always residual Cyrene especially in small-pore aperture MOFs, so further washing and activation routines might be needed, but were not suggested.
Table 8 Obtained BET SA for MOFs
Entry |
MOFs |
Expected (m2 g−1) |
Observed in DMF (m2 g−1) |
Observed in Cyrene (m2 g−1) |
1 |
HKUST-1 |
1740 |
1400 |
1500 |
2 |
UiO-66 |
1700 |
1300 |
500 |
3 |
Co-MOF-74 |
1572 |
800 |
200 |
4 |
ZIF-8 |
1950 |
1700 |
600 |
5 |
Zn2(BDC)2(DABCO) |
1750 |
1950 |
1300 |
Cyrene for the dispersion of graphene
The augmentation in ink demand makes its production more systematic. However, most solvents used for the graphene ink process are toxic and not environmentally friendly. Thus, their industrial use is not favored, and new solutions must be found to overcome this problem. In 2017, Salavagione and coworkers investigated the use of several green solvents for graphene exfoliation, including Cyrene.42 Having in mind that an essential factor for graphene dispersion is the solvent surface energy and that the concentration might influence the solvent polarity, they conducted a solvent screening to determine which solvents could be efficient. Cyrene was used for the dispersion of graphene while applying a 15-minute ultrasound treatment. A high concentration of dispersed graphene was achieved, perhaps due to the greater viscosity of Cyrene, in comparison to other solvents previously employed. As far as the quality of the dispersed graphene is concerned, the flakes obtained presented some diversity in their thickness and dimensions. In conclusion, the Cyrene-based process generated better quality graphene than regularly used solvents.
In 2018, Pan and coworkers also studied the use of Cyrene for the dispersion of graphene.43 More specifically, they compared Cyrene with N-methylpyrrolidone (NMP). NMP is a very toxic dipolar aprotic organic solvent whose use is suggested to be reduced, due to its hazardous effects.7 Firstly, conductivity was evaluated. For the exfoliation of ink, they also used a sonication treatment. It was observed that the sheet resistance of the laminate made with Cyrene decreased faster than the one made with DMF. By increasing the sonication time, the graphite flakes become smaller, so the conductivity decreases, and the sheet resistance rises again. Cyrene was found to be more effective, since it required less time than NMP to achieve the same results of conductivity.43 Next, the addition of adhesive materials was examined, which generally decreases the conductivity. In this case cellulose acetate butyrate (CAB) was added into the Cyrene-derived graphene ink and different concentrations of CAB were tested. Also, the graphite flakes were characterized and evaluated by atomic force microscopy (AFM) and the stable existence of few-layer graphene nanoflakes was confirmed.43 For the evaluation, FT-IR spectroscopy and Raman spectroscopy were also used. In conclusion, graphene/CAB ink was developed by exfoliation and dispersion of graphene sheets in Cyrene, achieving great conductivity with and without the addition of CAB.
ROM polymerization
In 2021, the polymerization of levoglucosenyl alkyl ethers 75 was investigated using various green solvents, including Cyrene.44 The specific polymerization was mostly succeeded by employing a Grubb's catalyst (C793) (Scheme 19). In that work, C793 was used as well and the ROM polymerization of the levoglucosenyl alkyl ethers afforded amorphous polyacetals with thermoplastic behavior, which were easily degradable by hydrolysis.44 The degrees of polymerization fluctuated around 100 and molar mass distributions were broad with a dispersity of around 2. The products obtained by this polymerization are plausible plastic materials and more importantly, they are sustainably synthesized.
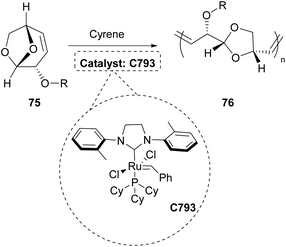 |
| Scheme 19 Cyrene in the polymerization of levoglucosenyl alkyl ethers 75. | |
Cyrene for the preparation of membranes
In 2019, Figoli and coworkers proposed the use of Cyrene for membrane preparation.45 More specifically, the use of polyethersulfone (PES) and poly(vinylidene fluoride) (PVDF) to produce membranes was studied. For these procedures, toxic organic solvents are often used, such as N-methyl-pyrrolidone (NMP) or N,N-dimethyl acetamide (DMAc). It was observed that the moisture, the viscosity of the solution and the interactions between the polymer and the solvent played an important role. Moreover, several factors were assessed to draw a conclusion. The factors evaluated were the membrane morphology, the pore diameter, their hydrophilic character, thermal behavior, and crystallinity degree. Overall, Cyrene proved to be a new choice for sustainable membrane fabrication.46
Another group approached the membrane synthesis using Cyrene. In 2021, Pellis and coworkers studied membrane fabrication with Cyrene using four polymers: cellulose acetate (CA), polysulfone (PSf), polyethersulfone (PES) and polyimide (PI).47 Cyrene and Cygnet (77), a Cyrene derivative, were used (Scheme 20). Intriguingly, their mixture seemed to be more efficient as the solvent for the PES polymer. The morphologies of the membranes prepared were highly dependent on the ratio of the solvent used. Furthermore, the authors reported the important role temperature played in membrane synthesis.47 In conclusion, the ability to determine the morphologies of the membranes formed by these polymers was shown. By just altering the temperature and the Cygnet–Cyrene analogy, different membranes could be formed with various properties and numerous applications.
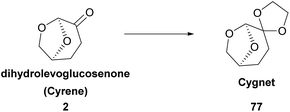 |
| Scheme 20 Cyrene 2 and Cygnet 77. | |
In 2022, Paugam and coworkers developed a more sustainable method for the fabrication of polymeric membranes by a phase-inversion technique.48 As the polymeric matrix, the authors employed poly(hydroxybutyrate-cohydroxyvalerate) (PHBHV), which is a biopolymer and belongs to a family of polymers known for their biocompatible properties as microbial polymers, PHAs. The produced membranes were permeable to water and they were asymmetric. Various membranes were produced, with or without additives. All of them due to their hydrophilicity presented some selectivity towards MeOH, which depended on the type of each membrane. Moreover, all of them performed well when stability tests in demanding environments were conducted.48
Cyrene in the field of antimicrobial susceptibility testing
DMSO is one of the most common solvents used as the vehicle in antimicrobial susceptibility testing. A tendency to find greener alternatives led Camp and coworkers, in 2020, to assess the efficiency of Cyrene as an alternative to DMSO.49 Initially, the toxicity of Cyrene and DMSO towards bacteria was compared and found to be quite similar. Next, they were compared as vehicles for standard antibacterial drugs in minimum inhibitory concentration (MIC) tests and a drug discovery screening protocol was used. Cyrene was found to be equally effective. They proceeded by testing the activity of antibacterial drugs in both solvents and concluded that the results were within the experimental setup, although there were differences between the two solvents.49 Additionally, their ability to preserve long-term dissolved antibacterial drugs was assessed and the results were satisfactory. Finally, dissimilar to DMSO, which protects ESKAPE pathogens from ROS-mediated cell death, induced by stress, Cyrene does not protect them from dying. In conclusion, they confirmed that Cyrene could be a noteworthy substitute for DMSO in antimicrobial susceptibility testing.
Lignins
In the past decade many researchers have worldwide focused their research on developing new processes to isolate lignin. To obtain better properties, chemical modifications are required which are impossible without their solubilization. However, their solubility presents difficulties, and a small number of solvents can overcome them and solubilize lignins. Mostly these solvents are reprotoxic and hazardous for the environment. In 2021, Averous and coworkers examined Cyrene's efficiency as a lignin solvent for lignins Kraft (KL), soda (SL) and organosolv (OSL).50 Also, their efficacy as solvents for the fractionalization of Kraft lignin (KL) and for the chemical modifications of lignins was tested. Firstly, the solubility of lignins in Cyrene was assessed. None of the lignins tested were fully soluble in Cyrene, whilst with the addition of water, their solubility increased significantly. This can be explained by the formation of the germinal diol and the increase of hydrogen bonding, resulting in better lignin solubilisation. Moreover, the fractional precipitation of KL was tested again when divided into smaller fractions and the solvent couple Cyrene–water worked effectively. Finally, Cyrene was tested in esterification and urethane formation reactions of lignins and even if compared to DMF, it presented some difficulties, the results were positive. To conclude, Cyrene can be a potential biobased solvent for lignin, although it presents some difficulties.50
4. Conclusions
In conclusion, Cyrene is becoming a more and more popular green, non-mutagenic and non-toxic reaction medium, due to its compatibility as a solvent with plenty of reactions of great significance for the chemical industry. Even though Cyrene might present some difficulties in its use, due to its chemical properties, Cyrene's safe use and easy disposal outweigh many popular organic but toxic solvents. The last decade has created numerous opportunities, where Cyrene was successfully employed as a green and safe alternative to toxic solvents. Indeed, more surveys are coming to light day by day, where Cyrene can be employed in every-day and traditional reactions in organic chemistry, biocatalysis, materials chemistry, graphene and lignin manipulation. Initial studies showed the enormous potential Cyrene has. We believe that further research would solve the existing difficulties and give new data to develop significant applications of this promising solvent. Building on easy removal and recycling, Cyrene can become the future number one choice as a green solvent. The future hopefully will reveal new and powerful transformations and hopefully new reactivities will arise.
Author contributions
N. A. S. and P. L. G. prepared the draft of the manuscript and C. G. K. performed the final editing. The manuscript was written through the contributions of all authors.
Conflicts of interest
There are no conflicts to declare.
Acknowledgements
The authors gratefully acknowledge the Hellenic Foundation for Research and Innovation (HFRI) for financial support through a grant, which is financed by 1st Call for H.F.R.I. Research Projects to Support Faculty Members & Researchers and the procurement of a high-cost research equipment grant (grant number 655).
Notes and references
-
(a) L. Wu, T. Moteki, A. A. Gokhale, D. W. Flaherty and F. D. Toste, Chem. Biomol. Eng., 2016, 1, 32–58 CAS;
(b) Y. Sarrafi, M. Sadatshahabi, K. Alimohammadi and M. Tajbakhsh, Green Chem., 2011, 13, 2851–2858 RSC.
-
P. T. Anastas and J. C. Warner, Green Chemistry: Theory and Practice, Oxford University Press, New York, 1988 Search PubMed.
-
V. L. Budarin, K. J. Milkowski, P. Shuttleworth, B. Lanigan, J. H. Clark, D. J. Macquarrie and A. Wilson, US Pat, US20110219679A1, 2011 Search PubMed.
- J. Sherwood, M. De Bruyn, A. Constantinou, L. Moity, C. R. McElroy, T. J. Farmer, T. Duncan, W. Raverty, A. J. Hunt and J. H. Clark, Chem. Commun., 2014, 50, 9650–9652 RSC.
- J. E. Camp, ChemSusChem, 2018, 11, 3048–3055 CrossRef CAS PubMed.
- F. Gao, R. Bai, F. Ferlin, L. Vaccaro, M. Li and Y. Gu, Green Chem., 2020, 22, 6240–6257 RSC.
-
(a) F. P. Byrne, S. Jin, G. Paggiola, T. H. M. Petchey, J. H. Clark, T. J. Farmer, A. J. Hunt, C. R. McElroy and J. Sherwood, Sustainable Chem. Processes, 2016, 4, 1–24 CrossRef;
(b) J. Sherwood, T. J. Farmer and J. H. Clark, Chem, 2018, 4, 2004–2012 CrossRef;
(c) S. Kar, H. Sanderson, K. Roy, E. Benfenati and J. Leszczynski, Chem. Rev., 2022, 122, 3637–3710 CrossRef PubMed.
-
(a) Y. Halpern, R. Riffer and A. Broido, J. Org. Chem., 1973, 38, 204–209 CrossRef CAS;
(b) F. Shafizadeh and P. P. S. Chin, Carbohydr. Res., 1977, 58, 79–87 CrossRef CAS;
(c) F. Shafizadeh, R. H. Furneaux and T. T. Stevenson, Carbohydr. Res., 1979, 51, 169–191 CrossRef;
(d) L. K. M. Lam, J. Appl. Polym. Sci., 1973, 17, 391–399 CrossRef CAS.
-
G. R. Court, C. H. Lawrence, W. D. Raverty and A. J. Duncan, US Pat, 2012/0111714, 2012 Search PubMed.
- S. Krishna, D. J. McCelland, Q. A. Rashke, J. A. Dumesic and G. W. Huber, Green Chem., 2017, 19, 1278–1285 RSC.
-
(a) S. Kudo, N. Goto, J. Sperry, K. Norinaga and J. Hayashi, ACS Sustainable Chem. Eng., 2017, 5, 1132–1140 CrossRef CAS;
(b) J. Mazario, M. P. Romero, P. Concepcion, M. Chavez-Sifontes, R. Spanevello, M. B. Comba, A. G. Suarez and M. E. Domine, Green Chem., 2019, 21, 4769–4785 RSC.
- Z. Wang, Q. Lu, X. F. Zhu and Y. Zhang, ChemSusChem, 2011, 4, 79–84 CrossRef CAS PubMed.
- L. M. M. Mouterde, F. Allais and J. D. Stewart, Green Chem., 2018, 20, 5528–5532 RSC.
- A. Alhifthi, B. L. Harris, L. Goerigk, J. M. White and S. J. Williams, Org. Biomol. Chem., 2017, 15, 10105–10115 RSC.
- L. Hughes, C. R. McElroy, A. C. Whitwood and A. J. Hunt, Green Chem., 2018, 20, 4423–4427 RSC.
- G. Bonneau, A. M. Peru, A. L. Flourat and F. Allais, Green Chem., 2018, 20, 2455–2458 RSC.
- M. De Bruyn, C. Sener, D. D. Petrolini, D. J. McClelland, J. He, M. R. Ball, Y. Liu, L. Martins, J. A. Dumesic, G. W. Huber and B. Weckhuysen, Green Chem., 2019, 21, 5000–5007 RSC.
-
(a) J. Klepp, C. J. Sumby and B. W. Greatrex, Synlett, 2018, 29, 1441–1446 CrossRef CAS;
(b) J. Klepp, H. Podversnik, J. Pusching, A. Wallace and B. W. Greatex, Tetrahedron Lett., 2019, 75, 3894–3903 CrossRef CAS.
-
(a) Z. J. Witczak, R. Bielski and D. E. Mencer, Tetrahedron Lett., 2017, 58, 4069–4072 CrossRef CAS;
(b) R. E. Hohol, H. Arcure, Z. J. Witczak, R. Bileski, K. Kirschbaum, P. Andreana and D. E. Mencer, Tetrahedron Lett., 2018, 74, 7303–7309 CrossRef CAS.
- P. Ray, T. Hughes, C. Smith, M. Hibbert, K. Saito and G. P. Simon, Polym. Chem., 2019, 10, 3334–3341 RSC.
- A. Iemhoff, J. Sherwood, C. R. McElroy and A. J. Hunt, Green Chem., 2018, 20, 136–140 RSC.
- A. G. Lanctot, T. M. Attard, J. Sherwood, C. R. McElroy and A. J. Hunt, RSC Adv., 2016, 6, 48753–49756 RSC.
- H. A. Le Phuong, L. Cserti, G. F. S. Whitehead, A. Garforth, P. Budd and G. Szekely, RSC Adv., 2017, 7, 53278–53289 RSC.
- K. L. Wilson, A. R. Kennedy, J. Murray, B. Greatex, C. Jamieson and A. J. B. Watson, Beilstein J. Org. Chem., 2016, 12, 2005–2011 CrossRef CAS PubMed.
- K. L. Wilson, J. Murray, C. Jamieson and A. J. B. Watson, Synlett, 2018, 650–654 CAS.
- A. S. Jaufurally, A. R. S. Teixeira, L. Hollande, F. Allais and P. H. Ducrot, ChemistrySelect, 2016, 1, 5165–5171 CrossRef CAS.
- M. M. Mention, A. L. Flourat, C. Peyrot and F. Allais, Green Chem., 2020, 22, 2077–2085 RSC.
- U. Veerabagu, G. Jaikumar, F. Lu and F. Quero, React. Chem. Eng., 2021, 6, 1900–1910 RSC.
- L. Mistry, K. Mapesa, T. W. Bousfield and J. E. Camp, Green Chem., 2017, 19, 2123–2128 RSC.
- R. Nickisch, P. Conen, S. M. Gabrielsen and M. A. R. Meier, RSC Adv., 2021, 11, 3134–3142 RSC.
- K. L. Wilson, J. Murray, C. Jamieson and A. J. B. Watson, Org. Biomol. Chem., 2018, 16, 2851–2854 RSC.
- L. Ferrazzano, D. Corbisiero, G. Martelli, A. Tolomelli, A. Viola, A. Ricci and W. Cabri, ACS Sustainable Chem. Eng., 2019, 7(13), 11036–11043 CrossRef.
- S. Lawrenson, M. North, F. Peigneguy and A. Routledge, Green Chem., 2017, 19, 952–962 RSC.
- T. W. Bousfield, K. P. R. Pearce, S. B. Nyamini, A. A. Dimakis and J. E. Camp, Green Chem., 2019, 21, 3675–3681 RSC.
- D. Procopio, C. Siciliano, S. Trombino, D. E. Dumitrescu, F. Suciu and M. L. Di Gioia, Org. Biomol. Chem., 2022, 20, 1137–1149 RSC.
- S. Sangon, N. Supanchaiyamat, J. Sherwood, C. R. McElroy and A. J. Hunt, React. Chem. Eng., 2020, 5, 1798–1804 RSC.
- H. Yu, D. Yu, Z. Xue, B. Zhang and T. Mu, Chem. Eng. J., 2022, 431, 13397–133406 Search PubMed.
- R. J. I. Tamargo, P. Y. M. Rubio, S. Mohandoss, J. J. Shim and Y. R. Lee, ChemSusChem, 2021, 14, 2133–2140 CrossRef CAS PubMed.
- G. De Gonzalo, Biocatal. Biotransform., 2022, 40, 252–257 CrossRef CAS.
- N. Guajardo and P. D. De Maria, Mol. Catal., 2020, 485, 110813–111018 CrossRef CAS.
- J. Zhang, G. B. White, M. D. Ryan, A. J. Hunt and M. J. Katz, ACS Sustainable Chem. Eng., 2016, 4, 7186–7192 CrossRef CAS.
- H. J. Salavagione, M. De Bruyn, V. L. Budarin, G. J. Ellis, J. H. Clark and P. S. Shuttleworth, Green Chem., 2017, 19, 2550–2560 RSC.
- K. Pan, Y. Fan, T. Leng, J. Li, Z. Xin, J. Zhang, L. Hao, J. Gallop, K. S. Novoselov and Z. Hu, Nat. Commun., 2018, 9, 5197–5206 CrossRef PubMed.
- T. Debshama, B. Schmidt, A. Laschewsky and H. Schlaad, Macromolecules, 2021, 54, 2720–2728 CrossRef.
- T. Marino, F. Galiano, A. Molino and A. Figoli, J. Membr. Sci., 2019, 580, 224–234 CrossRef CAS.
- A. Mohamed and S. Yousef, Sustainable Technol. Green Econ., 2021, 1, 14–23 CrossRef.
- R. A. Milescu, A. Zhenova, M. Vastano, R. Grammons, S. Lin, C. H. Lau, J. H. Clark, C. R. McElroy and A. Pellis, ChemSusChem, 2021, 14, 3367–3381 CrossRef CAS PubMed.
- P. Tomietto, F. Russo, F. Galiano, P. Loulergue, S. Salerno, L. Paugam, J. L. Audic, L. De Bartolo and A. Figoli, J. Membr. Sci., 2022, 643, 120061–120076 CrossRef CAS.
- J. E. Camp, S. B. Nyamini and F. J. Scott, RSC Med. Chem., 2020, 11, 111–117 RSC.
- A. Duval and L. Averous, Green Chem., 2022, 24, 338–349 RSC.
Footnote |
† Denotes equal contribution. |
|
This journal is © The Royal Society of Chemistry 2022 |