Copper-catalyzed Goldberg-type C–N coupling in deep eutectic solvents (DESs) and water under aerobic conditions†‡
Received
15th December 2020
, Accepted 29th January 2021
First published on 29th January 2021
Abstract
An efficient and selective N-functionalization of amides is first reported via a CuI-catalyzed Goldberg-type C–N coupling reaction between aryl iodides and primary/secondary amides run either in Deep Eutectic Solvents (DESs) or water as sustainable reaction media, under mild and bench-type reaction conditions (absence of protecting atmosphere). Higher activities were observed in an aqueous medium, though the employment of DESs expanded and improved the scope of the reaction to include also aliphatic amides. Additional valuable features of the reported protocol include: (i) the possibility to scale up the reaction without any erosion of the yield/reaction time; (ii) the recyclability of both the catalyst and the eutectic solvent up to 4 consecutive runs; and (iii) the feasibility of the proposed catalytic system for the synthesis of biologically active molecules.
1. Introduction
The search for organic transformations catalyzed by first-row, cheap and earth-crust abundant transition metals is nowadays playing a pivotal role in synthetic organic chemistry.1 In this sense and within the coinage metals group (Cu, Ag and Au),2 copper occupies a prominent and strategic position as it: (i) is an essential metal in biology3 and presents moderate toxicity;4 (ii) displays a versatile reactivity with rich and diverse redox chemistry [possibility to access different oxidations states: Cu(0); Cu(I); Cu(II) or even Cu(III)];5 (iii) can allocate in its coordination sphere either soft (containing P- and S- donor atoms) as Cu(I) or hard donor ligands (like O and N) as Cu(II); and (iv) can participate in both σ- or π-interactions with unsaturated organic substrates (alkenes or alkynes). Thus, it is not surprising to find a plethora of applications for Cu-based catalysts in several hot-topic areas of catalysis spanning cross-coupling reactions6 and asymmetric synthesis.7 As for C–N bond formations,8 typical Cu-catalyzed cross-coupling processes like the Ullmann9 or the Goldberg10 reactions are reputed by organic chemists as key and valuable synthetic tools for the formation of C–N bonds either in academic or in industrial laboratories all over the world,8 especially considering the ongoing depletion of petroleum reserves and the severe environmental problems associate with their use. Thus, the employment of sustainable reaction media in organic synthesis is today highly sought after in order to fulfill one of the most important Principles of Green Chemistry.11 In this context, it is worth mentioning that 80–90% of the mass balance of chemical reactions is directly related with the volatile organic solvents (VOCs) employed, either used as a bulk reaction medium or in the usually required isolation/purification steps.12
In this vein, we have recently reported on a variety of highly selective and effective transition-metal-catalyzed organic transformations promoted by Ru,13 Au,14 or Pd15 complexes, which have been performed in a new family of sustainable reaction media, that is the so-called Deep Eutectic Solvents (DESs).16 These environmentally friendly and tunable eutectic mixtures can be obtained by mixing and heating two (binary DESs) or even three (ternary DESs) components that are amenable to interact through a tridimensional hydrogen-bond-type network, which is established between hydrogen-bond-acceptor components [HBAs, usually biorenewable quaternary ammonium salts like choline chloride (ChCl)17 (A, Fig. 1)] and hydrogen-bond-donors [HBDs, typically natural polyols (like glycerol (Gly), ethylene glycol (EG), sugars), urea derivatives and biorenewable carboxylic acids (e.g., oxalic, malonic acid (B, Fig. 1)].18 At this point, it is important to remark that DESs have been today used as privileged sustainable solvents in a wide variety of chemical applications.19
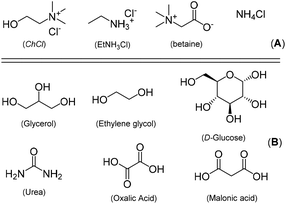 |
| Fig. 1 Hydrogen-bond-acceptors (HBAs, A) and hydrogen-bond-donors (HBDs, B) usually employed in the synthesis of sustainable Deep Eutectic Solvents (DESs). | |
Despite the large number of Pd-catalyzed C–C coupling reactions successfully run in different eutectic mixtures (e.g., Sukuzi, Heck, Stille, Sonogashira and Hiyama-type couplings),15c,e–h,20 there are only few examples, independently reported by Shaabani21 and Capriati22 research groups, which illustrate the possibility to perform the Cu-catalyzed Ullman-type C–N coupling between aryl halides and primary or secondary amines in sustainable eutectic mixtures. Conversely, as far as we are aware, the corresponding counterpart studies on the Cu-catalyzed Goldberg-type C–N coupling in DESs have been totally neglected.23 Accordingly, we decided to fill this gap by studying the C–N coupling reaction of aryl halides with either aromatic or primary and secondary aliphatic amides (Scheme 1): (i) in different DESs or water as sustainable solvents; (ii) under mild and bench-type reaction conditions (presence of air and lower reaction temperature); and (iii) using different bases (KOH or tBuOK) and bidentate ligands (like ethylene diamine, glycine, L-proline, bipyridine). The robustness of the proposed methodology was further highlighted by successful recycling studies of the catalytic system and its application in drug synthesis.
 |
| Scheme 1 CuI-Catalyzed Goldberg-type C–N coupling of aryl iodides with primary/secondary amides under air in DES or in water. | |
2. Results and discussion
We started our investigation by exploring sustainable reaction media (DESs or water) useful to design a Cu-catalyzed Goldberg-type C–N coupling under milder and bench-type reaction conditions. We elected to study, as a model reaction, the CuI-catalyzed (10 mol%) coupling of 4-iodotoluene (1a) with 2-methylbenzamide (2a) in the archetypical eutectic mixture 1ChCl/2Gly, in the absence of protecting atmosphere (under air) and at 80 °C. Building on previously reported studies on Goldberg-type C–N couplings,23 we decided to use ethylene diamine (L1) (10 mol%) and tBuOK (3 equiv.) as a base. After 12 h reaction time, we observed the formation of the desired secondary amide 3a in moderate yield (66%), but with a complete chemoselectivity. No side products were observed in the crude reaction mixture aside from unreacted starting materials (1a and 2a) (Table 1, entry 1). At this point, it is important to note the significant effect of the temperature on the outcome of the catalytic reaction. Indeed, the yield of 3a dropped to 41% when the temperature was lowered down to 60 °C (Table 1, entry 2). On the other hand, enhancement of the reaction temperature up to 100 °C only produced a moderate increase of the yield of 3a (72%, Table 1, entry 3). We also found that the suppression of either the external base (Table 1, entry 4) or the bidentate ligand ethylene diamine (L1) (Table 1, entry 5) produced a loss of the catalytic activity or a dramatic decrease of the 3a yield, respectively. Finally, in accordance with previous studies on transition-metal-catalyzed organic reaction in ChCl-based eutectic mixtures,14 the Goldberg-type C–N coupling was similarly found to proceed at higher rates in the 1ChCl/2Gly eutectic mixture than in pure glycerol (Table 1, entry 6), thus highlighting the positive effect of this quaternary ammonium salt in the catalytic procedure when synergistically combined with Gly.
Table 1 CuI-Catalyzed Goldberg type C–N coupling between 4-iodotoluene (1a) and 2-methylbenzamide (2a) under air and at 80 °C in different sustainable solvents (DESs or water)a

|
Entry |
X
|
Solvent |
T (°C) |
Ligandb |
Base (%) |
Yieldc (%) |
General conditions: Reactions performed under air, at 60–100 °C using 0.5 mmol of 1a and 2a in 1 mL of the desired solvent.
L1 = ethylene diamine; L2 = N,N-dimethylethylenediamine; L3 = glycine; L4 = L-proline; L5 = 2,2′-bipyridine; L6 = (1R,2S)-cyclopentane-1,2-diamine; L7 = (1R,2S)-cyclohexane-1,2-diamine; L8 = benzene-1,2-diamine; L9 = (1R,2S)-2,3-dihydro-1H-indene-1,2-diamine.
Yield determined by HPLC.
|
1 |
I |
1ChCl/2Gly |
80 |
L1 |
t
BuOK |
66 |
2 |
I |
1ChCl/2Gly |
60 |
L1 |
t
BuOK |
41 |
3 |
I |
1ChCl/2Gly |
100 |
L1 |
t
BuOK |
72 |
4 |
I |
1ChCl/2Gly |
80 |
L1 |
— |
0 |
5 |
I |
1ChCl/2Gly |
80 |
— |
t
BuOK |
10 |
6 |
I |
Gly |
80 |
L1 |
t
BuOK |
51 |
7 |
I |
1ChCl/2Urea |
80 |
L1 |
t
BuOK |
74 |
8 |
I |
1ChCl/2Urea |
80 |
L1 |
— |
0 |
9 |
I |
1ChCl/2Urea |
80 |
L2 |
t
BuOK |
72 |
10 |
I |
1ChCl/2Urea |
80 |
L3 |
t
BuOK |
0 |
11 |
I |
1ChCl/2Urea |
80 |
L4 |
t
BuOK |
9 |
12 |
I |
1ChCl/2Urea |
80 |
L5 |
t
BuOK |
20 |
13 |
I |
1ChCl/2Urea |
80 |
L6 |
t
BuOK |
27 |
14 |
I |
1ChCl/2Urea |
80 |
L7 |
t
BuOK |
37 |
15 |
I |
1ChCl/2Urea |
80 |
L8 |
t
BuOK |
2 |
16 |
I |
1ChCl/2Urea |
80 |
L9 |
t
BuOK |
10 |
17 |
Br |
1ChCl/2Urea |
80 |
L1 |
t
BuOK |
8 |
18 |
Cl |
1ChCl/2Urea |
80 |
L1 |
t
BuOK |
0 |
19 |
I |
1Bet/1Urea |
80 |
L1 |
t
BuOK |
60 |
20 |
I |
1ChCl/2H2O |
80 |
L1 |
t
BuOK |
80 |
21 |
I |
H2O |
80 |
L1 |
t
BuOK |
85 |
22 |
I |
H2O |
80 |
L1 |
KOH |
92 |
23 |
I |
H2O |
80 |
L1 |
Cs2CO3 |
0 |
24 |
I |
H2O |
80 |
L1 |
K2CO3 |
11 |
25 |
I |
H2O |
80 |
L1 |
K3PO4 |
4 |
Next, we decided to study the effect of different HBDs forming the eutectic mixture on the outcome of the catalytic reaction. To this end, we firstly replaced the natural polyol Gly by urea to form the so-called eutectic mixture reline (1ChCl/2Urea).18 Under the aforementioned conditions (80 °C, 10 mol% of CuI, 10 mol% of L1, 3 equiv. of tBuOK, under air), we observed an increase of the yield of 3a (74%), while maintaining the total chemoselectivity of the reaction (Table 1, entry 7). Probably, this result could be related to the enhanced basic character of the eutectic mixture 1ChCl/2Urea when compared with 1ChCl/2Gly.24 At this point, we explored: (i) the possibility to run the reaction in the absence of base, but no reaction was observed (Table 1, entry 8); (ii) the effect of different chelating ligands (10 mol% of L1–L9), with the best result obtained by the ethylene diamine (Table 1, entries 9–16); (iii) the influence of the halogen atom present in the aromatic coupling partner (4-Br- or 4-Cl-toluene), observing a decrease in the yield of the process (Table 1, entries 17 and 18); and (iv) the employment of a different HBA, detecting a decrease of the catalytic activity when using betaine (Bet, Table 1, entry 19). To finish with the parametrization studies on the role of HBD of the eutectic mixture, we decided to extend our studies to the prototypical HBD present in nature, that is water, thus using the eutectic mixture 1ChCl/2H2O as a solvent. In this case, we found an increased catalytic activity (80%; Table 1, entry 20). This enhancement could even be improved using bulk water as the solvent (85%; Table 1, entry 21). Finally, a yield as high as 92% could be achieved when switching from tBuOK to KOH (Table 1, entry 22), while the use of carbonate- or phosphate-containing inorganic bases considerably decreased the activity of the catalytic system (Table 1, entries 23–25). At this point, it is worth noting that a similar yield (90%) was achieved also in 1ChCl/2H2O by performing the coupling reaction on a 2.5 mmol scale (Scheme 2).25
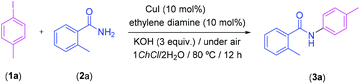 |
| Scheme 2 CuI-Catalyzed Goldberg type C–N coupling between 4-iodotoluene (1a) and 2-methylbenzamide (2a) for the scaled-up synthesis of 3a (reaction performed on a 2.5 mmol scale). | |
Since the use of water was associated to the best catalytic performance, we decided to expand the scope of this reaction to the coupling of a library of amides 2a–e (aliphatic/aromatic, secondary/primary amides containing either electron-donor or electron-withdrawing groups) with a variety of substituted aryl iodides (1a–c), under the previously optimized catalytic conditions (80 °C, 10 mol% of CuI, 10 mol% of ethylene diamine, 3 equiv. of KOH and under air; see Table 2). As for aromatic amides, the presence of electron-donating substituents in the aromatic ring, like methyl (2a) or methoxy (2b) groups, gave rise to the corresponding secondary amides 3a–e in good to quantitative yields (81–99%, Table 2, entries 1–5) when 4-iodotoluene (1a), 4-iodobenzene (1b) or 3-iodoanisole (1c) were employed as coupling partners. However, the presence of electron-withdrawing substituents in the aromatic ring of the amide, like in the case of 2-fluorobenzamide (2c), produced a slight erosion in the yield of the corresponding secondary amides 3f,g (64–74%; Table 2, entries 6 and 7). This catalytic system also tolerates the use of secondary amides, like N-methylbenzamide (2d) giving rise to the expected tertiary amide 3h in good yield (70%; Table 2, entry 8). One of the main drawbacks of our aqueous catalytic system is related to the fact that aliphatic amides proved to be totally unreactive. Pleasantly, switching water for the 1ChCl/2H2O eutectic mixture, we observed moderate to good coupling yields (up to 99%) of aliphatic propionamide (2e) with iodo-aryl partners containing different substituents [4-Me (1a) or 3-MeO (1c); Table 2, entries 9–11). Finally, in order to promote an efficient mass transfer in the synthesis of compounds 3g, 3j and 3k (Table 2, entries 7,10 and 11), we performed the coupling reaction under ultrasonic irradiation using a conventional ultrasound bath operating at 35 kHz and 160 W.26 However, the use of these new reaction conditions did not improve the yield/reaction times previously observed in any of the studied cases.
Table 2 CuI-Catalyzed Goldberg type C–N coupling between different aryl iodides (1a–c) and several aromatic/aliphatic amides (2a–e), in water or 1ChCl/2H2O, under air and at 80 °Ca
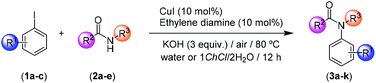
|
Entry |
R
1
|
R
2/R3 |
Solvent |
Yieldb (%) |
General conditions: Reactions performed under air, at 80 °C using 0.5 mmol of 1a–c and 2a–e and 3 equiv. of KOH in 1 mL of the desired solvent.
Yield determined by HPLC. Isolated yields after column chromatography in brackets.
|
1 |
4-Me (1a) |
2-Me-Ph/H (2a) |
H2O |
92 (85, 3a) |
2 |
H (1b) |
2-Me-Ph/H (2a) |
H2O |
95 (90, 3b) |
3 |
3-OMe (1c) |
2-Me-Ph/H (2a) |
H2O |
81 (74, 3c) |
4 |
4-Me (1a) |
3-MeO-Ph/H (2b) |
H2O |
88 (83, 3d) |
5 |
H (1b) |
3-MeO-Ph/H (2b) |
H2O |
99 (95, 3e) |
6 |
H (1b) |
2-F-Ph/H (2c) |
H2O |
74 (68, 3f) |
7 |
4-Me (1a) |
2-F-Ph/H (2c) |
H2O |
64 (57, 3g) |
8 |
H (1b)) |
Ph/Me (2d) |
H2O |
70 (65, 3h) |
9 |
4-Me (1a) |
Et/H (2e) |
1ChCl/2H2O |
99 (92, 3i) |
10 |
H (1b) |
Et/H (2e) |
1ChCl/2H2O |
62 (60, 3j) |
11 |
3-OMe (1c) |
Et/H (2e) |
1ChCl/2H2O |
45 (42, 3k) |
In the framework of a sustainable catalysis,27 the lifetime of a catalytic system and its level of reusability are key factors that could improve the environmental footprint of a given catalytic system.28 In this sense, and taking into account the feasibility of recycling eutectic mixtures in metal-catalyzed organic reactions,16e we decided to investigate the reusability of our catalytic system taking, as a model reaction, the CuI-catalyzed Goldberg-type coupling between iodobenzene (1b) and 2-methylbenzamide (2a), at 80 °C, in the presence of ethylene diamine (10 mol%) as a ligand and KOH (3 equiv.) as a base, under air and using the eutectic mixture 1ChCl/2H2O as a reaction medium (Scheme 3). Under these conditions, the desired amide 3b was isolated in 87% yield after 12 h reaction time. Subsequent extraction of the resulting crude with a sustainable green solvent like cyclopentyl methyl ether (CPME)29 and concomitant addition of fresh reagents, allowed us to recycle the catalyst and the DES successfully up to three consecutive runs with only a negligible erosion of the final yield of 3b (Scheme 3). In order to provide metrics to support the “greenness” profile of the reported methodology, we have calculated the Sheldon's environmental factor (E-factor) (total mass of waste/mass of product),30 finding a value of 15,31 which is within the recommended range for fine chemicals (5–50).30
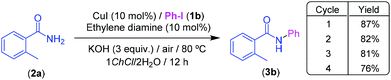 |
| Scheme 3 CuI-catalyzed Goldberg type C–N coupling between iodobenzene (1b) and 2-methylbenzamide (2a) under air and at 80 °C in the eutectic mixture 1ChCl/2H2O: recycling studies. | |
Finally, we decided to apply our methodology to the synthesis of biologically active molecules. Our target was 4-chloro-N-(4-methoxyphenyl)benzamide (3l), which belongs to the N-arylbenzamide class of cyclooxygenase (COX-1) selective inhibitors (Scheme 4).32 We envisaged as a key step to access this secondary amide, a CuI-catalyzed Goldberg-type C–N coupling between 4-iodoanisole (1e) and 4-chlorobenzamide (2f), under the previously optimized reaction conditions in the 1ChCl/2H2O eutectic mixture (Scheme 4), finding that the desired secondary amide 3l chemoselectively formed in a 87% yield. As a final point, it is worth mentioning that the biologically active compound 3l was isolated in the same yield (87%) when working on a 2.5 mmol scale.
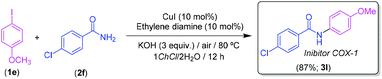 |
| Scheme 4 Synthesis of the biologically active aromatic secondary amide 3l through CuI-catalyzed Goldberg type C–N coupling between 4-iodoanisole (1e) and 4-chlorobenzamide (2f), under air and at 80 °C in the eutectic mixture 1ChCl/2H2O. | |
3. Conclusions
In summary, we have developed the first Cu-catalyzed Goldberg-type C–N coupling working in ChCl-based deep eutectic solvents and water. The utilization of these sustainable reaction media allowed us to carry out this coupling process: (i) under milder reaction conditions (80 °C; temperatures as high as 110 °C are usually required); (ii) employing bench-type settings (presence of air); and (iii) with high chemoselectivity, as a variety of coupling partners with different electron-donating or electron-withdrawing substituents are tolerated. The inactivity of the catalytic system in water when using aliphatic amides was overcome upon simple switching of water for a water-based eutectic mixture, that is 1ChCl/2H2O.
Furthermore, we demonstrated: (i) the possibility to scale up the reaction without observing any erosion of the yield/reaction time; (ii) the successful recycle of both the catalyst and the eutectic solvent up to 4 consecutive runs; and (iii) the competence of our catalytic system for the synthesis of biologically active organic molecules. Overall, this methodology provides a synthetic tool for the efficient, sustainable and selective formation of C–N bonds, which is an important endeavour in the field of metal-catalyzed organic transformations.
Conflicts of interest
There are no conflicts to declare.
Acknowledgements
L. C., J. A. H. -F., M. R. -M. and J. G. A thanks the Spanish MINECO (Project CTQ2016-75986-P and CTQ2016-81797-REDC). M. R. -M. and A. P. S are indebted to Spanish MINECO (Project CTQ2014-56345-P, CTQ2017-88357-P, and RYC-2012-09800), and Gobierno del Principado de Asturias (FICYT, Project FC-15-GRUPIN14-106) for financial support. M. R. -M. thanks “Vicerrectorado de Investigación” from the University of Oviedo for the award of a predoctoral grant through the “Plan de Apoyo y Promoción de la Investigación, Universidad de Oviedo”. A. P. S. is also grateful to the COST action Smart Inorganic Polymers (SIPs-CM1302—http://www.sips-cost.org/home/index.html), and Spanish MEC for the Juan de la Cierva and Ramón y Cajal programs. J. G. -A. thanks: (i) the Fundación BBVA for the award of a “Beca Leonardo a Investigadores y Creadores Culturales 2017”;33 and (ii) PhosAgro/UNESCO/IUPAC for the award of a “Green Chemistry for Life Grant”. This work was also carried out under the framework of the projects: (a) “Development of Sustainable Synthetic Processes in Unconventional Solvents for the Preparation of Molecules of Pharmaceutical Interest” realized with the contribution of Fondazione Puglia, which is gratefully acknowledged by L. C. and V. C; and (b) “Unlocking Sustainable Technologies Through Nature-inspired Solvents (NATUREChem) (grant number: 2017A5HXFC_002)” financially supported by the Ministero dell'Università e della Ricerca (MUR-PRIN).
Notes and references
-
(a) B. Su, Z.-C. Cao and Z.-J. Shi, Acc. Chem. Res., 2015, 48, 886 CrossRef CAS;
(b) J. Miao and H. Ge, Eur. J. Org. Chem., 2015, 7859 CrossRef CAS;
(c) M. D. Kärkäs and B. Åkermark, Dalton Trans., 2016, 45, 14421 RSC;
(d) D. Wang and D. Astruc, Chem. Soc. Rev., 2017, 46, 816 RSC;
(e) J. V. Obligacion and P. J. Chirik, Nat. Rev. Chem., 2018, 2, 15 CrossRef CAS.
-
(a) B. Lipshutz and Y. Yoshinori, Chem. Rev., 2008, 108, 2793 CrossRef CAS;
(b) A. M. Echavarren, N. Jiao and V. Gevorgyan, Chem. Soc. Rev., 2016, 45, 4445 RSC;
(c)
H. V. Huynh, Group 11 Metal–NHC Complexes, in The Organometallic Chemistry of N–Heterocyclic Carbenes, ed. H. V. Huynh, John Wiley & Sons Ltd, Hoboken, USA, 2017 CrossRef.
- R. A. Festa and D. J. Thiele, Curr. Biol., 2011, 21, R877 CrossRef CAS.
- With exclusion of iron, copper is considered the less toxic 1st row transition metal:
(a) A. J. Hickman and M. S. Sanford, Nature, 2012, 484, 177 CrossRef CAS;
(b) S. D. McCann and S. S. Stahl, Acc. Chem. Res., 2015, 48, 1756 CrossRef CAS.
- Cu catalysts can trigger either two-electron or radical-promoted transformations, see: R. Trammell, K. Rajabimoghadam and I. García-Bosch, Chem. Rev., 2019, 119, 2954 CrossRef CAS.
-
Copper-Mediated Cross-Coupling Reactions, ed. G. Evano and N. Blanchard, John Wiley & Sons Ltd, Hoboken, USA, 2014 Search PubMed.
-
Copper Catalyzed Asymmetric Synthesis, ed. A. Alexakis, N. Krause and S. Woodward, Wiley–VCH Verlag GmbH & Co. KgaA, Weinheim, Germany, 2014 Search PubMed.
- The transition-metal-catalyzed C–N bond formation allows the construction of a wide variety of N-containing building blocks with a plethora of applications ranging from pharmaceutical and agrochemical to chemistry of natural products and material sciences. For outstanding book chapters and reviews on C–N couplings, see:
(a)
A. R. Muci and S. L. Buchwald, Practical Palladium Catalysts for C–N and C–O Bond Formation, in Cross-Coupling Reactions, Topics in Current Chemistry, ed. N. Miyaura, vol 219, pp. 131–209, Springer, Berlin, Heidelberg, Germany, 2002 Search PubMed;
(b)
L. Jiang and S. L. Buchwald, Palladium–Catalyzed Aromatic Carbon–Nitrogen Bond Formation, in Metal–Catalyzed Cross–Coupling Reactions, ed A. de Meijere and F. Diederich, Wiley–VCH Verlag GmbH & Co. KgaA, Weinheim, Germany, 2004 Search PubMed;
(c) J. Bariwal and E. Van der Eycken, Chem. Soc. Rev., 2013, 42, 9283 RSC;
(d)
Amination and Formation of sp2 C–N Bonds, ed. M. Taillefer and D. Ma, Springer, New York, USA, 2014 Search PubMed;
(e) P. Ruiz-Castillo and S. L. Buchwald, Chem. Rev., 2016, 116, 12564 CrossRef CAS;
(f) J. Luo and W.-T. Wei, Adv. Synth. Catal., 2018, 360, 2076 CrossRef CAS.
-
(a) F. Ullmann, Ber. Dtsch. Chem. Ges., 1903, 36, 2382 CrossRef;
(b) F. Ullmann and P. Sponagel, Ber. Dtsch. Chem. Ges., 1905, 38, 2211 CrossRef CAS.
- I. Goldberg, Ber. Dtsch. Chem. Ges., 1906, 39, 1691 CrossRef.
-
(a)
P. T. Anastas and J. C. Warner, Green Chemistry Theory and Practice, Oxford University Press, Oxford, UK, 1998 Search PubMed;
(b)
A. S. Matlack, Introduction to Green Chemistry, Marcel Dekker, New York, USA, 2001 Search PubMed;
(c) M. Poliakoff, J. M. Fitzpatrick, T. R. Farren and P. T. Anastas, Science, 2002, 297, 807 CrossRef CAS;
(d)
M. Lancaster, Green Chemistry: An Introductory Text, RSC Publishing, Cambridge, UK, 2002 Search PubMed;
(e)
R. A. Sheldon, I. Arends and U. Henefeld, Green Chemistry and Catalysis, Wiley-VCH, Weinheim, Germany, 2007 CrossRef.
-
(a) D. J. C. Constable, C. Jiménez-González and R. K. Henderson, Org. Process Res. Dev., 2007, 11, 133 CrossRef CAS;
(b) J. H. Clark and S. J. Tavener, Org. Process Res. Dev., 2007, 11, 149 CrossRef CAS;
(c) P. G. Jessop, Green Chem., 2011, 13, 1391 RSC;
(d) T. Laird, Org. Process Res. Dev., 2012, 16, 1 CrossRef CAS;
(e) B. H. Lipshutz, Chem, 2018, 4, 2004 CrossRef CAS.
-
(a) C. Vidal, F. J. Suárez and J. García-Álvarez, Catal. Commun., 2014, 44, 76 CrossRef CAS;
(b) L. Cicco, M. J. Rodríguez-Álvarez, F. M. Perna, J. García-Álvarez and V. Capriati, Green Chem., 2017, 19, 3069 RSC;
(c) L. Cicco, N. Ríos-Lombardía, M. J. Rodríguez-Álvarez, F. Morís, F. M. Perna, V. Capriati, J. García-Álvarez and J. González-Sabín, Green Chem., 2018, 20, 3468 RSC;
(d) N. Ríos-Lombardía, M. J. Rodríguez-Álvarez, F. Moris, R. Kourist, N. Comino, F. López-Gallego, J. González-Sabín and J. García-Álvarez, Front. Chem., 2020, 8, 139 CrossRef.
-
(a) M. J. Rodríguez-Álvarez, C. Vidal, J. Díez and J. García-Álvarez, Chem. Commun., 2014, 50, 12927 RSC;
(b) C. Vidal, L. Merz and J. García-Álvarez, Green Chem., 2015, 17, 3870 RSC;
(c) M. J. Rodríguez-Álvarez, C. Vidal, S. Schumacher, J. Borge and J. García-Álvarez, Chem. – Eur. J., 2017, 23, 3425 CrossRef.
-
(a) R. Mancuso, A. Maner, L. Cicco, F. M. Perna and V. Capriati, Tetrahedron, 2016, 72, 4239 CrossRef CAS;
(b) F. Messa, S. Perrone, M. Capua, F. Tolomeo, L. Troisi, V. Capriati and A. Salomone, Chem. Commun., 2018, 54, 8100 RSC;
(c) G. Dilauro, S. M. García, D. Tagarelli, P. Vitale, F. M. Perna and V. Capriati, ChemSusChem, 2018, 11, 3495 CrossRef CAS;
(d) B. Saavedra, J. M. Pérez, M. J. Rodríguez-Álvarez, J. García-Álvarez and D. J. Ramón, Green Chem., 2018, 20, 2151 RSC;
(e) S. Ghinato, G. Dilauro, F. M. Perna, V. Capriati, M. Blangetti and C. Prandi, Chem. Commun., 2019, 55, 7741 RSC;
(f) G. Dilauro, A. F. Quivelli, P. Vitale, V. Capriati and F. M. Perna, Angew. Chem., Int. Ed., 2019, 58, 1799 CrossRef CAS;
(g) F. Messa, G. Dilauro, F. M. Perna, P. Vitale, V. Capriati and A. Salomone, ChemCatChem, 2020, 12, 1979 CrossRef CAS;
(h) V. Pelliccioli, G. Dilauro, S. Grecchi, S. Arnaboldi, C. Graiff, F. M. Perna, P. Vitale, E. Licandro, A. Aliprandi, S. Cauteruccio and V. Capriati, Eur. J. Org. Chem., 2020, 6981 CrossRef CAS.
- For general reviews or book chapters that cover the use of Deep Eutectic Solvents (DESs) in transition-metal-catalyzed organic transformations see:
(a) J. García-Álvarez, Eur. J. Inorg. Chem., 2015, 5147 CrossRef;
(b) J. García-Álvarez, E. Hevia and V. Capriati, Eur. J. Org. Chem., 2015, 6779 CrossRef;
(c) P. Liu, J.-W. Hao, L.-P. Mo and Z.-H. Zhang, RSC Adv., 2015, 5, 48675 RSC;
(d) D. A. Alonso, A. Baeza, R. Chinchilla, G. Guillena, I. M. Pastor and D. J. Ramón, Eur. J. Org. Chem., 2016, 612 CrossRef CAS;
(e)
C. Vidal and J. García-Álvarez, Metal–Promoted Organic Transformation in DES, in Deep Eutectic Solvents: Synthesis, Properties, and Applications, ed. D. J. Ramón and G. Guillena, Wiley-VCH, Weinheim, Germany, 2019 Search PubMed.
- (2-Hydroxyethyl)trimethylammonium chloride (choline chloride, ChCl), which is produced in million of tons/year, has been described as an essential micro- and human nutrient within the Recommended Dietary Allowance (RDA) of 50 mg: J. K. Blusztajn, Science, 1998, 284, 794 CrossRef.
- The term Deep Eutectic Solvent (DES) was firstly coined by A. P. Abbott et al. to describe the eutectic mixture formed by mixing choline chloride (ChCl) with urea in a 1
:
2 molar ratio (1ChCl/2Urea). This eutectic mixture (reline) is liquid at room temperature (melting point 12 °C), while its isolated solid components have higher melting points (urea: 133 °C; ChCl: 302 °C): A. P. Abbott, G. Capper, D. L. Davies, R. K. Rasheed and V. Tambyrajah, Chem. Commun., 2003, 70 RSC.
- For general reviews and books dealing with the use of Deep Eutectic Solvents as sustainable reaction media in different fields of chemistry, see:
(a) A. P. Abbott, R. C. Harris, K. Ryder, C. d'Agostino, L. Gladden and M. D. Mantle, Green Chem., 2011, 13, 82 RSC;
(b) C. Ruß and B. König, Green Chem., 2012, 14, 2969 RSC;
(c) Q. Zhang, K. de Oliveira Vigier, S. Royer and F. Jérôme, Chem. Soc. Rev., 2012, 41, 7108 RSC;
(d) D. Carriazo, M. C. Serrano, M. C. Gutiérrez, M. L. Ferrer and F. Del Monte, Chem. Soc. Rev., 2012, 41, 4996 RSC;
(e) M. Francisco, A. van den Bruinhorst and M. C. Kroon, Angew. Chem., Int. Ed., 2013, 52, 3074 CrossRef CAS;
(f) G. Yu and F. Jérôme, Chem. Soc. Rev., 2013, 42, 9550 RSC;
(g) A. Paiva, R. Craveiro, I. Aroso, M. Martins, R. L. Reis and A. R. C. Duarte, ACS Sustainable Chem. Eng., 2014, 2, 1063 CrossRef CAS;
(h) D. V. Wagle, H. Zhao and G. A. Baker, Acc. Chem. Res., 2014, 47, 2299 CrossRef CAS;
(i) E. L. Smith, A. P. Abbott and K. S. Ryder, Chem. Rev., 2014, 114, 11060 CrossRef CAS;
(j) J. García-Álvarez, E. Hevia and V. Capriati, Chem. – Eur. J., 2018, 24, 14854 CrossRef;
(k)
Deep Eutectic Solvents: Synthesis, Properties, and Applications, ed. D. J. Ramón, G. Guillena, Wiley-VCH, Weinheim, Germany, 2019 Search PubMed;
(l) F. M. Perna, P. Vitale and V. Capriati, Curr. Opin. Green Sust. Chem., 2020, 21, 27 Search PubMed. For recent works dealing with the use of DESs in organic synthesis see:
(m) J. Xu, W. Huang, R. Bai, Y. Queneau, F. Jérôme and Y. Gu, Green Chem., 2019, 21, 2061 RSC;
(n) Z. Cao, Q. Zhu, Y.-W. Lin and W.-M. He, Chin. Chem. Lett., 2019, 30, 2132 CrossRef CAS;
(o) M. Li, F. Wu and Y. Gu, Chin. J. Catal., 2019, 40, 1135 CrossRef CAS;
(p) P. Vitale, F. Lavolpe, F. Valerio, M. Di Biase, F. M. Perna, E. Messina, G. Agrimi, I. Pisano and V. Capriati, React. Chem. Eng., 2020, 5, 859 RSC;
(q)
F. M. Perna, P. Vitale and V. Capriati, Organic Synthesis in DESs, in Deep Eutectic Solvents: Synthesis, Properties, and Applications, ed. D. J. Ramòn and G. Guillena, Wiley-VCH, Weinheim, Germany, 2019 Search PubMed. For recent use of DESs in solar technology, photosynthesis and electrochemistry, see:
(r) C. L. Boldrini, N. Manfredi, F. M. Perna, V. Trifiletti, V. Capriati and A. Abbotto, Energy Technol., 2017, 5, 345 CrossRef CAS;
(s) C. L. Boldrini, N. Manfredi, F. M. Perna, V. Capriati and A. Abbotto, Chem. – Eur. J., 2018, 24, 17656 CrossRef CAS;
(t) C. L. Boldrini, N. Manfredi, F. M. Perna, V. Capriati and A. Abbotto, ChemElectroChem, 2020, 7, 1707 CrossRef CAS;
(u) F. Milano, L. Giotta, M. R. Guascito, A. Agostiano, S. Sblendorio, L. Valli, F. M. Perna, L. Cicco, M. Trotta and V. Capriati, ACS Sustainable Chem. Eng., 2017, 5, 7768 CrossRef CAS;
(v) L. Millia, V. Dall'Asta, C. Ferrara, V. Berbenni, E. Quartarone, F. M. Perna, V. Capriati and P. Mustarelli, Solid State Ionics, 2018, 323, 44 CrossRef CAS.
-
(a) G. Imperato, S. Höger, D. Lenoir and B. König, Green Chem., 2006, 8, 1051 RSC;
(b) G. Imperato, R. Vasold and B. König, Adv. Synth. Catal., 2006, 348, 2243 CrossRef CAS;
(c) F. Illgen and B. König, Green Chem., 2009, 11, 848 RSC;
(d) X. Marset, A. Khoshnood, L. Sotorríos, E. Gómez-Bengoa, D. A. Alonso and D. J. Ramón, ChemCatChem, 2017, 9, 1269 CrossRef CAS;
(e) X. Marset, S. De Gea, G. Guillena and D. J. Ramón, ACS Sustainable Chem. Eng., 2018, 6, 5743 CrossRef CAS;
(f) B. Saavedra, N. González-Gallardo, A. Meli and D. J. Ramón, Adv. Synth. Catal., 2019, 361, 3868 CrossRef CAS;
(g) S. E. Hooshmand, R. Afshari, D. J. Ramón and R. S. Varma, Green Chem., 2020, 22, 3668 RSC;
(h) L. Cicco, G. Dilauro, F. M. Perna, P. Vitale and V. Capriati, Org. Biomol. Chem., 2021 10.1039/D0OB02491K.
- A. Shaabani and R. Afshari, J. Colloid Interface Sci., 2018, 510, 384 CrossRef CAS.
- A. F. Quivelli, P. Vitale, F. M. Perna and V. Capriati, Front. Chem., 2019, 7, 723 CrossRef CAS.
- For recent examples on Cu-catalyzed Goldberg type C–N couplings in either volatile organic solvents or water, see:
(a) A. Klapars, X. Huang and S. L. Buchwald, J. Am. Chem. Soc., 2002, 124, 7421 CrossRef CAS;
(b) Y. Z. Huang, J. Gao, H. Ma, H. Miao and J. Xu, Tetrahedron Lett., 2008, 49, 948 CrossRef CAS;
(c) Z. Q. Zhu, S. Xiang, Q. Y. Chen, C. Chen, Z. Zeng, Y. P. Cui and J. C. Xiao, Chem. Commun., 2008, 5016 RSC;
(d) B. Sreedhar, R. Arundhati, P. L. Reddy and M. L. Kantam, J. Org. Chem., 2009, 74, 7951 CrossRef CAS;
(e) S. Jammi, S. Sakthivel, L. Rout, T. Mukherjee, S. Mandal, R. Mitra, P. Saha and T. Punniamurthy, J. Org. Chem., 2009, 74, 1971 CrossRef CAS;
(f) C. T. Yang, Y. Fu, Y. B. Huang, J. Yi, Q. X. Guo and L. Liu, Angew. Chem., Int. Ed., 2009, 48, 7398 CrossRef CAS;
(g) K. Swapna, S. N. Murthy and Y. V. D. Nageswar, Eur. J. Org. Chem., 2010, 6678 CrossRef CAS;
(h) A. M. Thomas and G. Anilkumar, Mini-Rev. Org. Chem., 2015, 12, 3 CrossRef CAS;
(i) M. Bollenbach, P. G. V. Aquino, J. X. de Araújo-Júnior, J.-J. Bourguignon, F. Bihel, C. Salomé, P. Wagner and M. Schmitt, Chem. – Eur. J., 2017, 23, 13676 CrossRef CAS.
-
(a) A. Pandey, R. Rai, M. Pal and S. Pandey, Phys. Chem. Chem. Phys., 2014, 16, 1559 RSC;
(b)
J. García-Álvarez, Deep Eutectic Solvents and Their Applications as New Green and Biorenewable Reaction Media, in Handbook of Solvents, Vol. 2: Use, Health, and Environment, ed. G. Wypych, ChemTec Publishing, Toronto, Canada, 3rd edn, 2019 Search PubMed.
- For more information, see ESI.†.
- Ultrasonic irradiation has been previously employed to improve the yield/reaction time in organic transformations performed in DESs. For a recent example see: C. Wu, H.-J. Xiao, S.-W. Wang, M.-S. Tang, Z.-L. Tang, W. Xia, W.-F. Li, Z. Cao and W.-M. He, ACS Sustainable Chem. Eng., 2019, 7, 2169 CrossRef CAS.
- In modern organic synthesis, catalysis is considered as a key concept for sustainability; see:
G. Rothenberg, Catalysis, Wiley-VCH, Weinheim, Germany, 2008 Search PubMed.
-
(a)
Catalyst Separation, Recovery and Recycling. Chemistry and Process Design ed. D. Cole-Hamilton and R. Tooze, Springer, Dordrecht, The Netherlands, 2006 Search PubMed;
(b)
Recoverable and Recyclable Catalyst ed. M. Benaglia, Wiley, Chichester, UK, 2009 Search PubMed.
- U. Azzena, M. Carraro, L. Pisano, S. Monticelli, R. Bartolotta and V. Pace, ChemSusChem, 2019, 12, 40 CrossRef CAS.
- R. A. Sheldon, Green Chem., 2007, 9, 1273 RSC.
- For more details on the E-factor calculation, see ESI.†.
- Cyclooxygenase-1 (COX-1) is an emerging target for prevention and treatment of atherosclerosis and ovarian epithelial cancer:
(a) T. Daikoku, D. Wang, S. Tranguch, J. D. Morrow, S. Orsulic, R. N. DuBois and S. K. Dey, Cancer Res., 2005, 65, 3735 CrossRef CAS;
(b) P. Vitale, A. Panella, A. Scilimati and M. G. Perrone, Med. Res. Rev., 2016, 36, 641 CrossRef CAS;
(c) P. Malerba, B. C. Crews, K. Ghebreselasie, C. K. Daniel, E. Jashim, A. M. Aleem, R. A. Salam, L. J. Marnett and Md. J. Uddin, ACS Med. Chem. Lett., 2020, 11, 1837 CrossRef CAS. Although the inhibition of COX-1 is involved in gastrointestinal side effects of nonsteroidal anti-inflammatory drugs (NSAIDs), some N-arylbenzamides exhibit strong analgesic effects as COX-1-selective inhibitors without causing gastric damage; see:
(d) H. Kakuta, X. Zheng, H. Oda, S. Harada, Y. Sugimoto, K. Sasaki and A. Tai, J. Med. Chem., 2008, 51, 2400 CrossRef CAS.
- The Fundación BBVA accepts no responsibility for the opinions, statements and contents included in the project and/or the results thereof, which are entirely the responsibility of the authors.
Footnotes |
† Dedicated to Professor Robert E. Mulvey on the occasion of his 60th birthday. A truly inspiring pioneer and model for mixed-metal main-group organometallic chemists worldwide. |
‡ Electronic supplementary information (ESI) available: General experimental procedures, characterization data and NMR spectra for obtained compounds (PDF). See DOI: 10.1039/d0ob02501a |
|
This journal is © The Royal Society of Chemistry 2021 |