DOI:
10.1039/D1EN00929J
(Tutorial Review)
Environ. Sci.: Nano, 2022,
9, 81-104
Adsorption and desorption mechanism of aromatic VOCs onto porous carbon adsorbents for emission control and resource recovery: recent progress and challenges†
Received
10th October 2021
, Accepted 9th November 2021
First published on 15th November 2021
Abstract
As is universally acknowledged, the fast advances in industrialization and urbanization have caused the rapid increase in the emission of aromatic volatile organic compounds (VOCs), which results in not only much environmental pollution and human health hazard, but also a significant waste of resources. Under such circumstances, it is urgent that some scientific strategies should be adopted to eliminate or reuse those VOCs. Two important strategies for VOC emission control are adsorption and dynamic desorption on porous carbon adsorbents (PCAs), which help to capture valuable VOCs or remove unvalued VOCs. In this review, the research developments of selective adsorption of gaseous aromatic VOCs onto different porous PCAs were systematically reviewed, especially the interaction mechanism between adsorbates and adsorbents during the adsorption process. Besides, the critical influential factors for the desorption of aromatic VOCs onto PCAs are also discussed, and the mechanisms focused on deactivation and regeneration of PCAs associated with the interaction mechanism of adsorbents and adsorbates are presented. Finally, the integrated processes based on adsorption and desorption for emission control and resource recovery are carefully summarized. Overall, this review expounds the characteristics of adsorption and desorption of aromatic VOCs on various PCAs as well as the integrated technologies for emission control and resource recovery of industrial VOC exhaust. We hope that this work can lay the foundation for the efficient abatement and recovery technologies of VOCs from industrial exhaust.
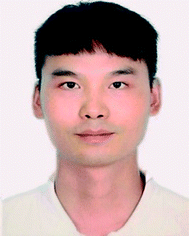 Weiping Zhang | Weiping Zhang received his Ph.D. degree in Chemical Engineering from South China University of Technology, China in 2016. He conducted postdoctoral work at the Guangdong University of Technology from 2016 to 2020 under the supervision of Professor Taicheng An. He is now a lecturer at Guangdong University of Technology, China. His research mainly focuses on the preparation of nanomaterials, and their applications in environmental remediation and resource recovery and conversion. |
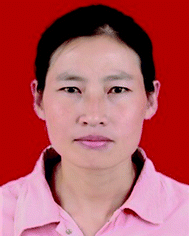 Guiying Li | Prof. Guiying Li received her bachelor's and master's degrees from Nanjing Agricultural University and Northwest Normal University, China, respectively, and her PhD degree in environmental science from Griffith University, Australia. She is now a full professor in the Institute of Environmental Health and Pollution Control as well as the School of Environmental Science and Engineering, Guangdong University of Technology. Her academic background covers both environmental and microbiology science. Her research interest mainly focuses on the development of photocatalysis and photoelectrocatalysis-based bactericidal methods; the application mechanism of photocatalytic bactericidal decomposition; and the biodegradation of organic pollutants in liquid and gaseous phases using isolated bacterial strains. She has published more than 300 SCI papers in reputable journals, such as Water Res., Appl. Catal. B, ES&T and J. Catal. She is the holder of 70 issued patents both from China and USA, and the second award winner of the Natural Science Prize from the Ministry of Education (MOE), China. Now, she also serves on the editorial board of Research Journal of Biotechnology. |
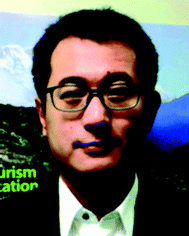 Huajie Yin | Huajie Yin obtained his Ph.D. in 2015 from the National Center for Nanoscience & Technology and Tsinghua University, China. He subsequently took a research fellow position at Griffith University's Center for Clean Environment and Energy, Queensland, Australia, led by Prof. Huijun Zhao. He won a Griffith University Research Fellow award in 2016. He has focused his research on controllable synthesis of 2D materials and their related applications in electrocatalysis, energy storage, and conversion. |
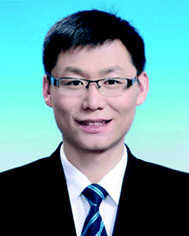 Kun Zhao | Kun Zhao received his PhD in metallurgy physical Chemistry in 2017 (with Professor Ranbo Yu) from the University of Science and Technology Beijing (USTB). During his PhD research, he had five years of training (2012–2017) with Professor Zhiyong Tang at the National Center for Nanoscience and Technology (NCNST). He worked as a postdoctoral research fellow with Professor Huijun Zhao and Dan Wang at the Griffith University, Australia, from 2017 to 2019. He is currently an associate professor at North China Electric Power University, China. His current research interests are focused on the design and exploration of novel photo & photothermal catalytic systems in the science and engineering field, aiming to accomplish the entire life cycle evaluation for CO2 utilization. |
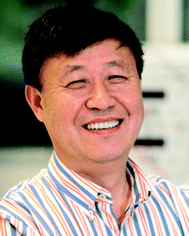 Huijun Zhao | Huijun Zhao is the founding director of the Center for Clean Environment and Energy, Griffith University, Queensland, Australia. He is a Fellow of Royal Society of Chemistry (FRSC) and Fellow of the Royal Australian Chemical Institute (FRACI), and the recipient of the R.H. Stokes Medal for electrochemistry. He has expertise in energy and environmental nanomaterials. The development of environmental and energy materials is one of his current research focuses. He has published more than 300 SCI papers in reputable journals, such as Nature, Nature Energy, Angewandte Chemie International Edition and Advanced Materials. |
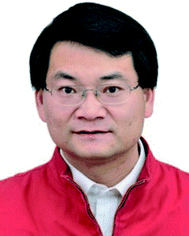 Taicheng An | Taicheng An received his PhD in environmental science from Sun Yat-Sen University (Zhongshan University), China in 2002. He is currently a full professor in the School of Environmental Sciences and Engineering and the director of the Institute of Environmental Health and Pollution Control, Guangdong University of Technology (GDUT). His research interests mainly focus on: 1. the transportation mechanisms, fate prediction and health effects of emerging organic contaminants; 2. synthesis, characterization, optimization and application of nanostructured catalysts and natural mineral materials in environmental remediation; 3. applications of integrated technology, especially adsorption–photocatalysis and adsorption–biotrickling filtration in VOC degradation for industrial exhaust abatement. He has over 100 patents (60 issued) and published over 480 referenced research papers (including 430 SCI papers) in reputable journals, such as PNAS, JACS, ES&T, Water Res., Appl. Catal. B and J. Catal. In recognition of his research achievements, he was selected as one of the most cited Chinese authors in the field of Environmental Sciences by Elsevier's Scopus database for seven consecutive years from 2014 to 2020. He is the winner of the National Natural Science Funds for Distinguished Young Scholars, and the second award winner of the Natural Science Prize from Ministry of Education (MOE, China), Distinguished Professor of Chang Jiang Scholars of MOE as well as the Pearl River Scholars Program, and the Young Scientist Winner of Scientific Committee on Problems of the Environment (SCOPE) in 2011. Now, he is also co-chair of the China-Ireland Consortium, and Asian/Pacific section Chairman of the International Society for Environmental Geochemistry and Health. He also serves as an Associate Editor of Appl. Catal. B: Environ. and Crit. Rev. Env. Sci. Tec., and on the Editorial board of Nature-Sci. Rep., ACS EST Eng., Journal of Environmental Chemical Engineering, Environ. Geochem. Health and Atmos. Pollut. Res. |
Environmental significance
Engineered porous carbon adsorbents (PCAs) are recognized as one of the efficient VOC adsorbents for recovering and eliminating industrial VOCs due to their low cost and high adsorption capacity. In this review, starting from the emission characteristics of aromatic VOCs from industrial processes, we focus on the elaboration of the adsorption and desorption characteristics and mechanism for aromatic VOCs, and a systematic documentation associated with the interaction mechanism between adsorbates and adsorbents during the adsorption process onto different PCAs is presented. The integrated processes based on adsorption and desorption for emission control and resource recovery are carefully summarized. Overall, this review expounds the characteristics of adsorption and desorption of aromatic VOCs onto PCAs as well as the integrated technologies for emission control and resource recovery of industrial VOC exhaust.
|
1. Introduction
Industrial processes such as petroleum refining, furniture manufacturing, machinery and equipment manufacturing, printing, and many other industrial activities have led to massive emission of volatile organic compounds (VOCs).1–3 Most of them are toxic and can result in the formation of secondary pollutants, including secondary organic aerosols (SOAs),4,5 tropospheric ozone,6,7 and photochemical smog,8,9 which will threaten the human and environmental health.1,10–12 Unfortunately, the industrial VOC emission has been rapidly increasing in the past few years as modernization, industrialization and urbanization continue in China (rising from 15.3 to 29.4 Tg from 2011 to 2013 with an average growth rate of 38.3% each year).13 The emitted VOCs include alkenes, alkanes, aromatics, ketones, esters, aldehydes, alcohols, halocarbons, and nitrogen/sulfur-containing compounds.2,14–16 Among all those components, aromatics are considered as the greatest atmospheric pollutants due to their high contribution to SOAs and photochemical O3 formation.6,7,17 However, aromatics are essential raw materials in industrial manufacture, which also makes them valuable regardless of their industrial exhaust.18,19 Thus, recycling and reusing them will also bring great economic value and environmental benefits.
In recent years, policies and regulations of industrial VOC emission have been implemented by many countries and regions to reduce the serious impact on the environment.14,20 To meet those regulations, numerous approaches, such as adsorption,18,21,22 condensation,23 absorption,24–26 catalytic oxidation,27,28 biological degradation,29–31 and photocatalytic oxidation,32–36 have developed rapidly to reduce the industrial VOC emission. However, due to the diversity and complexity of industrial VOCs, most of these technologies are limited to the decomposition of VOCs into H2O and CO2 which goes against the peaking carbon dioxide emissions and carbon neutrality. Selective adsorption-based technologies, by contrast, can be recognized as economical and efficient control strategies for their potential to recycle both adsorbents and adsorbates,18 typically to capture highly toxic and value-added compounds, such as aromatic VOCs, from industrial exhaust. Thus, it is also considered as a good strategy to improve the environment as well as develop the economy sustainably, which ultimately governs the design of adsorbent materials in the future.
With the above advantages, numerous porous adsorbents with selective properties including metal organic frameworks (MOFs),37,38 porous carbon (activated carbon (AC), biochar, graphene, carbon nanotubes (CNTs), and ordered mesoporous carbon (OMC)),18,39–44 porous polymers (hypercrosslinked polymeric resins, covalent organic frameworks, porous copolymers, and porous polystyrene),45–48 and some inorganic adsorbents (such as molecular sieve, silica gel)49–51 have been developed. Among them, porous carbon adsorbents (PCAs) are recognized as one of the efficient VOC adsorbents to remove industrial VOCs due to their low cost and high adsorption capacity.52 The carbon materials can be regulated by tuning the pore structure such as the micropores, mesopores, and macropores for VOC accessibility during the adsorption process.39,53,54 Porous carbon materials can selectively adsorb particular VOC molecules by controlling the pore structure and surface functional groups but show lower selectivity with non-polar VOC molecules.55,56 Hence, it is still a challenge to design PCAs with highly selective adsorption for some high-value VOCs because carbon materials cannot be flexibly modulated in terms of structure and composition like MOFs. Besides the adsorption selectivity, having a high adsorption capacity is another essential element for efficient carbon adsorbents, which is closely associated with a high specific surface area, suitable pore structure, functional groups, and adsorption active sites. Thus, balancing the percentage of pore structure and functional groups and their distribution characteristics on PCAs is a key point to improve the adsorption selectivity and adsorption capacity.
Although adsorption is the vital issue for recycling valuable VOCs, the effective desorption of VOC molecules from adsorbents also plays a key role in both generation of adsorbents and resource recovery of VOCs. There are three issues which need to be paid attention to when desorbing VOCs for the efficiency and degree of desorption. Firstly, traditional desorption methods, including thermal desorption (thermal, electrothermal, and microwave heating desorption), vacuum desorption, and solvent-extraction, are energy costly or go against economical and effective desorption when recycling valuable industrial VOCs.18 Another issue lies in the initial adsorption interaction between adsorbents and adsorbates which is closely related to desorption, governing the conditions of desorption methods. In general, these interactions can be divided into physical interactions and chemical interactions. The usual physical interactions refer to electrostatic interactions (dipole–dipole and π–π stacking) and van der Waals interactions (dispersive forces); the chemical interactions are hydrogen bonding and weak covalent bonding.18,57 Typically, strong adsorption interactions such as chemical interactions will certainly go against the desorption process of adsorbates, which usually causes higher energy consumption.58,59 Thirdly, the hydrophobic surface, channel structure, defects, and confinement effect of adsorbents will also have significant impacts on the selectivity and strength associated with these interactions.60–64 Thus, design of adsorbents for adsorption of target VOC molecules, desorption efficiency and difficulty should also be considered.
Previous reviews have predominantly focused on the progress on the adsorption characteristics of VOCs, but there is almost no summary associated with the adsorption and desorption mechanism, and their correlation with emission control and resource recovery of VOCs. In this review, we give a systematic commentary on not only the selective adsorption and desorption characteristics of aromatic VOCs but also their mechanisms associated with the properties of PCAs. Firstly, we carefully discuss the key points: the superiority and drawback of PCAs on the selective adsorption of aromatic VOCs and their mechanism. Secondly, the regeneration approaches and influential factors of adsorbents are discussed, and the corresponding procedure effects and their potential consequences (transformation of active sites, secondary products, etc.) are also clarified. Then, we clarify the desorption mechanism associated with the chemical interactions, VOC diffusion and adsorbent deactivation. Finally, the integrated processes based on adsorption and desorption for emission control and resource recovery are carefully summarized. We are confident that this comprehensive review will provide information and be instructive for understanding the basic principle of aromatic VOC adsorption on porous adsorbents which will promote the emission control and resource recovery of typical VOCs in practical industrial exhaust.
2. Emission characteristics of aromatic VOCs from industrial processes
As important chemical raw materials and organic solvents, aromatic VOCs such as BETX (benzene, toluene, ethylbenzene, and xylenes) are vital components of the total industrial emission due to their wide use in industrial processes of the petroleum industry, surface coating industry, vehicle manufacturing, printing industry, paint manufacturing, medicine production, and storage and transport.13,65,66 Thus, the recycling and reuse of these aromatic VOCs will be conducive not only to reducing the environmental hazard but also to earning secondary economic values. However, the process of effective recycling is complicated as the composition, proportion, and concentration of aromatic VOCs are distinctively different in different industrial emission sources. Hence, a summary of emission characteristics based on the composition and contribution of aromatic VOCs from different emission sources is of great significance for emission control and resource recovery of aromatic VOCs.
2.1 Emission from the petroleum industry
The petroleum industry refers to a number of plants including petrochemical plants (crude distillation, catalytic cracking, catalytic reforming, hydrocracking, coking, refining, refinery gas processing, etc.), basic chemical plants (production of secondary products), feedstock storage tanks, and wastewater treatment plants, which are the major emission sources of VOCs during petrochemical processing.14,67 In these processes, aromatic VOCs account for a major proportion even though alkanes are the most abundant species, which are principally emitted from unexpected leaks and process waste streams and during handling products or reactants.14,68 As shown in Table S1,† the aromatic VOCs emitted from these processes are toluene, styrene, benzene, ethylbenzene, diethylbenzene, ethyltoluene, 1,3,5-trimethylbenzene, m/p-xylene, and 1,2,4-trimethylbenzene.68–70 The mass concentrations of aromatic VOCs in source samples differ widely, probably because of various emission strengths or leakage from the process unit. As previously reported, the alkanes contributed the most to the VOC emission (ranging from 62.4 wt% to 75.4 wt%), and the percentage of aromatics only ranged from 6.1 wt% to 7.7 wt% in the petrochemical plant.69 However, aromatic VOCs are considered to be the abundant species due to their contribution to the production of large quantities of organic chemicals in the basic chemical plant, such as aromatic hydrocarbon-containing polymers and fine chemicals.69 Another source of fugitive aromatic VOC emission (the second largest contributor of TVOCs, 21.1 wt% to 45.5 wt%) in addition to the alkanes (the largest contributor) is from wastewater treatment facilities.70 Although paraffin emissions in the petrochemical industry occupy a major part, aromatic VOCs play an important part in certain plants. Therefore, the emission control and resource recovery of aromatic VOCs are particularly important in some plants of the petroleum industry.
2.2 Emission from storage and transportation
The VOC emission from storage and transportation refers to the emission produced from oil storage (crude oil, fuel oil, diesel oil, and gasoline) and organic liquids (toluene, xylene, benzene, and ethylbenzene), due to some particular industry structures (Table S2†).6,71 The VOC emission of a fixed roof storage tank primarily includes evaporation loss during the static storage (small breathing loss) and working loss during the delivery and receipt of materials (large breathing loss).72 Due to the working losses, a large amount of aromatics, including styrene, toluene, benzene, xylene, and ethylbenzene, will be emitted from the storage of organic solvents and organic raw materials in petrochemical and chemical industries.73–75 In addition, a mass of aromatic VOCs, including xylene, toluene, benzene, ethylbenzene, 1,3,5-trimethylbenzene, ethyltoluene, and 1,2,3-trimethylbenzene, will also be produced in the course of the loading, leakage, and trans-shipment of crude oil, fuel oil, diesel oil, and gasoline.6,74 In order to decrease the emission of these harmful gases, recovery technologies are usually adopted to realize the recycling of these VOCs.76,77
2.3 Emission from paint manufacturing and surface coating industry
The aromatic VOC emissions from paint manufacturing and surface coating are attributed to the use of aromatics-containing raw materials or solvents. As listed in Table S3,† the aromatic VOC emission from paint manufacturing includes toluene, m/p-xylene, o-xylene, ethylbenzene, and styrene.6,74,78,79 Besides, the surface coating industry includes metal surface coating, wood furniture coating, plastic surface coating, fabric surface coating, vehicle manufacturing (automobiles and trucks), and other coating processes. In these processes, aromatics are often used as solvents to dilute paint or improve the drying effect, which certainly produce a lot of aromatic VOCs (the details can be seen in Table S4†). Especially in fugitive emission, a large amount of aromatic VOCs, such as toluene, ethylbenzene, m/o/p-xylene, o/m/p-ethyltoluene, 1,3,5-trimethylbenzene, styrene, and 1,2,4-trimethylbenzene,65,74,78,80–84 is emitted from spraying, airing and drying processes, which will pose a risk to the workers and environment. Therefore, it is very necessary to realize the emission control of these worthless VOCs. However, for these value-added VOCs with high concentration, the method of recovery and reuse is of great significance for resource saving and reducing the burden of terminal treatment.
2.4 Emission from the printing industry and other source profiles
The printing industry includes gravure printing, letterpress printing, offset printing, flexographic printing, and 3D printing. Aromatic VOC emission in the printing industry may occur from the printing process and cleaning the operation system, solvent recovery and drying process, including toluene, benzene, m-ethyl toluene, styrene, m/p-xylene, o-xylene, 1,2,3,5-tetramethylbenzene, and 2-ethyl-1,4-dimethylbenzene (Table S5†).66,78,80,81,85,86 Most emission of VOCs, especially, the fugitive emission, occurs while printing, using an organic solvent in ink or cleaning the machine. For example, aromatics of fugitive emission from gravure printing account for 10.6 wt% and from letterpress printing 51.11 wt%.78 Although aromatic VOCs account for the main emission components in the printing industry, the overall concentration and proportion are relatively low compared with other VOCs. Therefore, emission control measures rather than resource recovery are usually adopted to reduce the emissions of these pollutants.
These problems also exist in other industrial processes such as medicine production, plastic production, synthetic rubber/fiber production, manufacture of chemical raw materials, boiler manufacture, and shoemaking, which also produce aromatic VOCs, such as m/p-xylene, toluene, ethylbenzene, o-xylene, benzene, styrene, and 1,2,4-trimethylbenzene (Table S6†).6,74,78,87 These VOCs are highly volatile, easily diffusing to the whole facility and difficult to be collected in the exhaust-collecting system, which will bring environmental and health hazards.
Considering the above threat posed by the emission of VOCs, it is urgent to control or reduce the VOC emission. However, the difficulty lies in the fact that VOCs can only be collected under well-ventilated conditions but the manufactories equipped with a treatment or exhaust collection system either do not operate or operate only at fairly low efficiencies.88,89 Thus, some strategies such as optimizing the industrial processes, reducing the use of volatile solvents in the raw materials, adopting new materials and new energy, developing more reasonable sealing equipment, and using exhaust gas recovery and elimination equipment can be used to reduce the fugitive VOC emission.
3. Adsorption and desorption of aromatic VOCs onto PCAs and their mechanism
Porous carbon adsorption is considered as one of the most promising and economic strategies for emission control and resource recovery of industrial VOCs due to its high efficiency and low cost.90–93 The core issues of porous carbon adsorption commonly involve the adsorbents with high adsorption capacity, stable physical and chemical properties, efficient desorption, etc.94 Therefore, many PCAs including AC, activated carbon fiber (ACF), biochar, graphene, CNTs, and OMC,39,95–99 have been developed for removing or recovering different aromatic VOCs. These adsorbents can be designed as unique functional structures with high adsorption selectivity for recovering or removing highly toxic aromatic VOCs.100–102 In this section, we systematically summarize the structural characteristics of different PCAs and their selective adsorption properties for aromatic VOCs. What's more, the key effects of the pore structure, specific surface area, hydrophobic surface, and surface functional groups of adsorbents on the adsorption or desorption process are also discussed. Finally, the relationship between the adsorption and desorption mechanism of the PCAs and the characteristics of aromatic VOCs was carefully reviewed.
3.1 Adsorption of aromatic VOCs onto PCAs and its mechanism
3.1.1 Adsorption onto PCAs.
Porous carbon adsorbents can be designed as a particular structure for recovering some value-added aromatic VOCs or removing worthless VOCs by controlling the pore structure, hydrophobic sites, π-electronic structure and functional groups (Fig. 1).18,43,52,103 Here, we made a detailed review on the adsorption of aromatic VOCs by PCAs to illustrate the correlation mechanism between the adsorption selectivity of aromatic VOCs and the basic characteristics of PCAs.
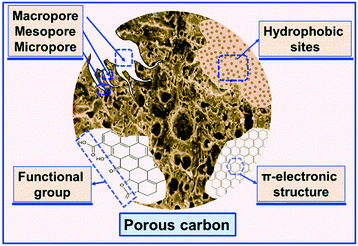 |
| Fig. 1 Basic structure of porous carbon adsorbents. | |
Activated carbon.
Activated carbon (AC) has a microporous structure with less than 2 nm of pore size, and can be used as an adsorbent to recover most types of VOCs including aromatics, esters, alkanes, and aldehydes.104 The adsorption capacity is often closely related to the microporous structure of AC.105 Izquierdo et al. reported a series of ACs with high surface areas (1128 m2 g−1) and high micropore volumes (0.52 cm3 g−1) for adsorption of toluene. Due to the high percentage of microporosity (64.6% to 89.1%), AC exhibited a high adsorption capacity of 7.44 mmol g−1 for toluene.106 This mainly benefits from the accessibility of micropores for toluene and their high-density distribution in AC. Besides, the pore structure of AC plays an important role in selective adsorption of aromatic VOCs because the micro-pore on AC determines the size selection of VOCs according to the nature of aromatics,107 and the porosity of AC also contributes more to the adsorption capacity of aromatic VOCs.108 For example, AC derived from peanut shell shows higher adsorption capacity for toluene as compared with p-xylene and ethyl benzene, particularly in low vapor concentration ranges, which is due to the right pore size for access to toluene except for the hydrophobic surface.104 In addition, AC is a natively nonpolar adsorbent due to the absence of oxygen functional groups such as COOH, –OH, and C–O–C, which often tend to adsorb hydrophobic VOCs, such as aromatic VOCs.107 For instance, the adsorption capacity of AC is higher for toluene than for alcohols because aromatic VOCs show higher affinity for AC due to their hydrophobic surface.107,109 That is, the surface hydrophobicity and microporous structure of AC together determine the adsorption capacity and selectivity for different types of VOCs.18,107 Therefore, surface functionalization and fabrication of hierarchical pores on AC will provide a big chance to achieve the selective adsorption, separation, and recovery of different types of VOCs.
Activated carbon fibers.
Activated carbon fibers (ACFs) are typical dimensional carbon materials, and often obtained from the carbonization and activation of polyacrylonitrile fibers, cellulose fibers, phenolic resin, etc.110,111 As ACFs consist of microfilaments with a high specific surface area, they present fewer mass transfer limitations and lower pressure than the solid of AC.112 Generally, the larger total specific surface area and micropore volume of ACFs can result in higher adsorption capacities for VOCs.110 ACFs with narrow micropores show superior adsorption capability to VOCs, and in particular, ACFs with ultra-microporous structure (dpore < 1 nm) have higher adsorption capacity for aromatic VOCs compared with ACFs with microporous structure (1 nm < dpore < 2 nm).18,107,112 Due to surplus π-electrons on ACFs, the π-electron rich region can also promote the adsorption of aromatic hydrocarbon on the carbon surface though π–π stacking interaction.110,113 ACFs are hydrophobic materials as well as AC, which can easily adsorb non-polar or weak polar VOCs such aromatic VOCs.114,115 As previous reported, ACFs possessed higher adsorption capacity for toluene and benzene compared to polar acetaldehyde, which was attributed to the hydrophobic property.116 However, ACFs have limitations in that their surface contains a very small number of oxygen groups, and the surface oxygen groups could restrict this interaction and weaken the adsorption of aromatic hydrocarbons.110 Hence, it is necessary to remove the water vapor before aromatic VOC adsorption.
Biochar.
Biochar is mainly produced by the slow pyrolysis of biomass (such as corn straw, rice husk, wheat straw, bamboo, pine wood) in inert atmosphere at 700 °C.18,117 Biochar has been thought to be a low-budget substitute and extensively used in VOC removal due to its rich source and milder pyrolysis conditions compared with commercial AC.118 The adsorption properties of VOCs on biochars are closely related to their structural properties including pore size, surface area, bulk properties, and hydrophobic properties, all of which are determined by the source type and pyrolysis conditions.118 It has been widely reported that removing the oxygen containing groups (increasing hydrophobicity) and increasing the aromaticity of biochar through tuning the pyrolysis temperature can consequently improve the adsorption of hydrophobic VOCs.119–121 Besides, the formation of π–π electron donor–acceptor interactions and hydrogen bonding interactions on the surface sites of biochar will also influence the adsorption selectivity and capacity of aromatic VOCs.121 However, the problem is that the adsorption mechanism of aromatic VOCs on biochar needs further research to promote the applications of biochar in VOC recovery due to the complexity of the VOC system.
Graphene.
Graphene is a typical two-dimensional material consisting of repeating hexagonal carbon rings, similar to the structure of the benzene molecule. The π and σ bond interaction between graphene and benzene molecules can increase the pervaporation performance of membranes for separating aliphatic/aromatic hydrocarbon mixtures.99,122 Generally, non-polar aromatic VOCs are mainly subject to electrostatic interactions, π–π bonds and hydrophobic interactions between the hydrophobic surface and aromatic VOCs in the adsorption process. Because of its hydrophobic surface, graphene shows high affinity for non-polar aromatic VOCs. rGO with a hydrophobic surface shows a longer breakthrough time and higher adsorption capacity than GO with oxygenated functional groups in the adsorption of benzene and toluene.98 Because of the presence of the hexagonal carbon ring and hydrophobic surface, the hydrophobic interactions between aromatic VOCs and graphene, π–π bonds and electrostatic interactions might be the effective forces for aromatic VOC adsorption on graphene.18,40,98,123,124 It is reported that activated graphene shows a higher specific surface area and surface properties for the adsorption capacity and selectivity of aromatic VOCs.40,125 For instance, after heat treatment at around 800 °C under KOH activation, enlargement of the pristine graphene surface and specific surface area is observed, with maximum volume capacities of 630 and 3510 m3 g−1 for acetaldehyde and toluene gas, respectively. The higher removal efficiency for toluene (98%) than for acetaldehyde (30%) is due to the π–π interactions between toluene molecules and the surface of pristine graphene.125
Carbon nanotubes.
Similar to the constituent unit of graphene, the CNT is a one-dimensional tubular structure formed by rolling up graphite sheets resulting in π confinement.126,127 CNTs have been attracted much attention in the removal of aromatic VOCs owing to their high specific surface area and lower mass transfer resistance.33 Recently, many adsorption interaction mechanisms of aromatic VOCs on CNTs have been discussed, which include π–π interactions, hydrophobic interactions, electrostatic interactions, and hydrogen bonds.126,128,129 However, the individual mechanism cannot explain all the adsorption interactions between aromatic VOCs and CNTs. The basic structure and nature such as the side chain and polarity of several benzene derivatives should be also considered, which will influence the adsorption mechanism.127,130 For instance, Chen et al. found that the hydrophobicity of several aromatic derivatives and the adsorption affinity are not significantly correlated.131 Besides, the functional group of CNTs is also an important factor for adsorption selectivity because it can not only change the hydrophobic properties and surface area of CNTs, but also seriously affect the surface charge distribution of CNTs, which is closely related to the strength of electron-donor–acceptor (EDA) π–π interaction between aromatic VOCs and the benzene-like ring (Fig. S1†).126,127,131–133 Although many literature studies discuss the mechanism of adsorption from π–π interactions, few reports explain them by quantitatively analysing the individual interaction.
Ordered mesoporous carbon.
Ordered mesoporous carbon (OMC) is a kind of mesoporous material with a large pore volume, high specific surface area, tunable pore size, and mechanical stability, which can serve as an adsorbent for VOC adsorption.42,134–137 It is reported that the surface chemistry and pore size of OMCs are considered as two of the most important factors that affect the adsorption selectivity and capacity of organic compounds.136 OMCs with well-controlled pores with pore size larger than 3.5 nm and bimodal-like pore size distribution endow the adsorbates with a higher diffusion rate than some conventional adsorbents like AC.18,42 As for the adsorption of aromatic VOCs, OMCs show high adsorption capacity for aromatic compounds as compared with alkanes, which is attributed to the higher π–π interaction between OMCs and the benzene ring.42,138 Compared with microporous adsorbents, OMCs have unique advantages. Although microporous adsorbents such as ACs show high adsorption ability to VOCs because the pore size of ACs is close to the molecule sizes of VOCs, the strong affinity will unavoidably increase the difficulty of VOC desorption due to their high mass transfer resistance. OMCs with relatively large pore size can completely overcome this shortcoming, and the adsorbed VOCs can be decomposed easily due to their higher diffusion rate.18
Functionalized PCAs.
The modification of PCAs aims to enhance the trapping efficiency and adsorption selectivity of gaseous VOCs by improving their surface functionalities. Wang et al. reported carbonized polydopamine adsorbents (C-PDAs) for aromatic VOC adsorption.52 It was found that, at low pressure, the adsorption capacity of C-PDAs for VOCs increased with the surface O and N contents of C-PDAs, and their adsorption capacities for C7H8 and C6H6 were up to 1455.8 and 1491.9 mg g−1 at a P/P0 of 0.6, which were much higher than those of many other adsorbents. Kim et al. compared three types of ACs modified with sulfur/amine/amino-silane groups for benzene adsorption.139 It indicated that ACs modified by incorporating sulfur/amine (electron-donating) groups can acquire high surface acidity to show greatly enhanced capturing capability to benzene compared with other modified adsorbents. Besides, the functionalized PCAs also can be used as gas sensors for VOC detection.140 Ndiaye et al. presented the gas sensor responses of functionalized CNTs to xylene and benzene vapors.141 It was found that the desorption process was very quick with a very short response time in the absence of a methyl group (benzene) as compared with aromatic VOCs bearing methyl groups (xylene). This demonstrates that the entire gas response is driven by π–π interaction and π–alkyl or alkyl–alkyl interaction, i.e., van der Waals interactions.
3.1.2 Adsorption mechanism.
Commonly, the adsorption mechanism of VOCs on PCAs includes pore selectivity and electrostatic attraction. These adsorption mechanisms, especially in selective adsorption, are closely related to the characteristics of PCAs (functional groups, pore structure, hydrophobicity, etc.), characteristics of VOCs (polarity, structure, etc.), and environmental variables (water, etc.).55,142 Here, the importance of the research on the adsorption mechanisms of VOCs and their close relationship with the interaction between VOCs and PCAs, adsorption capacity and adsorption selectivity are displayed. In addition, some new mechanisms or expounding mechanisms from a new perspective are also discussed in this summary.
In terms of the characteristics of PCAs, the adsorption rate, adsorption selectivity, adsorption capacity, and adsorption strength of VOCs usually depend on the pore structure, specific surface area, functional groups and hydrophobicity (Fig. 2).145 Yang et al. studied the kinetics and behaviors of toluene adsorption on ACs with various pore structures.146 It was found that the adsorption rate during external surface adsorption, particle diffusion and final equilibrium were determined by the pore volume and structure, specific surface area, and ratio of meso- and macro-pores to the total pore volume. Although meso- and macro-pores contribute more to interfacial mass transfer, the micropores dominate the adsorption capacity and main adsorptive sites of adsorbents. Lu et al. reported a N-doped hierarchical porous carbon possessing high adsorption capacity for toluene (up to 585 mg g−1).147 They found that the pore size of the microporous material was the major contributor to the adsorption capacity for toluene, and this result was also proved in a previous report (Fig. 2A and B).144 Furthermore, the pore size also determined the adsorption selectivity, because only the suitable size of VOC molecules can access into the microporous structure of PCAs.107 In addition, the adsorption strength associated with interactions between PCAs and VOCs mainly depends on functional groups including –OH, –COOH,
O and other modified groups (–NH2 and –NO2) (Fig. 2C). Vohra et al. explored the role of the functional groups on the surface and the interaction between the functional groups and benzene rings in the adsorption, and found that the surface oxygen-based groups possibly initiate an electron donor–acceptor mechanism with the benzene aromatic ring π electrons.148 Su et al. also found the positive contribution of oxygen-containing and nitrogen-containing functional groups to electrostatic force for VOC adsorption.149 Furthermore, the hydrophobic sites of PCAs was usually regarded as the main adsorption sites for aromatic VOCs due to their enhanced π–π stacking interaction. Yu et al. investigated the adsorption performance of GO and rGO for toluene and benzene.124 It was found that the adsorption capacity of rGO for toluene (304.4 mg g−1) and benzene (276.4 mg g−1) was higher than that of GO. The enhanced adsorption capacity was attributed to the hydrophobic surface of rGO, resulting in the increase of the hydrophobic sites and improvement of the hydrophobic interaction between the benzene ring and rGO. These results all showed that suitable pore structure, functional group modification and hydrophobic π–π active sites could significantly improve the adsorption selectivity of aromatic VOCs on PCAs.
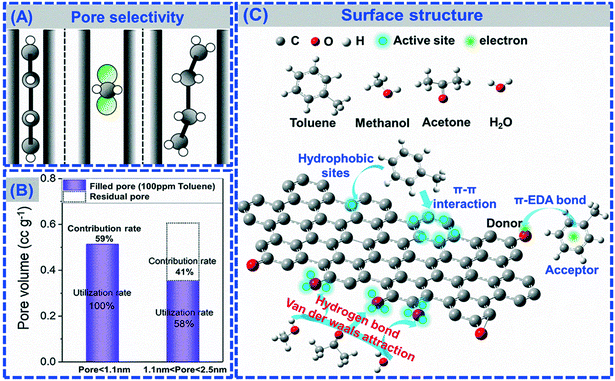 |
| Fig. 2 Adsorption mechanism of VOCs on PCAs. (A) Illustrations of the H/wall distance for benzene, DCM, and cyclohexane molecules, respectively; (B) the contribution and utilization rates of different pores for toluene adsorption at 100 ppm; (C) the competitive adsorption process (reproduced with permission from ref. 143. Copyright 2017 American Chemical Society, ref. 144. Copyright 2020 Elsevier, and ref. 113. Copyright 2019 Springer). | |
Besides, the characteristics (molecular polarity or structure) of VOCs will lead to the competitive adsorption mechanism of VOCs on PCAs. Stronger adsorption affinity was observed between the adsorbent with a weak polar surface and weak polar VOCs than polar VOCs. PCAs with a weak polar surface and benzene-like ring structure commonly showed excellent adsorption performance for aromatics such as BTEX, attributed to their properties of weak or non-polarity and structure of benzene aromatic ring π electrons. Chen et al. studied the competitive adsorption of toluene, cyclohexane, and methyl ethyl ketone on mesoporous graphitized carbon.150 It was found that, at 298 K, mesoporous graphitized carbon possessed the highest dynamic adsorption capacity of 4.63 mmol g−1 to toluene, because of the π–π interaction between the aromatic ring and graphene sheet. Rajabi et al. displayed the competitive adsorption mechanism of hexane, acetone, p-xylene, and toluene on biochar.151 They found that chemical interactions via electrostatic attraction, hydrogen bonding and pore-filling were the main mechanisms for VOC adsorption. Besides, environmental variables such as humidity also play a significant role in competitive adsorption of VOCs on PCAs. Huang et al. indicated that pore filling occurred with increased relative humidity since the water molecules with high polarity showed higher affinity to the surface functional groups, and the water molecules preferentially occupied the adsorption sites to form water clusters through hydrogen bonding.152 Su et al. pointed out that humidity caused the pronounced decrease in the non-electrostatic contribution to all functional groups of AC, leading to a decrease of adsorption capacity of VOCs.149
Recently, numerous reports also have elaborated on the adsorption mechanism of VOCs on PCAs from a relatively novel perspective, such as linear solvation energy relationship (LSER) and linear free energy relationships (LFER). The LSER method is frequently used to clarify the adsorption interactions for VOCs with various characteristics, which is helpful for improving the understanding of the interactions that affect VOC adsorption onto adsorbents and their contributions to adsorption.153 Li et al. investigated the interactions between VOCs and multiwalled CNTs (MWCNTs) by regressing the obtained log
Kd with LSER.154 They found that the carboxyl and carbonyl groups of MWCNT offer high adsorption capacity for VOCs due to the hydrogen-bond acidity and π-/n-electron pair interactions. Jia et al. investigated the adsorption interactions between AC and VOCs at various initial water contents using the combination of LSER and Dubinin–Radushkevich equation.155 It was found that the initial water vapor can decrease the adsorption capacity and partition coefficient of VOCs, especially at low VOC concentration. According to LSER, the electron acceptor ability and dispersive force of VOCs play major roles in adsorption. LFER has been frequently used to model molecular interactions between different environmental media and organic pollutants.156 Li et al. investigated the VOC adsorption mechanisms on MWCNTs using the LFER approach.157 They found that the hydrogen-bond donor and acceptor on the surface of the sorbent contributed to the sorption in the gaseous phase compared with the negative one found for sorbents completely in water. Those results show that LSER and LFER are useful to predict the adsorption performance of VOCs on adsorbents and facilitate the design of efficient systems for VOC removal or recycling.
Although there have been many studies on the adsorption mechanism of VOCs on PCAs, many of which are only at the laboratory stage with relatively clean conditions. The complex environment (including fine dust, high boiling point oil, sulfur/nitrogen-containing VOCs) encountered in the actual industrial process will significantly decrease the removal efficiency of VOCs, and the related mechanism is rarely researched. In particular, the research on the interface change mechanism after PCAs adsorbed VOCs is also insufficient.
3.2 Desorption of aromatic VOCs onto PCAs and its mechanism
Desorption is an important step in recovery or post-processing of VOCs for adsorbents. A perfect sorbent for preconcentrating VOCs should not only possess boundless adsorption capacity for interesting compounds but also completely desorb these compounds at moderate temperatures. However, it is difficult to reach the ideal level mentioned for the adsorption capacity and desorption efficiency of the same adsorbent. This is because the desorption is not only related to the desorption strategies, but also affected by the surface molecular structure and pore structure of the adsorbent.18 Among them, it will not only involve the accessibility of the adsorbate during the adsorption process, but also the steric hindrance effect caused by the change of the pores and the surface molecular force during the desorption process of the adsorbate, which will depress the diffusion and mass transfer efficiency of VOC molecules in the pore channel. Moreover, in many cases, the adsorbent is not completely inert but has some weak activities (such as oxidation or reduction activity). During the desorption process, the adsorbate will receive external energy and undergo a conversion reaction on the active interface, which usually induces the formation of hardly volatile species, and subsequently causes blockage of pores and passivation of active surfaces.158
3.2.1 Desorption strategies.
Desorption strategies include purge gas stripping, displacement desorption, vacuum swing desorption, and thermal swing desorption.159 These desorption technologies have great differences in desorption efficiency and relevance to adsorbents except for their processing properties. In this section, the characteristics, advantages, and disadvantages of these desorption technologies in desorption of aromatic VOCs are summarized.
Purge gas stripping.
Purge gas stripping is conducted by passing an inert gas at the same pressure and temperature used while adsorbing. The adsorbate can be desorbed under the effect of partial pressure drop caused by the inert gas passing through the fixed bed.159 Desorption is known as an endothermic process, but the strategies of purge gas stripping are usually limited due to the lack of external energy resulting in lower desorption efficiency. Montes-Morán reported a series of sludge-based adsorbents for removing odorous VOCs. Desorption experiments under similar conditions demonstrate that a large part of the VOCs pre adsorbed is irreversibly adsorbed. For highly microporous materials, only the molecules adsorbed in the outer-most pores can be desorbed.160 Although purge gas stripping has low desorption efficiency, it has low requirements on equipment.
Displacement desorption.
Displacement desorption means that the adsorbent is regenerated by a competitive adsorbed species (desorbent) that displaces the species previously adsorbed. Typical displacement desorption, such as organic solvent extraction and acid aqueous washing, can achieve efficient desorption of VOCs. Falletta et al. attempted to use CH3OH as a extraction solvent of VOCs for regeneration of polyaniline (PANI)-based materials, and found that the best PANI-based material demonstrated high adsorption/desorption efficiency retention after more than four cycles of solvent extraction.161 Kim et al. found that acid aqueous washing (H2SO4 and C2H2O4) combined with thermal air is also an effective method for recovering the micro-porosity of the spent AC, and this combined technique can be reused to remove toluene without obvious loss of its adsorption ability.162 It can be seen from the above examples that displacement desorption is a very effective method for adsorbent regeneration at some levels. However, because the new desorbent is introduced in the process, it needs to be separated from both the adsorbent and product, so that the adsorbent can be reused and the adsorbate can be effectively recovered.
Vacuum swing desorption.
Vacuum swing desorption usually carries out the desorption process under vacuum for separating VOCs from adsorbates, which is an important part of vacuum swing adsorption (such as pressure swing adsorption).94 Due to relatively less energy consumption, lower capital investment, and shorter recycle time in industry applications, vacuum swing desorption has been extensively applied to recover VOCs. The vacuum swing desorption process is not only related to the porous structure but also the interaction force between the adsorbate and adsorbent. He et al. found that the desorption rate of o-xylene over mesoporous silica gel is faster than that over microporous silica gel in the vacuum but it will be restricted by the interaction force between the adsorbate and adsorbent.163 This is mainly because the confinement effect (adsorption interactions) of the pore interface in the adsorbate will severely inhibit the mass transfer and diffusion of VOC molecules although the driving force of the higher-pressure difference can promote the gasification process of VOCs.164 That is, vacuum swing desorption cannot achieve 100% desorption efficiency, and it will gradually decrease as the cycle runs.163,165 These retained VOC molecules occupy the adsorption sites of the adsorbents, resulting in the loss of their original adsorption capacity in the end.
Thermal swing desorption.
Thermal swing desorption is carried out by heating the adsorbents with flow gas, which mainly provides an external energy to achieve rapid mass transfer and desorption. Because of its fast and efficient properties, thermal swing desorption has been widely used in adsorbent regeneration and VOC recovery. Kong et al. reported a high desorption performance of p-xylene on carbon–silicon adsorbents during thermal desorption. The adsorption capacity in the fifth cycle was around 201 mg g−1 as compared with the initial adsorption capacity (229 mg g−1), which dropped by only 1.5% for the last test.166 However, thermal desorption will be affected by the pore structure of the adsorbent and will cause incomplete desorption. Typically, in some adsorbents with sinusoidal and straight channels, aromatic VOCs, such as toluene, are much easier to be desorbed from the channel intersection and external surface, but they are difficult to be desorbed from the sinusoidal and straight channels.165 Furthermore, the microporous structure will severely limit the desorption efficiency to aromatic VOCs, because the microporous structure has a stronger steric effect and mass transfer resistance.167 In this case, aromatic VOCs can only be completely desorbed at high temperatures.
Photoinduced desorption.
Photoinduced desorption means that the adsorbents convert light energy to heat energy under light induction to realize VOC desorption. Liu et al. used the photoinduced method to investigate the desorption behavior of styrene over Ag nanoparticles incorporated in MOFs.168 It was found that silver nanoparticles could convert light energy into thermal energy upon exposure to simulated solar light, and then this localized heat initiated the thermal desorption of the adsorbed styrene from Ag/UiO-66. Thus, efficient in situ regeneration of Ag/UiO-66 was successfully realized under sun light illumination. Owing to the rapid VOC desorption and adsorbent regeneration, photoinduced desorption can be selected as one more promising candidate method for VOC removal or recycling. However, there are relatively few studies in this area, especially the light-induced desorption mechanism and its relationship with the surface changes of the adsorbent are still unclear.
3.2.2 Influencing factors on desorption.
Desorption of aromatic VOCs is influenced by many factors including the temperature of the external environment and the structure and characteristics of the adsorbent. The clarification of influencing factors on desorption aims to reveal their key interaction mechanism for VOC desorption.
Temperature.
Temperature-based desorption is a conventional strategy for recovering the adsorbate and allowing adsorbent reuse. For some adsorbents such as AC and zeolites, the high temperature allows the complete elimination of the adsorbates; however, for some polymers, these adsorbents are not stable at high temperatures (>350 °C).46,169,170 These unstable adsorbents can be only used at a very low temperature, which leads to many adsorbates remaining after regeneration. Hence, the selection of optimal desorption temperature should not only consider the desorption efficiency but also the properties of the adsorbent. Besides, the efficiency of a temperature-based desorption strategy depends on the adsorption intensity as well as adhesion of adsorbents–adsorbates. Due to the unequal adsorption force on porous adsorbents, the desorption efficiency of VOCs varies greatly in a certain temperature range. The optimal desorption temperature is often determined by the interaction intensity between adsorbates and adsorption sites, which is usually obtained by temperature programed desorption (TPD).171,172 To qualitatively determine the interaction intensity, Li et al. used the parameter ΔT (difference in temperature between the boiling point of aromatic VOCs and temperature of the highest desorption peak) to evaluate the affinities of ZSM-5/SBA-15 for toluene, and the highest ΔT of the ZSM-5/SBA-15-hexagonal prism was very consistent with its adsorption properties of having the strongest adsorption capacity and the longest breakthrough time for toluene.173
Porous structure.
The porous structure associated with the specific surface area is a key factor in the VOC adsorption and desorption process. Generally, a microporous structure is beneficial for enhancing the adsorption capacity but adverse for the desorption efficiency due to its high mass-transfer resistance.174 To enhance the mass transfer, the fabrication of a hierarchically porous structure, including micropores, mesopores and macropores, is often considered.173 Kosuge et al. studied the desorption differentiation of toluene and benzene in mesoporous silicas and microporous AC and found that the desorption rate and efficiency in mesoporous silicas were much higher than those in AC; meanwhile, the complete elimination of VOCs in mesoporous silicas needed a lower desorption temperature than that in AC (mesoporous silica, 150 °C; AC, 300 °C).175 It is not difficult to speculate that the mesoporous structure of mesoporous silicon can provide more space for the mass transfer and diffusion of VOCs, and the rapid transfer of VOC molecules will block the micropores of AC and cause slower mass transfer and diffusion, leading to low desorption efficiency.176,177
Composition and functional groups.
The composition has significant relevance with interactions between adsorbents and aromatic VOCs. For example, in benzene-ring containing microporous carbon or materials, the π–π stacking interaction will make the desorption of aromatic VOCs in a microporous environment more difficult.162,178 Under this typical interaction, microporous carbon has better selectivity and stronger force for aromatic VOCs, but the aromatic VOCs can only achieve desorption under higher external energy.175,177,179 The functional groups in some adsorbents can be complementary to desorption processes. For some hydrophobic porous silica adsorbents, the rapid desorption of aromatic VOCs can be achieved under low external energy, which is attributed to the weak interactions of weak π-system hydrogen bonding between aromatic VOC molecules and silanols on the silica surface.175,180,181 However, the desorption complexity of VOCs is jointly determined by factors such as composition, pore structure and basic functional groups other than a single factor. Hence, how to balance the interactions between the adsorbate and adsorbent associated with these factors, to achieve efficient, stable, and low-energy desorption of the adsorbate from the adsorbent, is a core challenge for developing adsorbent materials in the future.
3.2.3 Desorption mechanism and deactivation.
In the present research, the adsorption mechanism of VOCs has been extensively studied; however, there are still relatively few studies on the desorption mechanism either at the theoretical level or the experimental level. Herein, thermal desorption, as a typical method of VOC recovery and adsorbent regeneration, is used as a sample to illustrate the desorption mechanism. The thermal desorption process can be grouped into three stages. The first stage is the evaporation of VOCs adsorbed on the surface layer; the second stage is the desorption of toluene adsorbed on mesopores and micropores during the multi-layer adsorption process; in the third stage, residue VOC molecules adsorbed in narrow micropores on the internal face start to desorb.182 This is just an ideal model to speculate on the adsorption and desorption process of aromatic VOCs. Actually, just as the adsorption mechanism described, the desorption mechanism is also a relatively complicated process, which can be summarized into three aspects such as the relevance between the chemical interface and the adsorbent, diffusion mechanism, and deactivation mechanism (Fig. 3).158,165,171,176,178,183,184
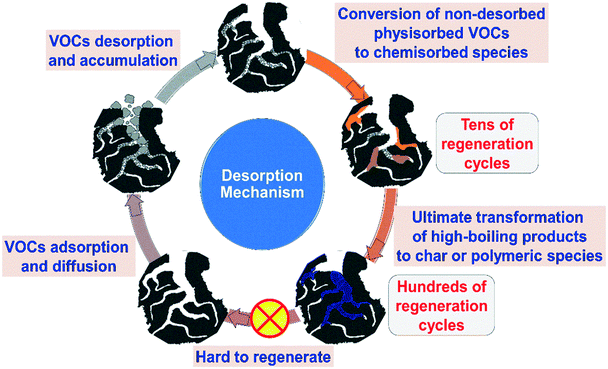 |
| Fig. 3 Desorption mechanism of VOCs on porous carbon materials (reproduced with permission from ref. 176. Copyright 2020 Elsevier). | |
In terms of chemical interfaces, it commonly determines the desorption difficulty of VOCs because strong adsorption interaction need higher energy to achieve complete VOC desorption. Lin et al. used the Pearson HSAB theory to interpret the influence of local hardness of carbon surfaces on the mechanism of adsorption/desorption for DCM of the ACs modified with various metal ions.171 According to the HSAB principle “hard acids prefer to be bonded to hard bases”, the interactions between AC and hard-acid DCM were enhanced when AC was doped with hard-acid metal ions, and the desorption activation energy of DCM on the hard-acid metal ions/AC is higher than that on the original AC.
In addition, mass diffusion is closely related to the desorption rate, which is determined by the porous structure and steric hindrance. For aromatic VOCs, the porous structure of adsorbents contributes greatly to mass diffusion, and the hierarchical pore, such as meso/micro pores, is surely beneficial for enhancing the desorption performances. More importantly, the mesoporous structure of adsorbents can significantly improve the diffusion constant of aromatic VOCs, which provides a high-speed access for VOC diffusion.165 In some respect, the desorption efficiency of VOC molecules is also associated with their properties; for example, the condensed phase of VOCs with a lower boiling point in the mesopores is likely to be more easily vaporized at a low desorption temperature, which is controlled by both external and internal diffusion mechanisms.178
The key influencing factors of desorption including chemical interfaces, porous structure, and properties of VOCs are also closely related to deactivation of PCAs because the strong chemical interaction and steric hindrance will cause VOC molecules to react with the active interface when accepting the external energy during the desorption process, thereby blocking the pores, occupying the active sites, and reducing the porosity, bringing a sharp drop in the adsorption capacity of the adsorbent.158,182 Furthermore, some unstable VOCs will also undergo thermal polymerization and pyrolysis on the interface, which cause the adsorbent deactivation because of the formation of some high-boiling point products attached to the adsorption site.176,185 Typically, in molecular oxygen containing systems, oxygen free radicals are generated to accelerate initiation reactions, leading to thermal oxidation of non-desorbed VOCs. The desorption reaction mechanism can be summarized as follows:176
1) Initiation reactions.
c. Ph–CH2–CH3 → Ph–CH2–CH2˙ + H˙ |
d. Ph–CH2–CH2–CH2–CH3 → Ph–CH2–CH2–CH2–CH2˙ + H˙ |
where Ph = phenyl group.
2) Propagation reactions.
a. Ph˙ + Ph(Alkene + CH3) → Ph–H + Ph(Alkene + CH2)˙ |
b. Ph–CH2 + Ph(Alkene + CH3) → Ph–CH3 + Ph(Alkene + CH2)˙ |
c. Ph˙ + Ph(C4H9) → Ph–H + Ph(C4H8)˙ |
d. Ph–CH2 + Ph(C4H9) → Ph–CH3 + Ph(C4H8)˙ |
3) Termination reactions.
a. Ph–CH2˙ + Ph–CH2–CH2˙ → Ph–CH2–CH2–CH2–Ph |
b. Ph(C4H8)˙ + Ph(Alkene + CH2)˙ → Ph(C4H9)–Ph(Alkene + CH3)(Alkene substituted biphenyl + C4H9) |
c. Ph(C4H9)–Ph(Alkene + CH2)˙– + –Ph(Alkene + CH2)˙ → Ph(C4H9)–Ph(Alkene)–Ph(Alkene + CH3)(Alkene substituted terphenyl + C4H9) |
d. Ph(C4H9)–Ph(Alkene)–Ph(Alkene + CH2)˙– + –Ph(Alkene + CH2)˙ → Ph(C4H9)–Ph(Alkene)–Ph(Alkene)–Ph(Alkene + CH3)(Alkene substituted phenyl terphenyl + C4H9) |
In summary, the study of the desorption mechanism is an important preliminary basis for adsorbent regeneration and VOC recovery technologies. However, no matter what kind of desorption technology has been adopted, how to avoid adsorbent deactivation under the premise of ensuring efficient adsorption is the key scientific problem.
4. Integrated technologies for VOC emission control and resource recovery
Waste gas treatment technology based on adsorption or desorption plays an irreplaceable role in VOC emission control and resource recovery. The integrated technologies of adsorption-based VOC emission control include adsorption–catalytic oxidation, adsorption–photocatalytic oxidation, adsorption–biotrickling filtration, adsorption–nonthermal plasma, etc., which have been widely used in the treatment of industrial VOCs. In terms of resource recovery of VOCs, the used technologies include adsorption–desorption–condensation recovery, adsorption–membrane separation and so on. All of these technologies associated with emission control or resource recovery are closely related to adsorption or desorption. In this summary, we have carefully reviewed the role of adsorption or desorption in different emission control and resource recovery technologies and discussed the synergistic mechanism among them.
4.1 Emission control
4.1.1 VOC control technologies based on PCA-adsorption.
Emission control technologies, by coupling adsorption technology based on PCAs or other porous materials with catalytic oxidation (catalytic combustion), photocatalytic oxidation, biotrickling filtration, and non-thermal plasma (NTP) oxidation technology, have aroused great interest in treatment of industrial waste gas. The adsorption component in the integrated technology can facilitate efficient degradation and transformation of VOCs under the oxidation of free radicals or the action of bacterial colonies, of which the fundamental purpose is to improve the selective conversion efficiency of VOCs to CO2. Alvarez-Merino et al. developed a number of WOx/AC materials for the catalytic combustion of toluene.186 Due to the hydrophobic character of the AC surface, WOx/AC materials show higher activity and CO2 selectivity at low reaction temperatures through preventing the adsorption of H2O generated during combustion of toluene, thereby preventing the deactivation of active centres. Nevertheless, using the WOx/AC system is frequently limited by its gasification temperature (about 400 °C), which restricts the ability to increase conversion values by rising the temperature of reaction. An's group has developed a series of adsorption–photocatalytic technology materials for degradation of aromatic VOCs. For example, by coupling CNTs with TiO2, the mass transfer and conversion efficiency of gaseous styrene can be significantly enhanced compared with pristine TiO2.187–189 Zhang et al. also coupled CNTs with visible-light photocatalytic Au/TiO2 to obtain an efficient and stable adsorption-photocatalyst, which greatly reduced the accumulation of VOC conversion products and improved the selective conversion of CO2.33,129 However, adsorption–photocatalytic technologies are limited to application in disposal of VOCs with low concentration. Besides, the biotrickling filtration method has been considered to be an effective technology for VOC emission control. Appling this method, PCAs can be used as packing materials, which can improve the transfer of hydrophobic VOCs from gas to liquid.190,191 Malhautier et al. used AC as a packing material in the biofilter to evaluate the VOC removal performance.192 For a load of 110 g VOC m−3 AC h−1, after a 55-day operation, the removal efficiency was higher in the biotic (85%) than in the abiotic filter (55%). Nevertheless, AC used as a packing material in biofilters to treat mixtures of VOCs is limited due to its poor affinity for pollutants including acetone, methanol, and halogenated compounds. Further, the cyclic operation of VOC adsorption and NTP assisted regeneration has attracted increasing interest because of cost-effectiveness and optimized energy consumption. Xu et al. reported an efficient adsorption–NTP catalyst for benzene removal.193 It was found that the coupling of the CuO/AC adsorption catalyst with NTP significantly improved the benzene removal efficiency (96.5%). However, the energy consumption and the formation of unwanted by products are still important concerns for removing VOCs from waste gas with NTP.194
Though the affinity between VOCs and active interfaces can be improved by PCA-based adsorption materials, inefficient disposal techniques or weak anti-interference ability can cause the deactivation of active components, making it difficult to face the challenge of the complex industrial VOC system. Therefore, the development of VOC emission control technology with high efficiency and stability is still difficult. So the research and development of adsorption–catalytic materials, high-efficiency bacterial strains and their corresponding in-depth mechanism are the top priority.
4.1.2 Integrated technologies for VOC emission control.
The emergence of integrated technologies is mainly because of the low efficiency and variability of a single technology, which cannot meet the emission standards for the disposal of VOCs in the actual industrial process. This is mainly because the industrial exhaust system includes a large amount of fine dust, high-boiling point oil, and sulfur/nitrogen-containing VOCs, which will lead to rapid deactivation of active components. Considering these problems, many integrated technologies have been developed and used in emission control of real industrial exhaust systems.10,191,195,197
Integrated technologies, such as biotrickling filtration–photocatalytic oxidation, electrostatic precipitation–photocatalytic oxidation, etc., have been developed by An's group for VOC emission control (Fig. 4). For example, Li et al. investigated an integrated technology of biotrickling filter–photocatalytic flow system at the pilot scale for elimination of VOCs emitted from a municipal solid waste transfer station.198 During the whole 60-day operation, the average removal efficiencies were >92%. Chen et al. investigated the integrated techniques of electrostatic precipitation and photocatalysis for VOC elimination in an e-waste dismantling workshop.199 No obvious difference was observed in the level and composition of VOCs, but electrostatic precipitation could eliminate 47.2% of total suspended particles, significantly decreasing the side effect of the particles on the subsequent photocatalysis. After photocatalytic purification, high average removal efficiencies were achieved, for aliphatic hydrocarbons (95.4%), aromatic hydrocarbons (95.7%), and halogenated hydrocarbons (87.4%), as well as nitrogen- and oxygen-containing compounds (97.5%) over the 60-day operation. Besides, organic waste gas in a paint plant was also treated using an integrated system with biotrickling filtration–photocatalysis at the pilot scale by the same group.196 It indicated that the removal efficiencies of the main components including toluene, ethyl acetate, xylene, ethyltoluene, ethylbenzene, and trimethylbenzene were within the range of 79.4–99.8% by the integrated technology even after 90-day operation. These integrated technologies can realize the emission control of the complex VOC system with high efficiency and stability as well as solve the interference of particulate matter and humidity in industrial exhaust systems. However, due to the limitations of the process, these integrated technologies can only be used for the disposal of low- and medium-concentration VOCs. The emission control of high-concentration VOCs usually adopts catalytic oxidation or combustion, but it usually encounters high energy consumption and disposal costs due to their high temperature and the noble metal needed.200,201
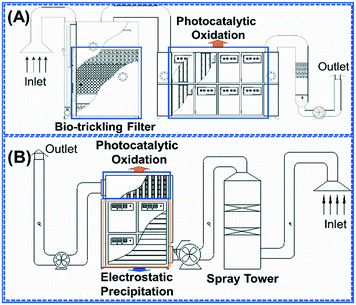 |
| Fig. 4 Integrated technologies for VOC emission control (A, biotrickling filtration–photocatalytic oxidation; B, electrostatic precipitation–photocatalytic oxidation) (reproduced with permission from ref. 195 and 196. Copyright 2017 Elsevier and Copyright 2012 Elsevier). | |
4.2 Resource recovery
VOC emission not only brings much environmental pollution and human health hazards, but also a great waste of resources. Therefore, many recovery technologies such as adsorption, absorption, condensation, and membrane separation have been employed to recycle VOCs emitted from industrial stationary sources, gas stations, oil & gas storage and transportation, etc.202 Each of these recovery technologies has its applicability and limitations because the most suitable recovery plan must be selected on the basis of the properties of the recovered gas (VOCs), the volume of gas and the utility conditions of the work area. Here, the merits and demerits of four recovery technologies and their integrated process in VOC recovery are reviewed, and the tendency of their development is discussed.
4.2.1 Resource recovery based on adsorption or desorption technology.
Adsorption technology can be used for resource recovery of VOCs mainly due to its high efficiency and stability, low equipment and operating costs, and recyclable adsorbents.72 However, during the recovery process, the bearing capacity and regeneration of the adsorbent as well as the subsequent recovery of VOCs are important issues to be considered. Hence, a series of integrated technologies such as adsorption–temperature swing/vacuum swing/microwave desorption, vacuum swing adsorption–condensation recovery, and adsorption–desorption–condensation recovery have been developed.203–205 Ramalingam et al. investigated dichloromethane recovery on an AC bed in a combined temperature and vacuum pressure swing adsorption process.206 It indicated that the integration of thermal and vacuum regeneration allowed achievement of 82% recovery of dichloromethane; moreover, it reduced the temperature of the AC bed from 93 °C to 63 °C, which significantly shortened the cooling time before starting the process over again. Han et al. reported a hybrid process of temperature swing–vacuum pressure swing adsorption (TS-VSA) to investigate the behaviors of adsorption and desorption of n-butyl acetate and p-xylene (typical polar–nonpolar VOCs) on AC.207 It was found that AC can steadily run for recovery and removal of VOCs using this hybrid process with >85% of adsorption capacity being maintained. Ambrożek et al. found that toluene was able to be recovered in the medium condensation temperature range, even at medium temperature of the purge gas using the integration of a thermal swing adsorption system with closed-loop regeneration of the adsorbent.208 Besides, to meet the needs of different engineering and economic conditions, other adsorption-based integrated technologies such as fixed bed adsorption–steam replacement regeneration–condensation recovery, fixed bed adsorption–vacuum regeneration–absorption recovery, and zeolite rotor concentration have been also developed to recover VOC waste gas.209,210
Although the adsorption-based integrated technologies have the characteristics of low energy consumption and high adsorption efficiency, the adsorption efficiency and capacity are also dependent on the efficiency of the adsorption device. Due to the limited capacity of the adsorbent, the adsorbent needs to be replaced regularly with new ones. The regeneration process will gradually cause the capacity of the adsorbents to decrease or even be deactivated. Therefore, it is difficult for the adsorption method to withstand a large load and it is generally more suitable for the recovery of low-concentration VOCs.
Besides, PCAs, as one of the most effective adsorbents in adsorption-based integrated technology, have a wider market demand because of their low cost, high adsorption capacity, reproducibility, etc. Nevertheless, now and then, PCAs often suffer from a number of problems such as pore blocking, combustion, inefficient desorption of high-boiling point solvents, and hygroscopicity.150,176 These problems will all lead to difficulty in regeneration, high operating costs, and secondary waste in the process of resource recovery of VOCs. In addition, low selectivity is also an important problem faced by such carbon materials in the resource recovery of VOCs, including: (1) low selectivity will cause the competitive adsorption of VOCs with water or other waste gas, resulting in the occupation of effective active sites, and the adsorption capacity of the target VOCs will drop sharply; (2) the structural defects will also lead to the deactivation of the adsorbent and difficulty in regeneration. Hence, the research and development of PCAs with high adsorption capacity, high selectivity and stability are the mainstream direction in the future.
4.2.2 Other technologies for resource recovery.
Considering the defects of adsorption-based technologies, membrane separation, absorption, and condensation-based technologies have been developed to be applied in gas stations, petrochemical storage tanks, petroleum refinery and automobile painting process for VOC recovery. As early as 1990, GKSS Forschungszentrum (Germany) developed an integrated process of membrane separation and condensation to achieve oil and gas recovery, and this process can effectively improve the separation efficiency of the gas membrane and the recovered oil and gas existing in a liquid state.211 However, the process was too complicated and had a high failure rate. After many improvements, the newly Vaconovent system developed by GKSS has been able to achieve stable and efficient oil and gas recovery.212 For VOC recovery in a petrochemical storage tank, Shen reported an integrated technology of absorption–membrane separation–pressure swing adsorption applied for BTX oil vapour recovery (Fig. 5).213 It was found that this integrated process has wide applicability for recovery of BTX from oil vapour produced in refineries/chemical plants, and it was proved to be the best solution for BTX oil vapour recovery with high separation efficiency, good recovery effects, and high exhaust gas emission standards. In addition, Zhang et al. investigated the upgrading of integrated technology of condensation–membrane separation–pressure swing adsorption to recycle oil and gas from refineries, and a higher efficiency (99.96%) for non-methane hydrocarbon removal was obtained after upgrading.76 Kim et al. used a membrane system for the recovery of VOCs from the painting process.214 It was found that the integrated membrane system showed better performance than the single stage process.
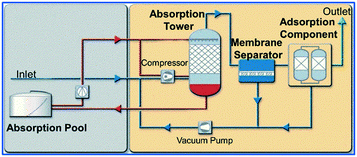 |
| Fig. 5 Integrated technologies for VOC recovery (reproduced from ref. 213 with permission from Environmental Protection of Chemical Industry, copyright 2021). | |
Although the integrated technologies based on the above have been applied in the VOC recovery of many industries, they still can only be applied in a few industrial processes due to issues such as high investment cost and floor space, energy consumption, low service life and applicability. Therefore, the development of VOC recovery integrated technologies with intelligence, integration, miniaturization and energy saving materials is the key research direction in the future.
5. Conclusions and future perspectives
5.1 Conclusions
This article started from the emission characteristics of aromatic VOCs from industrial processes, focusing on the elaboration of adsorption and desorption characteristics as well as the mechanism for aromatic VOCs, and a systematic documentation of the interaction mechanism between adsorbates and adsorbents during the adsorption process on different PCAs has been presented. Besides, the critical influential factors for desorption of aromatic VOCs onto PCAs are summarized, and the mechanisms focused on the deactivation and regeneration of PCAs associated with the interaction of adsorbents and adsorbates are carefully discussed. Finally, the integrated processes based on adsorption and desorption for emission control and resource recovery are systemically summarized. Overall, this review expounds the characteristics of adsorption and desorption of aromatic VOCs on PCAs as well as the integrated technologies for emission control and resource recovery of industrial VOC exhaust. We hope to provide references for the efficient abatement and recovery of VOCs from industrial exhaust.
5.2 Future perspectives
Aromatic VOCs are important parts of industrial waste gas, but they are also an important “urban resource”. While meeting the stringent emission standards in an effective and economical manner, many issues remain to be resolved to realize the utilization of aromatic VOCs. Here are the future efforts which should be focused on:
(1) In terms of material design, the construction of an effective adsorbent should not only take into account high selectivity and high adsorption capacity, but also the renewable capacity of the adsorbent and the availability of the adsorbate, such as designing adsorbents with hierarchical porous and ordered meso-porous structure. However, it is still a big challenge to design adsorbents with high selectivity and high regeneration efficiency for aromatic VOCs. The current adsorbents generally have low selectivity and regeneration efficiency. Apart from that, the adsorbent and adsorbent cannot be effectively reused, which severely limits their practical industrial applications due to high cost. Besides, the correlation between the desorption properties and adsorption active sites (i.e., acid sites, metal sites, π-electronic structure, defect sites, and active functional groups) should be established to efficiently capture more aromatic VOCs, which ultimately helps to deepen our understanding of the desirable properties and aid the future adsorbent design.
(2) For the benefits of emission control, the adsorption technology should be combined with other recovery and elimination technologies to form a more effective VOC emission control system. A single adsorption removal technology cannot meet the current emission standards. The development of more efficient tandem adsorption recovery technology and the combination of pollutant control technology (such as catalytic oxidation, photocatalytic oxidation) can not only realize resource recovery, but also eliminate useless exhaust gas, which is of great significance for environment improvement as well as the sustainable development of the economy.
(3) In terms of the mechanism, more work should be done for understanding the desorption mechanism: i) combining experiment with theory to explore the distribution, interfacial interactions, steric hindrance effects and diffusion mechanism of aromatic VOCs in the pore structure of the adsorbent; ii) demonstrating how the release and desorption mechanisms of VOCs are affected by desorption technologies and desorption conditions at the molecular level; iii) deriving greater knowledge of the deactivation mechanisms of various adsorbents by developing correlations between interfacial physical chemistry and their adsorption–desorption performance, and exploring effective regeneration methods for deactivated adsorbents (in situ regeneration in particular), to cut down operating costs and ultimately improve the viability of the industry.
(4) For the sake of VOC recovery, aromatic VOCs should be not only recovered from industrial VOC exhaust gas, but also separated as a single component. Industrial VOC exhaust gas contains not only various highly toxic aromatic VOCs, but also alkanes, alkenes, aldehydes, ketones, esters, VOCs, etc. Most of these adsorbents have adsorption effects on these VOCs and retain a certain adsorption capacity, which makes the subsequent separation process of aromatic VOCs complicated. To separate them more efficiently, some issues should be of concern as follows: i) developing efficient integrated technologies for recycling different types of high value-added VOCs; ii) optimizing integrated technologies and materials to achieve high-purity aromatic VOCs with high added-value.
Author contributions
Weiping Zhang wrote the original draft. Guiying Li helped in writing–editing. Huajie Yin contributed to the investigation of the main content. Kun Zhao contributed to the resources. Huijun Zhao contributed significantly to conceptualization and manuscript preparation. Taicheng An provided supervision and contributed significantly to manuscript preparation and review.
Conflicts of interest
There are no conflicts to declare.
Acknowledgements
This work was supported by the Local Innovative and Research Teams Project of Guangdong Pearl River Talents Program (2017BT01Z032), National Natural Science Foundation of China (42020104001 and 42007327), Basic and Applied Basic Research Foundation of Guangdong Province (2019A1515110298), and Guangdong Provincial Key R&D Program (2019B110206002).
Notes and references
- T. C. An, Y. Huang, G. Y. Li, Z. G. He, J. Y. Chen and C. S. Zhang, Pollution profiles and health risk assessment of VOCs emitted during e-waste dismantling processes associated with different dismantling methods, Environ. Int., 2014, 73, 186–194 CrossRef CAS PubMed.
- Z. He, G. Li, J. Chen, Y. Huang, T. An and C. Zhang, Pollution characteristics and health risk assessment of volatile organic compounds emitted from different plastic solid waste recycling workshops, Environ. Int., 2015, 77, 85–94 CrossRef CAS PubMed.
- L. J. Shen, P. Xiang, S. W. Liang, W. T. Chen, M. Wang, S. H. Lu and Z. W. Wang, Sources profiles of volatile organic compounds (VOCs) measured in a typical industrial process in Wuhan, Central China, Atmosphere, 2018, 9, 297 CrossRef.
- J. Sun, F. K. Wu, B. Hu, G. Q. Tang, J. K. Zhang and Y. S. Wang, VOC characteristics, emissions and contributions to SOA formation during hazy episodes, Atmos. Environ., 2016, 141, 560–570 CrossRef CAS.
- Z. Zhang, H. Wang, D. Chen, Q. Li, P. Thai, D. Gong, Y. Li, C. Zhang, Y. Gu, L. Zhou, L. Morawska and B. Wang, Emission characteristics of volatile organic compounds and their secondary organic aerosol formation potentials from a petroleum refinery in Pearl River Delta, China, Sci. Total Environ., 2017, 584–585, 1162–1174 CrossRef CAS PubMed.
- Q. Wang, S. Li, M. Dong, W. Li, X. Gao, R. Ye and D. Zhang, VOCs emission characteristics and priority control analysis based on VOCs emission inventories and ozone formation potentials in Zhoushan, Atmos. Environ., 2018, 182, 234–241 CrossRef CAS.
- H. Luo, G. Li, J. Chen, Q. Lin, S. Ma, Y. Wang and T. An, Spatial and temporal distribution characteristics and ozone formation potentials of volatile organic compounds from three typical functional areas in China, Environ. Res., 2020, 183, 109141 CrossRef CAS PubMed.
- J. J. Schauer, M. P. Fraser, G. R. Cass and B. R. T. Simoneit, Source Reconciliation of Atmospheric Gas-Phase and Particle-Phase Pollutants during a Severe Photochemical Smog Episode, Environ. Sci. Technol., 2002, 36, 3806–3814 CrossRef CAS PubMed.
- L. K. Xue, R. R. Gu, T. Wang, X. F. Wang, S. Saunders, D. Blake, P. K. K. Louie, C. W. Y. Luk, I. Simpson, Z. Xu, Z. Wang, Y. Gao, S. C. Lee, A. Mellouki and W. X. Wang, Oxidative capacity and radical chemistry in the polluted atmosphere of Hong Kong and Pearl River Delta region: analysis of a severe photochemical smog episode, Atmos. Chem. Phys., 2016, 16, 9891–9903 CrossRef CAS.
- J. Y. Chen, D. L. Zhang, G. Y. Li, T. C. An and J. M. Fu, The health risk attenuation by simultaneous elimination of atmospheric VOCs and POPs from an e-waste dismantling workshop by an integrated de-dusting with decontamination technique, Chem. Eng. J., 2016, 301, 299–305 CrossRef CAS.
- Y. Yu, Z. Qi, S. Ma, L. Xu, J. Zheng, Y. Gao, X. Shen, Y. Cai, W. Zhang, G. Li and T. An, Human exposome and biomarker database for soil pollutants at typical sites of industrial contamination, Sci. Bull., 2021, 66, 1705–1708 CrossRef.
- Y. Yang, H. Luo, R. Liu, G. Li, Y. Yu and T. An, The exposure risk of typical VOCs to the human beings via inhalation based on the respiratory deposition rates by proton transfer reaction-time of flight-mass spectrometer, Ecotoxicol. Environ. Saf., 2020, 197, 110615 CrossRef CAS PubMed.
- C. H. Zheng, J. L. Shen, Y. X. Zhang, W. W. Huang, X. B. Zhu, X. C. Wu, L. H. Chen, X. Gao and K. F. Cen, Quantitative assessment of industrial VOC emissions in China: Historical trend, spatial distribution, uncertainties, and projection, Atmos. Environ., 2017, 150, 116–125 CrossRef CAS.
- C. He, J. Cheng, X. Zhang, M. Douthwaite, S. Pattisson and Z. P. Hao, Recent advances in the catalytic oxidation of volatile organic compounds: A review based on pollutant sorts and sources, Chem. Rev., 2019, 119, 4471–4568 CrossRef CAS PubMed.
- R. P. Tong, L. Zhang, X. Y. Yang, J. F. Liu, P. N. Zhou and J. F. Li, Emission characteristics and probabilistic health risk of volatile organic compounds from solvents in wooden furniture manufacturing, J. Cleaner Prod., 2019, 208, 1096–1108 CrossRef CAS.
- W. Wei, S. X. Wang, S. Chatani, Z. Klimont, J. Cofala and J. M. Hao, Emission and speciation of non-methane volatile organic compounds from anthropogenic sources in China, Atmos. Environ., 2008, 42, 4976–4988 CrossRef CAS.
- Y. Ji, J. Zhao, H. Terazono, K. Misawa, N. P. Levitt, Y. Li, Y. Lin, J. Peng, Y. Wang, L. Duan, B. Pan, F. Zhang, X. Feng, T. An, W. Marrero-Ortiz, J. Secrest, A. L. Zhang, K. Shibuya, M. J. Molina and R. Zhang, Reassessing the atmospheric oxidation mechanism of toluene, Proc. Natl. Acad. Sci. U. S. A., 2017, 114, 8169–8174 CrossRef CAS PubMed.
- X. Y. Zhang, B. Gao, A. E. Creamer, C. C. Cao and Y. C. Li, Adsorption of VOCs onto engineered carbon materials: A review, J. Hazard. Mater., 2017, 338, 102–123 CrossRef CAS PubMed.
- W. Huang, J. Jiang, D. Wu, J. Xu, B. Xue and A. M. Kirillov, A Highly Stable Nanotubular MOF Rotator for Selective Adsorption of Benzene and Separation of Xylene Isomers, Inorg. Chem., 2015, 54, 10524–10526 CrossRef CAS PubMed.
- J. Ou, J. Zheng, R. Li, X. Huang, Z. Zhong, L. Zhong and H. Lin, Speciated OVOC and VOC emission inventories and their implications for reactivity-based ozone control strategy in the Pearl River Delta region, China, Sci. Total Environ., 2015, 530–531, 393–402 CrossRef CAS PubMed.
- C. Long, P. Liu, Y. Li, A. Li and Q. Zhang, Characterization of hydrophobic hypercrosslinked polymer as an adsorbent for removal of chlorinated volatile organic compounds, Environ. Sci. Technol., 2011, 45, 4506–4512 CrossRef CAS PubMed.
- L. Gibson, Mesosilica materials and organic pollutant adsorption: part A removal from air, Chem. Soc. Rev., 2014, 43, 5163–5172 RSC.
- B. Belaissaoui, Y. Le Moullec and E. Favre, Energy efficiency of a hybrid membrane/condensation process for VOC (Volatile Organic Compounds) recovery from air: A generic approach, Energy, 2016, 95, 291–302 CrossRef CAS.
- E. Dumont, A. Couvert, A. Amrane, C. Couriol, G. Darracq and P. Le Cloirec, Equivalent Absorption Capacity (EAC) concept applied to the absorption of hydrophobic VOCs in a water/PDMS mixture, Chem. Eng. J., 2016, 287, 205–216 CrossRef CAS.
- J. Ge and N. Choi, Fabrication of functional polyurethane/rare earth nanocomposite membranes by electrospinning and its VOCs absorption capacity from air, Nanomaterials, 2017, 7, 60 CrossRef PubMed.
- M. Guillerm, A. Couvert, A. Amrane, É. Dumont, E. Norrant, N. Lesage and C. Juery, Characterization and selection of PDMS solvents for the absorption and biodegradation of hydrophobic VOCs, J. Chem. Technol. Biotechnol., 2016, 91, 1923–1927 CrossRef CAS.
- J. R. Li, S. F. Zuo, P. Yang and C. Z. Qi, Study of CeO2 modified AlNi mixed pillared clays supported palladium catalysts for benzene adsorption/desorption-catalytic combustion, Materials, 2017, 10, 949 CrossRef PubMed.
- L. F. Liotta, Catalytic oxidation of volatile organic compounds on supported noble metals, Appl. Catal., B, 2010, 100, 403–412 CrossRef CAS.
- H. Liu, Y. Ma, J. Chen, M. Wen, G. Li and T. An, Highly efficient visible-light-driven photocatalytic degradation of VOCs by CO2-assisted synthesized mesoporous carbon confined mixed-phase TiO2 nanocomposites derived from MOFs, Appl. Catal., B, 2019, 250, 337–346 CrossRef CAS.
- H. Atagana, Biological degradation of crude oil refinery sludge with commercial surfactant and sewage sludge by Co-composting, Soil Sediment Contam., 2014, 24, 494–513 CrossRef.
- S. Padhi and S. Gokhale, Biological oxidation of gaseous VOCs–rotating biological contactor a promising and eco-friendly technique, J. Environ. Chem. Eng., 2014, 2, 2085–2102 CrossRef CAS.
- J. Y. Chen, G. Y. Li, Z. G. He and T. C. An, Adsorption and degradation of model volatile organic compounds by a combined titania-montmorillonite-silica photocatalyst, J. Hazard. Mater., 2011, 190, 416–423 CrossRef CAS PubMed.
- W. Zhang, G. Li, H. Liu, J. Chen, S. Ma and T. An, Micro/nano-bubble assisted synthesis of Au/TiO2@CNTs composite photocatalyst for photocatalytic degradation of gaseous styrene and its enhanced catalytic mechanism, Environ. Sci.: Nano, 2019, 6, 948–958 RSC.
- J. Y. Chen, Z. G. He, Y. M. Ji, G. Y. Li, T. C. An and W. Choi, •OH radicals determined photocatalytic degradation mechanisms of gaseous styrene in TiO2 system under 254 nm versus 185 nm irradiation: Combined experimental and theoretical studies, Appl. Catal., B, 2019, 257, 117912 CrossRef CAS.
- M. Yao, Y. Ji, H. Wang, Z. Ao, G. Li and T. An, Adsorption Mechanisms of Typical Carbonyl-Containing Volatile Organic Compounds on Anatase TiO2 (001) Surface: A DFT Investigation, J. Phys. Chem. C, 2017, 121, 13717–13722 CrossRef CAS.
- H. Wang, Y. Ji, J. Chen, G. Li and T. An, Theoretical investigation on the adsorption configuration and (*)OH-initiated photocatalytic degradation mechanism of typical atmospheric VOCs styrene onto (TiO2)n clusters, Sci. Rep., 2015, 5, 15059 CrossRef CAS PubMed.
- B. Manna, S. Mukherjee, A. V. Desai, S. Sharma, R. Krishna and S. K. Ghosh, A π-electron deficient diaminotriazine functionalized MOF for selective sorption of benzene over cyclohexane, Chem. Commun., 2015, 51, 15386–15389 RSC.
- M. Wen, G. Li, H. Liu, J. Chen, T. An and H. Yamashita, Metal-organic framework-based nanomaterials for adsorption and photocatalytic degradation of gaseous pollutants: recent progress and challenges, Environ. Sci.: Nano, 2019, 6, 1006–1025 RSC.
- M. P. Zhu, Z. F. Tong, Z. X. Zhao, Y. Z. Jiang and Z. X. Zhao, A microporous graphitized biocarbon with high adsorption capacity toward benzene volatile organic compounds (VOCs) from humid air at ultralow pressures, Ind. Eng. Chem. Res., 2016, 55, 3765–3774 CrossRef CAS.
- K. Rahbar Shamskar, A. Rashidi, P. Aberoomand Azar, M. Yousefi and S. Baniyaghoob, Synthesis of graphene by in situ catalytic chemical vapor deposition of reed as a carbon source for VOC adsorption, Environ. Sci. Pollut. Res., 2019, 26, 3643–3650 CrossRef CAS PubMed.
- S. Ragunath and S. Mitra, Carbon nanotube immobilized composite hollow fiber membranes for extraction of volatile organics from air, J. Phys. Chem. C, 2015, 119, 13231–13237 CrossRef CAS.
- G. Wang, B. J. Dou, Z. S. Zhang, J. H. Wang, H. E. Liu and Z. P. Hao, Adsorption of benzene, cyclohexane and hexane on ordered mesoporous carbon, J. Environ. Sci., 2015, 30, 65–73 CrossRef CAS PubMed.
- W. Zhang, G. Li, W. Wang, Y. Qin, T. An, X. Xiao and W. Choi, Enhanced photocatalytic mechanism of Ag3PO4 nano-sheets using MS2 (M = Mo, W)/rGO hybrids as co-catalysts for 4-nitrophenol degradation in water, Appl. Catal., B, 2018, 232, 11–18 CrossRef CAS.
- X. An, K. Zhao, W. Zhang, J. Yang, Y. Liao, L. Wang and D. Fu, Tailoring the pore structure modified with functional groups for superior CO2 adsorption capacity and the selectivity of separation, Fuel, 2022, 309, 122175 CrossRef CAS.
- S.-W. Lv, J.-M. Liu, Z.-H. Wang, H. Ma, C.-Y. Li, N. Zhao and S. Wang, Recent advances on porous organic frameworks for the adsorptive removal of hazardous materials, J. Environ. Sci., 2019, 80, 169–185 CrossRef PubMed.
- W.-Q. Wang, J. Wang, J.-G. Chen, X.-S. Fan, Z.-T. Liu, Z.-W. Liu, J. Jiang and Z. Hao, Synthesis of novel hyper-cross-linked polymers as adsorbent for removing organic pollutants from humid streams, Chem. Eng. J., 2015, 281, 34–41 CrossRef CAS.
- C. Daniel, P. Antico, H. Yamaguchi, M. Kogure and G. Guerra, Microporous-crystalline microfibers by eco-friendly guests: An efficient tool for sorption of volatile organic pollutants, Microporous Mesoporous Mater., 2016, 232, 205–210 CrossRef CAS.
- S. Zhang, Q. Yang, Z. Li, W. Wang, C. Wang and Z. Wang, Covalent organic frameworks as a novel fiber coating for solid-phase microextraction of volatile benzene homologues, Anal. Bioanal. Chem., 2017, 409, 3429–3439 CrossRef CAS PubMed.
- S. Liu, Y. Peng, J. Chen, W. Shi, T. Yan, B. Li, Y. Zhang and J. Li, Engineering surface functional groups on mesoporous silica: towards a humidity-resistant hydrophobic adsorbent, J. Mater. Chem. A, 2018, 6, 13769–13777 RSC.
- M. Kraus, U. Trommler, F. Holzer, F. Kopinke and U. Roland, Competing adsorption of toluene and water on various zeolites, Chem. Eng. J., 2018, 351, 356–363 CrossRef CAS.
- G. Zhang, Y. Liu, S. Zheng and Z. Hashisho, Adsorption of volatile organic compounds onto natural porous minerals, J. Hazard. Mater., 2019, 364, 317–324 CrossRef CAS PubMed.
- X. Wang, C. Ma, J. Xiao, Q. Xia, J. Wu and Z. Li, Benzene/toluene/water vapor adsorption and selectivity of novel C-PDA adsorbents with high uptakes of benzene and toluene, Chem. Eng. J., 2018, 335, 970–978 CrossRef CAS.
- Z. Guo, J. Huang, Z. Xue and X. Wang, Electrospun graphene oxide/carbon composite nanofibers with well-developed mesoporous structure and their adsorption performance for benzene and butanone, Chem. Eng. J., 2016, 306, 99–106 CrossRef CAS.
- Z. Guo, D. Zhou, X. Dong, Z. Qiu, Y. Wang and Y. Xia, Ordered hierarchical mesoporous/macroporous carbon: A high-performance catalyst for rechargeable Li-O2 Batteries, Adv. Mater., 2013, 25, 5668–5672 CrossRef CAS PubMed.
- M. P. Zhu, K. B. Zhou, X. D. Sun, Z. X. Zhao, Z. F. Tong and Z. X. Zhao, Hydrophobic N-doped porous biocarbon from dopamine for high selective adsorption of p-Xylene under humid conditions, Chem. Eng. J., 2017, 317, 660–672 CrossRef CAS.
- X. He, H. Sun, M. Zhu, M. Yaseen, D. Liao, X. Cui, H. Guan, Z. Tong and Z. Zhao, N-Doped porous graphitic carbon with multi-flaky shell hollow structure prepared using a green and 'useful' template of CaCO3 for VOC fast adsorption and small peptide enrichment, Chem. Commun., 2017, 53, 3442–3445 RSC.
- K. Vellingiri, P. Kumar, A. Deep and K.-H. Kim, Metal-organic frameworks for the adsorption of gaseous toluene under ambient temperature and pressure, Chem. Eng. J., 2017, 307, 1116–1126 CrossRef CAS.
- Y. Chen, Z. Qiao, H. Wu, D. Lv, R. Shi, Q. Xia, J. Zhou and Z. Li, An ethane-trapping MOF PCN-250 for highly selective adsorption of ethane over ethylene, Chem. Eng. Sci., 2018, 175, 110–117 CrossRef CAS.
- Y. Guo, Y. Li, J. Wang, T. Zhu and M. Ye, Effects of activated carbon properties on chlorobenzene adsorption and adsorption product analysis, Chem. Eng. J., 2014, 236, 506–512 CrossRef CAS.
- T. H. Noh, H. Lee, J. Jang and O. S. Jung, Organization and energy transfer of fused aromatic hydrocarbon guests within anion-confining nanochannel MOFs, Angew. Chem., Int. Ed., 2015, 54, 9284–9288 CrossRef CAS PubMed.
- C. Yang, U. Kaipa, Q. Z. Mather, X. Wang, V. Nesterov, A. F. Venero and M. A. Omary, Fluorous metal-organic frameworks with superior adsorption and hydrophobic properties toward oil spill cleanup and hydrocarbon storage, J. Am. Chem. Soc., 2011, 133, 18094–18097 CrossRef CAS PubMed.
- X. Zhang, X. Lv, X. Shi, Y. Yang and Y. Yang, Enhanced hydrophobic UiO-66 (University of Oslo 66) metal-organic framework with high capacity and selectivity for toluene capture from high humid air, J. Colloid Interface Sci., 2019, 539, 152–160 CrossRef CAS PubMed.
- A. Planchais, S. Devautour-Vinot, S. Giret, F. Salles, P. Trens, A. Fateeva, T. Devic, P. Yot, C. Serre, N. Ramsahye and G. Maurin, Adsorption of benzene in the cation-containing MOFs MIL-141, J. Phys. Chem. C, 2013, 117, 19393–19401 CAS.
- M. A. Granato, V. D. Martins, A. F. P. Ferreira and A. E. Rodrigues, Adsorption of xylene isomers in MOF UiO-66 by molecular simulation, Microporous Mesoporous Mater., 2014, 190, 165–170 CrossRef CAS.
- Z. Zhong, Q. Sha, J. Zheng, Z. Yuan, Z. Gao, J. Ou, Z. Zheng, C. Li and Z. Huang, Sector-based VOCs emission factors and source profiles for the surface coating industry in the Pearl River Delta region of China, Sci. Total Environ., 2017, 583, 19–28 CrossRef CAS PubMed.
- H. Wang, Y. Qiao, C. Chen, J. Lu, H. Dai, L. Qiao, S. Lou, C. Huang, L. Li, S. Jing and J. Wu, Source profiles and chemical reactivity of volatile organic compounds from solvent use in Shanghai, China, Aerosol Air Qual. Res., 2014, 14, 301–310 CrossRef CAS.
- W. Walls, Petroleum refining industry in China, Energy Policy, 2010, 38, 2110–2115 CrossRef.
- Z. Zhang, X. Yan, F. Gao, P. Thai, H. Wang, D. Chen, L. Zhou, D. Gong, Q. Li, L. Morawska and B. Wang, Emission and health risk assessment of volatile organic compounds in various processes of a petroleum refinery in the Pearl River Delta, China, Environ. Pollut., 2018, 238, 452–461 CrossRef CAS PubMed.
- W. Wei, S. Cheng, G. Li, G. Wang and H. Wang, Characteristics of volatile organic compounds (VOCs) emitted from a petroleum refinery in Beijing, China, Atmos. Environ., 2014, 89, 358–366 CrossRef CAS.
- Z. Mo, M. Shao, S. Lu, H. Qu, M. Zhou, J. Sun and B. Gou, Process-specific emission characteristics of volatile organic compounds (VOCs) from petrochemical facilities in the Yangtze River Delta, China, Sci. Total Environ., 2015, 533, 422–431 CrossRef CAS PubMed.
- M. Jackson, Organic liquids storage tanks volatile organic compounds (VOCS) emissions dispersion and risk assessment in developing countries: the case of Dar-es-Salaam City, Tanzania, Environ. Monit. Assess., 2006, 116, 363–382 CrossRef CAS PubMed.
- S.-J. Yang, X.-H. Liao, M.-Y. Dou, Q. Feng, H.-M. Li and S. Zou, Summary of VOCs Treatment Technology in Product Oil Depot, IOP Conf. Ser. Earth Environ. Sci., 2020, 508, 012199 CrossRef.
- T. Paulauskienė, V. Zabukas and P. Vaitiekūnas, Investigation of volatile organic compound (VOC) emission in oil terminal storage tank parks, J. Environ. Eng. Landsc. Manag., 2009, 17, 81–88 CrossRef.
- Q. He, Y. Yan, H. Li, Y. Zhang, L. Chen and Y. Wang, Characteristics and reactivity of volatile organic compounds from non-coal emission sources in China, Atmos. Environ., 2015, 115, 153–162 CrossRef CAS.
- C. Lu, H. Huang, S. Chang and S. Hsu, Emission characteristics of VOCs from three fixed-roof p-xylene liquid storage tanks, Environ. Monit. Assess., 2013, 185, 6819–6830 CrossRef CAS PubMed.
- C. Zhang, K. Li, Y. Zhang and G. Li, Status analysis and technical upgrading transform of VOCs recovery unit in refinery, Shiyou Yu Tianranqi Huagong, 2019, 48, 33–36 Search PubMed.
- H. Wang, H. Guo, Y. Zhao, X. Dong and M. Gong, Thermodynamic analysis of a petroleum volatile organic compounds (VOCs) condensation recovery system combined with mixed-refrigerant refrigeration, Int. J. Refrig., 2020, 116, 23–35 CrossRef CAS.
- J. Zheng, Y. Yu, Z. Mo, Z. Zhang, X. Wang, S. Yin, K. Peng, Y. Yang, X. Feng and H. Cai, Industrial sector-based volatile organic compound (VOC) source profiles measured in manufacturing facilities in the Pearl River Delta, China, Sci. Total Environ., 2013, 456–457, 127–136 CrossRef CAS PubMed.
- K. Na, Y. Kim, I. Moon and K. Moon, Chemical composition of major VOC emission sources in the Seoul atmosphere, Chemosphere, 2004, 55, 585–594 CrossRef CAS PubMed.
- G. Li, W. Wei, X. Shao, L. Nie, H. Wang, X. Yan and R. Zhang, A comprehensive classification method for VOC emission sources to tackle air pollution based on VOC species reactivity and emission amounts, J. Environ. Sci., 2018, 67, 78–88 CrossRef PubMed.
- H. Wang, L. Nie, J. Li, Y. Wang, G. Wang, J. Wang and Z. Hao, Characterization and assessment of volatile organic compounds (VOCs) emissions from typical industries, Chin. Sci. Bull., 2013, 58, 724–730 CrossRef CAS.
- R. Tong, X. Ma, Y. Zhang, G. Shao and M. Shi, Source analysis and health risk-assessment of ambient volatile organic compounds in automobile manufacturing processes, Hum. Ecol. Risk Assess., 2018, 26, 1–25 Search PubMed.
- J. Colman Lerner, E. Sanchez, J. Sambeth and A. Porta, Characterization and health risk assessment of VOCs in occupational environments in Buenos Aires, Argentina, Atmos. Environ., 2012, 55, 440–447 CrossRef CAS.
- Z. Zhou, W. Fang, Q. Luo, X. Wang and L. Wu, Emission characteristics of volatile organic compounds from automobile coating industry in Chongqing city, J. Environ. Sci. Eng. B, 2017, 6, 543–552 CAS.
- B. Yuan, M. Shao, S. Lu and B. Wang, Source profiles of volatile organic compounds associated with solvent use in Beijing, China, Atmos. Environ., 2010, 44, 1919–1926 CrossRef CAS.
- P. Potter, S. Al-Abed, D. Lay and S. Lomnicki, VOC emissions and formation mechanisms from carbon nanotube composites during 3D printing, Environ. Sci. Technol., 2019, 53, 4364–4370 CrossRef CAS PubMed.
- Y. Zhao, P. Mao, Y. Zhou, Y. Yang, J. Zhang, S. Wang, Y. Dong, F. Xie, Y. Yu and W. Li, Improved provincial emission inventory and speciation profiles of anthropogenic non-methane volatile organic compounds: a case study for Jiangsu, China, Atmos. Chem. Phys., 2017, 17, 7733–7756 CrossRef CAS.
- L. Tohid, Z. Sabeti, P. Sarbakhsh, K. Zoroufchi Benis, M. Shakerkhatibi, Y. Rasoulzadeh, R. Rahimian and S. Darvishali, Spatiotemporal variation, ozone formation potential and health risk assessment of ambient air VOCs in an industrialized city in Iran, Atmos. Pollut. Res., 2019, 10, 556–563 CrossRef CAS.
- M. Su, R. Sun, X. Zhang, S. Wang, P. Zhang, Z. Yuan, C. Liu and Q. Wang, Assessment of the inhalation risks associated with working in printing rooms: a study on the staff of eight printing rooms in Beijing, China, Environ. Sci. Pollut. Res., 2018, 25, 17137–17143 CrossRef PubMed.
- F. Gironi and V. Piemonte, VOCs removal from dilute vapour streams by adsorption onto activated carbon, Chem. Eng. J., 2011, 172, 671–677 CrossRef CAS.
- K. N. Gupta, N. J. Rao and G. K. Agarwal, Gaseous Phase Adsorption of Volatile Organic Compounds on Granular Activated Carbon, Chem. Eng. Commun., 2014, 202, 384–401 CrossRef.
- Q. Qian, C. Gong, Z. Zhang and G. Yuan, Removal of VOCs by activated carbon microspheres derived from polymer: a comparative study, Adsorption, 2015, 21, 333–341 CrossRef CAS.
- J. F. Vivo-Vilches, E. Bailon-Garcia, A. F. Perez-Cadenas, F. Carrasco-Marin and F. J. Maldonado-Hodar, Tailoring activated carbons for the development of specific adsorbents of gasoline vapors, J. Hazard. Mater., 2013, 263, 533–540 CrossRef CAS PubMed.
- C. Long, W. Yu and A. Li, Adsorption of n-hexane vapor by macroporous and hypercrosslinked polymeric resins: Equilibrium and breakthrough analysis, Chem. Eng. J., 2013, 221, 105–110 CrossRef CAS.
- F. Carrasco-Marín, D. Fairén-Jiménez and C. Moreno-Castilla, Carbon aerogels from gallic acid–resorcinol mixtures as adsorbents of benzene, toluene and xylenes from dry and wet air under dynamic conditions, Carbon, 2009, 47, 463–469 CrossRef.
- Y. Chiang, P. Chiang and E. Chang, Effects of surface characteristics of activated carbons on VOC adsorption, J. Environ. Eng., 2001, 127, 54–62 CrossRef CAS.
- L. Fournel, P. Mocho, J. L. Fanlo and P. Le Cloirec, External capillary condensation and adsorption of VOCs onto activated carbon fiber cloth and felt, Environ. Technol., 2005, 26, 1277–1287 CrossRef CAS PubMed.
- P. Samaddar, Y.-S. Son, D. C. W. Tsang, K.-H. Kim and S. Kumar, Progress in graphene-based materials as superior media for sensing, sorption, and separation of gaseous pollutants, Coord. Chem. Rev., 2018, 368, 93–114 CrossRef CAS.
- H.-X. Liu, N. Wang, C. Zhao, S. Ji and J.-R. Li, Membrane materials in the pervaporation separation of aromatic/aliphatic hydrocarbon mixtures — A review, Chin. J. Chem. Eng., 2018, 26, 1–16 CrossRef CAS.
- J. Y. Cheng, P. Wang, J. P. Ma, Q. K. Liu and Y. B. Dong, A nanoporous Ag(I)-MOF showing unique selective adsorption of benzene among its organic analogues, Chem. Commun., 2014, 50, 13672–13675 RSC.
- W. Choi, K. Min, C. Kim, Y. S. Ko, J. W. Jeon, H. Seo, Y. K. Park and M. Choi, Epoxide-functionalization of polyethyleneimine for synthesis of stable carbon dioxide adsorbent in temperature swing adsorption, Nat. Commun., 2016, 7, 12640 CrossRef CAS PubMed.
- D. J. Li, Z. G. Gu, I. Vohra, Y. Kang, Y. S. Zhu and J. Zhang, Epitaxial Growth of Oriented Metalloporphyrin Network Thin Film for Improved Selectivity of Volatile Organic Compounds, Small, 2017, 13, 1604035 CrossRef PubMed.
- J. Y. Chen, G. Y. Li, Y. Huang, H. M. Zhang, H. J. Zhao and T. C. An, Optimization synthesis of carbon nanotubes-anatase TiO2 composite photocatalyst by response surface methodology for photocatalytic degradation of gaseous styrene, Appl. Catal., B, 2012, 123–124, 69–77 CrossRef CAS.
- A. H. Bedane, T. X. Guo, M. Eić and H. Xiao, Adsorption of volatile organic compounds on peanut shell activated carbon, Can. J. Chem. Eng., 2018, 97, 238–246 CrossRef.
- B. Cardoso, A. S. Mestre, A. P. Carvalho and J. Pires, Activated carbon derived from cork powder waste by KOH activation: preparation, characterization, and VOCs adsorption, Ind. Eng. Chem. Res., 2008, 47, 5841–5846 CrossRef CAS.
- M. T. Izquierdo, A. M. de Yuso, R. Valenciano, B. Rubio and M. R. Pino, Influence of activated carbon characteristics on toluene and hexane adsorption: Application of surface response methodology, Appl. Surf. Sci., 2013, 264, 335–343 CrossRef CAS.
- M. A. Lillo-Ródenas, D. Cazorla-Amorós and A. Linares-Solano, Behaviour of activated carbons with different pore size distributions and surface oxygen groups for benzene and toluene adsorption at low concentrations, Carbon, 2005, 43, 1758–1767 CrossRef.
- Y.-C. Chiang, P.-C. Chiang and C.-P. Huang, Effects of pore structure and temperature on VOC adsorption on activated carbon, Carbon, 2001, 39, 523–534 CrossRef CAS.
- I. Ushiki, K. Kikuchi, Y. Sato, Y. Ito, S. Takishima and H. Inomata, Adsorption equilibria of VOCs (n-octane, propylene glycol monomethyl ether, ethanol, and 2-propanol) on activated carbon under supercritical carbon dioxide conditions, Fluid Phase Equilib., 2018, 462, 59–64 CrossRef CAS.
- Y. Bai, Z.-H. Huang, M.-X. Wang and F. Kang, Adsorption of benzene and ethanol on activated carbon nanofibers prepared by electrospinning, Adsorption, 2013, 19, 1035–1043 CrossRef CAS.
- P. Le Cloirec, Adsorption onto Activated Carbon Fiber Cloth and Electrothermal Desorption of Volatile Organic Compound (VOCs): A Specific Review, Chin. J. Chem. Eng., 2012, 20, 461–468 CrossRef CAS.
- G. Baur, I. Yuranov, A. Renken and L. Kiwi-Minsker, Activated carbon fibers for efficient VOC removal from diluted streams: the role of surface morphology, Adsorption, 2015, 21, 479–488 CrossRef CAS.
- F. Meng, M. Song, Y. Wei and Y. Wang, The contribution of oxygen-containing functional groups to the gas-phase adsorption of volatile organic compounds with different polarities onto lignin-derived activated carbon fibers, Environ. Sci. Pollut. Res., 2019, 26, 7195–7204 CrossRef CAS PubMed.
- G. Y. Oh, Y. W. Ju, M. Y. Kim, H. R. Jung, H. J. Kim and W. J. Lee, Adsorption of toluene on carbon nanofibers prepared by electrospinning, Sci. Total Environ., 2008, 393, 341–347 CrossRef CAS PubMed.
- Z. Xie, F. Wang, N. Zhao, W. Wei and Y. Sun, Hydrophobisation of activated carbon fiber and the influence on the adsorption selectivity towards carbon disulfide, Appl. Surf. Sci., 2011, 257, 3596–3602 CrossRef CAS.
- M. A. Lillo-Ródenas, D. Cazorla-Amorós and A. Linares-Solano, Benzene and toluene adsorption at low concentration on activated carbon fibres, Adsorption, 2010, 17, 473–481 CrossRef.
- C. Yan, Z. Cheng, Y. Tian, F. Qiu, H. Chang, S. Li, Y. Cai and X. Quan, Adsorption of Ni(II) on detoxified chromite ore processing residue using citrus peel as reductive mediator: Adsorbent preparation, kinetics, isotherm, and thermodynamics analysis, J. Cleaner Prod., 2021, 315, 128209 CrossRef CAS.
- X. Y. Zhang, B. Gao, Y. L. Zheng, X. Hu, A. E. Creamer, M. D. Annable and Y. C. Li, Biochar for volatile organic compound (VOC) removal: Sorption performance and governing mechanisms, Bioresour. Technol., 2017, 245, 606–614 CrossRef CAS PubMed.
- L. Li, S. Liu and J. Liu, Surface modification of coconut shell based activated carbon for the improvement of hydrophobic VOC removal, J. Hazard. Mater., 2011, 192, 683–690 CrossRef CAS PubMed.
- K. Sun, M. Keiluweit, M. Kleber, Z. Pan and B. Xing, Sorption of fluorinated herbicides to plant biomass-derived biochars as a function of molecular structure, Bioresour. Technol., 2011, 102, 9897–9903 CrossRef CAS PubMed.
- K. Yang, J. Yang, Y. Jiang, W. Wu and D. Lin, Correlations and adsorption mechanisms of aromatic compounds on a high heat temperature treated bamboo biochar, Environ. Pollut., 2016, 210, 57–64 CrossRef CAS PubMed.
- M. Kunaseth, P. Poldorn, A. Junkeaw, J. Meeprasert, C. Rungnim, S. Namuangruk, N. Kungwan, C. Inntam and S. Jungsuttiwong, A DFT study of volatile organic compounds adsorption on transition metal deposited graphene, Appl. Surf. Sci., 2017, 396, 1712–1718 CrossRef CAS.
- Y. Zhang, L. Zhang and C. Zhou, Review of Chemical Vapor Deposition of Graphene and Related Applications, Acc. Chem. Res., 2013, 46, 2329–2339 CrossRef CAS PubMed.
- L. Yu, L. Wang, W. Xu, L. Chen, M. Fu, J. Wu and D. Ye, Adsorption of VOCs on reduced graphene oxide, J. Environ. Sci., 2018, 67, 171–178 CrossRef PubMed.
- J. Kim, J. Kim, C. Lee, D. Jerng and H. Ahn, Toluene and acetaldehyde removal from air on to graphene-based adsorbents with microsized pores, J. Hazard. Mater., 2018, 344, 458–465 CrossRef CAS PubMed.
- B. Pan and B. Xing, Adsorption Mechanisms of Organic Chemicals on Carbon Nanotubes, Environ. Sci. Technol., 2008, 42, 9005–9013 CrossRef CAS PubMed.
- E. Diaz, S. Ordonez and A. Vega, Adsorption of volatile organic compounds onto carbon nanotubes, carbon nanofibers, and high-surface-area graphites, J. Colloid Interface Sci., 2007, 305, 7–16 CrossRef CAS PubMed.
- R. Amade, S. Hussain, I. R. Ocaña and E. Bertran, Growth and functionalization of carbon nanotubes on quartz filter for environmental applications, J. Environ. Eng. Ecol. Sci., 2014, 3, 1–7 CrossRef CAS.
- W. P. Zhang, G. Y. Li, H. L. Liu, J. Y. Chen, S. T. Ma, M. C. Wen, J. J. Kong and T. C. An, Photocatalytic degradation mechanism of gaseous styrene over Au/TiO2@CNTs: Relevance of superficial state with deactivation mechanism, Appl. Catal., B, 2020, 272, 118969 CrossRef CAS.
- F. Pourfayaz, Sh. Boroun, J. Babaei and B. Ebrahimi Hoseinzadeh, An Evaluation of the Adsorption Potential of MWCNTs for Benzene and Toluene Removal, Int. J. Nanosci. Nanotechnol., 2014, 10, 27–34 Search PubMed.
- W. Chen, L. Duan, L. Wang and D. Zhu, Adsorption of hydroxyl- and amino-substituted aromatics to carbon nanotubes, Environ. Sci. Technol., 2008, 42, 6862–6868 CrossRef CAS PubMed.
- D. Lin and B. Xing, Adsorption of Phenolic Compounds by Carbon Nanotubes: Role of Aromaticity and Substitution of Hydroxyl Groups, Environ. Sci. Technol., 2008, 42, 7254–7259 CrossRef CAS PubMed.
- W. Chen, L. Duan and D. Zhu, Adsorption of Polar and Nonpolar Organic Chemicals to Carbon Nanotubes, Environ. Sci. Technol., 2007, 41, 8295–8300 CrossRef CAS PubMed.
- B. Yuan, X. Wu, Y. Chen, J. Huang, H. Luo and S. Deng, Adsorption of CO2, CH4, and N2 on ordered mesoporous carbon: approach for greenhouse gases capture and biogas upgrading, Environ. Sci. Technol., 2013, 47, 5474–5480 CrossRef CAS PubMed.
- B. R. Ryoo, S. H. Joo, M. Kruk and M. Jaroniec, Ordered Mesoporous Carbons, Adv. Mater., 2001, 13, 677–681 CrossRef.
- D. J. Kim, H. I. Lee, J. E. Yie, S.-J. Kim and J. M. Kim, Ordered mesoporous carbons: Implication of surface chemistry, pore structure and adsorption of methyl mercaptan, Carbon, 2005, 43, 1868–1873 CrossRef CAS.
- M. Konggidinata, B. Chao, Q. Lian, R. Subramaniam, M. Zappi and D. Gang, Equilibrium, kinetic and thermodynamic studies for adsorption of BTEX onto Ordered Mesoporous Carbon (OMC), J. Hazard. Mater., 2017, 336, 249–259 CrossRef CAS PubMed.
- J. Zheng, K. Wang, Y. Liang, F. Zhu, D. Wu and G. Ouyang, Application of ordered mesoporous carbon in solid phase microextraction for fast mass transfer and high sensitivity, Chem. Commun., 2016, 52, 6829–6832 RSC.
- W. K. Kim, S. A. Younis and K. H. Kim, A strategy for the enhancement of trapping efficiency of gaseous benzene on activated carbon (AC) through modification of their surface functionalities, Environ. Pollut., 2021, 270, 116239 CrossRef CAS PubMed.
- A. Pauly, J. Brunet, C. Varenne and A. L. Ndiaye, Insight in the interaction mechanisms between functionalized CNTs and BTX vapors in gas sensors: Are the functional peripheral groups the key for selectivity?, Sens. Actuators, B, 2019, 298, 126768 CrossRef CAS.
- A. L. Ndiaye, J. Brunet, C. Varenne and A. Pauly, Functionalized CNTs-Based Gas Sensors for BTX-Type Gases: How Functional Peripheral Groups Can Affect the Time Response through Surface Reactivity, J. Phys. Chem. C, 2018, 122, 21632–21643 CrossRef CAS.
- R. Yamashita, Y. Saito and S. Sakuragawa, Molecular sieving behavior of carbonized wood: selective adsorption of toluene from a gas mixture containing α-pinene, J. Wood Sci., 2009, 55, 446–452 CrossRef CAS.
- G. Le Bozec, S. Giraudet, L. Le Polles and P. Le Cloirec,
1H NMR investigations of activated carbon loaded with volatile organic compounds: Quantification, mechanisms, and diffusivity determination, Langmuir, 2017, 33, 1605–1613 CrossRef CAS PubMed.
- S. Liu, Y. Peng, J. Chen, T. Yan, Y. Zhang, J. Liu and J. Li, A new insight into adsorption state and mechanism of adsorbates in porous materials, J. Hazard. Mater., 2020, 382, 121103 CrossRef CAS PubMed.
- L. Zhu, D. Shen and K. H. Luo, A critical review on VOCs adsorption by different porous materials: Species, mechanisms and modification methods, J. Hazard. Mater., 2020, 389, 122102 CrossRef CAS PubMed.
- X. Yang, H. Yi, X. Tang, S. Zhao, Z. Yang, Y. Ma, T. Feng and X. Cui, Behaviors and kinetics of toluene adsorption-desorption on activated carbons with varying pore structure, J. Environ. Sci., 2018, 67, 104–114 CrossRef PubMed.
- S. Lu, X. Huang, M. Tang, Y. Peng, S. Wang and C. P. Makwarimba, Synthesis of N-doped hierarchical porous carbon with excellent toluene adsorption properties and its activation mechanism, Environ. Pollut., 2021, 284, 117113 CrossRef CAS PubMed.
- M. S. Vohra, Adsorption-Based Removal of Gas-Phase Benzene Using Granular Activated Carbon (GAC) Produced from Date Palm Pits, Arabian J. Sci. Eng., 2015, 40, 3007–3017 CrossRef CAS.
- Z. Su, Y. Zhang, L. Huang, S. Wang, Y. Zhu, L. Li and X. Lu, Acetone adsorption on activated carbons: Roles of functional groups and humidity, Fluid Phase Equilib., 2020, 521, 112645 CrossRef CAS.
- T. Chen, C. Fu, Y. Liu, F. Pan, F. Wu, Z. You and J. Li, Adsorption of volatile organic compounds by mesoporous graphitized carbon: Enhanced organophilicity, humidity resistance, and mass transfer, Sep. Purif. Technol., 2021, 264, 118464 CrossRef CAS.
- H. Rajabi, M. Hadi Mosleh, T. Prakoso, N. Ghaemi, P. Mandal, A. Lea-Langton and M. Sedighi, Competitive adsorption of multicomponent volatile organic compounds on biochar, Chemosphere, 2021, 283, 131288 CrossRef CAS PubMed.
- H. Huang, F. Haghighat and P. Blondeau, Volatile organic compound (VOC) adsorption on material: influence of gas phase concentration, relative humidity and VOC type, Indoor Air, 2006, 16, 236–247 CrossRef CAS PubMed.
- Y.-H. Shih and P. M. Gschwend, Evaluating Activated Carbon−Water Sorption Coefficients of Organic Compounds Using a Linear Solvation Energy Relationship Approach and Sorbate Chemical Activities, Environ. Sci. Technol., 2009, 43, 851–857 CrossRef CAS PubMed.
- M. S. Li, S. C. Wu and Y. H. Shih, Characterization of volatile organic compound adsorption on multiwall carbon nanotubes under different levels of relative humidity using linear solvation energy relationship, J. Hazard. Mater., 2016, 315, 35–41 CrossRef CAS PubMed.
- L. Jia, J. Shi, C. Long, F. Lian and B. Xing, VOCs adsorption on activated carbon with initial water vapor contents: Adsorption mechanism and modified characteristic curves, Sci. Total Environ., 2020, 731, 139184 CrossRef CAS PubMed.
- S. Endo and K. U. Goss, Applications of polyparameter linear free energy relationships in environmental chemistry, Environ. Sci. Technol., 2014, 48, 12477–12491 CrossRef CAS PubMed.
- M. S. Li, R. Wang, D. T. Fu Kuo and Y. H. Shih, Linear free energy relationships for the adsorption of volatile organic compounds onto multiwalled carbon nanotubes at different relative humidities: comparison with organoclays and activated carbon, Environ. Sci.: Processes Impacts, 2017, 19, 276–287 RSC.
- Y. Suyadal, Deactivation model for desorption of tricholoroethylene vapor from granular activated carbon, Ind. Eng. Chem. Res., 2003, 42, 897–903 CrossRef CAS.
- R. P. P. L. Ribeiro, C. A. Grande and A. E. Rodrigues, Electric Swing Adsorption for Gas Separation and Purification: A Review, Sep. Sci. Technol., 2014, 49, 1985–2002 CrossRef CAS.
- A. Anfruns, M. J. Martin and M. A. Montes-Morán, Removal of odourous VOCs using sludge-based adsorbents, Chem. Eng. J., 2011, 166, 1022–1031 CrossRef CAS.
- C. Della Pina, M. A. De Gregorio, L. Clerici, P. Dellavedova and E. Falletta, Polyaniline (PANI): an innovative support for sampling and removal of VOCs in air matrices, J. Hazard. Mater., 2018, 344, 308–315 CrossRef CAS PubMed.
- S. W. Nahm, W. G. Shim, Y.-K. Park and S. C. Kim, Thermal and chemical regeneration of spent activated carbon and its adsorption property for toluene, Chem. Eng. J., 2012, 210, 500–509 CrossRef CAS.
- X. Li, C. Zheng, Z. Wang, H. Sui and L. He, Optimization of the loading patterns of silica gels for o-xylene recovery by vacuum swing adsorption, Can. J. Chem. Eng., 2021, 99, 458–466 CrossRef CAS.
- H. Sui, H. Liu, P. An, L. He, X. Li and S. Cong, Application of silica gel in removing high concentrations toluene vapor by adsorption and desorption process, J. Taiwan Inst. Chem. Eng., 2017, 74, 218–224 CrossRef CAS.
- J. Dai, C. Zhao, X. Hu, D. Chen, J. Yun, C. Liu, M. Zhong, D. Huang, C. Cen and Z. Chen, One-pot synthesis of meso-microporous ZSM-5 and its excellent performance in VOCs adsorption/desorption, J. Chem. Technol. Biotechnol., 2021, 96, 78–87 CrossRef CAS.
- Z. Han, S. Kong, H. Sui, X. Li and Z. Zhang, Preparation of Carbon-Silicon Doping Composite Adsorbent Material for Removal of VOCs, Materials, 2019, 12, 2438 CrossRef CAS PubMed.
- A. Mekki and B. Boukoussa, Structural, textural and toluene adsorption properties of microporous-mesoporous zeolite omega synthesized by different methods, J. Mater. Sci., 2019, 54, 8096–8107 CrossRef CAS.
- H. Liu, M. Xu, G. Li, W. Zhang and T. An, Solar-light-triggered regenerative adsorption removal of styrene by silver nanoparticles incorporated in metal–organic frameworks, Environ. Sci.: Nano, 2021, 8, 543–553 RSC.
- K.-J. Kim, C.-S. Kang, Y.-J. You, M.-C. Chung, M.-W. Woo, W.-J. Jeong, N.-C. Park and H.-G. Ahn, Adsorption–desorption characteristics of VOCs over impregnated activated carbons, Catal. Today, 2006, 111, 223–228 CrossRef CAS.
- M. Ghafari and J. D. Atkinson, Impact of styrenic polymer one-step hyper-cross-linking on volatile organic compound adsorption and desorption performance, J. Hazard. Mater., 2018, 351, 117–123 CrossRef CAS PubMed.
- H. Pan, M. Tian, H. Zhang, Y. Zhang and Q. Lin, Adsorption and Desorption Performance of Dichloromethane over Activated Carbons Modified by Metal Ions, J. Chem. Eng. Data, 2013, 58, 2449–2454 CrossRef CAS.
- X. Zhang, Y. Yang, X. Lv, Y. Wang, N. Liu, D. Chen and L. Cui, Adsorption/desorption kinetics and breakthrough of gaseous toluene for modified microporous-mesoporous UiO-66 metal organic framework, J. Hazard. Mater., 2019, 366, 140–150 CrossRef CAS PubMed.
- R. Li, T. Xue, Z. Li and Q. Wang, Hierarchical structure ZSM-5/SBA-15 composite with improved hydrophobicity for adsorption-desorption behavior of toluene, Chem. Eng. J., 2020, 392, 124861 CrossRef CAS.
- X. Zhang, W. Xiang, B. Wang, J. Fang, W. Zou, F. He, Y. Li, D. C. W. Tsang, Y. S. Ok and B. Gao, Adsorption of acetone and cyclohexane onto CO2 activated hydrochars, Chemosphere, 2020, 245, 125664 CrossRef CAS PubMed.
- K. Kosuge, S. Kubo, N. Kikukawa and M. Takemori, Effect of pore structure in mesoporous silicas on VOC dynamic adsorption/desorption performance, Langmuir, 2007, 23, 3095–3102 CrossRef CAS PubMed.
- M. J. Lashaki, Z. Hashisho, J. H. Phillips, D. Crompton, J. E. Anderson and M. Nichols, Mechanisms of heel buildup during cyclic adsorption-desorption of volatile organic compounds in a full-scale adsorber-desorber, Chem. Eng. J., 2020, 400, 124937 CrossRef.
- S. Kamravaei, P. Shariaty, M. J. Lashaki, J. D. Atkinson, Z. Hashisho, J. H. Phillips, J. E. Anderson and M. Nichols, Effect of beaded activated carbon fluidization on adsorption of volatile organic compounds, Ind. Eng. Chem. Res., 2017, 56, 1297–1305 CrossRef CAS.
- I. K. Shah, P. Pre and B. J. Alappat, Effect of thermal regeneration of spent activated carbon on volatile organic compound adsorption performances, J. Taiwan Inst. Chem. Eng., 2014, 45, 1733–1738 CrossRef CAS.
- F. J. Maldonado-Hodar, C. Moreno-Castilla, F. Carrasco-Marin and A. F. Perez-Cadenas, Reversible toluene adsorption on monolithic carbon aerogels, J. Hazard. Mater., 2007, 148, 548–552 CrossRef CAS PubMed.
- W. Zhang, Z. Qu, X. Li, Y. Wang, D. Ma and J. Wu, Comparison of dynamic adsorption/desorption characteristics of toluene on different porous materials, J. Environ. Sci., 2012, 24, 520–528 CrossRef CAS.
- J. Chen, C. Sun, Z. Huang, F. Qjn, H. Xu and W. Shen, Fabrication of functionalized porous silica nanocapsules with a hollow structure for high performance of toluene adsorption- desorption, ACS Omega, 2020, 5, 5805–5814 CrossRef CAS PubMed.
- Y. Yang, C. Sun, B. Lin and Q. Huang, Surface modified and activated waste bone char for rapid and efficient VOCs adsorption, Chemosphere, 2020, 256, 127054 CrossRef CAS PubMed.
- B. Lei, B. Liu, H. Zhang, L. Yam, H. Xie and G. Zhou, CuO-modified activated carbon for the improvement of toluene removal in air, J. Environ. Sci., 2020, 88, 122–132 CrossRef PubMed.
- R. Zaleski, A. Kierys and M. Gorgol, Positron insight into evolution of pore volume and penetration of the polymer network by n-heptane molecules in mesoporous XAD4, Phys. Chem. Chem. Phys., 2017, 19, 10009–10019 RSC.
- M. Popescu, J. P. Joly, J. Carré and C. Danatoiu, Dynamical adsorption and temperature-programmed desorption of VOCs (toluene, butyl acetate and butanol) on activated carbons, Carbon, 2003, 41, 739–748 CrossRef CAS.
- M. A. Alvarez-Merino, M. F. Ribeiro, J. M. Silva, F. Carrasco-Marín and F. J. Maldonado-Hódar, Activated Carbon and Tungsten Oxide Supported on Activated Carbon Catalysts for Toluene Catalytic Combustion, Environ. Sci. Technol., 2004, 38, 4664–4670 CrossRef CAS PubMed.
- J. Chen, G. Li, Y. Huang, H. Zhang, H. Zhao and T. An, Optimization synthesis of carbon nanotubes-anatase TiO2 composite photocatalyst by response surface methodology for photocatalytic degradation of gaseous styrene, Appl. Catal., B, 2012, 123, 69–77 CrossRef.
- T. An, J. Chen, X. Nie, G. Li, H. Zhang, X. Liu and H. Zhao, Synthesis of Carbon Nanotube-Anatase TiO2 Sub-micrometer-sized Sphere Composite Photocatalyst for Synergistic Degradation of Gaseous Styrene, ACS Appl. Mater. Interfaces, 2012, 4, 5988–5996 CrossRef CAS PubMed.
- J. Chen, H. Luo, H. Shi, G. Li and T. An, Anatase TiO2 nanoparticles–carbon nanotubes composite: Optimization synthesis and the relationship of photocatalytic degradation activity of acyclovir in water, Appl. Catal., A, 2014, 485, 188–195 CrossRef CAS.
- Z. He, L. Zhou, G. Li, X. Zeng, T. An, G. Sheng, J. Fu and Z. Bai, Comparative study of the eliminating of waste gas containing toluene in twin biotrickling filters packed with molecular sieve and polyurethane foam, J. Hazard. Mater., 2009, 167, 275–281 CrossRef CAS PubMed.
- Z. Liang, J. Wang, Y. Zhang, C. Han, S. Ma, J. Chen, G. Li and T. An, Removal of volatile organic compounds (VOCs) emitted from a textile dyeing wastewater treatment plant and the attenuation of respiratory health risks using a pilot-scale biofilter, J. Cleaner Prod., 2020, 253, 120019 CrossRef CAS.
- A. Aizpuru, L. Malhautier, J. C. Roux and J. L. Fanlo, Biofiltration of a mixture of volatile organic compounds on granular activated carbon, Biotechnol. Bioeng., 2003, 83, 479–488 CrossRef CAS PubMed.
- N. Xu, W. Fu, C. He, L. Cao, X. Liu, J. Zhao and H. Pan, Benzene Removal Using Non-thermal Plasma with CuO/AC Catalyst: Reaction Condition Optimization and Decomposition Mechanism, Plasma Chem. Plasma Process., 2014, 34, 1387–1402 CrossRef CAS.
- S. Sultana, A. Vandenbroucke, C. Leys, N. De Geyter and R. Morent, Abatement of VOCs with Alternate Adsorption and Plasma-Assisted Regeneration: A Review, Catalysts, 2015, 5, 718–746 CrossRef CAS.
- R. Liu, J. Chen, G. Li and T. An, Using an integrated decontamination technique to remove VOCs and attenuate health risks from an e-waste dismantling workshop, Chem. Eng. J., 2017, 318, 57–63 CrossRef CAS.
- Z.-G. He, J.-J. Li, J.-Y. Chen, Z.-P. Chen, G.-Y. Li, G.-P. Sun and T.-C. An, Treatment of organic waste gas in a paint plant by combined technique of biotrickling filtration with photocatalytic oxidation, Chem. Eng. J., 2012, 200–202, 645–653 CrossRef CAS.
- J. Chen, R. Liu, Y. Gao, G. Li and T. An, Preferential purification of oxygenated volatile organic compounds than monoaromatics emitted from paint spray booth and risk attenuation by the integrated decontamination technique, J. Cleaner Prod., 2017, 148, 268–275 CrossRef CAS.
- G. Li, Z. Zhang, H. Sun, J. Chen, T. An and B. Li, Pollution profiles, health risk of VOCs and biohazards emitted from municipal solid waste transfer station and elimination by an integrated biological-photocatalytic flow system: A pilot-scale investigation, J. Hazard. Mater., 2013, 250, 147–154 CrossRef PubMed.
- J. Y. Chen, Y. Huang, G. Y. Li, T. C. An, Y. K. Hu and Y. L. Li, VOCs elimination and health risk reduction in e-waste dismantling workshop using integrated techniques of electrostatic precipitation with advanced oxidation technologies, J. Hazard. Mater., 2016, 302, 395–403 CrossRef CAS PubMed.
- L. Li, C. Zhang, H. He and J. Liu, An integrated system of biological and catalytic oxidation for the removal of o-xylene from exhaust, Catal. Today, 2007, 126, 338–344 CrossRef CAS.
- Q. Dai, J. Wu, W. Deng, J. Hu, Q. Wu, L. Guo, W. Sun, W. Zhan and X. Wang, Comparative studies of P/CeO2 and Ru/CeO2 catalysts for catalytic combustion of dichloromethane: From effects of H2O to distribution of chlorinated by-products, Appl. Catal., B, 2019, 249, 9–18 CrossRef CAS.
- C. Zhou, K. Zhou, H. Li, X. Xu, B. Liu, H. Li, Z. Zeng, W. Ma and L. Li, Pressure swing adsorption properties of activated carbon for methanol, acetone and toluene, Chem. Eng. J., 2021, 413, 127384 CrossRef CAS.
- Z. Hashisho, M. Rood and L. Botich, Microwave-Swing Adsorption To Capture and Recover Vapors from Air Streams with Activated Carbon Fiber Cloth, Environ. Sci. Technol., 2005, 39, 6851–6859 CrossRef CAS PubMed.
- D. W. Price and P. S. Schmidt, VOC Recovery through Microwave Regeneration of Adsorbents: Process Design Studies, J. Air Waste Manage. Assoc., 1998, 48, 1135–1145 CrossRef CAS PubMed.
- X. Wang, R. Daniels and R. W. Baker, Recovery of VOCs from high-volume, low-VOC-concentration air streams, AIChE J., 2001, 47, 1094–1100 CrossRef CAS.
- S. G. Ramalingam, J. Saussac, P. Pre, S. Giraudet, L. Le Coq, P. Le Cloirec, S. Nicolas, O. Baudouin, S. Dechelotte and A. Medevielle, Hazardous dichloromethane recovery in combined temperature and vacuum pressure swing adsorption process, J. Hazard. Mater., 2011, 198, 95–102 CrossRef CAS PubMed.
- Z. Han, D. Wang, P. Jiang, H. Sui, L. He and X. Li, Enhanced removal and recovery of binary mixture of n-butyl acetate and p-xylene by temperature swing–Vacuum pressure swing hybrid adsorption process, Process Saf. Environ. Prot., 2020, 135, 273–281 CrossRef CAS.
- B. Ambrożek and K. Zwarycz-Makles, Theoretical and experimental studies of the recovery of volatile organic compounds from waste air streams in the thermal swing adsorption system with closed-loop regeneration of adsorbent, Energy Convers. Manage., 2014, 85, 646–654 CrossRef.
- L. Zhou, Advance of Volatile Organic Compounds ( VOCs) Adsorption Recycling Technology, Guangzhou, Chem. & Ind., 2016, 44, 6–7+15 CAS.
- Z. Li and F. Luo, Analysis of the Treatment Technology Pathway of VOCs Released from Oven, Huan Jing Ke Xue, 2021, 32, 3685–3688 Search PubMed.
- K. Ohlrogge, K. V. Peinemann, J. Wind and R. D. Behling, The Separation of Hydrocarbon Vapors with Membranes, Sep. Sci. Technol., 1990, 25, 1375–1386 CrossRef CAS.
- J. Wang, J. Chen and J. Cao, Analysis of Membrane based hydrocarbon VOCs recovery technique at petrol stations, Mo Kexue Yu Jishu, 2009, 29, 93–98 CAS.
- Y. Shen, Application of membrane process in BTX recovery from oil vapour, Huagong Huanbao, 2021, 41, 382–387 Search PubMed.
- W. M. Choi, S. H. Cho, S. T. Kim, C. S. Lee and S. Y. Nam, Field Application of the Membrane System for the Recovery of VOCs from the Automobile Painting Process, Membr. J., 2013, 23, 129–135 Search PubMed.
Footnote |
† Electronic supplementary information (ESI) available. See DOI: 10.1039/d1en00929j |
|
This journal is © The Royal Society of Chemistry 2022 |